Nanosilicon alters oxidative stress and defence reactions in plants: a meta-analysis, mechanism and perspective†
Received
18th May 2022
, Accepted 4th August 2022
First published on 9th August 2022
Abstract
Alleviating the adverse effects of abiotic and biotic stress factors on crop plants is essential to achieve higher productivity for satisfying the future food demands of the rapidly-growing global human population. The application of Si nanomaterials (Si NMs) could be an ecologically-sound alternative, as there is evidence that Si NMs could positively mitigate oxidative stress in plants. However, not all studies report clear positive effects of Si NM amendment, suggesting a high level of context-dependency. To explore variation in Si NM benefits on plants, we conducted a meta-analysis of 51 independent studies conducted worldwide. We found that Si NM addition overall increased antioxidant enzyme activities (16.3%), antioxidant metabolite contents (15.2%) and defence-related gene expression (178.2%), overall decreasing oxidative stress in plant by 24.0%. These effects varied depending on several factors such as application dose, application duration and application method. Moreover, we observed that Si NM-mediated effects on plant physiology could last several weeks, but this depended on the application dose of Si NMs. Therefore, we conclude that Si NMs could be further developed as an efficient and environmentally-friendly alternative to synthetic agrochemicals for plant stress mitigation.
Environmental significance
The application of Si nanomaterials (Si NMs) could be an ecologically-sound alternative to pesticides and synthetic fertilizers. By means of a quantitative meta-analysis, we studied the context-dependency of Si NM-mediated effects on plant anti-oxidative responses in relation to application dose, culture medium, and other factors. By showing widespread benefits of Si NM application, either at the level of gene expression or at the level of antioxidant molecules, we suggest that Si NMs could be further developed as an efficient and environmentally-friendly alternative to synthetic agrochemicals for protecting plants against oxidative stress under various abiotic and biotic stress conditions.
|
1. Introduction
Due to global warming, land-use change and agricultural intensification, crops typically face an increased number of abiotic (e.g., drought, heavy metal contamination, salinity and organic pollutant) and biotic (e.g., disease, herbivores and parasitic weed) challenges, which can majorly affect crop production and quality, ultimately threatening global food security.1,2 Over the past few decades, pesticides and fertilizers have been extensively used to respond to the high food demands worldwide.3,4 However, the heavy application of these agrochemicals poses substantial threats to the environment and biodiversity.5–7 Therefore, developing sustainable and environmentally-friendly technologies is urgently needed to assist plants in mitigating abiotic and biotic stresses for enhancing crop yield.
Generally, abiotic or biotic stresses spur the overproduction of reactive oxygen species (ROS), such as H2O2, O2˙− and ˙OH, which could damage plant cells.8 To cope with ROS damage, plants can activate a battery of antioxidant enzymes (e.g., SOD and POD), or non-enzymatic antioxidant metabolites (e.g., tocopherol, flavonoids, and glycine betaine).9–11 In this context, the application of nanomaterials (NMs), including TiO2 NMs, ZnO NMs, and SiO2 NMs, has been shown to stimulate the expression of stress response genes and the production of antioxidants, and thus could enhance plant resistance to both abiotic and biotic stresses.12–15 By inducing the activity of key defence enzymes (e.g., PPO and PAL) and the expression of defence-related genes (e.g., PPO, PR1, and HMA1) in response to a variety of stresses, NMs can therefore boost the immune response of plants to inhibit potential cell damage caused by ROS.2,15,16 The efficacy of NMs in activating plant responses also partially relies on their small size, which allows them to relatively easily pass through plant cell walls, and thus directly impact their physiology, more effectively than other plant stimulants.17
One major element of the Earth's crust is silicon (Si), and the application of bulk Si, as well as its nanoform, has been shown to increase and sustain plant growth.18–20 Si MNs have indeed been shown to activate the antioxidant system of plants, in turn reducing oxidative stress, and ultimately enhancing plant resistance against abiotic21–24 and biotic stresses.16,25 Therefore, the application of Si NMs in mitigating plant stress and promoting plant growth has attracted extensive attention. However, it seems that Si NM application can be beneficial, but also be ineffective,16,21 or even cause harm,17 to the plant defence system, depending on the dose and duration of application, or on the system under investigation. For example, foliar spray with both 0.5 mM and 1 mM nano-SiO2 (20–30 nm) increased the activities of CAT, POD, SOD and APX in soybean under salinity stress conditions, but 2 mM nano-SiO2 inhibited these antioxidant enzyme activities.22 Under heavy metal stress, root-applied 3 mg kg−1 nano-SiO2 for 14 days had no effect on AsA and DHA content,26 whereas root-applied for 21 days increased phenolics, flavonoids and AsA content.10 Different growth media also affect the performance of Si NMs. For instance, under arsenic stress conditions, foliar application of nano-Si (20–30 nm) increased GSH contents in hydroponic rice,27 but did not change GSH and phenol contents in soil-grown tomato plants.28 Concerning biotic pressure, it was for instance shown that the foliar application of 50 mg L−1 Si nanodots (8 nm) for 14 days significantly increased the expression of defence-related genes such as PAL, CAL, HCT, C4H and C3H, which induced the production of chlorogenic acid biosynthesis, and finally inhibited the growth of the oriental armyworm (Mythimna separata) caterpillars.16 On the other hand, foliar application of 500 mg L−1 mesoporous silica nanoparticles (MSN, 35 nm) inhibited the expression of defence-related genes (PAO, PPO, and PR1) under fungal disease stress conditions.29 Moreover, it has reported that surface charge and surface chemical groups of Si NMs are also generally related to the absorption and solubility of Si NMs,30,31 which in turn could affect their bioavailability and biological regulatory effects on plants.32,33 Taken together, all these observations indicate that the ability of Si NMs to protect plants against various stressors depends not only on plant growth media, but also on the particle size, types, application methods, application dose and application duration of Si NMs.34
With this work, we aimed to address such widespread context-dependency by a quantitative meta-analysis of the literature reporting on the effects of Si NM addition on oxidative stress and defence reactions in plants. We specifically addressed the following questions: i) does Si NM application improve the defence system of plants so as to reduce their oxidative stress? ii) How do different factors (e.g., growth media, Si NM types, application methods, particle sizes, application dose and application duration of Si NMs) influence the effect of Si NMs on plant oxidative stress and their defence systems under stress conditions? iii) What are the defence modes and mechanisms of plants to cope with oxidative stress when added with Si NMs? This work provides a comprehensive understanding of Si NM-mediated effects on plant defence against oxidative stress, and promotes the application of Si NMs for plant protection against abiotic and biotic stress for food security and sustainable agricultural development.
2. Method
2.1 Literature retrieval and data collection
We conducted a literature search using ISI Web of Science and Google Scholar with the combination of relevant terms: “Silicon nanomaterials OR Silica nanomaterials OR Si nanomaterials OR SiO2 nanomaterials OR Silicon nanoparticles OR Silica nanoparticles OR Si nanoparticles OR SiO2 nanoparticles” AND “plant stress” up to January 2022. Relevant peer-reviewed publications were retained according to the following criterias: i) the study reported on experiments designed to test the effect of Si NMs on plant stress response traits under abiotic or biotic stress conditions; ii) the experiments included the measurement of at least one plant stress response traits, which we classified as plant stress indicators (e.g., oxidative stress), or plant defence indicators (e.g., antioxidant enzymes, antioxidant metabolites, defence enzymes, and defence-related genes); iii) the studies reported the mean, standard deviation (SD) or standard error (SE), and at least three independent replicates for control (without application of Si NMs) and treatment groups (with application of Si NMs). In total, from an initial selection of 313 papers, we finally retained 51 original research papers that met all criterias and that were included in the meta-analysis (Appendix S1†). From each selected publication, we extracted the information on the particle size, application dose, application method and application duration of Si NMs, and mean value of plant growth parameter, plant oxidative stress indicators (e.g., H2O2, O2˙−, ˙OH, MDA, EL), antioxidant enzyme (e.g., SOD, CAT, POD, APX, MDHAR, DHAR, GPX, GST, GR, GPOX) activity, defence enzyme (e.g., PPO, PAL) activity, the abundance of antioxidant metabolites (e.g., proline, phenolics, flavonoids, tocopherol, glycine betaine, GSSG, GSH, DHA, and AsA), and the expression of defence-related genes (e.g., USP, PR1, PPO, PAO, PAL, and HMA1). Additionally, a group of data-points for plant defence response parameters under non-stress conditions was also extracted when available. For each observation, we extracted the sample size (n) of each endpoint and SD/SE as well. The reported SE was converted to SD by the following formula: SD = SE × sqrt (n). For the graphic data, the GetData Graph Digitizer V2.26 was used to extract each data point (https://www.getdata-graph-digitizer.com).
