DOI:
10.1039/D0SC01538E
(Minireview)
Chem. Sci., 2020,
11, 5630-5636
Recent advances in N-heterocyclic carbene-based radical catalysis
Received
16th March 2020
, Accepted 10th May 2020
First published on 11th May 2020
Abstract
In nature, a number of enzymes use thiamine diphosphate as a coenzyme to catalyze the pyruvate decarboxylation. The resultant enamine, a so-called “Breslow intermediate,” is known to perform single electron transfer to various electron acceptors. Inspired by this enzymatic catalysis, N-heterocyclic carbene (NHC)-catalyzed radical reactions have been developed. This minireview highlights the recent progress and developments in NHC-based radical catalysis. This minireview is categorized according to the reaction types; oxidation type reaction and carbon–carbon bond formation through single electron transfer/radical–radical coupling.
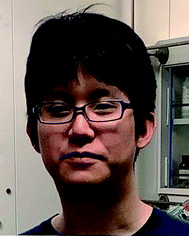 Takuya Ishii | Takuya Ishii received his MSc degree in 2020 from Kanazawa University under the supervision of Professor Hirohisa Ohmiya. In 2020, he started his doctoral studies as a JSPS Research Fellowship for Young Scientists (DC1) in the group of Prof. H. Ohmiya, working in the field of NHC-based radical catalysis. |
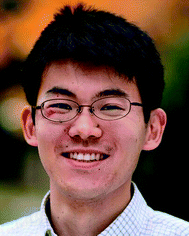 Kazunori Nagao | Kazunori Nagao is an Assistant Professor of Kanazawa University. He received his PhD degree from Hokkaido University in 2016 under the supervision of Professor Masaya Sawamura. Then, he spent two years as a postdoctoral researcher (JSPS overseas fellowship) at Princeton University (Professor David W. C. MacMillan, 2016–2018). After he served as a postdoctoral researcher at Kanazawa University (Professor Hirohisa Ohmiya, 2018–2019), he became an Assistant Professor of the same group in April 2019. Now he focuses on the development of new catalysis based on selective radical–radical coupling. |
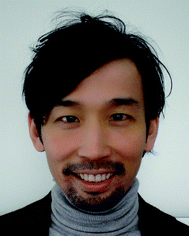 Hirohisa Ohmiya | Hirohisa Ohmiya is a Professor at Kanazawa University. He received his PhD degree from Kyoto University in 2007 under the supervision of Professor Koichiro Oshima. He spent one year as a JSPS postdoctoral fellow in the group of Professor Timothy F. Jamison at Massachusetts Institute of Technology. In 2008, he became an Assistant Professor at Hokkaido University working with Professor Masaya Sawamura. He was promoted to Associate Professor in 2010. Since 2017, he has been a Professor at Kanazawa University. He is acting as JST PRESTO Researcher (2019–2023). His research focuses on development of organic synthesis for new catalysis, reactions and molecules. |
1. Introduction
N-heterocyclic carbene (NHC) catalysis, which exhibits a characteristic property to harness umpolung reactivity, has received considerable attention as a powerful tool for organic synthetic reactions. NHC catalysis through a two-electron reaction pathway has been extensively studied.1 The two-electron reaction process can access umpolung reactivity of carbonyls as acyl anions, enolates and homoenolates. On the other hand, NHC-catalyzed radical reactions through a one-electron reaction pathway are also known although the process remains challenging and much less developed. In biological systems, there are a number of enzymes utilizing thiamine diphosphate (ThDP, vitamin B1 derivative) as a coenzyme to catalyze the oxidative decarboxylation of pyruvate (Scheme 1).2 The resultant enamine, a so-called “Breslow intermediate”,3 performs single electron transfer to various electron acceptors such as lipoamides, flavin adenine dinucleotide and Fe4S4.4 The NHC-catalyzed radical reactions in the single-electron transfer (SET) manifold have been introduced in 2001.5 The crystal structure of the free radical intermediate of pyruvate ferredoxin oxidoreductase was disclosed. The redox and electron transfer properties of Breslow intermediates have been also studied by Fukuzumi and co-worker in late 1990s.6 They noted that a series of enolate form of Breslow intermediate derived from a thiamine analogue and aldehyde in the presence of excess amount of base, which has been alternatively called as active aldehyde, has extremely low oxidation potential (Eox = −0.97 to −0.78 V vs. SCE) and small reorganization energy (λ = 12.0 to 12.9 kcal mol−1) (Scheme 2). These features make it a strong reducing agent. Additionally, the resultant Breslow intermediate-derived radical obtained by single electron oxidation was a persistent one.
