DOI:
10.1039/D3NA00467H
(Paper)
Nanoscale Adv., 2023,
5, 4286-4297
New application of a periodic mesoporous nanocrystal silicon–silica composite for hyperlipidemia
Received
28th June 2023
, Accepted 8th July 2023
First published on 31st July 2023
Abstract
The integration of the properties of silicon nano crystallinity with silica mesoporosity provides a wealth of new opportunities for emerging biomedicine. Cholesterol (CHO) and triglyceride (TG) levels have always been a challenge for cardiologists in the treatment of patients with chronic coronary artery disease (CAD). For patients with hyperlipidemia, statins and other lipid-lowering drugs are currently recommended. It should be noted, however, that significant side effects have been reported in the treatments, including liver damage, muscle pain, etc. We here found that our previously produced periodic mesoporous nanocrystalline silicon–silica, meso-ncSi/SiO2 (PMS), a nanocomposite material, has the properties of lowering CHO and TG, and is associated with better safety and biocompatibility compared to existing lipid-lowering drugs. After being incubated with PMS for 2 hours, CHO and TG levels in blood were significantly lower than before. In addition, CHO and TG adsorbed on with PMS could also be extracted and released, contributing to the recovery and recycling of PMS.
Background
According to the epidemiology of hyperlipidemia in the USA from 2007 to 2016, 48.6% to 57.9% of youths had elevated levels of cholesterol (CHO), high-density lipoprotein CHO, or non-high-density lipoprotein CHO,1 which would contribute to an increased rate of cardiovascular events by 20% and total mortality by 12% with the levels of CHO increased by 1 mmol L−1.2,3 Based on the administrative databases of 3 Italian Local Health Units, one retrospective longitudinal cohort analysis showed that patients with higher triglycerides (TG) would have a higher risk of all-cause mortality (HR: 1.49, 95% CI: 1.36–1.63, P < 0.001) and incident atherosclerotic cardiovascular disease events (HR: 1.61, 95% CI: 1.43–1.82, P < 0.001) in comparison.4 Based on an analysis of hyperlipidemia and medical expenditure among adults in the United States, it was observed that cardiovascular disease (CVD) patients with hyperlipidemia had higher expenditure ($1105), contributing to a $15.47 billion annual national expenditure.5
In addition to healthy lifestyle interventions, many therapies, including statins, fibrates, niacin, ezetimibe, and bile acid sequestrants are recommended for patients for reducing the level of blood lipids. In addition to the benefits, there are also limitations, such as myalgias, rhabdomyolysis, new-onset diabetes mellitus, blood transaminase elevation, an influence on appetite, etc.2,6
Recently, there has been growing interest in adsorbing agents such as resin and smectite, which have been found to effectively adsorb lipids and reduce weight. These agents have shown promising results in lipid adsorption. However, it is important to note that both resin and smectite exhibit poor biocompatibility, which is a significant drawback.7–9
Nowadays, silicon has been found to improve lipemia and insulin resistance, which would prevent atherogenic processes.10 Additionally, a silicon-enriched diet would decrease very low-density lipoprotein (VLDL) concentrations and VLDL oxidation compared to a diet without silicon.11 Based on silicon, our previously produced periodic mesoporous nanocrystalline silicon–silica, meso-ncSi/SiO2 (PMS) was a nanocomposite material.12 A groundbreaking approach for efficient organic functionalization of PMS was achieved by co-condensation of (R′O)3SiH and (R′O)3Si–R type terminal trialkoxy organosilanes. In the case of co-condensing MTES and TES, the organic PMS reached a maximum of 78% in the incorporation of the methyl group (CH3–SiO3 unit), which greatly broke the previous limit of 25%. Organic functionalization of PMS was an imperative and effective measure to advance its preeminent properties and widespread application due to its structural superiority.
Due to its ordered mesoporous structure, large surface area, and ease of functionalization, PMS exhibits excellent adsorption properties, which makes it an excellent solid-phase extraction adsorbent.13,14 Moreover, various studies have demonstrated the ability of PMS to adsorb a variety of substances, such as gaseous species, liquids and solids.15–17 Additionally, it was shown that co-administration of PMS in rats fed with a high-fat diet significantly reduced weight gain as compared to a negative control group, indicating that the PMS effectively adsorbed lipid substances within the gastrointestinal tract.18 In recent years, PMS has demonstrated excellent biocompatibility within living organisms and has been widely used as a drug carrier through intravenous administration.19–22 Nevertheless, it is unclear whether intravenous administration of PMS would further reduce blood lipid levels and atherosclerosis.
We therefore conducted this study to determine whether PMS is effective in reducing blood lipid levels. In our study, PMS was provided by the State Key Experiment in Biomedical Engineering at Southeast University. Overall, PMS was added into CHO/TG solutions to adsorb CHO/TG from solutions, and CHO/TG would also be separated from PMS (Fig. 1).
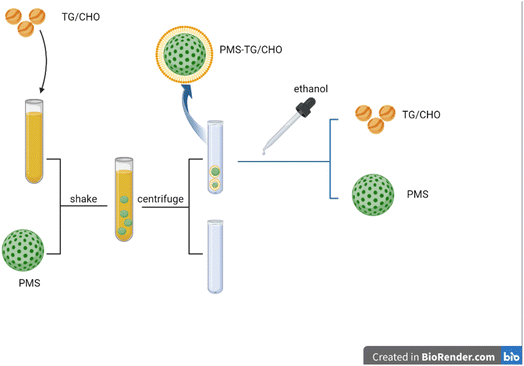 |
| Fig. 1 Model diagram for CHO/TG adsorbed on PMS and separated from PMS. In brief, PMS was introduced into the CHO/TG solution to optimize the adsorption of CHO/TG onto PMS. Following centrifugation, the sediment was obtained, forming PMS-CHO/TG. Moreover, the addition of ethanol enabled the effective extraction and release of the adsorbed CHO/TG from PMS-CHO/TG. | |
Experimental section
Various studies have demonstrated the ability of PMS to adsorb a variety of substances, such as gaseous species, liquids and solids.15–17 Meaningfully, PMS could be successfully applied to adsorb the substances and the substances could be released. Especially for the adsorption of substances,17 the adsorption amount of PMS could be determined by checking the concentration of substances in the supernatant. Similarly, the adsorption amount of PMS and the release characteristics of PMS for adsorbed substances could also be clarified. Similarly, our experiment was performed.