To evaluate the context dependency effects of Si NMs on plant oxidative stress and defence reactions against stress, we further collected the data of the six commonly-reported moderating (categorical) variables from each study, including plant growth media, Si NM type, application method, Si NM size, application dose, and application duration of Si NMs (Fig. 1). Notably, the data of Si NM size extracted from the published studies were measured using three different methods, including TEM (transmission electron microscopy, n = 1094, 78.5%), SEM (scanning electron microscopy, n = 215, 15.4%), DLS (dynamic light scattering, n = 85, 6.1%) under stress conditions. Since the size of the Si NMs determined by different methods (e.g., TEM, SEM, and DLS) might result in variation of the measured nanoparticle size, for this meta-analysis related to Si NM size, we selected the most common type of data, measured by TEM. For the application dose metric, since concentrations (mg kg−1 or mg L−1) of Si NMs are regularly reported in the literature rather than absolute amounts, we unified the application dose units as ppm level. However, due to the limited information of dose-related parameters, including the number of particles, suspension volume, and leaf surface area metrics in the published literature, we did not further calculate the absolute amounts of Si NMs for the application dose metric in this study.
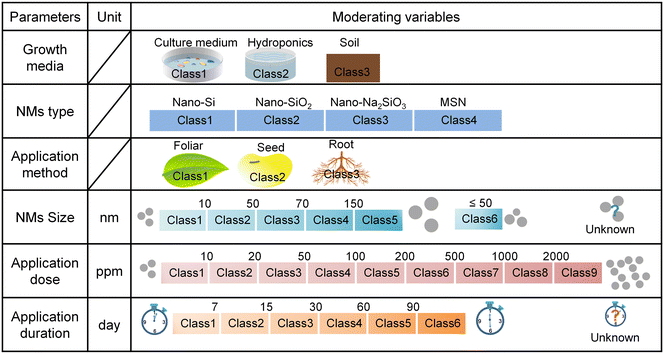 |
| Fig. 1 Graphical summary of the moderating variables that were retained to measure the context-dependency of Si NM-mediated effects on plant oxidative stress and defence reactions. “Unknown” was included when the publication did not include the relevant information. | |
2.2 Meta-analysis
We used the natural logarithm of the response ratio (lnRR) to assess the impact of Si NMs on oxidative stress and defence reactions in plants under stress conditions,35 and the effect size was estimated by the following equation: lnRR = ln(MT/MC), where MT and MC represent mean values with (treatment group) and without (control group) addition of Si NMs, respectively. To interpret the results, the formula: (elnRR − 1) × 100% was used to convert the estimated size effect to percentage (%).36 The variance (v) of each lnRR was calculated as:
where SDT and nT represent the SD and sample sizes of the treatment group, respectively, while SDC and nC represent the SD and sample sizes of the control group, respectively.
All statistical analyses were conducted in the R environment37 using the “metafor” package.38 The Si NM-mediated overall effect size for plant oxidative stress and defence response parameters (e.g., antioxidant enzyme activity, antioxidant metabolite content, defence enzyme activity, and defence-related gene expression) was assessed using the random-effect model with the REML method.39 Publication bias in this meta-analysis was estimated by Egger's test40 and the “trim and fill” method.41 To test the robustness of the effect of Si NM addition on oxidative stress and defence reactions in plants against stress, the fail-safe numbers (Nfs) of the overall database was assessed by Rosenberg's method.42
To test how the six moderating variables affected the Si NM-mediated changes in plant defence reactions against oxidative stress, we first performed a linear regression analysis (i.e., analyze all the six selected moderating variables together in the multiple regression model), and secondly, a random forest analysis, to determine the variables having the largest relative contribution to the Si NM-mediated effects on plant oxidative stress and defence reactions across all studies. We further did the meta-regression analysis to test the impacts of the two most salient moderating variables on the Si NM-mediated effects, and moderator analysis was also conducted with the moderator categorical variables used in this final analysis containing at least two levels and each level had more than three sample sizes (n ≥ 3). The difference between levels was estimated by between-group heterogeneity (Qb) analysis.39
3. Results
3.1 Research trends in Si NM-mediated effects on plant defence against oxidative stress
Overall, in this meta-analysis, the first study investigating the impact of Si-NMs on plant defence against oxidative stress was published in 2015. Subsequently, research interest in this area increased rapidly since 2018, with 22 publications in 2021 and with 4 publications in the first month of 2022 (Fig. 2a). The studies of Si NM-mediated plant defence against oxidative stress mainly focused on the heavy metal stress (40.3%), followed by salinity (20.4%), drought (11.8%), cold (6.1%), organic pollutant (2.9%), UV-B (1.8%), combined heavy metal and salinity (1.6%), and hypoxia stress (1.5%), combined drought and salinity (1.4%), and ultimately, fluoride stress (0.9%) (Fig. 2b). In addition, only 11.3% of the studies focused on biotic stressors, in which plant pathogens were the most studied biotic stress factor (7.0%), followed by crop–weed competition (3.2%) and herbivory (1.1%) (Fig. 2b). Concerning plant growth media, 67.0% of observations were conducted in soil, whereas 21.5% and 11.5% of the studies were conducted in hydroponic and culture medium, respectively (Fig. 2c). Regarding the type of Si NM applied, most studies focused on nano-SiO2 (61.8%), whereas the remainder of the studies used nano-Si (35.6%), nano-Na2SiO3 (1.6%) or MSN (1.0%) (Fig. 2d). For the application method, Si NMs were most applied through root exposure (49.6%), followed by foliar spray (39.1%) and seed priming method (11.3%) (Fig. 2e).
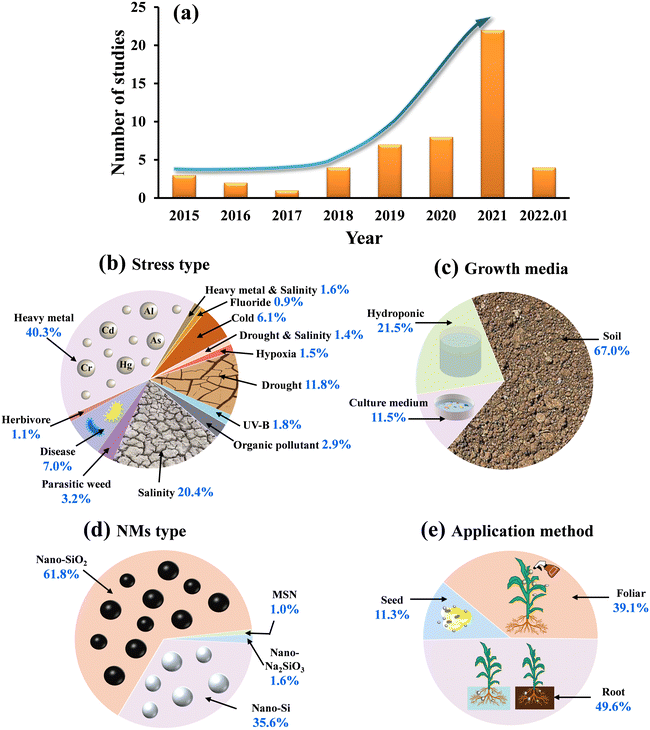 |
| Fig. 2 Data summary of the studies used in the meta-analysis. (a) Number of published studies that measured the impact of Si NMs on plant stress until January 2022. (b–e) Pie charts showing the relative ratios of the different stressors under investigation, the growth media, the type of Si NM application, and the application method, respectively, used across all studies. | |
3.2 Overall effect of Si NMs on plant oxidative stress, defence responses and growth
Across all observations, we found that Si NM addition significantly decreased oxidative stress in plants by 24.0% under stress conditions (Fig. 3), while by 4.7% under non-stress conditions (Fig. S1†). Moreover, Si NM application decreased oxidative stress in plants by 23.7% and 27.6% under abiotic and biotic stress conditions, respectively, but such an inhibitory effect was not significantly different between abiotic and biotic stress (p = 0.392, Fig. S2†), we thus pooled the data under abiotic and biotic conditions together for all subsequent analyses. Specifically, Si NM application significantly decreased oxidative stress by 23.6%, 21.6%, 18.1%, 32.3%, 67.2%, 31.0%, 14.6%, 26.0%, 21.3%, and 38.3% when plants grew under conditions of heavy metal pollution, salinity, drought, heavy metal & salinity, hypoxia, UV-B, organic pollutant, drought & salinity, disease and parasitic weed stress, respectively (Table S1†).
