DOI:
10.1039/D3QI00102D
(Research Article)
Inorg. Chem. Front., 2023,
10, 2626-2635
Introducing anthracene and amino groups into Ln-OFs for the photoreduction of Cr(VI) without additional photosensitizers or cocatalysts†
Received
15th January 2023
, Accepted 22nd March 2023
First published on 4th April 2023
Abstract
The utilization of catalysts with high stability and activity for the photoreduction of Cr(VI) is desirable but has many challenges. Since metal–organic frameworks (MOFs) have unique versatilities and facile structural modulations, we fabricated a stable MOF as a highly efficient photocatalyst by incorporating chromophores into lanthanide MOFs for efficient Cr(VI) reduction. Subsequently, a novel two-dimensional (2D) layered structural Sm(III) MOF (namely LCUH-100, [Sm4(AAPA)6(DMA)2(H2O)4][DMA]3[H2O]8, AAPA2− = 5-[(anthracen-9-yl-methyl)-amino]-1,3-isophthalate, DMA = N,N′-dimethylacetamide) with sql topology symbol was constructed by incorporating dinuclear clusters, {Sm2(COO)6} and AAPA2−, which demonstrated excellent chemical and thermal stability, especially retaining its complete framework in aqueous solutions at a pH range of 2–12 for 24 h. Interestingly, LCUH-100 could efficiently and rapidly photocatalyze the reduction of Cr(VI) to Cr(III) without additional photosensitizers or cocatalysts. In the photoreduction of Cr(VI), LCUH-100 showed a high rate constant (k) of 0.186 min−1 and a high Cr(VI) reduction rate of 1.67 mgCr(VI) g−1cata min−1 at pH 2. Furthermore, the photocatalyst LCUH-100 could be reused for 5 cycles without any significant loss of catalytic activity. This work provides a new strategy for the exploitation of stable and efficient catalysts for the photocatalytic reduction of Cr(VI).
Introduction
Environmental issues are among the major issues facing the whole world today, including water contamination, which has caused wide public concern over the recent years.1–4 Heavy metal ion pollution (i.e., Cr(VI), Hg(II), and Fe(III)) is a significant global problem.5–9 Especially, dichromate (Cr2O72−) ions are widely regarded as representative contaminant in water due to their high toxicity and carcinogenicity.10–12 Therefore, the concentration of Cr(VI) in drinking water has been limited to below 0.05 mg L−1 by the World Health Organization.13 Recently, many approaches have been exploited to efficiently adsorb and convert Cr(VI) into less toxic Cr ions.14–16 In particular, the conversion of Cr(VI) into less toxic Cr(III) is regarded as a promising solution to alleviate the pollution problem. Since photocatalytic reduction is an eco-friendly method, solar-energy-driven Cr2O72− ion reduction under the action of photocatalysts into Cr(III) has been extensively investigated.17–19 Some typical inorganic photocatalysts with multifunctional characteristics have been reported by scientific researchers, such as TiO2, α-Fe2O3, In2S3, and ZnO.20–24 However, there are some shortcomings, such as the electron–hole pair recombination rate, fast agglomeration speed, and low solar energy utilization, which limit the efficiency of the above catalysts for Cr(VI) removal.25–27 Therefore, the development of efficient photocatalysts to adsorb and convert Cr(VI) into a less toxic product Cr(III) is highly desired.
In recent years, diverse MOF catalysts have been exploited for Cr(VI) photoreduction.28–34 Compared with three-dimensional (3D) MOFs, 2D MOFs have unique properties originating from their ultrathin thickness, large surface area, excellent optical transparency, and superior electrical and thermal conductivity, which facilitate a more efficient transport of photogenerated carriers from the interior to the surface active sites. Therefore, 2D MOFs are considered as more suitable photocatalyst candidates.35–40 However, to the best of our knowledge, only a few articles have reported successful Cr(VI) photocatalytic reduction using 2D MOFs.41,42 Meanwhile, chemists have found that 2D MOFs can be used as semiconductor materials, whereby the highest occupied molecular orbital (HOMO)–lowest unoccupied molecular orbital (LUMO) gap of the organic ligand in MOF systems is equal to the band gap of inorganic semiconductors, so it makes these photocatalysts possess the capabilities for fine-tuning and rational design at the molecular level.43,44 For example, as the porphyrin unit is a typical photosensitizer, Ye's group successfully modified UiO-66 by introducing a porphyrin ring into the original UiO-66, and the photoreduction activity of the modified UiO-66 was much higher than that of the original UiO-66.45 Wu's group found that the photoreduction activity of MIL-68(In)-NH2 was higher than the unmodified MIL-68(In), which indicated that adding amino groups in BDC2− ligands could broaden the absorption edge to the visible region.46 Therefore, an intense visible-light-absorption π-electron-rich aromatic unit and strong toxic anions adsorbent were integrated into one single MOF simultaneously to strongly improve the photoreduction capability toward Cr(VI). However, the photocatalysts obtained by the MOFs post-modification method do not have unambiguous structures, and their synthetic process is also more complicated. Based on the above considerations, we designed and obtained the 2D Sm-MOF LCUH-100 using the ligand H2AAPA containing anthracyclines and amino groups as photosensitizers, and this LCUH-100 demonstrated excellent reactivity and good reproducibility for the reduction of Cr(VI) in water. As far as we know, this is the first example of a 2D lanthanide–organic frameworks (Ln-OFs) material among all the reported MOF-based photocatalysts for Cr(VI) photoreduction.
Herein, we rationally synthesized a stable Sm carboxylate MOF (LCUH-100) by using Sm(III) ions and the large conjugated dicarboxylate ligand H2AAPA with amino groups (Scheme 1), which could broaden the absorption edge to the visible region. Notably, LCUH-100 demonstrated excellent chemical and thermal stability, especially retaining its complete framework in aqueous solution at pH 2–12 for 24 h. Due to the introduction of highly photosensitive anthracene units and the cochromatic amino group, LCUH-100 could achieve the complete conversion of Cr(VI) to Cr(III) within 60 min under visible-light irradiation without the need for any photosensitizer or cocatalyst. The significant photocatalytic activity of LCUH-100 could be ascribed to its rich Lewis acidic Sm(III) sites, large conjugated dicarboxylate ligands, amino groups, and good charge-separation efficiency. Meanwhile, various factors affecting the reduction of Cr(VI), including the pH value of the reaction solution, the addition of a hole scavenger, and the reusability of the photocatalyst, were also studied in detail.
