Scope of surface-modified molecular and nanomaterials in gel/liquid forms for developing mechanically flexible DSSCs/QDSSCs
Received
9th July 2018
, Accepted 21st October 2018
First published on 22nd October 2018
Abstract
The advanced lifestyle of the human race involves heavy usage of various gadgets which require copious supplies of energy for uninterrupted functioning. Due to the ongoing depletion of fossil fuels and the accelerating demand for other energy resources, renewable energy sources, especially solar cells, are being extensively explored as viable alternatives. Flexible solar cells have recently emerged as an advanced member of the photovoltaic family; the flexibility and pliability of these photovoltaic materials are advantageous from a practical point of view. Conventional flexible solar cell materials, when dispersed in solvents, are usually volatile and create severe stability issues when incorporated in devices. Recently, non-volatile, less viscous functional molecular liquids/gels have been proposed as potential materials for use in foldable device applications. This perspective article discusses the scope of surface-modified non-volatile molecular and nanomaterials in liquid/gel forms in the manufacturing and deployment of flexible photovoltaics.
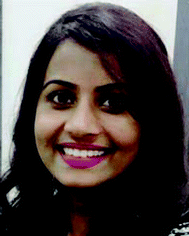 Soorya Sasi | Miss Soorya Sasi is a Research Scholar working towards her Ph.D. in the area of functional molecular liquids and composites for energy applications at the Advanced Molecular Materials Research Centre (AMMRC), Mahatma Gandhi University (MGU), Kottayam, Kerala, India. She received her M.Tech. in nanotechnology from Amrita Vishwa Vidya Peetham, India and worked as a Senior Research Fellow at the Amrita Center for Nanosciences and Molecular Medicine, Kochi, Kerala, India. Her areas of interest are flexible nanomaterial composites, dye/quantum dot-sensitized solar cells, supercapacitors and flow capacitors. |
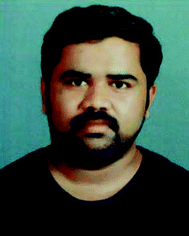 Sunish K. Sugunan | Dr Sunish K. Sugunan received his Ph.D. in Chemistry from the University of Saskatchewan, Canada and completed a subsequent two-year Post-doctoral term at Durham University, UK. Later, he worked as a research officer at Advanced Molecular Materials Research Centre (AMMRC), Mahatma Gandhi University (MGU), Kottayam, Kerala, India. Currently, he is working as an Assistant Professor at CMS College – Kottayam, Kerala, India. His areas of research are experimental and theoretical studies of materials for solar cells, flexible nanomaterials and composites, OLEDs, photosynthetic complexes and photocatalysis. |
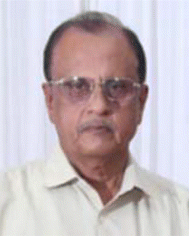 P. Radhakrishnan Nair | Dr P. Radhakrishnan Nair obtained his Ph. D in Polymer Chemistry from Cochin University of Science and Technology, Cochin, India. He has 38 years of R & D experience in the synthesis, characterization and scaleup of polymers as binders for solid propellants at Vikram Sarabhai Space Centre (VSSC) and the Indian Space Research Organization (ISRO); he has developed different binders which were successfully used in ISRO's missions. Presently, he is a Visiting Professor at the Advanced Molecular Material Research Centre (AMMRC), Mahatma Gandhi University, Kottayam, India and is engaged in research of high energy materials. |
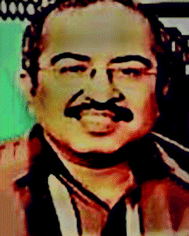 K. R. V. Subramanian | Dr K. R.V. Subramanian received his Ph.D. in nanotechnology from the University of Cambridge, UK and subsequently completed a Post-Doctoral term at the University of Illinois, Urbana-Champaign, USA. He worked as an Associate Professor at the Amrita Center for Nanosciences and Molecular Medicine, Kochi, Kerala, India. He worked as the Assistant Director of the Shriram Institute of Industrial Research, Bangalore, India. He is currently working as an Associate Professor at Department of Mechanical Engineering, GITAM University, Bengaluru, India. His research areas are thin films and semiconductor devices, flexible solar cells, supercapacitors and lithium-ion batteries. |
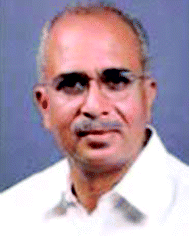 Suresh Mathew | Prof. (Dr) Suresh Mathew received his Ph.D. in Chemistry from the University of Kerala, India and subsequently completed an Alexander von Humbold postdoctoral fellowship at the Fraunhofer Institute fur Chemische Technologie, Karlsruhe, Germany. Presently, he is a Professor of Inorganic Chemistry at the School of Chemical Sciences, Mahatma Gandhi University. He is the Founder Director of the Advanced Molecular Materials Research Centre (AMMRC) and a Former Director of the School of Chemical Sciences, Mahatma Gandhi University, Kottayam, India. He was a Visiting Professor at Lunghwa University of Science and Technology, Taiwan and at Rzeszow University of Technology, Poland. His research areas are materials chemistry, flexible solar cells and metal–organic frameworks. (E-mail: sureshmathewmgu@gmail.com) |
1. Introduction