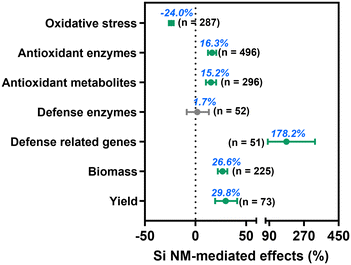 |
| Fig. 3 Overall effect of Si NM addition on plant stress response and growth when under stress. Sample size is shown in brackets. The significant individual effect with 95% CI (horizontal bar) is highlighted in green (p < 0.05). | |
Regarding the plant defence system, we found that Si NM addition, across all studies significantly enhanced the activity of antioxidant enzymes, the concentration of antioxidant metabolites, and the expression of defence-related genes by 16.3%, 15.2% and 178.2%, respectively, but had an overall non-significant effect on defence enzyme activity (Fig. 3, Table S2†). Particularly, Si NM addition increased plant antioxidant enzyme activity and antioxidant metabolite content against drought stress (by 44.1% and 20.9%), heavy metal stress (by 13.1% and 11.5%), hypoxia stress (by 62.2% and 119.7%) and parasitic weed stress (by 43.1% and 82.3%), respectively (Table S1†). By using the “trim and fill” method to assess the mean effect size of Si NM-mediated oxidative stress and antioxidant metabolites under stress conditions, we found that there was no missing data for the grand mean effect size, indicating non-significant publication bias (Table S2†). While the Egger's test results indicated asymmetry for the Si NM-mediated effect on oxidative stress (z = −2.2, p = 0.029), antioxidant enzyme activities (z = 2.7, p = 0.008) and defence related-gene expression (z = −2.6, p = 0.010), the Rosenberg's Nfs for this database were over 5990, 7203 and 154 times higher than the corresponding threshold values, respectively, thus also indicating that the mean effect size was robust (Table S2†). Finally, we found that Si NM addition significantly increased plant biomass and yield by 26.6% and 29.8% under stress conditions, respectively (Fig. 3, Table S2†).
3.3 Impact of moderating variables on the Si NM-mediated effect on oxidative stress in plants
Overall, the Si NM-mediated effect on plant oxidative stress was significantly influenced by application dose, application duration and Si NM types, but was not influenced by the Si NM size, application method and growth media (Table S3†). The application dose and application duration acted as two of the most important drivers of Si NM-mediated effects on oxidative stress in plants under stress conditions through both linear regression analysis and random forest analysis approaches (Table S3,†Fig. 4a). Therefore, we further tested the temporal-dependence of the Si NM-mediated effect on oxidative stress in plants exposed to various application doses under stress conditions (Fig. 4b–f). Specifically, when the application duration of Si NMs lasted less than 15 days or over 90 days, Si NM-mediated effects on plant oxidative stress were not dependent on their application dose (Fig. 4b and f). However, when the application duration of Si NMs lasted from 15 to 90 days, the Si NM-mediated effects on plant oxidative stress were in a dose-dependent manner (Fig. 4c–e). Particularly, the effect of Si NMs on oxidative stress in plants was negatively correlated with their application dose when the application duration lasted for 15–30 days (Fig. 4c). However, the effect of Si NMs on oxidative stress in plants was positively correlated with their application dose when the application duration lasted for 30–60 days (Fig. 4d).
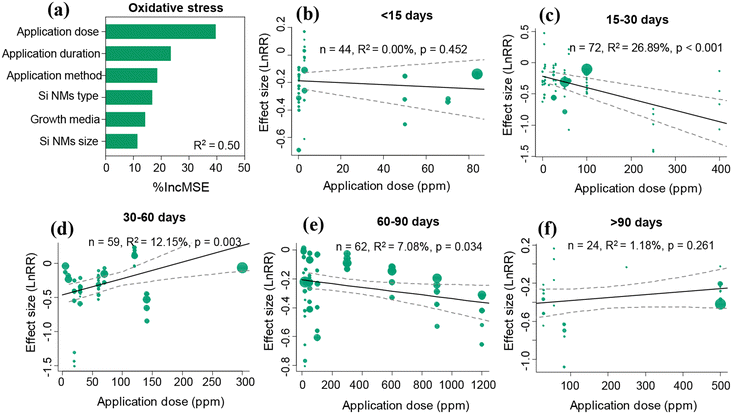 |
| Fig. 4 Variable importance of the moderators included for explaining variation in Si NM-mediated effects on the oxidative stress in plants under stress conditions (a). R2 is the variability of Si NM-mediated effects on plant oxidative stress explained by the random-forest model. Panels (b–f) show the results of the meta-regression analyses measuring the relationships between the Si NM-mediated effects on plant oxidative stress and their application dose across various application durations: <15, 15–30, 30–60, 60–90, and >90 days, respectively, under stress conditions. The size of the dots represents the relative weight of the associated variance of the effect size (lnRR) in the meta-regression. | |
3.4 Impact of moderating variables on the Si NM-mediated effect on antioxidant enzyme activities in plants
The Si NM-mediated effect on antioxidant enzyme activities under stress conditions was significantly influenced by growth media, Si NM size, Si NM type, application dose, and application duration, but not by the application method (Table S3†). Through both linear regression analysis and random forest analysis approaches, we found that the application dose and application duration were the two most important drivers of Si NM-mediated effects on the antioxidant enzyme activities in plants under stress conditions (Table S3,†Fig. 5a). Therefore, we further analysed the temporal-variations of the Si NM-mediated effect on the antioxidant enzyme activities in plants exposed to various application doses under stress conditions (Fig. 5b–f). We found that, only when the application duration lasted for 15–30 days or over 90 days, the Si NM-mediated effect on plant antioxidant enzyme activities was in a dose-dependent manner (Fig. 5c and f). Particularly, the effect of Si NMs on the antioxidant enzyme activities in plants negatively correlated with the application dose when their application duration lasted over 90 days (Fig. 5f). More specifically, when the application duration lasted over 90 days, the Si NM application at doses of 20–50 ppm and 50–100 ppm significantly increased the antioxidant enzyme activities in plants by 65.7% and 85.0%, respectively, while the increased application dose of 200–500 ppm and 500–1000 ppm notably decreased the antioxidant enzyme activities in plants by 20.5% and 23.8%, respectively (Fig. S3†).