Material
Sigma-Aldrich (Germany) provided cetyltrimethylammonium chloride (CTAC), triethanolamine (TEA), tetraethyl orthosilicate (TEOS) and ethanol; standard CHO and TG were purchased from Nanjing Jiancheng Bioengineering Institute (China).
Synthesis of PMS
Cetyltrimethylammonium chloride (CTAC, 8 g) and triethanolamine (TEA, 0.068 g) were dissolved in turn in 20 mL of water at 95 °C under intensive stirring. After 1 h, 1.5 mL of tetraethyl orthosilicate (TEOS) was added dropwise and the resulting mixture was stirred for another hour. The products were collected by centrifugation and washed several times with ethanol to remove the residual reactants. Then, the collected products were extracted for 12 h with a mixture of ethanol and hydrochloric acid at 60 °C to remove the CTAC template. This process was carried out several times. Finally, residual surfactants and organics were completely removed by calcination at 450 °C and dispersed in water after ultrasonic and centrifugal cleaning three times.23,24
Characterization
Observation by means of transmission electron microscopy (TEM) (JEM-2100; JEOL, Tokyo, Japan): the particles were dispersed in ddH2O and treated with ultrasound for 15 min to acquire the mixture for evaluation. The particles were dripped onto a 200-mesh copper TEM grid and dried afterwards. Finally, with the help of TEM, different images related to PMS were gathered. A nano particle potentiometer (Malvem, England) was used to measure the zeta potential and particle size of PMS before and after PMS adsorbed CHO and TG with the particles dissolved in PBS.
Preparation of a standard solution of CHO and TG
With the powder dissolved in ethanol, a standard solution of CHO (5.17 mmol L−1) was synthesized. Similarly, a standard solution of TG (2.16 mmol) was also prepared with ethanol. Then, standard CHO and TG solutions were acquired, which were used for the following experiments independently.
The capacity of PMS for adsorbing CHO and TG in comparison to polystyrene resin
To adsorb the CHO from a solution with PMS, 10, 20, 40, 60, 80, and 100
μL of a standard CHO solution (5.17 mmol L−1) were added into six 1.5 mL centrifuge tubes, in which 90, 80, 60, 40, 20, and 0
μL of water were then added respectively. Subsequently, 1 mg of PMS was added to each solution, which was dispersed and shaken at 4 °C for 2 h. Afterward, the solutions were centrifuged at 12
000 rpm at 4 °C for 10 min, the supernatant was then acquired to measure the concentration of CHO and the sediment was composed of PMS-CHO, which was washed twice with water. Finally, the capacity of PMS for adsorbing CHO was calculated based on the volume of CHO in the acquired supernatants and the previous solutions. Similarly, the supernatants of the TG solutions were acquired to test the concentration of TG and the sediment was composed of PMS-TG. The capacity of PMS for adsorbing TG was further calculated. In our study, we used polyethylene resin as the positive control group, considering its value in blood purification and lipid adsorption. In comparison, similar concentrations of CHO and TG solution were prepared, and 1 mg polystyrene resin was added into the centrifuge tubes respectively.
The capacity of PMS for adsorbing CHO and TG at different temperatures
According to our previous results, we evaluated the ability of PMS at different temperatures to adsorb CHO and TG from 60% of standard CHO solution (3.10 mmol L−1) and TG solution (1.36 mmol L−1), respectively.
In three 1.5 mL centrifuge tubes, 100
μL of CHO solution was added along with 1 mg of PMS, which was dispersed and shaken for 2 h at different temperatures, including 4 °C, 20 °C and 37 °C. Afterward, the solutions were centrifuged to obtain the supernatant, and the capacity of PMS to adsorb CHO at different temperatures could be determined.
Similarly, PMS's capacity to adsorb TG at different temperatures was assessed.
PMS for adsorbing CHO and TG in serum
To obtain serum, blood samples were centrifuged at 2000 rpm for 20 minutes and then heated to 80 °C for 1.5 minutes to remove lipases, which protect CHO and TG from hydrolysis. Furthermore, the serum was diluted in water to obtain the appropriate concentration, with the concentrations of CHO and TG adjusted to approximately 3.1 mmol L−1 and 1.2 mmol L−1, respectively.
Safety assessment of PMS in vivo
L02 cells were inoculated into 96-well plates in an incubator set at 37 °C until more than 80% of the cells had been inoculated. Different concentrations of PMS (0–100 μg mL−1) were added to the 96-well plate, with a total volume of 100 μL per well, and the culture continued for 24 hours. Afterwards, the proliferative ability of the cells was determined by CCK-8 reagent. During the experiment, the wells without cells served as a blank group. And the control group of cells was treated with 0 μg mL−1 of PMS.
Safety assessment of PMS in blood
As part of our study, blood was collected from 19 CAD 18–80 year-old patients, and safety parameters will be measured, including TBil (total bilirubin), DBil (direct bilirubin), IBil (indirect bilirubin), ALT (alanine aminotransferase), AST (aspartate aminotransferase), BUN (blood urea nitrogen) and Cr (creatinine). By mixing vitro blood with PMS and shaking it for 2 hours, safety parameters were obtained to evaluate PMS's safety. The Ethics Committee of Zhongda Hospital, Southeast University, China (approval number 2021ZDSYLL373-P01) approved all experiments. Informed consent was obtained from all subjects before samples were collected. The Ethics Committee of Zhongda Hospital approved and supervised all biosecurity, ethics and institutional safety procedures. Subjects were recruited in accordance with the Guidelines of the Declaration of Helsinki (Ethical Principles for Medical Research Involving Human Subjects, World Medical Association).
Comparison between PMS and statins for reducing CHO and TG
More importantly, PMS was added to the isolated blood of patients to judge its clinical role in reducing blood CHO and TG. 19 blood samples of patients visiting Zhongda Hospital affiliated to Southeast University were collected in our study. During our study, an automatic biochemical detector was used to test the blood lipids of the patients by collecting 5 mL of venous blood. A further 5 mL of venous blood was also obtained in order to assess the effect of PMS on lowering blood lipid. And 5 mL of venous blood was added to a 15 mL centrifuge tube and incubated with PMS. Then, an automatic biochemical detector was used to assess the level of blood lipids after shaking the mixture for two hours. Furthermore, the effectiveness of PMS in reducing blood cholesterol and triglycerides was compared. In our study, another 20 CAD patients taking a statin (atorvastatin 20 mg or rosuvastatin 10 mg) for at least 3 months were also enrolled in our study. And the lipid levels, including the levels of CHO and TG, were measured before and after statin treatment for 3 months. Furthermore, the lipid-lowering effect of a single use of PMS would be further compared with the lipid-lowering effect of statins used for 3 months.