The modern era of technology has created unprecedented transformations in the lifestyle of the human race and has led to the introduction of many electronic, electrical, mechanical and photovoltaic devices. Excessive usage of these devices has increased rates of energy consumption to new peaks, and the excessive consumption of traditional energy sources is leading to their accelerated depletion. An inevitable consequence of this phenomenon is that energy shortage has become a major issue which, if not solved in the near future, will lead to a severe energy crisis.1 This conundrum creates the necessity to explore more renewable energy sources, such as solar cells.2 When looking top-down at the evolution tree of solar cells, one can see that the first generation of crystalline solar cells comprises semiconducting materials, such as silicon and germanium, as the light harvesting materials.3 However, the rigid textures of solar modules and their expensive manufacturing processes, which cause long payback periods, are the main problems with these types of solar cells.4 The second generation of solar cells includes cadmium telluride/cadmium sulphide, copper/indium/gallium selenide and amorphous silicon-based thin film solar cells.5 They are inexpensive compared to their predecessors; however, they have lower efficiencies. Third generation solar cells include polymer-based and dye/quantum dot-sensitized solar cells.6 Dye-sensitized solar cells (DSSCs) were invented by Brian O'Regan and Michael Grätzel in 1991.7 Production of these solar cells is facile compared to the first and second generation technologies; while their efficiencies are currently inferior to those of first and second generation devices, the steady growth of their efficiency is promising.8 Another advantage of DSSCs is their impressive performance under artificial light, which makes them suitable for indoor applications (better performance compared to other devices in diffuse light).9 Among the three generations of solar cells, research activities to produce mechanically flexible second and third generation solar cells have been ongoing since the onset of the last decade.10
To address the rigidness and brittleness of conventional opaque solar cells, technologists are currently studying photovoltaic devices with versatile features such as light weight, portability, wearability and flexibility with superior mechanical stability to satisfy the needs of advanced lifestyles.11 These properties, in principle, should enable facile installation of flexible devices on irregular surfaces and decrease the difficulties in their installation and carriage.12 Thus, flexible solar cells13 are more appealing than rigid solar cells from an operational point of view. Flexible solar cells14 have potential terrestrial and space applications because of the ease of installing flexible devices of any shape on any surface.15 Conventionally, the fabrication of flexible devices involves dispersing or dissolving functionally active opto-electronic materials, such as nanoparticles and super lattices, in suitable solvents to form liquid or gel phase dispersions.16 It has been very well demonstrated that the properties of solvent-assisted systems are largely controlled by the solvent and its polarity.17,18 However, these solvents are usually volatile, which decreases the stability of the devices as they age.19 In addition, gradual solvent loss can create concentration gradients in the device over its performance period and can increase the electrical resistance of the device,20 thus decreasing its efficiency.
A multitude of published articles have extensively explored the synthesis and photo-electrochemical properties of novel optoelectronic materials, such as fullerenes, carbon nanotubes, graphene, and their composites, for use in solar cell applications.21 These materials are well known for their superior conductivities, charge transfer characteristics and other electrical properties.22 However, in virtually all these reports, the functionally active materials used to fabricate the devices are in the solid state, which ultimately results in devices with rigid morphologies. Recently, non-volatile, less viscous, functional molecular liquids/gels were proposed as potential materials for use in optoelectronic device applications.23 The advantage of using these materials is that no solvent is required to make slurries of the optoelectronic materials; therefore, any complications that may arise from using solvents in a device can be circumvented. These materials are developed by chemical functionalization of suitable molecules with long aliphatic side chains/bulky aromatic functional moieties.24 Recent work has demonstrated an approach for preparing functionalized organic and inorganic nanostructures with liquid-like behaviours.25 These structures are produced by grafting an organic canopy layer consisting of long aliphatic side chains onto the surfaces of charged nanoparticles of fullerenes, silica, iron oxides, titanium dioxide, etc. through electrostatic interactions; this results in solvent-free nanoparticle ionic fluids.