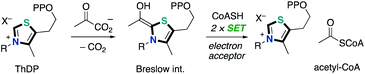 |
| Scheme 1 Enzymatic transformation of pyruvate. | |
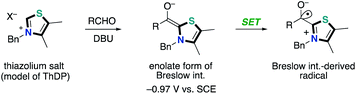 |
| Scheme 2 Redox properties of enolate form of Breslow intermediate. | |
Inspired by the enzymatic catalysis and these early studies, NHC-catalyzed radical reactions in organic synthesis have been developed to date. Due to the nature of highly reactive radicals, these reactions enabled the introduction of sterically bulky substituents, which used to be difficult in the well-known NHC catalysis involving a two-electron reaction pathway. This minireview highlights the recent progress and developments in the NHC-based radical catalysis. This minireview is categorized according to the reaction types; oxidation type reaction (Chapter 2) and carbon–carbon bond formation through SET/radical–radical coupling (Chapter 3).
2. Oxidation type reaction
In 2008, Studer and co-workers reported the pioneering example of the synthetic reaction through NHC-based radical catalysis (Scheme 3).7a The protocol enabled the oxidation of aldehydes to esters. This process involves two continuous single electron oxidations of Breslow intermediate by TEMPO to form the corresponding azolium ketone. In 2010, the Studer group used 3,3′,5,5′-tetra-tert-butyldiphenoquinone as the stoichiometric oxidant instead of TEMPO.7b–d In 2014, Chi and co-workers reported the NHC-based radical catalysis for reductive homo-coupling reaction of nitroethylenes (Scheme 4).8 The reaction goes through nitroalkene-derived radical anions under SET process. In this process, the Breslow intermediate did not participate in the bond formation, which just acted as an electron donor.
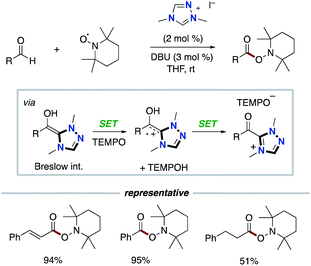 |
| Scheme 3 NHC-catalyzed oxidation of aldehydes to esters. | |
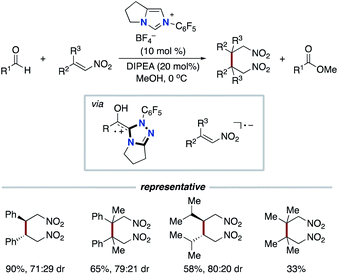 |
| Scheme 4 NHC-catalyzed reductive homo-coupling of nitroethylenes. | |
The Rovis group9 in 2014 and the Chi group10 in 2015 independently reported asymmetric β-hydroxylation reactions of enals using chiral triazolium NHC catalyst (Scheme 5). This process involves the SET event between Breslow intermediate and nitrobenzene or the derivative followed by radical recombination between the resultant two radicals, a homoenolate-centered radical and an oxygen-centered radical. Based on this system, several radical β-functionalizations using enals have been developed.11 In 2015, the Rovis group reported the enantioselective synthesis of 3,4-disubstituted cyclopentanones through dimerization of enals.12 In 2017, the Ye group reported oxidative [3 + 2] annulation of dioxindoles and enals.13
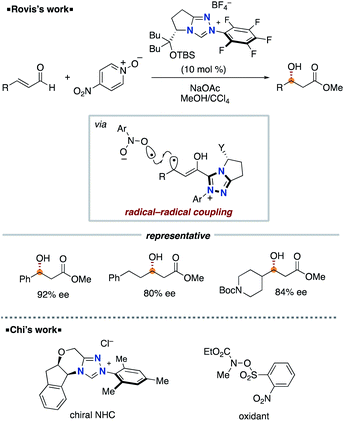 |
| Scheme 5 NHC-catalyzed enantioselective β-hydroxylatioin of enals. | |
In addition to the use of TEMPO or nitroarenes as the SET oxidant shown above, the Sun group and the Chi group independently enabled the use of polyhalides as oxidants for the SET process. In 2016, the Sun group reported dihalomethylenation of enals. The process involves the radical addition between carbon-centered trihalomethyl radical and dienolate intermediate derived from enal and triazolium type NHC catalyst to form carbon–carbon bond formation (Scheme 6).14 In 2017, the Chi group used polyhalides such as CCl4 and C2Cl6 as oxidants in the NHC-catalyzed functionalization of aldehydes or enals.15 The dienolate intermediate undergoes two SET processes with polyhalides to produce the corresponding acyl triazolium intermediate.