Recovery and recycling of PMS
In order to determine the amount of CHO in PMS-CHO, the mixture was incubated with lipase for 30 minutes, then the CHO kit was used to measure the amount of CHO. Then, PMS-CHO was dispersed in 1000
μL of ethanol to separate PMS from CHO. Afterwards, when the mixture was centrifuged at 12
000 rpm at 4 °C for 10 minutes, the supernatant was obtained to determine the amount of CHO using the CHO kit, while the sediment was named PMS-CHO-1, which was used to determine the amount of remaining CHO on PMS using the lipase and CHO kits.
To determine the amount of TG in PMS-TG, PMS-TG was also incubated with lipase. In addition, PMS-TG was dispersed in ethanol to separate TG from PMS. After centrifugation, the supernatant and sediment (PMS-TG-1) were acquired to evaluate the amount of TG.
In order to evaluate whether PMS could be regenerated for repeated use, PMS-CHO and PMS-TG were washed with ethanol and water to obtain PMS. Such PMS was also obtained to determine PMS' capacity to adsorb CHO and TG, and the process was repeated several times.
Statistical analysis
GraphPad Prism 7.0 software (GraphPad Software Inc., La Jolla, CA, USA) was used for data analysis and statistical comparison between experimental groups. According to the distribution of the data, continuous data were expressed by mean ± standard deviation, median, or quartile; paired t-test was conducted in our analysis to make the comparison between the two groups. Results were considered statistically significant when the two-sided P-value was ≤0.05.
Results and discussion
Characterization
As described in our previous work, with photoluminescent silicon nanocrystallites embedded within a silica matrix throughout the mesostructure, PMS was finally produced, and N2 adsorption–desorption isotherms with thermal treatment of PMS are shown in Fig. 2A.25 TEM images revealed that the particles and pores were homogeneous when not adsorbing CHO or TG (Fig. 2B). In comparison, after incubation with CHO or TG, PMS was observed to be surrounded by CHO (indicated by red arrows; Fig. 2C) or TG (indicated by green arrows; Fig. 2D), which contributed to the poor dispersion of particles. The size characterization of PMS before and after adsorption revealed that the PMS particles adsorbing CHO (Fig. 2E2) and TG (Fig. 2E3) exhibited a significant increase in particle size compared to PMS (Fig. 2E1) without lipid adsorption. This difference was statistically significant (Fig. 2E4), indicating the successful adsorption of CHO and TG by PMS. With respect to potential changes of PMS before and after lipid adsorption, it was indicated that PMS (Fig. 2F1) without lipid adsorption exhibited a higher negative potential. In comparison, the surface negative potential of PMS after adsorbing CHO (Fig. 2F2) and TG (Fig. 2F3) decreased. And the difference was statistically significant (Fig. 2F4).
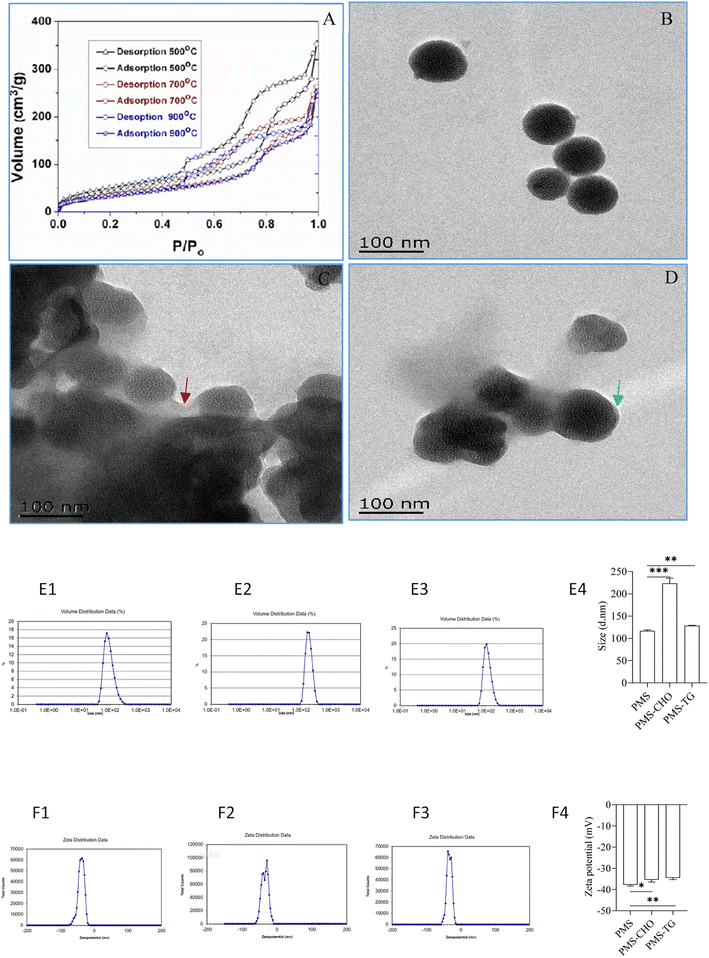 |
| Fig. 2 Characterization of different PMS. (A) N2 adsorption–desorption isotherms with thermal-treatment of PMS; (B) PMS without modifications; (C) PMS coated with CHO; (D) PMS coated with TG; (E) zeta potential of PMS; CHO: cholesterol; TG: triglyceride; (E1): size of PMS; (E2): size of PMS-CHO; (E3): size of PMS-TG; (E4) comparison of the size of different particles, including PMS, PMS-CHO and PMS-TG; (F1): zeta potential of PMS; (F2): zeta potential of PMS-CHO; (F3): zeta potential of PMS-TG; (F4) comparison of the zeta potential of different particles, including PMS, PMS-CHO and PMS-TG. | |
The capacity of PMS for adsorbing CHO and TG in comparison to polystyrene resin
In order to determine the ability of PMS to adsorb lipids, different concentrations of CHO have been used. As shown in Fig. 3A, PMS was mixed with different concentrations of CHO. Compared with CHO solutions with higher or lower concentrations, PMS showed better performance in the adsorption of lipids from a standard cholesterol solution with 60% concentration (3.1 mmol L−1). According to Fig. 3B, PMS adsorbed TG most efficiently in a standard TG solution with a 60% concentration when TG was adsorbed at different concentrations (1.2 mmol L−1). Similarly, a positive control group was treated with polyethylene resin. As shown in the figure, the resin exhibited strong adsorption capacity for CHO (Fig. 3C) and relatively weak adsorption capacity for TG (Fig. 3D).