The synthesis of luminescent solvent-less fluids26 and triplet–triplet annihilation (TTA)-based photon upconversion (UC) in a fluid system with a triplet sensitizer, Pt(II) porphyrin, as a dopant and a 9,10-diphenylanthracene-based fluid acceptor with a high upconversion quantum yield of 28% was recently reported.27,28 Another article describing the synthesis of composites consisting of an oligo-(p-phenyleneethylene) (OPE) derivative and solid fullerene (C60) demonstrated the successful synthesis of room temperature solvent-free fluids from solid precursors.29 Solvent-free liquids can incorporate various dopants to form composites which may find future applications in the energy harvesting sector, energy storage devices, sensing, and light emitting diodes.30 The performance of these devices can be improved by tuning the crystalline properties of the nanomaterial assembly by optimized and precise synthesis.31
In this perspective article, we discuss the possibilities of integrating liquefied organic and inorganic semiconducting materials with tunable optoelectronic properties into solvent-free fluidic systems of functional optoelectronic materials to generate flexible devices. We anticipate that these materials will open a new door to the next generation of all-liquid-based organic optoelectronic devices. A trending technology, dye-sensitized solar cells (DSSCs), fabricated using semiconducting materials coated on flexible plastic sheets such as polyethylene terephthalate (PET)/polyethylene naphthalate (PEN), face serious stability issues and short operational lifetimes because of the volatile components present in the DSSCs, which evaporate over the lifetime of the device.32 Solvent-free nanofluids with high melting points integrated into flexible solar cells where the functional materials are in a liquid/gel state have thus become a necessity rather than a requirement in this scenario; these devices can demonstrate high flexibility during operation, which can be a great advantage in practical applications. These devices can be fabricated by functionalizing their active materials via facile synthetic routes. The morphological properties of the materials modified with functional molecular liquids can be readily tuned33 by engineering their molecular structures and, hence, their molecular packing without compromising their optoelectronic properties. The optoelectronic properties of the required molecular liquids depend on the functional modifier molecules used for surface modification as well as the synthesis route by which the final molecular liquid is synthesized.34
2. Theory behind liquefaction
Recent research articles have discussed a number of interesting methods to develop solvent-free nanofluids/gels.35 Articles reporting liquid fullerene at room temperature generated by surface modification are some notable examples; among these, the development of fulleropyrrolidines substituted with a 2,4,6-tris(alkyloxy)phenyl group which can remain in fluid phase at room temperature is a proof of concept of this method.36 In this article, fullerene derivatives were synthesized by refluxing (alkyloxy)benzaldehyde components with N-methylglycine and fullerene powder in dry toluene. The fulleropyrrolidines synthesized by functionalization with alkyloxy-phenyl groups containing linear and branched alkyl chains are stable at ambient conditions and exhibit liquid-like properties without agglomeration of fullerene particles. Fulleropyrrolidines with shorter chain lengths showed high melting points due to π–π interactions between adjacent C60 moieties. In longer chain molecules, van der Waals interactions between the alkyl chains dominate the π–π interactions, causing increases in the melting points of the resulting liquids. At medium chain lengths, a competition between these two intermolecular forces results in decreased melting points, and liquid-like features are observed. Thus, the prepared fullerene derivatives appeared as dark brown oils and were stable at room temperature. Rheological properties affirming the liquid-like behaviours of these fullerene derivatives were also reported in some literature studies,37 and it was reported that the crystallinity of the resulting materials were found to decrease as the liquid behaviour increased.
Recent reports have showcased the synthesis of liquids of TiO2, ZnO, porphyrin, etc. by surface modification using similar methods. A yellow TiO2 nanofluid which is stable at room temperature was synthesized using the surface modifier (CH3O)3Si(CH2)3O-(CH2CH2O)6-9CH3.38 TiO2 nanoparticles coated by an organic canopy layer showed better dispersion properties after surface modification. The synthesis of ZnO nanofluids demonstrating good stability was reported in a recent article.39 In this report, transformation of the ZnO nanoparticles into the liquid phase was achieved by reaction of the ZnO nanoparticles with an organosilane compound, [(CH3O)3Si-(CH2)3N + (CH3)(C10H21)2Cl-], through covalent interactions of the positively charged silane moieties with the surface hydroxyl groups on the nanoparticles. The resulting compound was then allowed to react with poly(ethylene glycol) (PEG)-tailed sulfonate anions, leading to the interchange of ions present in the compounds; this resulted in the formation of nanofluidic ZnO. With similar motives of liquefying active materials, recent literature studies reported the development of liquid porphyrins.40 Porphyrins with trialkoxyphenyl substituents with liquid-like properties at room temperature were synthesized in a few simple steps.