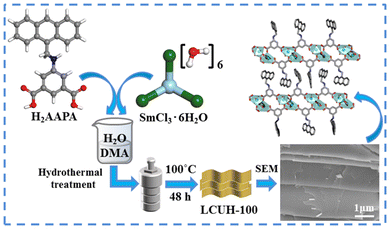 |
| Scheme 1 Schematic illustration of the synthesis processes of LCUH-100. | |
Results and discussion
Structure and morphology description of LCUH-100
As shown in Table S1,†LCUH-100 crystallized in a triclinic crystal system with space group P
, displaying a two-dimensional (2D) framework based on the AAPA2− ligand and binuclear metallic [Sm2(COO)6] cluster. In the asymmetric unit of LCUH-100, there are four independent Sm(III) ions, six deprotonated AAPA2− ligands, four coordinated H2O molecules, and two coordinated organic solvent molecules (DMA), taking no account of the free solvent molecules. The four independent Sm(III) ions in the asymmetric unit can form two different binuclear metal clusters, namely [Sm2(COO)6]a and [Sm2(COO)6]b. Four oxygen atoms (O5, O6, O16, O17) of one ligand link the binuclear clusters [Sm2(COO)6]a and [Sm2(COO)6]b (Fig. 1a). In the binuclear cluster [Sm2(COO)6]a, Sm1 is a cation octa-coordinated by eight carboxylate oxygen atoms from five different deprotonated AAPA2− ligands, displaying a distorted dodecahedral geometry [Sm1–O5 = 2.32(8) Å, Sm1–O8 = 2.34(8) Å, Sm1–O4 = 2.36(8) Å, Sm1–O2 = 2.41(10) Å, Table S2†]. The Sm2 center is nine-coordinated and composed of six carboxylate oxygen atoms from four different AAPA2−, one oxygen atom from the coordinated DMA, and two oxygen atoms from two H2O molecules [Sm2–O8 = 2.36(11) Å, Sm2–O3 = 2.41(8) Å, Sm2–O6 = 2.46(8) Å, Sm2–O7 = 2.46(8) Å, Table S2†]. The distance between Sm1 and Sm2 is 4.15 Å, and they are linked by two carboxylate oxygen atoms (O3 and O8) coming from two different AAPA2− ligands to form a binuclear metallic cluster [Sm2(COO)6]a (Fig. 1b).
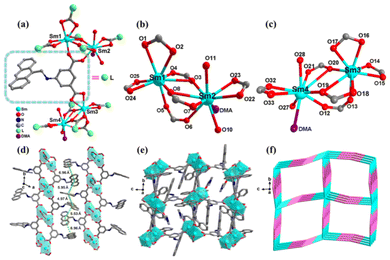 |
| Fig. 1 (a) Simplified coordination environments of LCUH-100 (green spheres represent H2AAPA). Coordination structures of binuclear [Sm2(COO)6]a (b) and binuclear [Sm2(COO)6]b (c). (d) Lamellar structure of the 2D framework viewed from the c axis. (e) Reticular structure viewed from the a axis in LCUH-100. (f) Simplified topology structure of LCUH-100. | |
In another binuclear cluster [Sm2(COO)6]b, the Sm3 center is octa-coordinated by eight oxygen atoms from five different AAPA2− ligands, and displays a distorted dodecahedral geometry [Sm3–O13 = 2.35(7) Å, Sm3–O20 = 2.36(8) Å, Sm3–O18 = 2.39(7) Å, Sm3–O142 = 2.41(8) Å] (Table S2†). The Sm4 center is nine-coordinated by nine oxygen atoms and constituted by six carboxylate oxygen atoms from four different AAPA2− ligands, one oxygen atom from one DMA, and two oxygen atoms from two H2O molecules [Sm4–O26 = 2.37(9) Å, Sm4–O19 = 2.41(8) Å, Sm4–O12 = 2.42(8) Å, Sm4–O21 = 2.48(8) Å. Table S2†]. The distance between Sm3 and Sm4 is 4.12 Å, and the adjacent two Sm(III) ions are connected by two carboxylate oxygen atoms (O19 and O20) from two different AAPA2− ligands (Fig. 1c). The adjacent binuclear metal clusters are linked through the deprotonated ligand AAPA2− and form 2D layered reticular structures (Fig. 1e). The distance between adjacent layers is in the range of 4.97–6.96 Å, so the adjacent 2D layers can be further connected to form 3D frameworks by the π⋯π stacking and hydrogen-bonding interactions (Fig. 1d). The framework of LCUH-100 can be simplified to a 4-connected network with the sql topology of point symbol {44·62} by the topological analysis method (Fig. 1f). After removing guest molecules, the total solvent-accessible volume of LCUH-100 was assessed to be 2043.2 Å3, or 25.8% of the unit volume 7914.1 Å3 calculated through PLATON.
The morphology and microstructure of the prepared LCUH-100 were studied by field emission scanning electron microscopy (FESEM) and transmission electron microscopy (TEM), as shown in Fig. 2. Given the 2D crystal structure of LCUH-100, its FESEM images showed a typical layered appearance of 30–300 nm thickness (Fig. 2a). The morphology of LCUH-100 was further observed by TEM (Fig. 2b), and the same 2D layered structure model was shown, which proved that we successfully prepared LCUH-100 with a 2D layered structure.
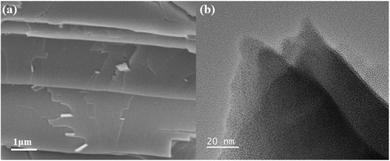 |
| Fig. 2 FESEM (a) and TEM (b) images of LCUH-100. | |
Stability test
The powder X-ray diffraction (PXRD) patterns of LCUH-100 indicated a good match between the simulated and as-synthesized LCUH-100 in key positions, which confirmed the purity of the samples of LCUH-100. To inspect the chemical stability of LCUH-100, it was soaked in deionized water (pH 7), hydrochloric solution (pH = 2, 4, 6), and sodium hydroxide solution (pH = 8, 10, 12) at 298 K for 24 h, respectively. After the above soaking treatment, the PXRD patterns of the treated LCUH-100 matched well with that of the simulated LCUH-100 in key positions (Fig. 3a), indicating LCUH-100 had excellent chemical stability, and ensure it can be used in further applications. Furthermore, the thermostability of MOFs is a significant element for their better application in industrial production. As shown in Fig. 3b, the thermogravimetric analysis indicated that LCUH-100 had excellent thermostability and was thermally stable at 303 °C. Eight lattice water molecules were gradually lost in the range of 30–100 °C, corresponding to a 4.20% weight loss (ca. 4.15%). The further weight loss of 2.10% (ca. 2.08%) at 100–170 °C corresponded with the loss of four coordinated water molecules, and then at even higher temperature, the skeleton of LCUH-100 began to collapse after 303 °C. In addition, LCUH-100 was tested with variable temperature powders at 25–325 °C to further verify its stability, and the test results are displayed in the ESI, as shown in Fig. S2.†
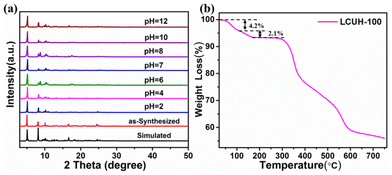 |
| Fig. 3 (a) Simulated and synthetic PXRD patterns of LCUH-100, as well as the PXRD patterns of LCUH-100 immersed in aqueous solutions of different pH values for 24 h; (b) thermogravimetric analysis (TGA) curves of LCUH-100 measured under a N2 atmosphere from 30 °C to 750 °C at a heating rate of 10 °C min−1. | |
Photocatalytic reduction of Cr(VI)
Photocatalytic properties.