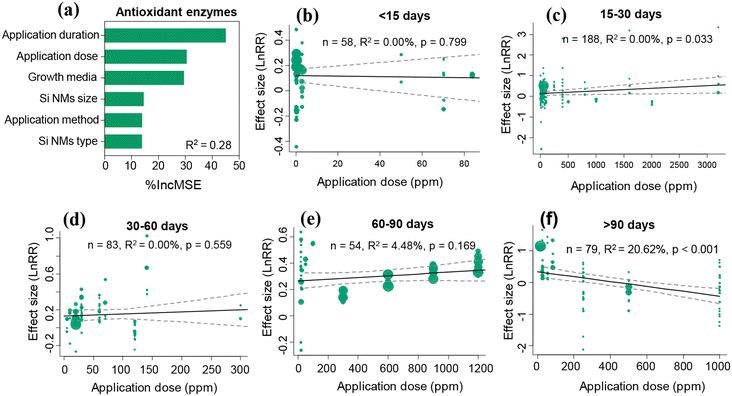 |
| Fig. 5 Variable importance of the moderators included for explaining variation in Si NM-mediated effects on the antioxidant enzyme activities in plants under stress conditions (a). R2 is the variability of the effects of Si NMs on the antioxidant enzyme activities in plants explained by the random-forest model. Panels (b–f) show the results of the meta-regression analyses measuring the relationships between the Si NM-mediated effects on plant antioxidant enzyme activities and their application dose across various application durations: <15, 15–30, 30–60, 60–90, and >90 days, respectively, under stress conditions. The size of the dots represents the relative weight of the associated variance of the effect size (lnRR) in the meta-regression. | |
3.5 Impact of moderating variables on the Si NM-mediated effect on plant antioxidant metabolites
The Si NM-mediated effect on the antioxidant metabolite content in plants under stress conditions was significantly influenced by the Si NM application method, application dose, and application duration, growth media, and Si NM type, but not by the Si NM size (Table S3†). The application dose and application method were the two most important drivers of Si NM-mediated effects on the antioxidant metabolite contents in plants under stress conditions based on the random forest analysis results (Fig. 6a). Therefore, we further tested the application method-dependence of Si NM-mediated effects on the antioxidant metabolite contents in plants exposed to various application doses under stress conditions (Fig. 6b–d). We found that the effect of Si NMs on plant antioxidant metabolite contents positively correlated with their application dose, when the application method was foliar exposure (Fig. 6b). However, the Si NM-mediated effects on plant oxidative stress were not significantly correlated to their application dose, when the application method was root exposure or seed exposure (Fig. 6c and d).
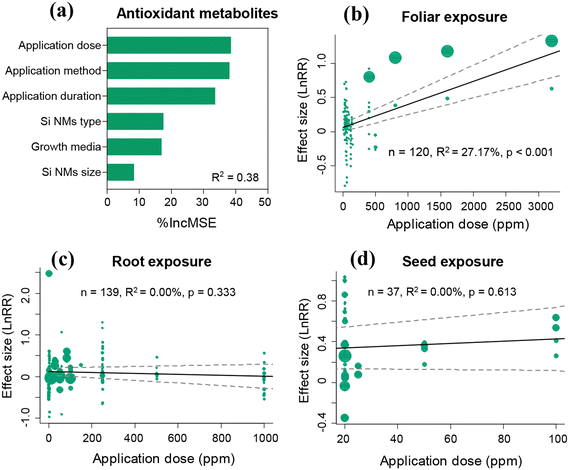 |
| Fig. 6 Variable importance of the moderators included for explaining variation in Si NM-mediated effects on the plant antioxidant metabolite contents under stress conditions (a). R2 is the variability of the effects of Si NMs on plant antioxidant metabolite content explained by the random-forest model. Panels (b–d) show the results of the meta-regression analyses measuring the relationships between the Si NM-mediated effects on plant antioxidant metabolite contents and their application dose across various application methods: foliar, root and seed exposure method, respectively, under stress conditions. The size of the dots represents the relative weight of the associated variance of the effect size (lnRR) in the meta-regression. | |
3.6 Impact of moderating variables on the Si NM-mediated effect on plant defence-related gene expression
Across all observations, Si NM-mediated effects on plant defence-related gene expression in plants under stress conditions were only influenced by the application method, with 53.5% of variance explained (Table S3†). This observation was corroborated by the random forest analysis, which also identified the application method as the most important predictor of Si NM-mediated effects on defence-related gene expression in plants under stress conditions (Fig. 7a). Therefore, we further only tested the application method-dependence of Si NM-mediated effect on defence-related gene expression in plants with moderator analysis (Fig. 7b). We found that the defence-related gene expression in plants was significantly up-regulated by 1152.1% with root exposure to Si NMs (Fig. 7b).
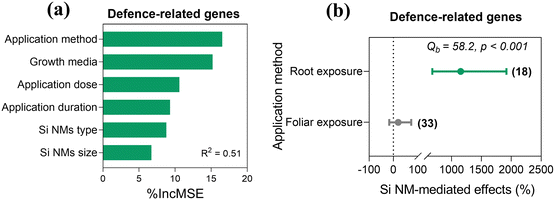 |
| Fig. 7 Variable importance of the moderators included for explaining variation in Si NM-mediated effects on the defence-related gene expression in plants under stress conditions (a). R2 is the variability of the effects of Si NMs on the defence-related gene expression in plants explained by the random-forest model. The impact of the application method on the Si NM-mediated effect on the expression of the defence-related genes in plants under stress conditions (b). Sample size is shown in brackets. The significant individual effect with a 95% CI (horizontal bar) is highlighted in green (p < 0.05). | |
3.7 Si NM-driven antioxidant defence mechanisms for mitigating oxidative stress in plants
To clarify how Si NM addition alters plant defence systems against different stressors, we further quantitatively evaluated the specific components of the plants' defence systems (Fig. 8, Table S4†). Across all observations, we observed that the Si NM application overall increased the activities of antioxidant enzyme systems, including SOD, CAT and POD, by 7.4%, 16.6% and 16.3%, respectively (Fig. 8, Table S4†). Regarding the AsA–GSH system, while the concentration of DHA generally decreased by 27.7%, other antioxidant enzyme activities and antioxidant metabolite contents increased by more than 16% after the addition of Si NMs (Fig. 8, Table S4†). Moreover, Si NM addition also induced a significant increase in plant non-enzymatic antioxidants, including flavonoids (16.9%), tocopherol (17.8%), phenolics (14.3%) and glycine betaine (53.5%) (Fig. 8, Table S4†). For the plant defence enzymes and defence-related genes, we found that Si NMs had no significant effect on PAL activity but they generated the up-regulation of the PAL genes by 436.9% (Fig. 8, Table S4†). Finally, we found that Si NMs up-regulated the expression of the defence-related genes HMA1 and USP by 336.4% and 2465.4%, respectively (Fig. 8, Table S4†). Consequently, O2˙− and H2O2 radicals were significantly decreased by 22.7% and 27.3% after the addition of Si NMs, which in turn inhibited the production of MDA and EL by 24.6% and 18.9%, respectively (Fig. 8, Table S4†).
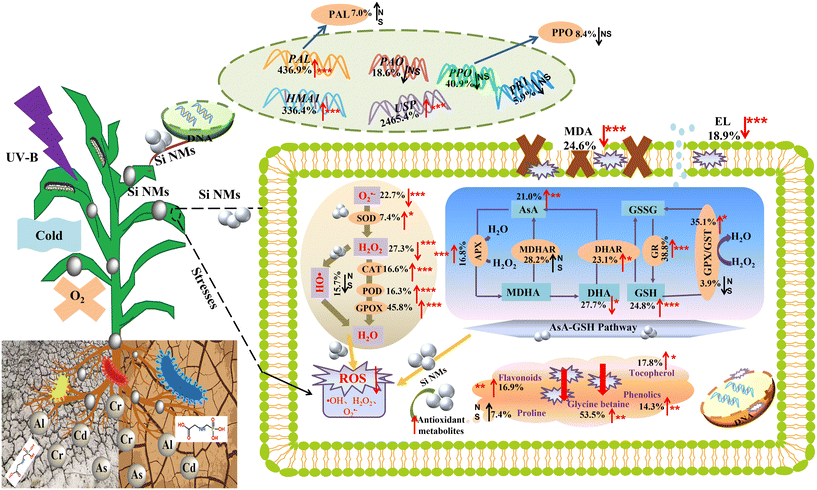 |
| Fig. 8 Mechanisms of Si NM-driven plant defences against abiotic and biotic stresses. Plant defence system components including: Antioxidant enzymes (e.g., SOD, CAT, POD, APX, MDHAR, DHAR, GPX, GST, GR, and GPOX); antioxidant metabolites (e.g., proline, phenolics, flavonoids, tocopherol, glycine betaine, GSSG, GSH, DHA, AsA, and MDHA); defence enzymes (e.g., PPO and PAL); defence-related genes (e.g., USP, PR1, PPO, PAO, PAL, and HMA1). “↑” and “↓” mean Si NM-mediated positive and negative effects on the specific components of plant defence against stress, respectively. *p < 0.05, **p < 0.01, ***p < 0.001 and NS non-significant. Abbreviations: superoxide radical (O2˙−), hydrogen peroxide (H2O2), hydroxyl radical (˙OH), malondialdehyde (MDA), electrolyte leakage (EL), ascorbic peroxidase (APX), catalase (CAT), dehydroascorbate reductase (DHAR), guaiacol peroxidase (GPOX), glutathione peroxidase (GPX), glutathione reductase (GR), glutathione S-transferase (GST), monodehydroascorbate reductase (MDHAR), peroxidase (POD), superoxide dismutase (SOD), ascorbic acid (AsA), dehydroascorbate (DHA), glutathione (GSH), oxidized glutathione (GSSH), phenylalanine ammonialyase (PAL), polyphenol oxidase (PPO), heavy metal ATPase1 (HMA1), polyamine oxidase (PAO), pathogenesis-related protein 1 (PR1), universal stress protein (USP). | |
4. Discussion
We found that the application of Si NMs can significantly inhibit oxidative stress in plants by activating their antioxidant defence systems, and in turn increase plant biomass and yield. However, the effect of Si NMs on plant stress mitigation varies depending on several variables such as plant growth media, the type, application method, particle size, application dose and application duration of Si NMs.