Focusing on the science behind this uncommon phenomenon and following the same rationale implied in the case of fluidic fullerenes, one can reach a general conclusion that when nanoparticles are modified into a nanofluid, the system containing a network of armed (modifier-attached) nanoparticles is constrained in a very limited space and repulsive interactions between adjacent neighbours becomes increasingly significant. This restriction forces the neighbouring molecules to remain at a specific distance from each other; as the intermolecular attractions begin to loosen and when an optimum intermolecular distance is attained through chemical modification, the sample begins to behave like a liquid.41,42 This peculiar phase transformation is shown in Fig. 1. Studies have shown that the distinction between the liquid and vapour phases of some materials disappears above the liquid–vapour critical point.43 The liquid range depends on the intermolecular forces between the components. When the surface of a material is modified with branched substituent chains, this branching can break the molecular symmetry of the parent material and can afford liquid-like products; however, the degree of branching should be optimum to obtain a stable liquid phase material.44 When the branching extends beyond the optimum level, the stability of the molecular system decreases; this leads to highly viscous, solid-like materials.45Fig. 2 shows images of a solvent-free anthracene nanofluid which was developed using the methods mentioned above.
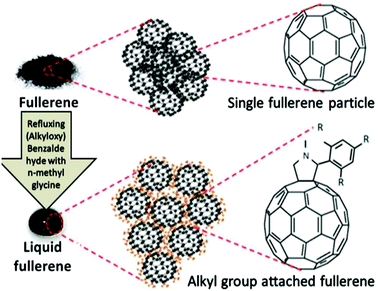 |
| Fig. 1 Schematic of the formation of a solvent-free room temperature liquid form of fullerene powder. | |
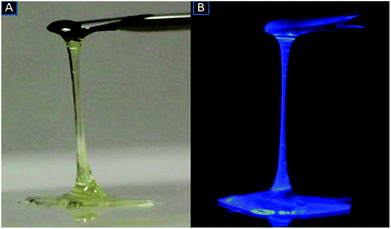 |
| Fig. 2 Photographs of solvent-free liquid anthracene (A) under visible light and (B) under UV light. Reprinted from S. S. Babu et al. Non-volatile liquid anthracenes for facile full colour luminescence tuning at single blue-light excitation, Nat. Commun., 4, 1969, DOI: 10.1038/ncomms2969 (2013) under Creative Commons Attribution 3.0. | |
Leading technologies in the optoelectronic device industry of flexible solar cells and OLEDs include organic heterojunctions and polymeric material-based technology.46,47 Third generation solar cells are comparatively new in R & D, and the development of these devices is in its infancy compared to the three generations of rigid solar cells.48 The third generation devices, dye-sensitized solar cells (DSSCs)49 and quantum dot-sensitized solar cells (QDSSCs), have secured their own places in the market by establishing improved efficiencies within a short period of time.50 It is essential to fabricate flexible and solvent-free dye/quantum dot-sensitized solar cells to obtain the benefits of mechanical flexibility. Combining the advantages of the two technologies, portable energy harvesting devices with high degrees of pliability and ease of installation without compromising efficiency can be developed; this may represent a tremendous improvement in the energy harvesting field.
3. Electronic and electrical properties of liquefied materials
Hundreds of molecular dyes, nanomaterials, and polymers have been reported in the literature as candidates for developing functional solvent-free, gel-type components of DSSCs/QDSSCs; thus, a thorough understanding of both the electronic and electrical properties of the materials in the gel form is required to develop combinatorial designs as integral components of all-flexible solar cells. Nonvolatile molecular liquids of anthracene51 show strong electronic absorption of UV-visible radiation to an extent comparable to that of the respective free functional dye molecules in dilute solution. In addition, they show high luminescence yields, with emission spectral characteristics similar to those of the free dye molecule in dilute solution; this implies monomeric features of the functional dyes in the non-volatile liquid state. The question of whether molecules in the liquid phase show excitonic properties dates back to the 1960s, when, in 1966, Rice and Jortner52 demonstrated that Wannier-type exciton states can exist in dense liquids, given that the conduction electrons in these liquids exist as plane waves and that localized excitations arising from poor overlap of molecular excited states are likely to propagate via diffusional hopping. The excitonic properties of non-volatile molecular liquids have been poorly elucidated to date; this is presumably due to the recent origin of these materials. However, the fact that these materials show decent charge carrier mobilities when being used in several applications (vide infra) demonstrates that they do form electrons and holes with comparable mobilities.53 The questions that now arise are: do excitons exist in these types of dense molecular liquids, and what are the natures and lifetimes of the excitons? If excitons do exist, is intermolecular exciton transport possible in these systems? If this transport is possible, what is the range of the exciton transport length and what are the factors limiting it? Can singlet or triplet exciton annihilation occur in these systems? It should now be mentioned that the observation of triplet–triplet annihilation (TTA) between liquefied DPA (A) molecules following triplet energy transfer from Pt(II) porphyrin (D) (both A and D in liquid form) (vide supra) has been attributed to long-range triplet energy migration between adjacent liquid DPA (A) molecules.28 Is this true triplet exciton migration and subsequent annihilation, as one would observe in crystalline, ordered systems? The questions raised above must be answered by employing both photophysical and electrochemical studies to gain a deeper understanding of how these systems can be used in optoelectronic devices.