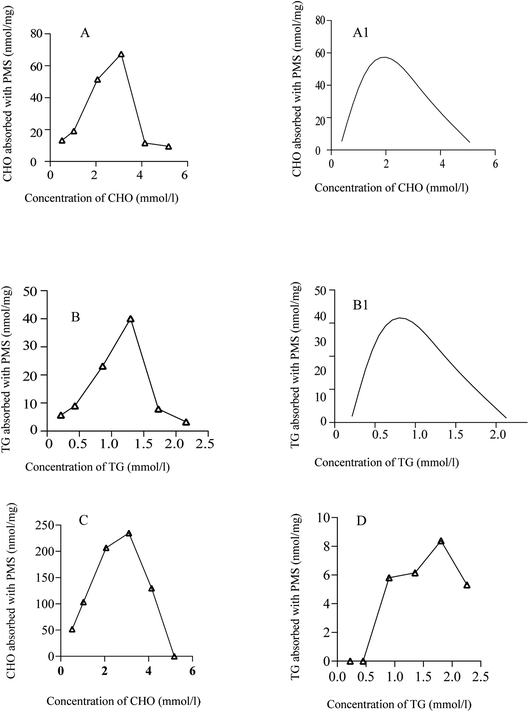 |
| Fig. 3 The capacity of PMS for adsorbing CHO or TG at different concentrations. (A) The capacity of PMS for adsorbing CHO at different concentrations; (A1) fitted curve for PMS adsorbing CHO at different concentrations; (B) the capacity of PMS for adsorbing TG at different concentrations; (B1) fitted curve for PMS adsorbing TG at different concentrations; (C) the capacity of polystyrene resin for adsorbing CHO at different concentrations; (D) the capacity of polystyrene resin for adsorbing TG at different concentrations. | |
The capacity of PMS for adsorbing CHO and TG at different temperatures
As shown in Fig. 4A, PMS showed a better performance for adsorbing CHO at lower temperature as compared to higher temperature. PMS also exhibited better performance at lower temperature when it came to adsorbing TG (Fig. 4B).
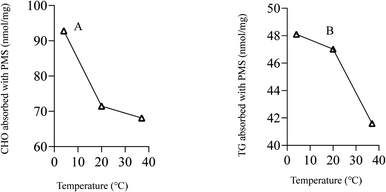 |
| Fig. 4 The capacity of PMS for adsorbing CHO or TG at different temperatures. (A) The capacity of PMS for adsorbing CHO at different temperatures. (B) The capacity of PMS for adsorbing TG at different temperatures. | |
Safety assessment of PMS in vivo
The viability of L02 cells was determined using CCK-8 reagent after cells were incubated with different concentrations of PMS for 24 hours. As shown in the figure, PMS did not cause significant toxicity, even at higher concentrations (100
μg mL−1) (Fig. 5).
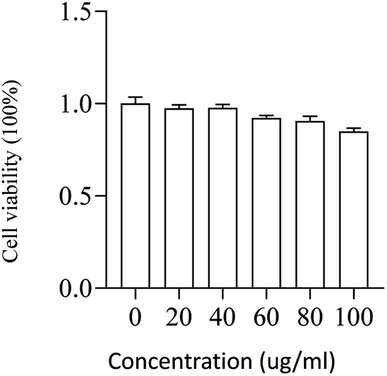 |
| Fig. 5 Safety assessment of PMS in L02. The cell viability of L02 that was incubated with varying concentrations (0, 20, 40, 60, 80, 100 μg mL−1) of the particles for 24 h. | |
Safety assessment of PMS in blood
We next analyzed the effect of PMS on blood biochemical indexes when lowering blood lipid; as shown in Table 1, there was no significant difference detected in terms of IBil (8.52 ± 3.42 vs. 8.12 ± 3.16, p = 0.22), ALT (22.68 ± 16.25 vs. 23.21 ± 16.39, p = 0.18) and BUN (6.82 ± 2.53 vs. 6.84 ± 2.52, p = 0.43). In comparison, PMS was associated with reduced TBil (12.31 ± 4.71 vs. 13.01 ± 4.71, p < 0.001), DBil (3.78 ± 2.24 vs. 4.88 ± 2.01, p = 0.001) and Cr (90.56 ± 46.86 vs. 93.06 ± 46.71, p = 0.008). These data indicated that PMS not only did not have negative effects on biochemical indicators such as IBil and ALT, but also had certain clearance effects on other biochemical metabolites such as DBil and Cr.
Table 1 Safety assessment of PMS in blooda
|
After the intervention |
Before the intervention |
P value |
TBil: total bilirubin; DBil: direct bilirubin; IBil: indirect bilirubin; ALT: alanine aminotransferase; AST: aspartate aminotransferase; BUN: blood urea nitrogen; Cr: creatinine.
|
TBil, μmol L−1 |
12.31 ± 4.71 |
13.01 ± 4.71 |
<0.001 |
DBil, μmol L−1 |
3.78 ± 2.24 |
4.88 ± 2.01 |
0.001 |
IBil, μmol L−1 |
8.52 ± 3.42 |
8.12 ± 3.16 |
0.22 |
ALT, U L−1 |
22.68 ± 16.25 |
23.21 ± 16.39 |
0.18 |
BUN, mmol L−1 |
6.82 ± 2.53 |
6.84 ± 2.52 |
0.43 |
Cr, μmol L−1 |
90.56 ± 46.86 |
93.06 ± 46.71 |
0.008 |
PMS was potent in lowering blood lipids in comparison with statin
The capacity of PMS for separating CHO and TG was first judged in the next study. With blood centrifuged at 2000 rpm (20 min), the serum was acquired and diluted with water to achieve a standard serum, with the concentration of CHO and TG at 3.1 mmol L−1 and 1.2 mmol L−1 respectively. After mixing with PMS and shaking for 2 h, the solution became more transparent and clearer, indicating that more CHO and TG were separated from the serum with PMS (Fig. 6). 19 whole blood samples were collected from patients. After being treated with PMS, the in vitro blood was identified with significant decreased levels of CHO (3.64 ± 1.01 vs. 3.71 ± 1.02, p < 0.001) and TG (1.43 ± 0.80 vs. 1.47 ± 0.82, p < 0.001) when compared to the control without intervention (Table 2).