4.1 Si NM-driven effects on antioxidant enzyme systems in plants
Generally, considered as the front line of defence against ROS, SOD decomposes O2˙− into less toxic H2O2, and then CAT and POD degrade H2O2 into H2O and O2.43 Moreover, antioxidant enzymes pertaining to the AsA–GSH cycle (APX, MDHAR, DHAR, GR, GST, and GPX) further contribute to scavenging H2O2 from the chloroplast and cytosol, and modulate ROS homeostasis under stress conditions.25,44 Our meta-analysis revealed that Si NMs can activate several of these antioxidant enzymes in plants under stress conditions, suggesting that plants after exposure to Si NMs activate a complex network of antioxidant enzymes that can act cooperatively or synergistically to cope with oxidative stress.
The efficiency of NMs strongly depends on their bioavailability for plants, which is directly related to their application dose.45,46 Accordingly, through variable selected analyses, we found that the application dose parameter was always among the most important drivers of Si NM-mediated effects on antioxidant enzymes activities, but such effects were temporally variable. Particularly, when the exposure duration lasted for a long time (i.e., >90 days), the regulatory effects of Si NMs on antioxidant enzyme activities showed a tendency of low promotion and high inhibition (Fig. S3†). Correspondingly, we also observed that plant oxidative stress was more reduced at the low application dose (20–50 ppm) of Si NMs (Fig. S4a†), and this effect was more pronounced when Si NMs were applied at the dose of 10–20 ppm for intermediate duration (e.g., 30–60 days) (Fig. S4b†). It has been shown that NMs applied at high concentrations would result in an over-accumulation of NMs in plant tissues, and even an agglomeration of the NMs in the cells, which in turn would inhibit their regulatory functions. For example, under high salinity conditions, foliar spray with 30 ppm (0.5 mM) nano-SiO2 (10–35 nm) for 43 days increased APX, CAT, SOD and POD activities in soybean grown in soil,22 while 500 ppm nano-SiO2 (10 nm) for 100 days significantly decreased the CAT, SOD and POD activities.47 In addition, the effect of Si NMs on antioxidant enzyme activities in plants was also affected to some extent by the plant growth media. Specifically, we also observed that Si NM addition mostly activated antioxidant enzyme activity when plants grew in the hydroponic and soil media, but not when plants grew in culture medium (Fig. S5†). We suggest that the acid–base balance of the culture medium, along with the non-uniform composition of the medium, could have led to the aggregation of Si NMs, which in turn reduced their bioavailability.48 Accordingly, Ozturk et al. (2020) showed that the pH and the homogeneity of the medium were the two major factors influencing the aggregation of NMs.49 Moreover, Nafee et al. (2009) reported that the heterogeneous composition of the cell culture medium, rich in amino acids, proteins, electrolytes and other organic macromolecules, favoured the aggregation of NMs, and reduced the bioavailability of NMs.50 Therefore, to summarize, even long-term Si-NM exposure can spur the antioxidant enzyme systems and alleviate oxidative stress, but this depends on the application dose and growing medium.
4.2 Si NM-driven effects on antioxidant metabolite systems in plants
In addition to antioxidant enzyme systems, non-enzymatic antioxidant metabolites, including AsA, GSH, GSSG, DHA, MDHA, flavonoids, phenolics, tocopherol, proline and glycine betaine, can also function as direct scavengers of ROS.9,51 Across all studies, we found that Si NM addition overall increased the AsA content, but it reduced the DHA content in plants. During the catalysis of DHAR, DHA is converted into AsA. High AsA/DHA ratios have been proven beneficial for restoring the redox status of cells under oxidative stress.52 Moreover, AsA is regarded as the most potent ROS scavenger.53 Accordingly, it is necessary for plants to maintain high AsA levels in order to alleviate oxidative stress and modulate multiple metabolic processes.25 For instance, AsA can transform tocopherol radicals into tocopherols for membrane protection.53 Tocopherols can scavenge ROS produced in the thylakoid membranes, in turn inhibiting the production of MDA and preventing lipid peroxidation.54
The antioxidant metabolite content after Si NM addition was mostly driven by the application dose and method of Si NMs. Particularly, the antioxidant metabolite contents in plants with foliar exposure to Si NMs were positively correlated with the application dose of Si NM, which was related to the observation that foliar application of Si NMs at the high dose of 200–500 ppm still increased the antioxidant metabolite contents in plants by 24.6% (Fig. S6†). However, this result is somewhat in opposition with what was discussed above for antioxidant defence enzyme systems, in which long-term Si NM application and at high concentration (e.g., 200–500 ppm) inhibited the activity of antioxidant enzymes.22,47 To understand such response variability between the antioxidant enzyme and metabolite systems, we advocate for more mechanistic studies, including the use of plant knock-out mutants, coupled with transcriptomics and metabolomics analyses.
Additionally, regardless of other factors, we found that different application methods of Si NMs generated different antioxidant metabolic responses in plants across all studies (Fig. S7†). Particularly, we observed that seed priming Si NMs spurred more antioxidant metabolites than foliar- and root-Si NM addition under both stress and non-stress conditions (Fig. S7a and b†), indicating that the Si NM-mediated promotion effects on plant metabolites were not dependent on stress conditions but were dependent on the application method. It has been reported that seed priming could alter plant metabolism and signalling pathways, which would influence not only the germination processes, but also the whole life-cycle of plants.55 Seed priming allows NMs to stimulate specific physiological states of plant prior to sowing.56 For example, El-Serafy et al. (2021) showed that Si NM seed-primed Lathyrus odoratus plants contained higher levels of proline, phenolics and flavonoids under salinity stress,57 while root applied Si did not up-regulated flavonoids and AsA.53 Moreover, although application duration was not the one of the top two moderating factors across all studies based on this meta-analysis, it also significantly affected the regulation of Si NMs on plant metabolism to a certain extent. Interestingly, we found that Si NM exposure lasting for a long time (e.g., >60 days) could also be effective for mediating the accumulation of plant antioxidant metabolites under both stress and non-stress conditions (Fig. S8a and b†), suggesting that the Si NM-mediated promotion effects on plant metabolites is independent of stress conditions. In accordance with the above results, Minoo et al. (2021) demonstrated that nano-Si addition for 100 days improved the phenolic content, flavonoid content, water content and total soluble sugars, thus promoting coriander (Coriandrum sativum L.) growth and yield under drought stress.58 Taken together, these results confirm that the antioxidant effect of metabolites can be activated by Si NMs, but again to some extent, while these effects can last over time, they are dose- and mode of application-dependent.