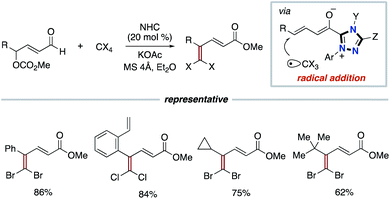 |
| Scheme 6 γ-Dihalomethylenation of enals by NHC and polyhalomethanes. | |
In 2019, the Ye group reported the synergistic combination of NHC catalysis with Ru photoredox catalysis for the γ- and ε-alkylation of enals with alkyl halides bearing an electron-withdrawing group such as cyano or ester to produce γ-multisubstituted-α,β-unsaturated esters (Scheme 7).16 The radical addition of alkyl radical, which is generated from alkyl halide by Ru photocatalysis, to dienolate intermediate derived from enal by NHC catalysis produces homoenolate radical. The subsequent SET event between the homoenolate radical and a radical cation form of Ru photocatalyst affords an acyl azolium intermediate.
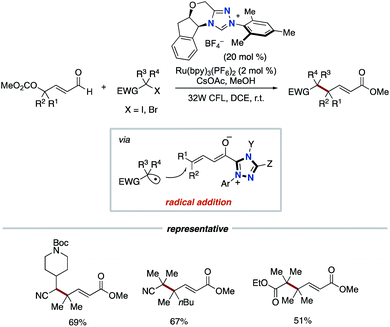 |
| Scheme 7 γ-Alkylation of enals with alkyl halides through synergistic Ru photoredox/NHC catalysis. | |
In 2016, the Chi group reported the reductive coupling of nitrobenzyl bromides and activated ketones or imines using aldehydes as a formal reductant (Scheme 8).17 The reaction involves the generation of nitrobenzyl radical intermediates from nitrobenzyl bromides followed by formal 1,2-addition. The authors did not rule out the reaction pathway involving nitrobenzyl anion generated from additional single electron reduction of the nitrobenzyl radical.
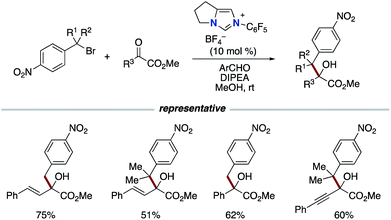 |
| Scheme 8 Reductive carbonyl coupling of nitrobenzyl bromides. | |
3. Carbon–carbon bond formation through SET/radical–radical coupling
The NHC-catalyzed radical reactions described in Chapter 2 afforded the formal oxidized product through single electron oxidations of Breslow intermediate. On the other hand, the direct radical–radical coupling of the persistent Breslow intermediate-derived radical with carbon-centered radical species has not been disclosed until quite recently.
In 2015, the Rehbein group re-evaluated the mechanism of NHC-catalyzed intermolecular Benzoin condensation (Scheme 9).18 Thus, radical pairs that is derived from Breslow-intermediate through SET process was observed and characterized. In 2019, Bertrand and Martin elucidated the characteristics of Breslow intermediate-derived radical species by EPR spectra, DFT calculation and CV spectrometry (Scheme 10).19 Then, they implied the intermediacy of captodatively stabilized radicals in NHC-catalyzed oxidative functionalization of aldehydes or enals using mild oxidants. These reports by Rehbein and Bertrand hinted that Breslow intermediate-derived radical could participate in radical–radical coupling for the bond forming reaction.