In the photocatalytic reaction, MOFs experience a process similar to that of traditional semiconductors. Note, the valence band (VB) and conduction band (CB) refer to the highest occupied molecular orbital (HOMO) and the lowest unoccupied molecular orbital (LUMO) in MOFs, respectively. The ultraviolet-visible-absorption band of LCUH-100 was measured by ultraviolet–visible-light diffuse reflectance spectroscopy (UV–vis DRS). As displayed in Fig. 4a, the strong visible-light absorption and broad range absorption band (300–500 nm) make LCUH-100 have potential application in visible-light photocatalysis.47 The UV region (300–400 nm) absorption band was ascribed to the π⋯π* and n⋯π* transitions of the aromatic rings (An) and amino groups (–NH–) in the organic ligand AAPA2− of LCUH-100. While the visible region (400–550 nm) absorption band could be ascribed to the charge-transfer transition from the ligand AAPA2− to the {Sm2} cluster (LMCT).48 The band-gap energy (Eg) of LCUH-100 could be calculated based on the Kubelka–Munk equation: αhv = B(hν − Eg)1/2.49 The Eg of LCUH-100 was estimated to be about 2.85 eV by linear fitting the curve of αhv2versus the photon energy (hν) (Fig. 4a). Therefore, the above DRS research endorsed the utilization of LCUH-100 as a visible-light-active photocatalyst. To further investigate the band structure of LCUH-100, the semiconductor type and band potential (generally considered to be the Fermi level (Ef)) were determined by Mott–Schottky electrochemical tests. As shown in Fig. 4b, the slopes of the Mott–Schottky plots of LCUH-100 (i.e., 1000, 1300, and 1600 Hz) were all positive, indicating that LCUH-100 can be regarded as an N-type characteristic material, and the Ef value was Ef (vs. Ag/AgCl) −1.37 eV, at pH 6.8. This could be converted into reversible hydrogen electrode (RHE) potential by the following formula: ERHE = EAg/AgCl + 0.198 eV + 0.0592 pH, whereby the Ef value of LCUH-100 was −0.77 eV (vs. RHE). Furthermore, the gap between Ef and VB obtained from the XPS VB spectra was 2.51 eV (vs. NHE) (Fig. 4c). The VB of LCUH-100 was determined to be 1.74 eV (vs. NHE). Combined with the band-gap values obtained above, the CB of LCUH-100 was calculated to be −1.11 eV (vs. NHE) (Table S5†), which was more negative than the Cr(VI)/Cr(III) potential (+0.51 eV, pH 6.8). Therefore, LCUH-100 is a potential catalyst for the photocatalytic reduction of Cr(VI). As shown in Fig. 4d, based on the above analysis, the energy band diagram of photocatalyst LCUH-100 was also schematically plotted.43,50,51
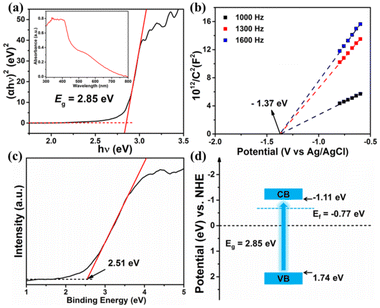 |
| Fig. 4 (a) UV–vis spectra of LCUH-100 and plot of (αhν)2versus photon energy (hν) of LCUH-100. Typical Mott–Schottky plots (b) and XPS VB spectra (c) of LCUH-100. (d) Band structure diagram of LCUH-100. | |
The separation efficiency of photogenerated charge carriers in LCUH-100 was then investigated by transient photocurrent measurements, electrochemical impedance spectroscopy (EIS), and steady-state photoluminescence (steady-state PL). Fig. 5a shows the apparent photocurrent response (0.5 μA cm−2) of the LCUH-100 electrodes over on–off cycles of intermittent visible-light irradiation. This proves that LCUH-100 was able to be stimulated to produce electron–hole pairs under visible irradiation.52 The semicircle in the EIS spectra was due to the contribution from the charge-transfer resistance (Rct) and constant phase element (CPE). The Rct should result from the resistance at the interface between the photocatalyst/electrolyte interfaces. The corresponding equivalent circuit is shown in the inset of Fig. 5b. The radius of the EIS arc is related to the charge-transfer resistance of a photocatalyst, and a smaller radius of the EIS arc corresponds to a smaller resistance. According to the EIS results, the Rct of LCUH-100 was 2.7 kΩ, which was smaller than the Rct of the photocatalysts reported in the literature. It is clear that LCUH-100 had less charge-transfer resistance (for example, the Rct of H2TCPP⊂(I−) Meim-UiO-66 reported by Ye's group was 5.47 kΩ), which can make the photoexciton migration more effective.10,15,16,23,43,45,50,51 This finding could be further confirmed by the steady-state PL spectrum (Fig. S3†). Interestingly, the PL intensity obtained over LCUH-100 was much weaker than that of H2AAPA, thus indicating the faster electron transfer of the photogenerated electrons and holes after forming the MOF material.45,50,51
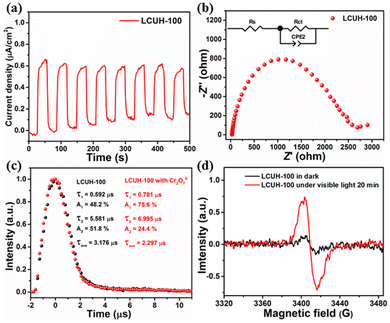 |
| Fig. 5 Transient photocurrent responses (a), electrochemical impedance spectroscopy plot (b) of LCUH-100, time-resolved PL spectra of LCUH-100 and LCUH-100 containing Cr2O72− suspensions (c), and of LCUH-100, (d) EPR analyses of LCUH-100 before and after visible-light irradiation. | |
Meanwhile, the time-resolved PL spectrum of LCUH-100 is shown in Fig. 5c. The average recovery lifetime (τ) of LCUH-100 was 3.176 μs. It is well known that prolongation of the exciton lifetime can provide more opportunities for photoinduced carriers to participate in surface-catalyzed reactions, thus improving the photocatalytic performance.45,50 The electron-transfer process of the photocatalysts was further studied by electron paramagnetic resonance (EPR), which was carried out in the dark and under visible-light irradiation, respectively (Fig. 5d). After 20 min visible-light irradiation, the EPR signal at about g = 2.002 was significantly enhanced, indicating that the photogenerated electrons transferred from the excited anthracene ring to the Sm–O cluster site.43,45 However, when the other conditions were identical, no obvious signal was observed in the dark.