4.3 Si NM-driven effects on defence-related gene systems in plants
At the core of the plant defence responses is the expression of defence-related genes.59 Across all studies, we found that Si NM addition significantly enhanced the expression of the PAL gene. However, we found no direct correlation between the expression of this gene and enzyme activity, suggesting that posttranscriptional and posttranslational modifications are at play.60 Additionally, across all studies we found that Si NMs also had no significant effect on the expression of PAO and PR1, but induced the overexpression of HMA1 and USP. However, these results should be taken with caution, since the high variability in the experimental systems under investigation could intrinsically generate variation in gene expression responses that vary across systems in a non-systematic fashion.29
Moreover, we found that, when compared with leaf exposure, root exposure overall had a more pronounced effect on the defence-related gene expression in plants under stress conditions. We speculate that this result might be driven by the lower absorption of Si-NMs in leaf tissues than in root tissues.61 Moreover, roots release many exudates, which could form complexes with NMs and then bind to membrane transporters or carrier proteins through ion channels and water channels, or are endocytosed.56
The efficacy of NMs in mediating gene expression can also depend on the type of Si NMs applied.15 Since plants cannot directly utilize the applied Si NMs, they can only utilize the silicic acid formed by the transformation of Si NMs.18 Meanwhile the formation of silicic acid is related to the concentration of three chemical groups on the surface of Si NMs directly depending on their molecular type: i) protonated (SiOH2+), ii) neutral (SiOH) and iii) deprotonated silanols (SiOH2+).30 The nano-SiO2 surface group is mainly composed of an oxidized layer of siloxanes (Si–O–Si) and silanol (Si–OH), while nano-Si involves silane (Si–H), silanol (Si–OH), and an oxidized layer of siloxanes (Si–O–Si).30 We speculated that silanols are the most abundant species in nano-SiO2 compared to nano-Si, thus more silicic acid is formed from the hydrolysis of SiO2. In addition, the solubility of Si NMs is also constrained by their applied dose. It has been reported that the degradation rate of Si NMs decreases with increasing concentration due to the existence of a dissolution threshold.62 Unfortunately, since the data of the modification (e.g., surface charge and coating) of Si NMs on the defence-related gene expression involved in this meta-analysis are very limited, we were not able to carry out the corresponding moderator analysis for the modification of Si NMs. Due to the existence of many polysaccharide containing galacturonic acid residues in plant cell walls, the plant cell wall is negatively charged.63 Therefore, positively charged NMs are more likely to be absorbed and captured by the cell wall due to electrostatic attraction, while negatively charged NMs are more likely to be transported due to electrostatic repulsion.63,64 Besides, the expression of plant defence-related genes also varied according to the time-length of the application, suggesting that the exposure time of Si NMs could directly affect defence-related gene expression. For instance, defence-related genes may have been activated during early stages of stress, while stress mitigation with prolonged application of Si NMs could result in a decreased gene expression.29,60 Due to spatiotemporal differences in gene expression and interaction effects, we here call for additional research that combines large-scale transcriptomics with time-implicit sampling of stressed plants.
5. Conclusions and perspectives
Using quantitative meta-analysis, we highlighted that Si NM addition can mitigate oxidative stress in plants through the activation of several defence-related genes, antioxidant enzymes, and metabolites. These effects however varied depending on several factors. Particularly, we found that: i) the Si NM-mediated effects on oxidative stress and antioxidant enzyme activity in plants were most driven by their application dose and duration; ii) the Si NM-mediated effects on antioxidant metabolite content in plants were most driven by their application dose and exposure method; iii) the Si NM-mediated effects on the expression of defence-related genes in plants were most driven by their application exposure method.
Based on these observations, several future research directions could be explored: i) because we found “only” eight studies on biotic stress, including five studies on disease stress, two studies on parasitic weed stress and one study on herbivore stress, we urge to explore further the role of Si NMs in mitigating biotic stress. ii) We suggest performing additional studies to address the regulation of plant defence substances and growth-related metabolic synthesis simultaneously using transcriptomic analysis combined with metabolomic analysis. iii) At present, research focusing on the modification of Si NMs, especially Si NMs of different molecular types in the same experimental system is still in its infancy. Since the properties of nanomaterials directly affect their bioavailability and biological regulation effects on plant defence against stress,29,31 it indeed calls for more research in this area in the future. iv) Another concern for NMs is their toxicity for plants and animals, which is currently restricting their practical application in the field.56 Si NMs are one of the top five nanoparticles widely used across research fields, including the cosmetic industry as well as drug and disease diagnosis.65 The large number of NMs used will not only accumulate in the environment, but also enter the skin, digestive tract and vital organs through the food chain.66 Therefore, in order to promote sustainable development of Si NMs, it is needed to evaluate the toxicity and environmental risks of Si NMs and their related products comprehensively and accurately.
Conflicts of interest
The authors declared no conflict of interest.
Acknowledgements
This work is supported by the National Natural Science Foundation of China (42177210, 41820104009). We would like to thank all the researchers whose data contributed to this meta-analysis.
References
- S. S. Porter, R. Bantay, C. A. Friel, A. Garoutte, K. Gdanetz, K. Ibarreta, B. M. Moore, P. Shetty, E. Siler and M. L. Friesen, Beneficial microbes ameliorate abiotic and biotic sources of stress on plants, Funct. Ecol., 2020, 34, 2075–2086 CrossRef.
- M. Sarraf, K. Vishwakarma, V. Kumar, N. Arif, S. Das, R. Johnson, E. Janeeshma, J. T. Puthur, S. Aliniaeifard, D. K. Chauhan, M. Fujita and M. Hasanuzzaman, Metal/metalloid-based nanomaterials for plant abiotic stress tolerance: An overview of the mechanisms, Plants, 2022, 11, 316 CrossRef CAS PubMed.
- W. Zhang, Global pesticide use: Profile, trend, cost/benefit and more, Proc. Int. Acad. Ecol. Environ. Sci., 2018, 8, 1–27 Search PubMed.
- M. Eisenstein, Natural solutions for agricultural productivity, Nature, 2020, 588, S58 CrossRef CAS PubMed.
- F. H. M. Tang, M. Lenzen, A. McBratney and F. Maggi, Risk of pesticide pollution at the global scale, Nat. Geosci., 2021, 14, 206–210 CrossRef CAS.
- M. A. Beketov, B. J. Kefford, R. B. Schäfer and M. Liess, Pesticides reduce regional biodiversity of stream invertebrates, Proc. Natl. Acad. Sci. U. S. A., 2013, 110, 11039–11043 CrossRef CAS PubMed.
- S. Knapp and M. G. A. van der Heijden, A global meta-analysis of yield stability in organic and conservation agriculture, Nat. Commun., 2018, 9, 3632 CrossRef PubMed.
- Y. Liu, Z. Xiao, F. Chen, L. Yue, H. Zou, J. Lyu and Z. Wang, Metallic oxide nanomaterials act as antioxidant nanozymes in higher plants: Trends, meta-analysis, and prospect, Sci. Total Environ., 2021, 780, 146578 CrossRef CAS PubMed.
- S. Namjoyan, A. Sorooshzadeh, A. Rajabi and M. Aghaalikhani, Nano-silicon protects sugar beet plants against water deficit stress by improving the antioxidant systems and compatible solutes, Acta Physiol. Plant., 2020, 42, 1–16 CrossRef.
- A. de Sousa, A. M. Saleh, T. H. Habeeb, Y. M. Hassan, R. Zrieq, M. A. M. Wadaan, W. N. Hozzein, S. Selim, M. Matos and H. AbdElgawad, Silicon dioxide nanoparticles ameliorate the phytotoxic hazards of aluminum in maize grown on acidic soil, Sci. Total Environ., 2019, 693, 133636 CrossRef CAS PubMed.
- S. Ali, M. Rizwan, A. Hussain, M. Zia Ur Rehman, B. Ali, B. Yousaf, L. Wijaya, M. N. Alyemeni and P. Ahmad, Silicon nanoparticles enhanced the growth and reduced the cadmium accumulation in grains of wheat (Triticum aestivum L.), Plant Physiol. Biochem., 2019, 140, 1–8 CrossRef CAS PubMed.
- D. Wang, N. B. Saleh, A. Byro, R. Zepp, E. Sahle-Demessie, T. P. Luxton, K. T. Ho, R. M. Burgess, M. Flury, J. C. White and C. Su, Nano-enabled pesticides for sustainable agriculture and global food security, Nat. Nanotechnol., 2022, 17, 347–360 CrossRef CAS PubMed.