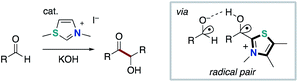 |
| Scheme 9 Re-evaluation of the mechanism of NHC-catalyzed Benzoin condensation. | |
 |
| Scheme 10 Radical intermediates in oxidative NHC catalysis. | |
In 2019, our group discovered that the persistent Breslow intermediate-derived radical couples with transient alkyl radical in the carbon–carbon bond forming process (Scheme 11).20,21 This unprecedented radical–radical coupling is based on kinetic phenomenon, persistent radical effect (PRE).22 This achievement could definitely expand the scope of the NHC-based radical catalysis. Specifically, thiazolium NHC catalysis promoted the unprecedented decarboxylative coupling of aryl aldehydes and tertiary or secondary alkyl carboxylic acid-derived redox-active esters to produce aryl alkyl ketones. The redox ester is a source of alkyl radical specie. In this report, Breslow intermediate is presumed to have necessity of deprotonation by Cs2CO3 base so that to reach enough reduction potential for SET with redox ester.6 Thus, an enolate form of Breslow intermediate performs SET to a redox ester, and the obtained Breslow intermediate-derived radical couples with an alkyl radical. The protocol has wide substrate scope and enabled the functionalization of pharmaceutical drugs and natural products.
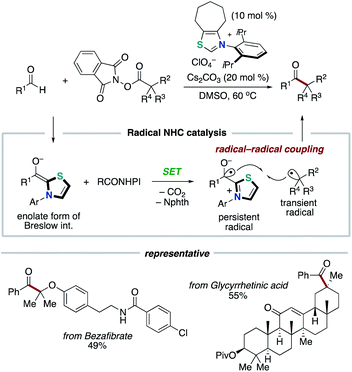 |
| Scheme 11 NHC-catalyzed decarboxylative alkylation of aldehydes. | |
Our group applied the NHC-based radical catalysis into three-component coupling using aldehydes, alkenes and tertiary alkyl carboxylic acid-derived redox-active esters (Scheme 12).23 The introduction of tertiary alkyl group and acyl group to carbon–carbon double bonds occurred with complete regioselectivity. The radical relay process involves SET from the enolate form of Breslow intermediate to a redox active ester and radical addition of the resultant alkyl radical to an alkene followed by radical–radical coupling. The key design of this radical relay process is the proper match on reaction rates of several competing radical reactions. Recently, this protocol was applied to the synthesis of δ-ketocarbonyls using tertiary α-bromocarbonyls instead of redox active esters (Scheme 13).24
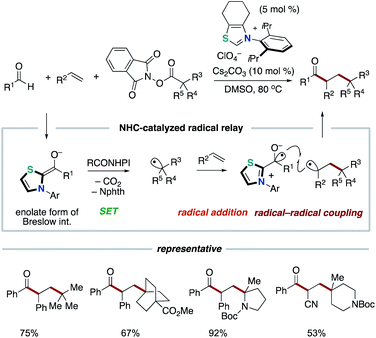 |
| Scheme 12 Acylalkylation of alkenes through NHC-catalyzed radical relay. | |
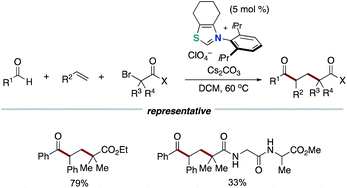 |
| Scheme 13 Synthesis of δ-ketocarbonyls through NHC-catalyzed radical relay. | |
In 2020, the Hong group enabled the use of redox active Katritzky pyridinium salts instead of redox active esters in the alkylation of aldehyde and the three-component alkylacylation of alkene (Scheme 14).25 Katritzky salts can be directly reduced by the enolate form of Breslow intermediate to generate a ketyl radical and an alkyl radical and then radical–radical coupling with two radical species proceeds to yield a ketone.