Photocatalytic reduction of Cr(VI) and its influencing factors.
The excellent physical properties, strong visible-light adsorption and high electron–hole separation efficiency encouraged us to assess the feasibility of LCUH-100 as a photocatalyst for Cr(VI) reduction. A negligible decrease in Cr(VI) was clearly observed in the presence of LCUH-100 in the dark conditions, which indicated that the decline in the Cr(VI) concentration was not caused by adsorption. Whereas in the presence of light and the catalyst LCUH-100, the reduction process of Cr(VI) proceeded smoothly, and a significant decline in Cr(VI) concentration was observed, indicating that the reduction of Cr(VI) was caused by LCUH-100 under visible-light irradiation. The Cr(VI) aqueous solution (50 ppm, 20 mL) could be 100% reduced within 60 min under the optimal conditions (pH 2, 3.0 mL EtOH) (Fig. 6a). As shown in Fig. 6b, the photoreduction of Cr(VI) followed a pseudo-first-order kinetics, and the reaction rate was calculated to be 0.1861 min−1 under the optimal conditions.45 Compared with other reported MOF-related photocatalysts, the reduction efficiency of LCUH-100 was at a high level for the reduction of Cr(VI) (Table 1). Moreover, the recycling tests indicated that LCUH-100 had excellent recyclable stability, as identified by the PXRD patterns, IR spectra, and XPS spectra before and after the photoreduction of Cr(VI) (Fig. 6f and S4†). However, the rate of the photocatalytic reduction reaction was strongly affected by some factors, such as the pH, hole scavenger, and electron-capture agent.49
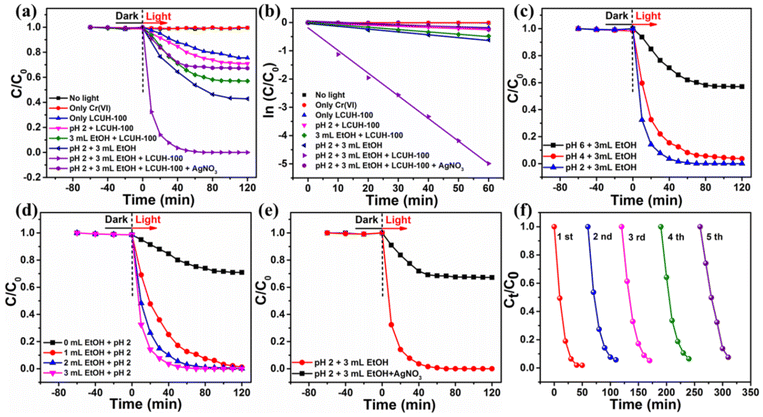 |
| Fig. 6 Photocatalytic reduction of Cr(VI) to Cr(III): (a) under different conditions for Cr(VI) reduction over LCUH-100 (pH 2, 3.0 mL of ethanol) and its pseudo-first-order kinetics curves (b); (c) with different pH in the presence of 3 mL ethanol; (d) with different amounts of EtOH at pH 2; (e) visible-light-driven photocatalytic Cr(VI) reduction of LCUH-100 without (black curve) or with (red curve) the electron scavenger AgNO3 (1 mg). (f) Cycling runs for the photocatalytic reduction of Cr(VI) over LCUH-100. | |
Table 1 Comparison of the photocatalytic reduction of Cr(VI) by different photocatalysts
Photocatalyst |
C
cata (g L−1) |
V
Cr(VI) (mL); CCr(VI) (ppm) |
Reduction percentage; time (min) |
Reduction rate (mgCr(VI) g−1cata min−1) |
k (min−1) |
Light source |
Ref. |
In2S3@MIL-53(Fe) |
0.60 |
50; 10 |
97%; 20 |
0.81 |
0.143 |
CEL-LAX 500 (300 W) |
10
|
Cd-MOF |
1.00 |
30; 20 |
95%; 180 |
0.11 |
— |
LED lamp (40 W) |
25
|
Cu(I)-MOF |
0.38 |
40; 10 |
95%; 9 |
2.81 |
— |
Xenon lamp (500 W) |
42
|
Ru-UiO-bpy |
0.25 |
40; 50 |
100%; 60 |
3.3 |
0.128 |
PLS-SXE300 (300 W) |
43
|
NH2-MIL-125-Ti |
0.40 |
50; 48 |
91%; 60 |
1.82 |
— |
Xenon lamp (500 W) |
44
|
H2TCPP⊂(I−)Meim-UiO-66 |
0.25 |
40; 100 |
100%; 30 |
13.3 |
0.154 |
Xenon lamp (300 W) |
45
|
MIL-68(In)-NH2 |
1.00 |
40; 20 |
97%; 180 |
0.11 |
— |
PLS-SXE300 (300 W) |
46
|
Zn-MOF |
1.00 |
40; 20 |
93%; 90 |
0.21 |
— |
Xenon lamp (300 W) |
47
|
Zr-MOF |
0.25 |
40; 50 |
73%; 60 |
3.33 |
0.073 |
PLS-SXE300 (300 W) |
49
|
LCUH-100
|
0.50 |
20; 50 |
100%; 60 |
1.67 |
0.186 |
CEL-LAX 500 (300 W) |
This work |
During the redox process, the pH value of the reaction solution had a great influence on the reduction rate, which was in agreement with that of other reported MOF catalysts.10,44 Hence, the effect of pH on the photocatalytic performance was investigated under the concentration of 50 ppm K2Cr2O7 solution (20 mL) and 10 mg of the catalyst LCUH-100. The reduction ratio increased rapidly from 41% to 100% with the pH decreasing from 6.0 to 2.0 (Fig. 6c). According to the surface zeta potential versus the pH of LCUH-100, the isoelectric point was about 2.56, which indicated that the photocatalyst surface was positive at the range of pH < 2.56 (Fig. S5†). Therefore, under the condition of pH 2, the positively charged photocatalyst LCUH-100 was more likely to attract Cr2O72− anions and accelerate the electron migration, thus promoting the photocatalytic activity. On the contrary, the significantly less reduction at higher pH (pH > 2.56) could be attributed to the highly anionic charged surface of the photocatalyst, which repelled the Cr2O72− anions.