- G. V. Lowry, A. Avellan and L. M. Gilbertson, Opportunities and challenges for nanotechnology in the agri-tech revolution, Nat. Nanotechnol., 2019, 14, 517–522 CrossRef CAS PubMed.
- P. Landa, R. Vankova, J. Andrlova, J. Hodek, P. Marsik, H. Storchova, J. C. White and T. Vanek, Nanoparticle-specific changes in Arabidopsis thaliana gene expression after exposure to ZnO, TiO2, and fullerene soot, J. Hazard. Mater., 2012, 241–242, 55–62 CrossRef CAS PubMed.
- H. Kang, W. Elmer, Y. Shen, N. Zuverza-Mena, C. Ma, P. Botella, J. C. White and C. L. Haynes, Silica nanoparticle dissolution rate controls the suppression of fusarium wilt of watermelon
(Citrullus lanatus), Environ. Sci. Technol., 2021, 55, 13513–13522 CrossRef CAS PubMed.
- Z. Y. Wang, W. Q. Zhu, F. R. Chen, L. Yue, Y. Ding, H. Xu, S. Rasmann and Z. G. Xiao, Nanosilicon enhances maize resistance against oriental armyworm (Mythimna separata) by activating the biosynthesis of chemical defenses, Sci. Total Environ., 2021, 778, 146378 CrossRef CAS PubMed.
- A. Rastogi, D. K. Tripathi, S. Yadav, D. K. Chauhan, M. Živčák, M. Ghorbanpour, N. I. El-Sheery and M. Brestic, Application of silicon nanoparticles in agriculture, 3 Biotech, 2019, 9, 90 CrossRef PubMed.
- Z. Souri, K. Khanna, N. Karimi and P. Ahmad, Silicon and plants: Current knowledge and future prospects, J. Plant Growth Regul., 2021, 40, 906–925 CrossRef CAS.
- S. N. Johnson, J. M. W. Ryalls, A. N. Gherlenda, A. Frew and S. E. Hartley, Benefits from below: Silicon supplementation maintains legume productivity under predicted climate change scenarios, Front. Plant Sci., 2018, 9, 202 CrossRef PubMed.
- S. N. Johnson, R. C. Rowe and C. R. Hall, Silicon is an inducible and effective herbivore defence against Helicoverpa punctigera (Lepidoptera: Noctuidae) in soybean, Bull. Entomol. Res., 2020, 110, 417–422 CrossRef CAS PubMed.
- H. S. Hajizadeh, M. Asadi, S. M. Zahedi, N. Hamzehpour, F. Rasouli, M. Helvacı and T. Alas, Silicon dioxide-nanoparticle nutrition mitigates salinity in gerbera by modulating ion accumulation and antioxidants, Folia Hortic., 2021, 33, 91–105 CrossRef.
- S. Farhangi-Abriz and S. Torabian, Nano-silicon alters antioxidant activities of soybean seedlings under salt toxicity, Protoplasma, 2018, 255, 953–962 CrossRef CAS PubMed.
- P. Rai-Kalal, R. S. Tomar and A. Jajoo, Seed nanopriming by silicon oxide improves drought stress alleviation potential in wheat plants, Funct. Plant Biol., 2021, 48, 905–915 CrossRef CAS PubMed.
- Z. S. Khan, M. Rizwan, M. Hafeez, S. Ali, M. Adrees, M. F. Qayyum, S. Khalid, M. Z. U. Rehman and M. A. Sarwar, Effects of silicon nanoparticles on growth and physiology of wheat in cadmium contaminated soil under different soil moisture levels, Environ. Sci. Pollut. Res., 2020, 27, 4958–4968 CrossRef CAS PubMed.
- M. M. Y. Madany, A. M. Saleh, T. H. Habeeb, W. N. Hozzein and H. AbdElgawad, Silicon dioxide nanoparticles alleviate the threats of broomrape infection in tomato by inducing cell wall fortification and modulating ROS homeostasis, Environ. Sci.: Nano, 2020, 7, 1415–1430 RSC.
- C. Soares, S. Branco-Neves, A. de Sousa, M. Azenha, A. Cunha, R. Pereira and F. Fidalgo, SiO2 nanomaterial as a tool to improve Hordeum vulgare L. tolerance to nano-NiO stress, Sci. Total Environ., 2018, 622–623, 517–525 CrossRef CAS PubMed.
- T. Kiany, L. Pishkar, N. Sartipnia, A. Iranbakhsh and G. Barzin, Effects of silicon and titanium dioxide nanoparticles on arsenic accumulation, phytochelatin metabolism, and antioxidant system by rice under arsenic toxicity, Environ. Sci. Pollut. Res., 2022, 24, 34725–34737 CrossRef PubMed.
- M. González-Moscoso, A. Juárez-Maldonado, G. Cadenas-Pliego, D. Meza-Figueroa, B. SenGupta and N. Martinez-Villegas, Silicon nanoparticles decrease arsenic translocation and mitigate phytotoxicity in tomato plants, Environ. Sci. Pollut. Res., 2022, 29, 34147–34163 CrossRef PubMed.
- J. T. Buchman, W. H. Elmer, C. Ma, K. M. Landy, J. C. White and C. L. Haynes, Chitosan-coated mesoporous silica nanoparticle treatment of Citrullus lanatus (watermelon): enhanced fungal disease suppression and modulated expression of stress-related genes, ACS Sustainable Chem. Eng., 2019, 7, 19649–19659 CrossRef CAS.
- J. G. Croissant, Y. Fatieiev and N. M. Khashab, Degradability and clearance of silicon, organosilica, silsesquioxane, silica mixed oxide, and mesoporous silica nanoparticles, Adv. Mater., 2017, 29, 1604634 CrossRef PubMed.
- X. Lu, D. Sun, X. Zhang, H. Hu, L. Kong, J. E. Rookes, J. Xie and D. M. Cahill, Stimulation of photosynthesis and enhancement of growth and yield in Arabidopsis thaliana treated with amine-functionalized mesoporous silica nanoparticles, Plant Physiol. Biochem., 2020, 156, 566–577 CrossRef CAS PubMed.
- H. Villagarcia, E. Dervishi, K. de Silva, A. S. Biris and M. V. Khodakovskaya, Surface chemistry of carbon nanotubes impacts the growth and expression of water channel protein in tomato plants, Small, 2012, 8, 2328–2334 CrossRef CAS PubMed.
- E. Spielman-Sun, A. Avellan, G. D. Bland, R. V. Tappero, A. S. Acerbo, J. M. Unrine, J. P. Giraldo and G. V. Lowry, Nanoparticle surface charge influences translocation and leaf distribution in vascular plants with contrasting anatomy, Environ. Sci.: Nano, 2019, 6, 2508–2519 RSC.
- J. Yang, W. Cao and Y. Rui, Interactions between nanoparticles and plants: phytotoxicity and defense mechanisms, J. Plant Interact., 2017, 12, 158–169 CrossRef CAS.
- L. V. Hedges, J. Gurevitch and P. S. Curtis, The meta-analysis of response ratios in experimental ecology, Ecology, 1999, 80, 1150–1156 CrossRef.
- P. U. S. Peiris, Y. Li, P. Brown and C. Xu, Efficacy of organic amendments to control Meloidogyne spp. in crops: a systematic review and meta-analysis, J. Soils Sediments, 2020, 20, 1584–1598 CrossRef.
-
R Development Core Team, R: A language and environment for statistical computing, Vienna, Austria, 2016, https://www.R-project.org Search PubMed.
- W. Viechtbauer, Conducting Meta-Analyses in R with the metafor Package, J. Stat. Softw., 2010, 36, 1–48 Search PubMed.
- Z. Xiao, S. Rasmann, L. Yue, F. Lian, H. Zou and Z. Wang, The effect of biochar amendment on N-cycling genes in soils: A meta-analysis, Sci. Total Environ., 2019, 696, 133984 CrossRef CAS PubMed.
-
J. A. C. Sterne and M. Egger, in Publication bias in meta-analysis, ed. A. J. S. Hannah, R. Rothstein and M. Borenstein, Wiley, West Sussex, 2005, pp. 99–110 Search PubMed.