Solvent-free liquids show comparable ionic conductivities to aqueous solutions.54 It is worth mentioning that in a fluidic carbazole system consisting of 9-(2-ethylhexyl)carbazole, a C60 – poly(N-vinylcarbazole) photorefractive material showed an appreciable charge carrier mobility55 of 4 × 10−6 cm2 V−1 s−1. When the side chain of this liquid carbazole was replaced with a linear poly(ethylene oxide) chain, a new liquid carbazole was obtained which showed a significant increase in charge carrier injection when used in a liquid OLED device.56 Interestingly, a liquid derivative of fluorene consisting of siloxane side chains was reported to exhibit better hole and electron mobilities of 1.30 × 10−4 and 1.53 × 10−4 cm2 V−1 s−1, respectively.57 Likewise, triarylamine-based liquid semiconductors showed a hole mobility of 1 × 10−4 cm2 V−1 s−1, which is comparable to that of the liquid fluorine derivative mentioned above.58
Aqueous solutions exhibit conductivity due to the dielectric and fluid properties of water.59 Conductivity in solvent-free nanofluids can be attributed to the Grotthus mechanism,60,61 where an excess proton or proton defect diffuses through the alkyl chains which are attached on the surface of the nanomaterial. The electrical conductivity of the liquefied system can be increased by selecting suitable chains bearing cations and anions and by allowing proton exchange between them.62 Increasing the temperature of the fluid system to an optimum value can greatly facilitate enhanced electrical conductivity without evaporation due to the non-volatile nature of the liquid. However, the alkyl chains can adversely affect the electrical conductivity of the material, where an increase in chain length may lead to an increase in the path length of Grotthuss proton transport and, hence, a decrease in conductivity.63
4. Flexible DSSCs/QDSSCs
A brief introduction of the working principles of normal DSSCs64,65 and QDSSCs66 is required to understand their working principles in flexible mode, where their rigid components are replaced by flexible ones. A DSSC/QDDSC consists of three functional parts: (1) a solar light-absorbing element, usually a deeply coloured organic dye molecule67 or a precisely synthesized quantum dot68 of a semiconductor with a high degree of light absorption, which can convert an absorbed photon into an exciton; (2) an electron acceptor (photoelectrode), which separates the exciton69 into electrons and holes by virtue of the energy difference between the LUMO/conduction band (C.B.) of the light harvester and the conduction band of the electrode;70 and (3) a redox couple, which injects the electron back to the dye/quantum dot. Electrons from the excited sensitizer travel through the photoanode, conducting electrode and counter electrode to complete the outer circuit. When the oxidized photosensitizer is regenerated by the electrolyte redox couple,71 the cycle is completed. In addition to the light harvesting ability of the light sensitizer, its charge separation and transport through the circuit and subsequent recombination, the redox potential of the electrolyte and the open circuit voltage of the device are the major factors that affect the efficiency of third generation solar cells.72 We will examine here in detail how these factors pose challenges in conventional DSSC/QDSSC devices and how the introduction of solvent-free, non-volatile gel-type functional components in a DSSC/QDSSC can potentially mitigate these issues.
4.1 The photoanode
When one considers the details of the fabrication steps of DSSCs/QDSSCs, the first element in the solar cell to be discussed is the photoanode.73 The flexibility of the photoanode sheet can be improved by replacing conventional photoanode materials, which are usually nano-crystalline solid films obtained by the thermal treatment of dispersions of semiconductors in solvents,74 with solvent-free gel phase photoanode materials. Semiconducting materials that are widely used for making photoanodes of solar cells can be converted into solvent-free liquid/gel forms and coated on flexible sheets of conducting electrodes. Photoanode films which are fabricated by surface functionalization will have lower chances of cracking and tearing because they are free of solvent evaporation problems; thus, the durability of the devices can be enhanced. A suitable photosensitizer can be embedded into the photoanode in the gel form, which can be very useful for QDSSC fabrication. In QDSSCs, the photosensitizer material, quantum dots, are usually adsorbed onto the photoanode material.75 The binding between the quantum dots and the photoanode material is usually a serious problem that arises in the device fabrication stage of QDSSCs. Conventionally, a linker molecule76 chemisorbed onto the quantum dot binds to the semiconductor photoanode. However, because the linker molecule is usually a non-conducting material, this decreases the conductivity of the system. Instead of this fabrication technique, embedding quantum dots directly into a gel-phase photoanode can strengthen the photosensitizer–photoanode linkage because the photosensitizer is encapsulated in the gelated photoanode material. Therefore, breakage of the bonds between the photoanode and the photosensitizer may be less likely to occur. Because the solvent evaporation problem and the consequent cracking of the photoanode material can be avoided, we perceive that the photoanode gel with the photosensitizer embedded in it will stick more readily to the substrate. Because the prepared quantum dots can be directly embedded in the photoanode gel, the quantum dot loading on the photoanode substrate can be improved compared to the extent of loading achievable by immersion and drop casting techniques. It is reasonable to expect that the functionalized liquid/gel photoanode will interact more strongly with the photosensitizer compared to the non-liquefied/non-gelated form of the photoanode material. Better interaction between the functional groups present in the surface-modified photoanode and the quantum dots will facilitate better charge separation and can lead to better efficiency.