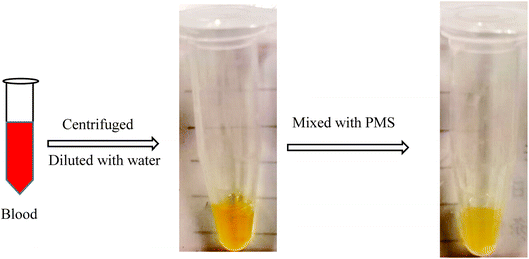 |
| Fig. 6 PMS for adsorbing CHO and TG in serum. In brief, peripheral blood plasma was obtained from patients with atherosclerosis through centrifugation, and PMS was introduced for the adsorption of CHO/TG from the plasma, resulting in a visible lightening of the plasma color. | |
Table 2 PMS for reducing CHO and TG in patients' blood
|
After the intervention |
Before the intervention |
P value |
CHO, mmol L−1 |
3.64 ± 1.01 |
3.71 ± 1.02 |
P < 0.001 |
TG, mmol L−1 |
1.43 ± 0.80 |
1.47 ± 0.82 |
P < 0.001 |
As a powerful lipid-lowering agent here, we further compared the difference between PMS and traditional statins in the degree of lipid-lowering ability for CAD patients. The efficacy of patients receiving statins for 3 months and the efficacy of one intervention with PMS were compared and are shown in Table 2. With respect to CHO, PMS was proved to be superior to statin (atorvastatin 20 mg or rosuvastatin 10 mg, 3 months) for reducing blood CHO (p = 0.021); with respect to TG, a single intervention with PMS achieved considerable results as against three months of statin treatment (p = 0.685) (Table 3).
Table 3 Comparison between PMS and statin for reducing blood CHO and TG
|
Mesoporous silicas |
Statin |
P value |
Reduced TG, mmol L−1 |
0.03 (0.02, 0.04) |
0.05 (−0.12, 0.36) |
0.685 |
Reduced CHO, mmol L−1 |
0.065 (0.035, 0.125) |
0.33 (0.07, 1.55) |
0.021 |
Recovery and recycling of PMS
In practice, it was important to ensure that PMS could be regenerated for repeated use. In this study, PMS was mixed with a 60% standard concentration of CHO (3.1 mmol L−1) or TG (1.2 mmol L−1) to evaluate the regeneration of PMS.
With PMS-CHO mixed with lipase, 64.0 nmol of CHO was detected using a CHO kit. Afterward, PMS-CHO was treated with ethanol to release the adsorbed CHO. After centrifugation, the supernatant was acquired to determine the amount of CHO (61.6 nmol), and the sediment was composed of PMS-CHO-1, which was also mixed with lipase to evaluate the remaining amount of CHO on PMS (close to 0) (Fig. 7).
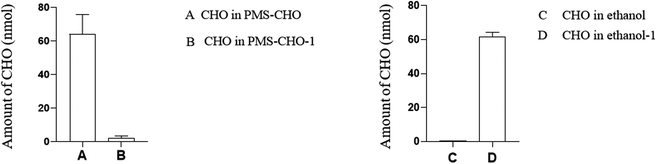 |
| Fig. 7 Estimation and extraction of CHO on PMS. (A) The amount of CHO in PMS-CHO; (B) the amount of CHO in ethanol; (C) the amount of CHO in ethanol before PMS-CHO mixed with ethanol; (D) the amount of CHO in ethanol after PMS-TG mixed with ethanol. | |
When treated with lipase, 38.8 nmol of TG was detected using a TG kit. With PMS-TG mixed with ethanol and centrifuged, 36.4 nmol of TG was detected in ethanol, while nearly 0 nmol of TG was detected in the remaining PMS-TG-1 (Fig. 8).
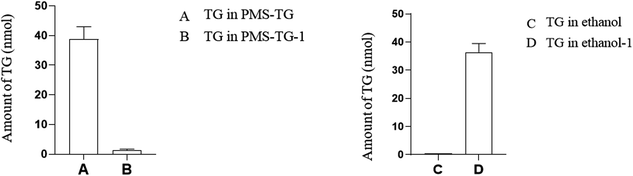 |
| Fig. 8 Estimation and extraction of TG on PMS. (A) The amount of TG in PMS-TG; (B) the amount of TG in ethanol; (C) the amount of TG in ethanol before PMS-TG mixed with ethanol; (D) the amount of TG in ethanol after PMS-TG mixed with ethanol. | |
It has been shown in the figure that PMS can be used repeatedly, which was associated with a better capacity to adsorb CHO with different numbers of cycles, similar to TG (Fig. 9).
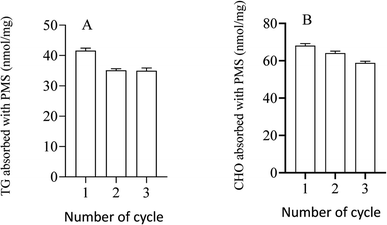 |
| Fig. 9 The capacity of PMS for adsorbing CHO or TG with different numbers of cycles. (A) The capacity of PMS for adsorbing CHO with different numbers of cycles; (B) the capacity of PMS for adsorbing TG with different numbers of cycles. | |
Adsorption mechanism
Zeta potential of the particles.
Our study further investigated the mechanism of the role of PMS in lipid adsorption. As shown in Fig. 2F1–F4, PMS caused a decrease in the surface negative potential level after adsorbing CHO and TG.
This suggested that PMS adsorbed some positively charged substances, leading to a reduction in surface negative potential, which demonstrated the significant role of electrostatic attraction in the adsorption of PMS.
FT-IR and XPS.
Furthermore, we conducted Fourier transform infrared (FTIR) and X-ray photoelectron spectroscopy (XPS) analyses on PMS before and after adsorption to further elucidate the adsorption mechanism. FTIR spectra were recorded on a Nicolet 5700 spectrometer. And XPS was performed on a ThermoFisher Nexsa (USA). The data were processed using Origin 8.0.
The results, as shown in Fig. 10, revealed specific spectral changes upon CHO (Fig. 10A) adsorption. The peaks corresponding to hydroxyl groups (3400–3500 cm−1) shifted backward and exhibited a noticeable decrease in transmittance. Additionally, changes in peak positions and intensities around 1500–1700 cm−1 and 1000 cm−1 are attributed to the vibrational modes of N–H and C–O functional groups. Similar spectral changes were observed for TG (Fig. 10B) adsorption.