-
M. D. Jennions, C. J. Lortie, M. S. Rosenberg and H. R. Rothstein, in Handbook of Meta-analysis in Ecology and Evolution, ed. J. Koricheva, J. Gurevitch and K. Mengersen, Princeton University Press, 2013, pp. 207–236 Search PubMed.
- M. S. Rosenberg, The file-drawer problem revisited: a general weighted method for calculating fail-safe numbers in meta-analysis, Evolution, 2005, 59, 464–468 CrossRef PubMed.
- M. Rizwan, S. Ali, M. Z. ur Rehman, S. Malik, M. Adrees, M. F. Qayyum, S. A. Alamri, M. N. Alyemeni and P. Ahmad, Effect of foliar applications of silicon and titanium dioxide nanoparticles on growth, oxidative stress, and cadmium accumulation by rice (Oryza sativa), Acta Physiol. Plant., 2019, 41, 1–12 CrossRef CAS.
- D. K. Tripathi, V. P. Singh, S. M. Prasad, D. K. Chauhan and N. K. Dubey, Silicon nanoparticles (SiNP) alleviate chromium (VI) phytotoxicity in Pisum sativum (L.) seedlings, Plant Physiol. Biochem., 2015, 96, 189–198 CrossRef CAS PubMed.
- Z. Xiao, N. Fan, L. Yue, F. Chen, H. Ji, Y. Shu, S. Rasmann and Z. Wang, Dose-dependent effects of CeO2 nanomaterials on tomato plant chemistry and insect herbivore resistance, Environ. Sci.: Nano, 2021, 8, 3577–3589 RSC.
- A. Hussain, M. Rizwan, Q. Ali and S. Ali, Seed priming with silicon nanoparticles improved the biomass and yield while reduced the oxidative stress and cadmium concentration in wheat grains, Environ. Sci. Pollut. Res., 2019, 26, 7579–7588 CrossRef CAS PubMed.
- E. M. Hafez, H. S. Osman, S. M. Gowayed, S. A. Okasha, A. E.-D. Omara, R. Sami, A. M. Abd El-Monem and U. A. Abd El-Razek, Minimizing the adversely impacts of water deficit and soil salinity on maize growth and productivity in response to the application of plant growth-promoting rhizobacteria and silica nanoparticles, Agronomy, 2021, 11, 676 CrossRef CAS.
- D. L. Slomberg and M. H. Schoenfisch, Silica nanoparticle phytotoxicity to Arabidopsis thaliana, Environ. Sci. Technol., 2012, 46, 10247–10254 CrossRef CAS PubMed.
- K. Ozturk, F. B. Arslan, E. Tavukcuoglu, G. Esendagli and S. Calis, Aggregation of chitosan nanoparticles in cell culture: Reasons and resolutions, Int. J. Pharm., 2020, 578, 119119 CrossRef CAS PubMed.
- N. Nafee, M. Schneider, U. F. Schaefer and C.-M. Lehr, Relevance of the colloidal stability of chitosan/PLGA nanoparticles on their cytotoxicity profile, Int. J. Pharm., 2009, 381, 130–139 CrossRef CAS PubMed.
- M. G. Mostofa, M. M. Rahman, M. M. U. Ansary, S. S. Keya, M. Abdelrahman, M. G. Miah and L.-S. P. Tran, Silicon in mitigation of abiotic stress-induced oxidative damage in plants, Crit. Rev. Biotechnol., 2021, 41, 918–934 CrossRef CAS PubMed.
- D. K. Tripathi, S. Singh, V. P. Singh, S. M. Prasad, D. K. Chauhan and N. K. Dubey, Silicon nanoparticles more efficiently alleviate arsenate toxicity than silicon in maize cultiver and hybrid differing in arsenate tolerance, Front. Environ. Sci., 2016, 4, 46 Search PubMed.
- Z. H. Pinedo-Guerrero, G. Cadenas-Pliego, H. Ortega-Ortiz, S. González-Morales, A. Benavides-Mendoza, J. Valdés-Reyna and A. Juárez-Maldonado, Form of silica improves yield, fruit quality and antioxidant defense system of tomato plants under salt stress, Agriculture, 2020, 10, 367 CrossRef CAS.
-
S. Kataria, in UV-B radiation, ed. V. P. Singh, S. Singh, S. M. Prasad and P. Parihar, Wiley, West Sussex, 2017, pp. 99–121 Search PubMed.
- A. do Espirito Santo Pereira, H. Caixeta Oliveira, L. Fernandes Fraceto and C. Santaella, Nanotechnology potential in seed priming for sustainable agriculture, Nanomaterials, 2021, 11, 267 CrossRef CAS PubMed.
- J. A. Bhat, N. Rajora, G. Raturi, S. Sharma, P. Dhiman, S. Sanand, S. M. Shivaraj, H. Sonah and R. Deshmukh, Silicon nanoparticles (SiNPs) in sustainable agriculture: major emphasis on the practicality, efficacy and concerns, Nanoscale Adv., 2021, 3, 4019–4028 RSC.
- R. S. El-Serafy, A. A. El-Sheshtawy, A. K. G. Atteya, A. Al-Hashimi, A. M. Abbasi and I. Al-Ashkar, Seed priming with silicon as a potential to increase salt stress tolerance in Lathyrus odoratus, Plants, 2021, 10, 2140 CrossRef CAS PubMed.
- A. Minoo, P. Alireza and S. Omid, Foliar-applied silicon and its nanoparticles stimulates physio-chemical changes to improve growth, yield and active constituents of coriander (Coriandrum sativum L.) essential oil under different irrigation regimes, Silicon, 2021, 13, 4177–4188 CrossRef.
- W. Islam, M. Tayyab, F. Khalil, Z. Hua, Z. Huang and H. Y. H. Chen, Silicon-mediated plant defense against pathogens and insect pests, Pestic. Biochem. Physiol., 2020, 168, 104641 CrossRef CAS PubMed.
- M. Moghanloo, A. Iranbakhsh, M. Ebadi and Z. O. Ardebili, Differential physiology and expression of phenylalanine ammonia lyase (PAL) and universal stress protein (USP) in the endangered species Astragalus fridae following seed priming with cold plasma and manipulation of culture medium with silica nanoparticles, 3 Biotech, 2019, 9, 1–13 CrossRef PubMed.
- R. Suriyaprabha, G. Karunakaran, R. Yuvakkumar, V. Rajendran and N. Kannan, Foliar application of silica nanoparticles on the phytochemical responses of maize (Zea mays L.) and its toxicological behavior, Synth. React. Inorg., Met.-Org., Nano-Met. Chem., 2014, 44, 1128–1131 CrossRef CAS.
- K. S. Finnie, D. J. Waller, F. L. Perret, A. M. Krause-Heuer, H. Q. Lin, J. V. Hanna and C. J. Barbé, Biodegradability of sol–gel silica microparticles for drug delivery, J. Sol-Gel Sci. Technol., 2009, 49, 12–18 CrossRef CAS.
- E. Spielman-Sun, E. Lombi, E. Donner, D. Howard, J. M. Unrine and G. V. Lowry, Impact of surface charge on cerium oxide nanoparticle uptake and translocation by wheat (Triticum aestivum), Environ. Sci. Technol., 2017, 51, 7361–7368 CrossRef CAS PubMed.
- C. Wang, X. Liu, F. Chen, L. Yue, X. Cao, J. Li, B. Cheng, Z. Wang and B. Xing, Selenium content and nutritional quality of Brassica chinensis L enhanced by selenium engineered nanomaterials: The role of surface charge, Environ. Pollut., 2022, 308, 119582 CrossRef CAS PubMed.
- B. Zhu, W. He, S. Hu, R. Kong and L. Yang, The fate and oxidative stress of different sized SiO2 nanoparticles in zebrafish (Danio rerio) larvae, Chemosphere, 2019, 225, 705–712 CrossRef CAS PubMed.
- A. Ale, M. F. Gutierrez, A. S. Rossi, C. Bacchetta, M. F. Desimone and J. Cazenave, Ecotoxicity of silica nanoparticles in aquatic organisms: An updated review, Environ. Toxicol. Pharmacol., 2021, 87, 103689 CrossRef CAS PubMed.
|
This journal is © The Royal Society of Chemistry 2022 |
Click here to see how this site uses Cookies. View our privacy policy here.