4.2 The counter electrode
The counter electrode in a dye/QD sensitized solar cell aids the regeneration of the oxidized dyes by supplying electrons from the reduced form of the electrolyte. Rapid and efficient reduction of the electrolyte molecules is essential to decrease the rate of electron recombination. To meet this requirement, the counter electrode must have excellent electro-catalytic activity, electrochemical stability, charge transfer, and conductance, as well as a high degree of corrosion resistance. In addition to these attributes, the morphology and the roughness of the surface of the counter electrode can affect the efficiency of the DSSC/QDSSC. Dye/QD-sensitized solar cells usually use platinum electrodes as the counter electrode.77,78 Because platinum is a costly material, reasonable efforts have been invested by several groups to find less expensive alternatives of Pt metal.79–81 One plausible way in which flexible counter electrodes can be integrated in all-flexible dye-sensitized solar cells is by coating the counter electrode material onto flexible conducting substrates, such as sheets of polyethylene naphthalate (PEN)/polyethylene terephthalate (PET).82 A number of articles have reported that electrochemically reduced graphene oxide multilayer films can be used as efficient counter electrodes for solvent-free ionic liquid electrolyte-based DSSCs.83 A recent article described the employability of robust organic materials as counter electrode materials in DSSCs.84 Less expensive conducting polymer-based and carbonaceous-based materials exhibiting reasonable electrical conductivities, excellent electrochemical stabilities, electro-catalytic abilities and high energy conversion efficiencies in the range of 7% to 9% are reported to be promising replacements for Pt metal. Some combinations of dye electrolyte-counter electrode systems (e.g. the combination of a PEDOT counter electrode with the Co2+/Co3+ redox couple and Y123 dye with 10.3% efficiency) yielded energy conversion efficiencies even higher than those of their Pt-based counterparts.85 DSSCs fabricated with PEDOT as the counter electrode, the T2/T− redox couple as the electrolyte and Z907 dye showed a significant enhancement in efficiency compared to DSSCs with Pt metal as the counter electrode.86 An article describing the performance of a quasi-solid DSSC87 reported an enhancement in the photocurrent of a DSSC incorporating a PEDOT-PSS blend as the counter electrode and a gel form of 1-methyl-3-propylimidazolium iodide, 2,4,5-tetrakis(bromomethyl)benzene and polyvinylpyridine as the electrolyte compared to the photocurrent generated in a DSSC with a Pt counter electrode and the same gel electrolyte. This is attributed to a decrease in the charge transfer resistance between the gel electrolyte and the PEDOT-PSS counter electrode due to a decrease in the hindrance of charge flow through the network structure of the gelated electrolyte-counter electrode interface; this apparently does not occur in the latter system. These reports unanimously indicate that switching from conventional Pt to conducting polymers or carbonaceous materials to fabricate the counter electrode can be advantageous when the electrolyte is in the gel form.