As is well known, the rate of photocatalytic reduction is greatly influenced by the presence of hole scavengers. The addition of a hole scavenger was thus used to trigger the reduction process, as it would react with the photoinduced holes. Herein, EtOH was investigated as a hole scavenger in the reaction, which could further promote the photocatalytic activities. As expected, as the amount of EtOH increased from 0 to 3 mL, the reduction rate increased rapidly from 23% to 100% (Fig. 6d). Therefore, EtOH could be beneficial to restrain the recombination of electrons and holes, which could afford sufficient electrons would be available for Cr(VI) reduction.49
The above experiments confirmed that the introduction of the hole (h+)-scavenger EtOH could accelerate the reduction efficiency of Cr(VI). Herein, AgNO3, as an electron scavenger, was introduced into the photocatalytic reduction process. As shown in Fig. 6e, the Cr(VI) reduction efficiency was obviously restrained by the solution of AgNO3, indicating that the electron e− had a dominant influence on the reduction process. The trapping of holes by EtOH may accelerate the migration of electrons e− and make them fully participate in the reduction process, thereby finally improving the photocatalytic activity.10
Mechanism of photocatalytic Cr(VI) reduction
To investigate the electron-transfer process in the photocatalyst during visible-light irradiation, we then resorted to time-resolved PL spectroscopy, a robust tool to track photoexcited carriers. The τ of LCUH-100 in Fig. 5c was 3.176 μs. After the addition of Cr2O72−, the average lifetime was reduced to 2.297 μs, indicating that the long-lived electrons generated by LCUH-100 were easily transferred to Cr2O72−.45,50 Meanwhile, EPR tests were performed to directly probe the photogenerated electrons in the reaction system. Here, 2,2,6,6-tetramethyl-l-piperidine-N-oxyl (TEMPO), as a typical spin label molecule, was utilized for capturing photogenerated electrons (e−) on the surface of LCUH-100 (Fig. 7a). The typical three signals of TEMPO with equal intensity were observed in the dark, but after visible-light irradiation for 20 min, the signal intensity dramatically declined. This could be ascribed to the TEMPO molecule being transformed into the corresponding hydroxylamine (TEMPOH) in aqueous system by trapping electrons.53 The electrons e− produced from the photoexcited LCUH-100 were captured by TEMPO, which led to a weakening of the signal. Similarly, it was found that there was no obvious electronic signal after 20 min of visible-light irradiation when TEMPO molecules were replaced with Cr2O72−. The EPR data proved that the electrons e− generated by photoexcited LCUH-100 were captured by Cr(VI) and then reduced to Cr(III), and then Cr2O72− ions were completely converted into Cr(III) ions under the irradiation of visible light, which was also proved by the XPS data of LCUH-100. As shown in Fig. 7b, the binding energies of Cr 2p3/2 and Cr 2p1/2 of Cr(III) corresponded to 578.0 and 587.3 eV, respectively.
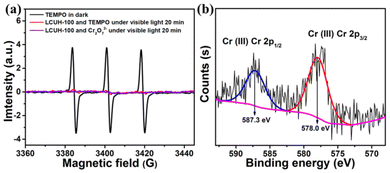 |
| Fig. 7 (a) EPR signal of TEMPO in the dark, and TEMPO and Cr2O72− radicals under visible light in aqueous dispersion of LCUH-100 for 20 min; (b) XPS spectra of the recovered LCUH-100 after the Cr(VI) reduction experiment. | |
According to the above experimental findings, a plausible mechanism is proposed for the reduction of Cr(VI) (Scheme 2). In this process, the Sm–O clusters surrounded by H2AAPA ligands in the framework of LCUH-100 can behave as quantum dots, while the –NH– groups act as auxochromic groups, and the anthracene nucleus acts as an antennae absorbing light. Upon irradiation with visible light, the anthracene nucleus in LCUH-100 strongly absorbs visible light and efficiently transfers the photogenerated electrons to the inorganic Sm–O clusters partly via ligand-to-metal charge transfer (LMCT) (eqn (1)). The electrons on the valence band leap into the conduction band and leave behind holes in the valence band, and then the electrons on CB are accepted by Cr2O72− ions adsorbed on the metal sites (eqn (2)). Meanwhile, the photogenerated holes can get enough electrons from the hole scavenger EtOH adsorbed on the amine sites to oxidize EtOH into CO2 and H2O (eqn (3)).43,46 Therefore, the redox process can be outlined as follows:
| Sm-MOF + hν → Sm-MOF (h+ + e−) | (1) |
| Cr2O72− + 14H+ + 6e− → 2Cr3+ + 7H2O | (2) |
| EtOH + h+ → CO2 + H2O + Others. | (3) |
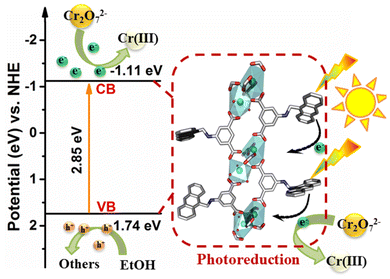 |
| Scheme 2 Proposed mechanism for the photocatalytic reduction of aqueous Cr(VI). | |
Conclusions
In summary, a 2D layered Sm-MOF, LCUH-100, with a good lattice and excellent stability was successfully prepared by a solvothermal method. The visible-light-absorption edge of LCUH-100 could be extended to 550 nm by combining an anthracene group and amino group into the MOF framework, thus showing efficient Cr(VI) photocatalytic reduction performance. Remarkably, the photocatalytic reduction of Cr(VI) by LCUH-100 did not require the aid of any photosensitizer or cocatalyst under visible-light irradiation, which is quite rare among reported MOF-based photocatalysts. LCUH-100 showed a high rate constant (k) of 0.186 min−1 and a high Cr(VI) photoreduction rate of 1.67 mgCr(VI) g−1cata min−1 for the photoreduction of Cr(VI), which are higher than most reported representative MOFs. In addition, LCUH-100 demonstrated excellent photocatalytic reduction stability (5 recycling runs), making LCUH-100 an outstanding candidate for the photocatalytic reduction of Cr(VI). This work provides a new method for the exploitation of stable and efficient catalysts for the photocatalytic reduction of Cr(VI).
Experimental section
Synthesis procedure
Synthesis of {[Sm4(AAPA)6(DMA)2(H2O)4]·DMA3·H2O8}n (LCUH-100).