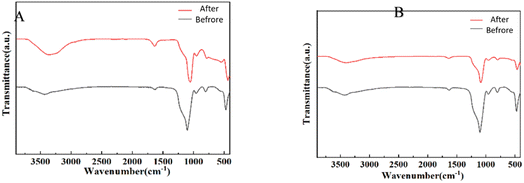 |
| Fig. 10 FT-IR spectra of PMS before and after adsorbing CHO (A) and TG (B). Following the completion of CHO/TG adsorption by PMS, specimens were obtained both before (PMS) and after adsorption (PMS-CHO/TG). Spectral analysis was conducted on each specimen, and the spectral information regarding the changes in functional groups before (black line) and after adsorption (red line) was processed using Origin software. | |
XPS further analyzed the chemical structure of PMS and evaluated potential interactions during the adsorption process. The adsorbents before and after adsorption were examined for the adsorption of individual elements: carbon (C), nitrogen (N), oxygen (O), and silicon (Si).
Regarding CHO adsorption, the results, as shown in Fig. 11, revealed the following spectral changes: C 1s spectrum (Fig. 11A): three peaks at 284.82 eV, 286.45 eV, and 288.43 eV corresponded to C–C, C–N, and C–O, respectively. The binding energies of C–N and C–O decreased significantly in the adsorption process, while other bonds showed no significant changes. N 1s spectrum (Fig. 11B): two independent peaks representing N–H and C–N groups were observed at 399.64 eV and 402.43 eV, respectively, on PMS after lipid adsorption. The changes in C–N and N–H were evident compared to the pre-adsorption state. O 1s spectrum (Fig. 11C): the main peaks at 531.13 eV and 532.61 eV corresponded to O–H and C–O, respectively. During lipid adsorption, the main changes occurred in the O–H bonding. Si spectrum (Fig. 11D): the changes before and after adsorption were not significant.
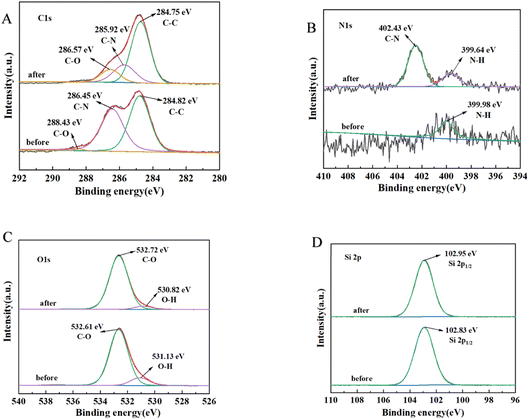 |
| Fig. 11 C 1s (A), N 1s (B), O 1s (C) and Si 2p (D) XPS spectra of PMS before and after adsorbing CHO. Following the completion of CHO adsorption by PMS, specimens were obtained both before (PMS) and after adsorption (PMS-CHO). XPS spectra analysis was conducted on each specimen, and the spectral information regarding the changes was processed using Origin software, including C, N, O and Si. | |
In terms of XPS spectra, regarding CHO adsorption, the significant changes in binding energy and intensity of C–N, C–O, N–H and O–H suggested that amino groups were one of the primary functional groups responsible for lipid adsorption.
Similarly, for TG adsorption, the results, as shown in Fig. 12, indicated the following: C 1s spectrum (Fig. 12A): slight decreases in the binding of C–N were observed. N 1s spectrum (Fig. 12B): no significant changes were observed before and after adsorption with respect to decreasing the binding. O 1s spectrum (Fig. 12C): the main changes occurred in the O–H bonding during lipid adsorption. Si spectrum (Fig. 12D): the changes before and after adsorption were not significant.
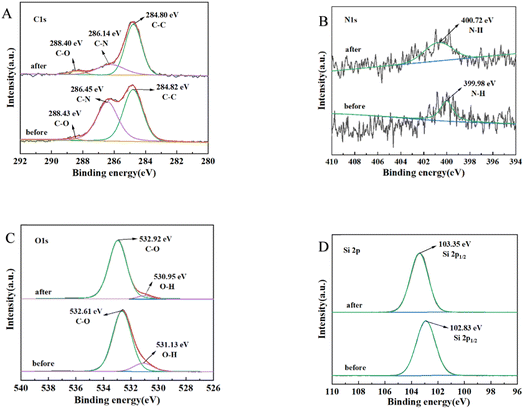 |
| Fig. 12 C 1s (A), N 1s (B), O 1s (C) and Si 2p (D) XPS spectra of PMS before and after adsorbing TG. Following the completion of TG adsorption by PMS, specimens were obtained both before (PMS) and after adsorption (PMS-TG). XPS spectra analysis was conducted on each specimen, and the spectral information regarding the changes was processed using Origin software, including C, N, O and Si. | |
These findings suggested that C–N, N–H, and O–H functional groups were primarily responsible for the adsorption of lipids.
Discussion
In our study, PMS was associated with a greater capacity to adsorb CHO and TG, which could be released from PMS, contributing to its repeated use, which might be applied as a crucial component of an extracorporeal blood lipid purifier or treated as a lipid-lowering drug in the future.
First introduced by Mobil corporation scientists in 1992, associated with a tailored mesoporous structure, huge surface area, large pore volume, selective surface functionality, and morphology control, mesoporous silicon is more popular for biomedical application and has been applied to deliver drugs in the medical field, which has achieved great success.26
Similar to our findings, in obese mice treated with a high-fat diet, animals fed mesoporous silicas had significantly reduced body weight (6%) and body fat composition (12%) as compared with animals in the control group, demonstrating that mesoporous silicas reduce lipid absorption in the gastrointestinal tract.27 However, Natalia et al. found that mesoporous silicas did not reduce blood lipid levels in a significant manner, which was different from our findings. In comparison, PMS was mixed with peripheral blood to reduce blood lipid levels in our study, while mesoporous silicas were added into the gastrointestinal tract and evaluated its influence on blood lipid levels in the study performed by Natalia et al., which might partly explain this difference. In addition, another study was also performed to evaluate the effects of mesoporous silicas supplemented in food on metabolic parameters, which also conveyed a similar message that mesoporous silicas can successfully reduce adipose tissue formation from 9.4 to 6.5 g and leptin levels from 32.8 to 16.9 ng mL−1 respectively due to a 33% reduction in food efficiency.28
There are several factors that may contribute to the potential effect of PMS-based adsorbents on reducing blood lipid levels and improving atherosclerosis. Firstly, PMS contributed to the reduction of lipid levels in the blood by exerting various forces to adsorb blood lipids. Secondly, PMS–blood lipid complexes can interfere with the normal interaction between blood lipids and lipase, thereby disrupting the functionality of lipase. The combined effect of these two factors led to the reduction of blood lipid levels and improvement of atherosclerosis.