4.3 The redox electrolyte
A redox couple (electrolyte), such as a tri-iodide based,88 polysulphide-based,89 or cobalt-based electrolyte,90,91 is usually used in third generation solar cells. These electrolytes are synthesized by dispersing the redox couple material in a suitable solvent. The solvents commonly used for fabricating these electrolytes are highly volatile. A major drawback of DSSCs is that solvent evaporation can occur outdoors, leading to changes in the concentration of conventional electrolyte in the device. Solvent evaporation can lead to aggregation of the electrolyte constituents and, hence, can decrease the electron mobility.92 Moreover, because the function of the electrolyte is to regenerate the oxidized photo-sensitizer by supplying electrons, solvent evaporation can potentially affect the electron transfer cycle and can decrease the lifespan of a solar cell.93 This problem was initially addressed by employing gel-type94 or ionic liquid-based electrolytes containing very little or no solvent.95 In order to briefly recount the recent developments in the field of gel-based electrolytes96 used in DSSCs, we will firstly discuss polymer gelator-based electrolytes such as polyethylene glycol,97 polymethyl methacrylate,98 polyacrylonitrile99 and polyvinylidene fluoride;100 these have recently received wide attention due to the tunable flexible structures of their molecular components. Devices manufactured using gel-type electrolytes101 were found to be robust because of their long-term stability and facile and rapid charge transfer between the electrolyte102 and electrodes due to the formation of hybrid network structures.103 A recent article104 describing the performance of a gel state DSSC using anodic TiO2 nanotube arrays and polymer-based gel electrolytes estimated that the overall power conversion efficiencies of these DSSCs were comparable (6.9%) to that of a liquid-type device (7.1%) while retaining ∼90% of their initial efficiency after 1000 h under 1 sun of illumination at 50 °C; meanwhile, a DSSC with a liquid-state electrolyte was found to be unstable, with poor performance after light soaking for 500 h. This finding is supported by another report105 which provides evidence for performance enhancement of DSSCs based on a (poly(oxyethylene)diamine amide–imide based gel electrolyte compared to a DSSC based on liquid electrolyte. Moreover, the overall power conversion efficiencies of DSSCs using gel-type electrolyte were found to decrease by only a marginal percentage of 5% after 1000 h of light illumination; meanwhile, the DSSCs containing liquid-type electrolytes showed a substantial decrease of efficiency by a factor of 39% after 1000 h of illumination under identical conditions. Another category of electrolyte that can be used in DSSCs is inorganic–organic hybrid polymer gelator-based materials, such as polyphosphazenes.106–108 These materials have unique advantages over classical organic polymers. The polyphosphazene macromolecules with phosphorus–nitrogen backbones and organic side groups provide great opportunities for structural diversity when they are used for surface functionalization. The presence of side groups allows easy tuning of the desired structures and properties of polyphosphazenes by simple replacement of the side groups with different groups. Some studies report that polyphosphazene-based gel electrolytes with iodide salts show better efficiencies when used in DSSCs.109 These findings unambiguously suggest that replacing liquid-type electrolytes with gel-type electrolytes110 can have significant impacts on the stabilities, structural flexibilities and energy conversion efficiencies of DSSCs/QDSSCs. The performance of polymer gelator-based electrolytes can be enhanced by incorporating additives such as tetrapropylammoniumiodide, 1-methyl-3-propyl imidazolium iodide and 4-tert-butylpyridine.111 The addition of these materials was reported to lead to an increase in the conductivity of the electrolyte, which resulted in better short circuit current density (Jsc). This finding was substantiated by the report of a quasi-solid-state dye-sensitized solar cell with a gel-type iodide anion electrolyte based on a poly(oxyethylene)-imide-imidazole complex, which yielded a high power conversion efficiency as well as good long-term stability.112
A recent addition to the list of these materials is nanocarbon materials such as carbon nanotubes; when integrated into the electrolyte, these materials facilitate charge transfer from the counter electrode to the electrolyte more efficiently, thus accelerating the rate of the dye regeneration process.113 A new member of the family of gel type electrolytes is highly stable solvent-free ionic liquid electrolytes, which were found to perform very well when integrated into a solar cell device.114 Of this category of ionic liquids, 1-alkyl-3-methylimidazolium iodides with lengthy alkyl chains showed high viscosity and low conductivity because of strong van der Waals forces between the chains.115 However, a DSSC containing an ionic liquid-molecular gelator with an optimum chain length was reported to give better performance, an IPCE greater than 5%, and high temperature stability under AM 1.5 irradiation.116 Another case study of a DSSC containing a ionic liquid-polymer gel electrolyte composed of 1-methyl-3-propylimidazolium iodide and poly(vinylidinefluoride-co-hexafluoropropylene) reported a power conversion efficiency of 5.3% under AM 1.5 illumination.117 While gelation increased the viscosity of the electrolyte in this case, the diffusion coefficients of the iodide and triiodide ions were not greatly affected by this process; this is largely because the distribution of passages that opened throughout the gel electrolyte facilitated efficient iodide and triiodide diffusion.