A mixture of SmCl3·6H2O (36.48 mg, 0.1 mmol), 5-[(anthracen-9-yl methyl)-amino]-isophthalic acid (H2AAPA) (13.7 mg, 0.05 mmol), dimethylacetamide (DMA, 3 mL), and deionized water (2.0 mL) was obtained with mechanical stirring for 30 min at room temperature. The mixture was transferred into a 10 mL Teflon-lined stainless steel vessel and heated at 100 °C for 48 h, and then cooled gradually to 298 K at a rate of 0.1 °C min−1. Yellow rod-shaped crystals were collected with a 70% yield based on H2AAPA. Anal. calcd for C158H159N11O41Sm4 (3469.22): C 54.65, N 4.44, O 18.91, H 4.58. Found: C 54.20, N 4.74, O 18.85, H 4.68. FT-IR (KBr, cm−1): 3403 (m), 3018 (w), 2980 (w), 1602 (s), 1425 (s), 1012 (w), 766 (s), 742 (s), 406 (s). The Fourier transform infrared spectra (FT-IR), as shown in Fig. S1.†
Photocatalytic reduction
The Cr(VI) photocatalytic reduction was achieved in a 100 mL quartz reactor at 298 K, and the reaction process was measured by UV–vis spectroscopy. The characteristic peak of Cr2O72− was at 540 nm, and its absorbance gradually declined with time under visible-light irradiation. In a typical test, dichromate (Cr2O72−) was the main form of Cr(VI) under acidic solution, and dichromate potassium (K2Cr2O7) was typically chosen as a representative Cr(VI) compound to achieve the photocatalytic reaction. Here, 10 mg catalyst LCUH-100 and 20 mL K2Cr2O7 aqueous solution (50 ppm) were uniformly dispersed into a 100 mL quartz reactor. The optimal pH value was 2.0 for the reaction solution, which could be adjusted with 0.2 mol L−1 H2SO4 dilute aqueous solution. First, the mixed solution was stirred vigorously in the dark for 60 min, which ensured LCUH-100 could reach adsorption–desorption equilibrium for K2Cr2O7. Second, the mixed solution was irradiated with a 300 W Xe lamp for 120 min. At regular intervals (10 min), 1.5 mL mixture was withdrawn from the 100 mL quartz reactor, and then filtered through a 0.22 μm hydrophilic membrane filter. At last, the filtered clear liquid was used for tracking tests by UV–vis spectroscopy using the previously reported diphenylcarbazide (DPC) method for the absorbance change of Cr2O72−. The above procedure was repeated for the rest of the mixture, and finally 13 group data points (including zero point) were successfully collected in 120 min.
Conflicts of interest
There are no conflicts to declare.
Acknowledgements
This work was supported by the National Natural Science Foundation of China (21401095, 21801107, 22178157), the Natural Science Foundation of Shandong Province (ZR2022MB010, ZR2019MB068, ZR2021QB123), Project of Shandong Province Higher Educational Science and Technology Program (KJ2018BZC043, J18KA113), the Liaocheng University Start-up Fund for Doctoral Scientific Research (318050104, 318052017), the Scientific Research Fund of Liaocheng University (318011913), the Youth Innovation Team of Shandong Colleges and Universities (2019KJC027, 2020KJC012), the Open Fund of Liaocheng University (2020CESNCTKL02), and the Shandong Students Innovation and Entrepreneurship Training Program (319260210).
References
- B. S. Rathi and P. S. Kumar, Application of adsorption process for effective removal of emerging contaminants from water and wastewater, Environ. Pollut., 2021, 280, 116995 CrossRef CAS PubMed.
- J. Theerthagiri, S. J. Lee, K. Karuppasamy, S. Arulmani, S. Veeralakshmi, M. Ashokkumar and M. Y. Choi, Application of advanced materials in sonophotocatalytic processes for the remediation of environmental pollutants, J. Hazard. Mater., 2021, 412, 125245 CrossRef CAS PubMed.
- Y. Wang, C. Kang, X. Li, Q. Hu and C. Wang, Ag NPs decorated C-TiO2/Cd0.5Zn0.5S Z-scheme heterojunction for simultaneous RhB degradation and Cr(VI) reduction, Environ. Pollut., 2021, 286, 117305 CrossRef CAS PubMed.
- B. Xue, L. Du, J. Jin, H. Meng and J. Mi, In situ growth of MIL-88A into polyacrylate and its application in highly efficient photocatalytic degradation of organic pollutants in water, Appl. Surf. Sci., 2021, 564, 150404 CrossRef CAS.
- J. Xiao, J. Liu, X. Gao, G. Ji, D. Wang and Z. Liu, A multi-chemosensor based on Zn-MOF: Ratio-dependent color transition detection of Hg(II) and highly sensitive sensor of Cr(VI), Sens. Actuators, B, 2018, 269, 164–172 CrossRef CAS.
- H. Abdullah and D. H. Kuo, Facile Synthesis of n-type (AgIn)xZn2(1-x)S2/p-type Ag2S Nanocomposite for Visible Light Photocatalytic Reduction To Detoxify Hexavalent Chromium, ACS Appl Mater Interfaces, 2015, 7(48), 26941–26951 CrossRef CAS PubMed.
- S. Das, S. Patnaik and K. M. Parida, Fabrication of a Au-loaded CaFe2O4/CoAl LDH p–n junction based architecture with stoichiometric H2&O2generation and Cr(VI) reduction under visible light, Inorg. Chem. Front., 2019, 6(1), 94–109 RSC.
- P. Mahato, S. Saha, P. Das, H. Agarwalla and A. Das, An overview of the recent developments on Hg2+ recognition, RSC Adv., 2014, 4(68), 36140–36174 RSC.
- D. Mallick, B. Biswal, M. Thirunavoukkarasu, R. Mohanty and B. Bag, Signalling probes appended with two rhodamine derivatives: inter-component preferences, Fe(iii)-ion selective fluorescence responses and bio-imaging in plant species, New J. Chem., 2017, 41(24), 15144–15156 RSC.
- L. Luo, S. Dong, H. Cui, L. Sun and T. Huang, Indium sulfide deposited MIL-53(Fe) microrods: Efficient visible-light-driven photocatalytic reduction of hexavalent chromium, J. Colloid Interface Sci., 2022, 606, 1299–1310 CrossRef CAS PubMed.
- P. Kar, T. K. Maji, P. K. Sarkar, P. Lemmens and S. K. Pal, Development of a photo-catalytic converter for potential use in the detoxification of Cr(VI) metal in water from natural resources, J. Mater. Chem. A, 2018, 6(8), 3674–3683 RSC.
- M. Faisal, F. A. Harraz, A. E. Al-Salami, A. M. El-Toni, A. A. Almadiy, A. Khan, J. P. Labis, S. A. Al-Sayari and M. S. Al-Assiri, Enhanced photocatalytic reduction of Cr(VI) on silver nanoparticles modified mesoporous silicon under visible light, J. Am. Ceram. Soc., 2019, 102(9), 5071–5081 CrossRef CAS.