In terms of raw materials of PMS, silicon was reported to affect the metabolism of blood lipids. Silicon is a crucial component of the human body, measured in many tissues, such as the aorta, the tracheae, bones, skin, etc. A number of biological effects and disorders have been linked to silicon, including bone mineralization, collagen synthesis, aging of the skin, atherosclerosis, Alzheimer's disease, as well as many others.29 As an explanation for silicon's role in reducing blood lipid levels, silicon is thought to form molecular crosslinks and contribute to the formation of ester bonds, which may contribute to reduced digestibility and bioavailability.11
Additionally, as demonstrated in this study, most CHO and TG on PMS could also be released when mixed with ethanol, demonstrating that CHO and TG were adsorbed or extracted from blood without any chemical reaction. Similarly, a previous study performed in 2018 found that mesoporous silicas could be used in solid-phase extraction for adsorbing phospholipids in human milk, which was associated with a higher adsorption capacity (544 μg of PC mg−1 of sorbent) and reusability (up to 15 reuses).30
Current research suggested that the specific adsorption mechanisms and forces of PMS on lipids are as follows: (1) PMS contained Si–OH and Si–OSi bonds, which can interact with polar molecules through dipole–dipole interactions and hydrogen bonding, while the interaction with nonpolar molecules on the silica surface was weak.31 Similar studies also acknowledged the importance of N–H and O–H in PMS adsorption.32 Cholesterol molecules, being weakly polar, can enhance their interaction with PMS, making PMS more prone to adsorb cholesterol. This was consistent with our research findings, which demonstrated that PMS exhibited high adsorption capacity for weakly polar molecules such as CHO. Our FT-IR and XPS further supported this possibility. (2) The role of surface charge: although CHO and TG themselves were uncharged, they may acquire positive or negative charges upon dissolution, while PMS surfaces carry negative charges, which led to mutual attraction.32 In our study, further zeta potential measurements confirmed the findings.
Regarding the cholesterol, a message was also conveyed that mesoporous silicas performed well in CHO separation, which was associated with 10–20, 40–60, and 50–65% of removal efficiencies for powder, crystal, and emulsion forms respectively.33 More importantly, concerning the safety of PMS in clinical trials, no severe adverse events occurred in participants intaking mesoporous silicas (≥9 g per day) according to one single blinded safety study consisting of 20 males, which demonstrated the safety and tolerance of mesoporous silicas in humans.34
Limitation
A study we conducted showed that PMS was associated with enhanced CHO and TG adsorption, whereas most of these compounds were found in the form of lipoproteins in the blood. Consequently, PMS would affect the protein level in the blood when it was used to adsorb CHO and TG.
Moreover, we evaluated the effect of temperature on the capacity of PMS to adsorb CHO and TG, however, we did not assess the influence of pH, since acidic and alkaline environments would both contribute to the breakdown of CHO and TG.
In addition, no animal studies were conducted in our study to demonstrate the effects of PMS on lipid levels and the development of atherosclerosis. There is a need for further research to verify these findings.
Evaluation of the lipid-lowering effect of PMS in peripheral blood in vivo was constrained by the extended co-incubation time, which may give rise to thrombus formation and coagulation, impeding the interaction between PMS and lipids. As a result, the lipid-lowering effect of PMS in peripheral demonstrated in vivo was not prominently observed.
Conclusion
In this study, we have demonstrated a novel method for lowering blood lipids, which was safe, effective and recyclable. It was concluded that PMS was associated with reduced levels of blood lipids, which might be beneficial to hyperlipemia patients suffering from potential negative effects and prone to cardiovascular events. Additionally, most of the adsorbed blood lipids by PMS can also be released and extracted, contributing to the recovery and recycling of PMS.
Data availability
The authors declare that all the data in this manuscript are available upon request.
Author contributions
Jin and Lu conceived the experiments. Jin, Zhang and Wu conducted the experiments; Jin and Lu did data analysis and interpretation. All authors discussed the results.
Conflicts of interest
The authors declare no competing interests.
Acknowledgements
This work was supported by “The key research and development program of Jiangsu Province” (BE2021735) and the Youth Medical Talents Project of Jiangsu Province (No. QNRC2016814). We are grateful to the State Key Experiment in Biomedical Engineering at Southeast University for providing the PMS.
References
- S. S. Virani, A. Alonso, E. J. Benjamin, M. S. Bittencourt, C. W. Callaway, A. P. Carson, A. M. Chamberlain, A. R. Chang, S. Cheng, F. N. Delling, L. Djousse, M. S. V. Elkind, J. F. Ferguson, M. Fornage, S. S. Khan, B. M. Kissela, K. L. Knutson, T. W. Kwan, D. T. Lackland, T. T. Lewis, J. H. Lichtman, C. T. Longenecker, M. S. Loop, P. L. Lutsey, S. S. Martin, K. Matsushita, A. E. Moran, M. E. Mussolino, A. M. Perak, W. D. Rosamond, G. A. Roth, U. K. A. Sampson, G. M. Satou, E. B. Schroeder, S. H. Shah, C. M. Shay, N. L. Spartano, A. Stokes, D. L. Tirschwell, L. B. VanWagner and C. W. Tsao, Circulation, 2020, 141(9), e139–e596 CrossRef PubMed.
- S. Fischer, U. Schatz and U. Julius, Atheroscler. Suppl., 2015, 18, 194–198 CrossRef CAS PubMed.
- Ž. Reiner, Nat. Rev. Cardiol., 2017, 14(7), 401–411 CrossRef PubMed.
- M. Arca, C. Veronesi, L. D'Erasmo, C. Borghi, F. Colivicchi, G. M. De Ferrari, G. Desideri, R. Pontremoli, P. L. Temporelli, V. Perrone and L. Degli Esposti, J. Am. Heart Assoc., 2020, 9(19), e015801 CrossRef CAS PubMed.
- D. Zhang, G. Wang, J. Fang and C. Mercado, Med. Care, 2017, 55(1), 4–11 CrossRef PubMed.
- S. M. Grundy, N. J. Stone, A. L. Bailey, C. Beam, K. K. Birtcher, R. S. Blumenthal, L. T. Braun, S. de Ferranti, J. Faiella-Tommasino, D. E. Forman, R. Goldberg, P. A. Heidenreich, M. A. Hlatky, D. W. Jones, D. Lloyd-Jones, N. Lopez-Pajares, C. E. Ndumele, C. E. Orringer, C. A. Peralta, J. J. Saseen, S. C. Smith Jr, L. Sperling, S. S. Virani and J. Yeboah, Circulation, 2019, 139(25), e1082–e1143 Search PubMed.