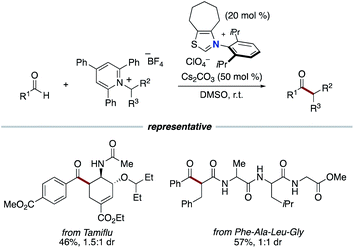 |
| Scheme 14 NHC-catalyzed deaminative alkylation of aldehydes. | |
In 2019 and 2020, the Li group,26 the Wang group,27 and the Yang and Wu group28 reported the acylfluoroalkylation of alkenes based on our NHC-catalyzed three-component coupling reaction, respectively (Schemes 15 and 16). Togni I reagent or perfluoroalkylbromide instead of redox ester is used as an electron acceptor in the step of SET.
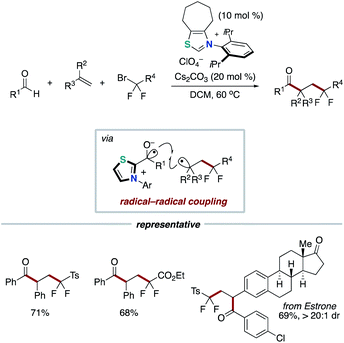 |
| Scheme 15 NHC-catalyzed acylfluoroalkylation of alkenes. | |
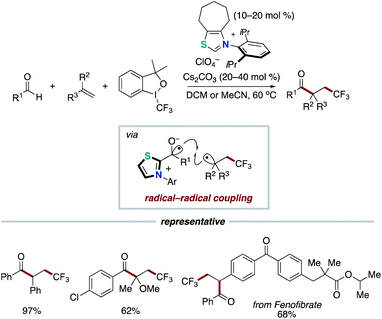 |
| Scheme 16 NHC-catalyzed acyltrifluorometylation of alkenes. | |
In 2020, the Scheidt group developed the synergistic merger of NHC-based radical catalysis and Ir photoredox catalysis for benzylation of acyl azoliums, which are prepared from carboxylic acids, to form ketones (Scheme 17).29 In this case, benzyl Hantzsch esters and acyl imidazoliums were used as precursor of alkyl radical and acyl azolium, respectively. The combination of NHC and Ir photoredox catalysis enabled a SET with the acyl azolium, and the subsequent radical–radical coupling with an alkyl radical allowed for the construction of a carbon–carbon bond to furnish a ketone.
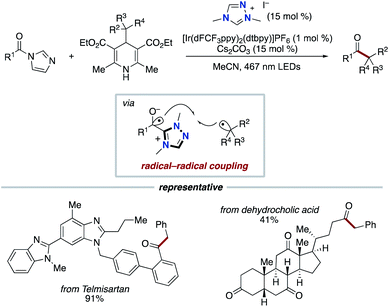 |
| Scheme 17 Conversion of carboxylic acids to ketones through synergistic Ir photoredox/NHC catalysis. | |
In 2020, the Hopkinson group reported a different type of NHC-based radical catalysis. The synergistic cooperation of NHC catalysis and light activation enables the annulation using o-toluoylfluorides and trifluoroacetophenones (Scheme 18).30 The mechanistic studies and time-dependent DFT calculations suggested that the direct excitation of acyl azolium, which is derived from acyl fluoride and NHC, by UV irradiation could create biradical-ketone-like photochemical reactivity. The triplet excited state is applied to the photoenolization and the subsequent Diels–Alder process to produce isochroman-1-one derivatives. This process does not involve the radical–radical coupling.
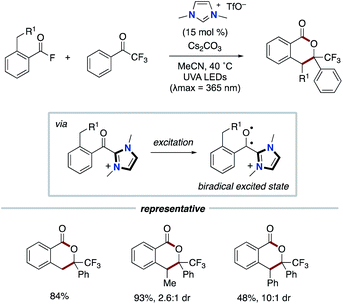 |
| Scheme 18 Photoenolization/Diels–Alder reaction of acid fluorides. | |
4. Conclusions
In this minireview, we summarized the NHC-catalyzed radical reaction with two categories; oxidation type reaction (Chapter 2) and carbon–carbon bond formation through SET/radical–radical coupling (Chapter 3). In particular, the finding that the persistent Breslow intermediate-derived radical couples with transient alkyl radical in the carbon–carbon bond forming process described in Chapter 3, opened the door to a new design guideline for NHC organocatalysis. Although these NHC-catalyzed radical reactions enabled the solution of various problems in organic synthesis, significant future contributions will be needed in terms of the development of stereoselective and universal bond-formation reactions, the application to biomolecules and the mechanistic studies by intermediate analysis and theoretical calculations.