- R. A. Hamouda, N. E. El-Naggar, N. M. Doleib and A. A. Saddiq, Bioprocessing strategies for cost-effective simultaneous removal of chromium and malachite green by marine alga Enteromorpha intestinalis, Sci. Rep., 2020, 10(1), 13479 CrossRef CAS PubMed.
- G. Yuan, F. Li, K. Li, J. Liu, J. Li, S. Zhang, Q. Jia and H. Zhang, Research Progress on Photocatalytic Reduction of Cr(VI) in Polluted Water, Bull. Chem. Soc. Jpn., 2021, 94(4), 1142–1155 CrossRef CAS.
- D. Dai, J. Qiu, L. Zhang, H. Ma and J. Yao, Amino-functionalized Ti-metal-organic framework decorated BiOI sphere for simultaneous elimination of Cr(VI) and tetracycline, J. Colloid Interface Sci., 2022, 607(2), 933–941 CrossRef CAS PubMed.
- S. Prakash Tripathy, S. Subudhi, S. Das, M. Kumar Ghosh, M. Das, R. Acharya, R. Acharya and K. Parida, Hydrolytically stable citrate capped Fe3O4@UiO-66-NH2 MOF: A hetero-structure composite with enhanced activity towards Cr(VI) adsorption and photocatalytic H2 evolution, J. Colloid Interface Sci., 2022, 606(1), 353–366 CrossRef CAS PubMed.
- Y.-X. Li, Y.-C. Han and C.-C. Wang, Fabrication strategies and Cr(VI) elimination activities of the MOF-derivatives and their composites, Chem. Eng. J., 2021, 405 Search PubMed.
- V. Kumar, V. Singh, K.-H. Kim, E. E. Kwon and S. A. Younis, Metal-organic frameworks for photocatalytic detoxification of chromium and uranium in water, Coord. Chem. Rev., 2021, 447, 214148 CrossRef CAS.
- B. Valizadeh, T. N. Nguyen, S. Kampouri, D. T. Sun, M. D. Mensi, K. Stylianou, B. Smit and W. L. Queen, A novel integrated Cr(VI) adsorption–photoreduction system using MOF@polymer composite beads, J. Mater. Chem. A, 2020, 8(19), 9629–9637 RSC.
- Y.-J. Lee, C.-G. Lee, J.-K. Kang, S.-J. Park and P. J. J. Alvarez, Simple preparation method for Styrofoam–TiO2 composites and their photocatalytic application for dye oxidation and Cr(VI) reduction in industrial wastewater, Environ. Sci.: Water Res. Technol., 2021, 7(1), 222–230 RSC.
- A. Mohamed, T. A. Osman, M. S. Toprak, M. Muhammed, E. Yilmaz and A. Uheida, Visible light photocatalytic reduction of Cr(VI) by surface modified CNT/titanium dioxide composites nanofibers, J. Mol. Catal. A: Chem., 2016, 424, 45–53 CrossRef CAS.
- V. P. Viswanathan, A. N. Nayarassery, M. M. Xavier and S. Mathew, A 2D/1D heterojunction nanocomposite built from polymeric carbon nitride and MIL-88A(Fe) derived α-Fe2O3 for enhanced photocatalytic degradation of rhodamine B, New J. Chem., 2022, 46(19), 9064–9074 RSC.
- C. Yang, R. Wang, W. Zhu, J. Wang, L. Zhang, T. Du, Z. Liu, L. Xie, J. Sun and J. Wang, Construction of In2S3@ZIF-8@ZnIn2S4 hierarchical nanoflower heterostructures to promote photocatalytic reduction activity, Inorg. Chem. Front., 2022, 9(1), 51–59 RSC.
- B. Abebe and H. C. A. Murthy, Insights into ZnO-based doped porous nanocrystal frameworks, RSC Adv., 2022, 12(10), 5816–5833 RSC.
- H. Kaur, R. Kumar, A. Kumar, V. Krishnan and R. R. Koner, Trifunctional metal-organic platform for environmental remediation: structural features with peripheral hydroxyl groups facilitate adsorption, degradation and reduction processes, Dalton Trans., 2019, 48(3), 915–927 RSC.
- S. Mondal, S. Das and U. K. Gautam, Defect-rich, negatively-charged SnS2 nanosheets for efficient photocatalytic Cr(VI) reduction and organic dye adsorption in water, J. Colloid Interface Sci., 2021, 603, 110–119 CrossRef CAS PubMed.
- C.-R. Chen, H.-Y. Zeng, S. Xu, J.-C. Shen, G. Hu, R.-L. Zhu, J.-Z. Du and Y.-X. Sun, Facile fabrication of CdS/ZnAlO heterojunction with enhanced photocatalytic activity for Cr(VI) reduction under visible light, Appl. Clay Sci., 2018, 165, 197–204 CrossRef CAS.
- H. C. Zhou and S. Kitagawa, Metal-organic frameworks (MOFs), Chem. Soc. Rev., 2014, 43(16), 5415–5418 RSC.
- A. Schneemann, V. Bon, I. Schwedler, I. Senkovska, S. Kaskel and R. A. Fischer, Flexible metal-organic frameworks, Chem. Soc. Rev., 2014, 43(16), 6062–6096 RSC.
- H. C. Zhou, J. R. Long and O. M. Yaghi, Introduction to metal-organic frameworks, Chem. Rev., 2012, 112(2), 673–674 CrossRef CAS PubMed.
- A. Pankajakshan, A. Ravarikkandy, B. P. Ratheesh, M. P. Maman and S. Mandal, Thiol decorated defective metal-organic frameworks embedded with palladium nanoparticles for efficient Cr(VI) reduction, Inorg. Chem. Front., 2021, 8(23), 5093–5099 RSC.
- J. Qiu, X. Zhang, Y. Feng, X. Zhang, H. Wang and J. Yao, Modified metal-organic frameworks as photocatalysts, Appl. Catal., B, 2018, 231, 317–342 CrossRef CAS.
- C.-C. Wang, X.-D. Du, J. Li, X.-X. Guo, P. Wang and J. Zhang, Photocatalytic Cr(VI) reduction in metal-organic frameworks: A mini-review, Appl. Catal., B, 2016, 193, 198–216 CrossRef CAS.
- Y. Gu, Y. N. Wu, L. Li, W. Chen, F. Li and S. Kitagawa, Controllable Modular Growth of Hierarchical MOF-on-MOF Architectures, Angew. Chem., Int. Ed., 2017, 56(49), 15658–15662 CrossRef CAS PubMed.
- Y. Pan, R. Abazari, J. Yao and J. Gao, Recent progress in 2D metal-organic framework photocatalysts: synthesis, photocatalytic mechanism and applications, J. Phys.: Energy, 2021, 3(3), 032010 CAS.