- T. J. Dening, P. Joyce, M. Kovalainen, H. Gustafsson and C. A. Prestidge, Pharm. Res., 2018, 36(1), 21 CrossRef PubMed.
- A. Vuorio, J. Kuoppala, P. T. Kovanen, S. E. Humphries, S. Tonstad, A. Wiegman, E. Drogari and U. Ramaswami, Cochrane Database Syst Rev, 2019, 2019(11), CD006401 Search PubMed.
- J. Pedro-Botet and X. Pintó, Clin. Invest. Arterios., 2019, 31(Suppl 2), 16–27 Search PubMed.
- J. Vidé, A. Virsolvy, C. Romain, J. Ramos, N. Jouy, S. Richard, J. P. Cristol, S. Gaillet and J. M. Rouanet, Nutrition, 2015, 31(9), 1148–1154 CrossRef PubMed.
- A. Garcimartín, J. A. Santos-López, S. Bastida, J. Benedí and F. J. Sánchez-Muniz, J. Nutr., 2015, 145(9), 2039–2045 CrossRef PubMed.
- Z. Xie, E. J. Henderson, Ö. Dag, W. Wang, J. E. Lofgreen, C. Kübel, T. Scherer, P. M. Brodersen, Z. Z. Gu and G. A. Ozin, J. Am. Chem. Soc., 2011, 133(13), 5094–5102 CrossRef CAS PubMed.
- R. Schmid, N. Neffgen and M. Lindén, J. Colloid Interface Sci., 2023, 640, 961–974 CrossRef CAS PubMed.
- D. Wang, X. Chen, J. Feng and M. Sun, J. Chromatogr. A, 2022, 1675, 463157 CrossRef CAS PubMed.
- M. Kang, J. T. Lee and J. Y. Bae, Int. J. Mol. Med. Adv. Sci., 2023, 24(4), 4208 CAS.
- Z. Xiao, H. Bao, S. Jia, Y. Bao, Y. Niu and X. Kou, Molecules, 2021, 26(9), 2744 CrossRef CAS PubMed.
- J. Tu, A. L. Boyle, H. Friedrich, P. H. Bomans, J. Bussmann, N. A. Sommerdijk, W. Jiskoot and A. Kros, ACS Appl. Mater. Interfaces, 2016, 8(47), 32211–32219 CrossRef CAS PubMed.
- P. Joyce, T. J. Dening, T. R. Meola, A. Wignall, H. Ulmefors, M. Kovalainen and C. A. Prestidge, ACS Appl. Bio Mater., 2020, 3(11), 7779–7788 CrossRef CAS PubMed.
- S. S. Hate, S. M. Reutzel-Edens and L. S. Taylor, Pharm. Res., 2020, 37(8), 163 CrossRef CAS PubMed.
- F. Xie, Z. Xie, P. Liu, Y. Yuan, J. Li, S. Chen, F. Guo, J. Chen and Z. J. M. Gu, Microporous Mesoporous Mater., 2018, S1387181118301197 Search PubMed.
- K. Trzeciak, A. Chotera-Ouda, S. Bak II and M. Potrzebowski, J. Pharm., 2021, 13(7), 950 CAS.
- M. Lindén, Enzymes, 2018, 43, 155–180 Search PubMed.
- Z. Xie, L. Bai, S. Huang, C. Zhu, Y. Zhao and Z. Z. Gu, J. Am. Chem. Soc., 2014, 136(4), 1178–1181 CrossRef CAS PubMed.
- L. Pan, Q. He, J. Liu, Y. Chen, M. Ma, L. Zhang and J. Shi, J. Am. Chem. Soc., 2012, 134(13), 5722–5725 CrossRef CAS PubMed.
- Z. Xie, E. J. Henderson, Ö. Dag, W. Wang, J. E. Lofgreen, C. Kübel, T. Scherer, P. M. Brodersen, Z.-Z. Gu and G. A. Ozin, J. Am. Chem. Soc., 2011, 133(13), 5094–5102 CrossRef CAS PubMed.
- Y. Zhou, G. Quan, Q. Wu, X. Zhang, B. Niu, B. Wu, Y. Huang, X. Pan and C. Wu, Acta Pharm. Sin. B, 2018, 8(2), 165–177 CrossRef PubMed.
- N. Kupferschmidt, R. I. Csikasz, L. Ballell, T. Bengtsson and A. E. Garcia-Bennett, Nanomedicine, 2014, 9(9), 1353–1362 CrossRef CAS PubMed.
- M. Rinde, N. Kupferschmidt, M. N. Iqbal, G. Robert-Nicoud, E. V. Johnston, M. Lindgren and T. Bengtsson, Nanomedicine, 2020, 15(2), 131–144 CrossRef CAS PubMed.
- L. M. Jurkić, I. Cepanec, S. K. Pavelić and K. Pavelić, Nutr. Metab., 2013, 10(1), 2 CrossRef PubMed.
- H. Martínez Pérez-Cejuela, I. Ten-Doménech, J. El Haskouri, P. Amorós, E. F. Simó-Alfonso and J. M. Herrero-Martínez, Anal. Bioanal. Chem., 2018, 410(20), 4847–4854 CrossRef PubMed.
- P. Samaddar, J. Hu, N. Barua, Y. Wang, T. A. Lee, M. Prodanović, Z. Heidari and T. Hutter, ACS Omega, 2022, 7(47), 43130–43138 CrossRef CAS PubMed.
- T. Hua, D. Li, X. Li, J. Lin, J. Niu, J. Cheng, X. Zhou and Y. Hu, Environ. Res., 2022, 215(Pt 3), 114433 CrossRef CAS PubMed.
- A. Sinha, S. Basiruddin, A. Chakraborty and N. R. Jana, ACS Appl. Mater. Interfaces, 2015, 7(2), 1340–1347 CrossRef CAS PubMed.
- E. Hagman, A. Elimam, N. Kupferschmidt, K. Ekbom, S. Rössner, M. N. Iqbal, E. Johnston, M. Lindgren, T. Bengtsson and P. Danielsson, PLoS One, 2020, 15(10), e0240030 CrossRef CAS PubMed.
|
This journal is © The Royal Society of Chemistry 2023 |
Click here to see how this site uses Cookies. View our privacy policy here.