Conflicts of interest
There are no conflicts to declare.
Acknowledgements
This work was supported by JSPS KAKENHI Grant Number JP18H01971 to Scientific Research (B), JSPS KAKENHI Grant Number JP17H06449 (Hybrid Catalysis) and JST, PRESTO Grant Number JPMJPR19T2 (to H. O.).
Notes and references
-
(a) D. Enders, O. Niemeier and A. Henseler, Chem. Rev., 2008, 107, 5606–5655 CrossRef PubMed
;
(b) D. M. Flanigan, F. Romanov-Michailidis, N. A. White and T. Rovis, Chem. Rev., 2015, 115, 9307 CrossRef CAS PubMed
;
(c) M. N. Hopkinson, C. Richter, M. Schedler and F. Glorius, Nature, 2014, 510, 485 CrossRef CAS
;
(d) X. Bugaut and F. Glorius, Chem. Soc. Rev., 2012, 41, 3511–3522 RSC
;
(e) R. S. Menon, A. T. Biju and V. Nair, Chem. Soc. Rev., 2015, 44, 5040–5052 RSC
.
-
(a) S. W. Ragsdale, Chem. Rev., 2003, 103, 2333 CrossRef CAS PubMed
;
(b) R. Kluger and K. Tittmann, Chem. Rev., 2008, 108, 1797 CrossRef CAS PubMed
;
(c) S. O. Mansoorabadi, J. Seravalli, C. Furdui, V. Krymov, G. J. Gerfen, T. P. Begley, J. Melnick, S. W. Ragsdale and G. H. Reed, Biochemistry, 2006, 45, 7122 CrossRef CAS
.
- R. Breslow, J. Am. Chem. Soc., 1958, 80, 3719 CrossRef CAS
.
- C. C. Chiu, K. Pan and F. Jordan, J. Am. Chem. Soc., 1995, 117, 7027 CrossRef CAS
.
- E. Chabriere, X. Vernede, B. Guigliarelli, M.-H. Charon, E. C. Hatchikian and J. C. Fontecilla-Camps, Science, 2001, 294, 2559 CrossRef CAS PubMed
.
-
(a) I. Nakanishi, S. Itoh, T. Suenobu, H. Inoue and S. Fukuzumi, Chem. Lett., 1997, 8, 707 CrossRef
;
(b) I. Nakanishi, S. Itoh, T. Suenobu and S. Fukuzumi, Chem. Commun., 1997, 1927 RSC
;
(c) I. Nakanishi, S. Itoh, T. Suenobu and S. Fukuzumi, Angew. Chem., Int. Ed., 1998, 37, 992 CrossRef CAS
;
(d) I. Nakanishi, S. Itoh and T. Fukuzumi, Chem.–Eur. J., 1999, 5, 2810 CrossRef CAS
.
-
(a) J. Guin, S. D. Sarkar, S. Grimme and A. Studer, Angew. Chem., Int. Ed., 2008, 47, 8727 CrossRef CAS PubMed
;
(b) S. D. Sarkar, S. Grimme and A. Studer, J. Am. Chem. Soc., 20150, 132, 1190 CrossRef PubMed
;
(c) D. Sarkar and A. Studer, Org. Lett., 2010, 12, 1992 CrossRef PubMed
;
(d) D. Sarkar and A. Studer, Angew. Chem., Int. Ed., 2010, 497, 9266 CrossRef PubMed
.
- Y. Du, Y. Wang, X. Li, Y. Shao, G. Li, R. D. Webster and Y. R. Chi, Org. Lett., 2014, 16, 5678 CrossRef CAS PubMed
.
- N. A. White and T. Rovis, J. Am. Chem. Soc., 2014, 136, 14674 CrossRef CAS PubMed
.