- H. Kaur, S. Sinha, V. Krishnan and R. R. Koner, Photocatalytic Reduction and Recognition of Cr(VI): New Zn(II)-Based Metal-Organic Framework as Catalytic Surface, Ind. Eng. Chem. Res., 2020, 59(18), 8538–8550 CrossRef CAS.
- Z. W. Jiang, T. T. Zhao, S. J. Zhen, C. M. Li, Y. F. Li and C. Z. Huang, A 2D MOF-based artificial light-harvesting system with chloroplast bionic structure for photochemical catalysis, J. Mater. Chem. A, 2021, 9(14), 9301–9306 RSC.
- Z. Zhang, Y. Wang, B. Niu, B. Liu, J. Li and W. Duan, Ultra-stable two-dimensional metal-organic frameworks for photocatalytic H2 production, Nanoscale, 2022, 14(19), 7146–7150 RSC.
- J. Wang, J. Zhang, S. B. Peh, L. Zhai, Y. Ying, G. Liu, Y. Cheng and D. Zhao, Dimensional Impact of Metal–Organic Frameworks in Catalyzing Photoinduced Hydrogen Evolution and Cyanosilylation Reactions, ACS Appl. Energy Mater., 2018, 2(1), 298–304 CrossRef.
- C. Tan, X. Cao, X. J. Wu, Q. He, J. Yang, X. Zhang, J. Chen, W. Zhao, S. Han, G. H. Nam, M. Sindoro and H. Zhang, Recent Advances in Ultrathin Two-Dimensional Nanomaterials, Chem. Rev., 2017, 117(9), 6225–6331 CrossRef CAS PubMed.
- Y. Xue, G. Zhao, R. Yang, F. Chu, J. Chen, L. Wang and X. Huang, 2D metal-organic framework-based materials for electrocatalytic, photocatalytic and thermocatalytic applications, Nanoscale, 2021, 13(7), 3911–3936 RSC.
- D. M. Chen, C. X. Sun, C. S. Liu and M. Du, Stable Layered Semiconductive Cu(I)-Organic Framework for Efficient Visible-Light-Driven Cr(VI) Reduction and H2 Evolution, Inorg. Chem., 2018, 57(13), 7975–7981 CrossRef CAS PubMed.
- H.-Q. Zheng, X.-H. He, Y.-N. Zeng, W.-H. Qiu, J. Chen, G.-J. Cao, R.-G. Lin, Z.-J. Lin and B. Chen, Boosting the photoreduction activity of Cr(VI) in metal–organic frameworks by photosensitiser incorporation and framework ionization, J. Mater. Chem. A, 2020, 8(33), 17219–17228 RSC.
- H. Wang, X. Yuan, Y. Wu, G. Zeng, X. Chen, L. Leng, Z. Wu, L. Jiang and H. Li, Facile synthesis of amino-functionalized titanium metal-organic frameworks and their superior visible-light photocatalytic activity for Cr(VI) reduction, J. Hazard. Mater., 2015, 286, 187–194 CrossRef CAS PubMed.
- X.-S. Wang, C.-H. Chen, F. Ichihara, M. Oshikiri, J. Liang, L. Li, Y. Li, H. Song, S. Wang, T. Zhang, Y.-B. Huang, R. Cao and J. Ye, Integration of adsorption and photosensitivity capabilities into a cationic multivariate metal-organic framework for enhanced visible-light photoreduction reaction, Appl. Catal., B, 2019, 253, 323–330 CrossRef CAS.
- R. Liang, L. Shen, F. Jing, W. Wu, N. Qin, R. Lin and L. Wu, NH 2 -mediated indium metal–organic framework as a novel visible-light-driven photocatalyst for reduction of the aqueous Cr(VI), Appl. Catal., B, 2015, 162, 245–251 CrossRef CAS.
- X.-D. Du, X.-H. Yi, P. Wang, W. Zheng, J. Deng and C.-C. Wang, Robust photocatalytic reduction of Cr(VI) on UiO-66-NH2(Zr/Hf) metal-organic framework membrane under sunlight irradiation, Chem. Eng. J., 2019, 356, 393–399 CrossRef CAS.
- R. Liang, F. Jing, G. Yan and L. Wu, Synthesis of CdS-decorated MIL-68(Fe) nanocomposites: Efficient and stable visible light photocatalysts for the selective reduction of 4-nitroaniline to p-phenylenediamine in water, Appl. Catal., B, 2017, 218, 452–459 CrossRef CAS.
- X. Wang, Y. Zhang, Z. Shi, T. Lu, Q. Wang and B. Li, Multifunctional Zr-MOF Based on Bisimidazole Tetracarboxylic Acid for pH Sensing and Photoreduction of Cr(VI), ACS Appl. Mater. Interfaces, 2021, 13(45), 54217–54226 CrossRef CAS PubMed.
- D. Liu, C. Li, C. Zhao, Q. Zhao, T. Niu, L. Pan, P. Xu, F. Zhang, W. Wu and T. Ni, Facile synthesis of three-dimensional hollow porous carbon doped polymeric carbon nitride with highly efficient photocatalytic performance, Chem. Eng. J., 2022, 438, 135623 CrossRef CAS.
- Y. Liu, Y. Liu, Y. Xu, Q. He, R. Yin, P. Sun and X. Dong, Phenanthroline bridging graphitic carbon nitride framework and Fe(II) ions to promote transfer of photogenerated electrons for selective photocatalytic reduction of Nitrophenols, J. Colloid Interface Sci., 2022, 608, 2088–2099 CrossRef CAS PubMed.
- H.-Y. Zhang, Y. Yang, C.-C. Li, H.-L. Tang, F.-M. Zhang, G.-L. Zhang and H. Yan, A new strategy for constructing covalently connected MOF@COF core–shell heterostructures for enhanced photocatalytic hydrogen evolution, J. Mater. Chem. A, 2021, 9(31), 16743–16750 RSC.
- C. Zhao, Z. Chen, J. Xu, Q. Liu, H. Xu, H. Tang, G. Li, Y. Jiang, F. Qu, Z. Lin and X. Yang, Probing supramolecular assembly and charge carrier dynamics toward enhanced photocatalytic hydrogen evolution in 2D graphitic carbon nitride nanosheets, Appl. Catal., B, 2019, 256, 117867 CrossRef CAS.
Footnotes |
† Electronic supplementary information (ESI) available: Experimental, characterization and additional figures. CCDC 2170346. For ESI and crystallographic data in CIF or other electronic format see DOI: https://doi.org/10.1039/d3qi00102d |
‡ These authors are contributed equally to this work. |
|
This journal is © the Partner Organisations 2023 |
Click here to see how this site uses Cookies. View our privacy policy here.