-
(a) Y. Zhang, Y. Du, Z. Huang, J. Xu, X. Wu, Y. Wang, M. Wang, S. Yang, R. D. Webster and Y. R. Chi, J. Am. Chem. Soc., 2015, 137, 2416 CrossRef CAS PubMed
;
(b) H. Wang, Y. Wang, X. Chen, C. Mou, S. Yu, H. Chai, Z. Jin and Y. R. Chi, Org. Lett., 2019, 21, 7440 CrossRef CAS PubMed
.
- K. Zhao and D. Enders, Angew. Chem., Int. Ed., 2017, 56, 3754 CrossRef CAS PubMed
.
- N. A. White and T. Rovis, J. Am. Chem. Soc., 2015, 137, 10112 CrossRef CAS PubMed
.
- X.-Y. Chen, K.-Q. Chen, D.-Q. Sun and S. Ye, Chem. Sci., 2017, 8, 1936 RSC
.
- W. Yang, W. Hu, X. Dong, X. Li and J. Sun, Angew. Chem., Int. Ed., 2016, 55, 15783 CrossRef CAS PubMed
.
- X. Wu, Y. Zhang, Y. Wang, J. Ke, M. Jeret, R. N. Reddi, S. Yang, B.-A. Song and Y. R. Chi, Angew. Chem., Int. Ed., 2017, 56, 2942 CrossRef CAS PubMed
.
- L. Dai, Z.-H. Xia, Y.-Y. Gao, Z.-H. Gao and S. Ye, Angew. Chem., Int. Ed., 2019, 58, 18124 CrossRef CAS PubMed
.
- B.-S. Li, Y. Wang, R. S. J. Proctor, Y. Zhang, R. D. Webster, S. Yang, B. Song and Y. R. Chi, Nat. Commun., 2016, 7, 12933 CrossRef PubMed
.
- J. Rehbein, S.-M. Ruser and J. Phan, Chem. Sci., 2015, 6, 6013 RSC
.
- V. Regnier, E. A. Romero, F. Molton, R. Jazzar, G. Bertrand and D. Martin, J. Am. Chem. Soc., 2019, 141, 1109 CrossRef CAS PubMed
.
- T. Ishii, Y. Kakeno, K. Nagao and H. Ohmiya, J. Am. Chem. Soc., 2019, 141, 3854 CrossRef CAS PubMed
.
- R. Song and Y. Chi, Angew. Chem., Int. Ed., 2019, 58, 8628 CrossRef CAS PubMed
.
- D. Leifert and A. Studer, Angew. Chem., Int. Ed., 2020, 59, 74 CrossRef CAS PubMed
.
- T. Ishii, K. Ota, K. Nagao and H. Ohmiya, J. Am. Chem. Soc., 2019, 141, 14073 CrossRef CAS PubMed
.
- K. Ota, K. Nagao and H. Ohmiya, Org. Lett., 2020, 22 DOI:10.1021/acs.orglett.0c01199
.
- I. Kim, H. Im, H. Lee and S. Hong, Chem. Sci., 2020, 11, 3192–3197 RSC
.
- J.-L. Li, Y.-Q. Liu, W.-L. Zou, R. Zeng, X. Zhang, Y. Liu, B. Han, Y. He, H.-J. Leng and Q.-Z. Li, Angew. Chem., Int. Ed., 2020, 59, 1863 CrossRef CAS PubMed
.
- B. Zhang, Q. Peng, D. Guo and J. Wang, Org. Lett., 2020, 22, 443 CrossRef CAS PubMed
.
- H.-B. Yang, Z.-H. Wang, J.-M. Li and C. Wu, Chem. Commun., 2020, 56, 3801–3804 RSC
.
- A. Davies, K. Fitzpatrick, R. Betori and K. Scheidt, Angew. Chem., Int. Ed., 2020 DOI:10.1002/anie.202001824
.
- A. Mavroskoufis, K. Rajes, P. Golz, A. Agrawal, V. Ruß, J. P. Götze and M. N. Hopkinson, Angew. Chem., Int. Ed., 2020, 59, 3190 CrossRef CAS PubMed
.
|
This journal is © The Royal Society of Chemistry 2020 |
Click here to see how this site uses Cookies. View our privacy policy here.