Evidence is mounting that gelation of the electrolyte has no significant adverse effects on the energy conversion efficiency of a solar cell if it is precisely fabricated. Compared to polymer and ionic liquid-type gelators, solvent-free molecular liquids as gel-type electrolytes have the following advantages: ease of synthesis; opportunity to easily tune the chain length and, hence, the branching; potential to tune the rheology through regulation of molecular packing; creation of open passages in the branched framework to permit ion diffusivity; and, finally, increased device stability due to the non-volatile nature of molecular liquids. Molecular liquid electrolytes with alkyl chains at their terminal ends should be able to maintain the solubility of active materials in the electrolyte liquid and regulate their self-assembly behaviour. Recently, Grätzel's group introduced a new cobalt(II/III)tris(bipyridyl)-complex-based redox electrolyte which performed well in DSSCs, achieving an open circuit voltage of about 1 V and a power conversion efficiency of about 12% under AM 1.5 sunlight-type conditions.118 The cobalt electrolyte can be converted into a non-volatile gel-like electrolyte form via structural modification. We recently synthesized a non-volatile cobalt(II/III) tris(bipyridyl) electrolyte in our lab by functionalizing the complex with a positively charged silane moiety and a PEG-tailored sulfonate anion (the detailed synthetic procedure and rheological and electrochemical characterization are subjects of a future manuscript); this electrolyte is presented in Fig. 3. A thorough study of the performance of the non-volatile electrolyte in various dye/QD-sensitized solar cells is required to determine the best-performing combinations of photoanode–electrolyte systems; these are subjects of active research in our lab. The open circuit voltage must be determined for devices in which the redox electrolyte is combined with various semiconductor nanoparticles with different Fermi levels.119
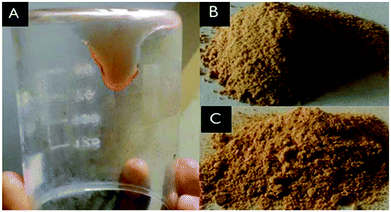 |
| Fig. 3 Photographs of a non-volatile cobalt(II/III) electrolyte (A) and cobalt(II) (B) and cobalt(III) (C) precursor complexes developed in our lab. | |
4.4 Factors that affect charge transfer and transport
The energy level matching of the dye/QD and the photoanode material and the extent of binding between them directly affect the efficiency of charge transfer from the photosensitizer to the photoanode and the further mobility of charge carriers.120 When the electrostatic and noncovalent interactions between two adjacent dye molecules exceed the interaction energy between the dye and the photoanode surface, aggregation of dye molecules can occur, which hinders the dye from anchoring onto the photoanode surface. Dye–dye interactions can be weakened by adding coadsorbents, such as deoxycholic acid and chenodeoxycholic acid.121 Alternatively, enhanced dye loading without agglomeration can be achieved by functionalizing the dye molecules with substituents such as phosphazenes to facilitate noncovalent N⋯S interactions, which can endow the molecules with desired planarity and thus prevent dye–dye aggregation.122 Further studies should be carried out to optimize the nature and functionalization strategies of these groups when considering them for introduction into liquefied systems. One of the challenges posed by liquefaction is that the introduction of non-conducting surface modifier moieties can increase the distance between the dye/QD and the photoanode; also, the modifier can act as a physical and an electrical barrier between them. This hinders charge transport and can decrease the efficiency of the charge transfer process. This issue can be solved by selecting an optimum surface modifier by examining its charge transfer kinetics and its efficiency at the dye/QD-photoanode interface separated by modifiers with varying chain lengths. Surface modifiers that preserve the planarity of the dye molecules while facilitating rapid and quantitative charge separation and reduced recombination yield should be de facto candidates.123 However, it should be noted that achieving mechanical flexibility involves a trade-off with the decrease in conductance that occurs when the solid is transformed into a liquid. When the ratio of the amount of semiconducting photoanode material with respect to the amount of surface modifier increases or the length of the modifier chain decreases, the thickness of the liquid increases; this ultimately leads to the formation of a gel,124 and the gel solidifies when an excess of the photoanode material is added. This criterion posits a need to tune the amount of photoanode with respect to the amount of modifier to an optimum level in order to obtain mechanical flexibility while retaining better charge transfer properties.
One major drawback of dye-sensitized solar cells is that they become heated under direct sunlight when placed outdoors; this can cause the solvent used in the electrolyte to evaporate. Moreover, the photoanode material coated on the conducting electrode may become heated when exposed to sunlight over a long time; as a result, cracks or surface irregularities may form. Micro-cracks can create a grid-like morphology and can act as trap sites of the charge carriers.125 However, if a solvent-free, non-volatile gel-type photoanode, such as one reported to withstand temperatures up to 65 °C,126 is used, the formation of surface irregularities can be prevented. Furthermore, thermal heating of the gel photoanode sheet by sunlight can be advantageous because the ionic conductivity usually increases with increasing temperature.127 The liquefaction of the materials may decrease the charge transport properties due to weakened intermolecular interactions; this can be compensated by adding superior conductive materials, such as graphene, to the gel. A multitude of papers have reported enhancements in the electrical conductivities of photoanode semiconductors when graphene is added in an optimum quantity.128 Conducting additives such as graphene, a ballistic transport medium of electrons,129 can promote electron transport if the HOMO–LUMO of the dye and conduction band-valance band thresholds of the photoanode and graphene are suitable. It is logical to anticipate that a gel in a photosensitizer–photoanode system with graphene will benefit from the advantages of flexibility without compromising conductivity. Further studies should be carried out to identify appropriate dye/QD combinations with energy levels that match those of the photoanode semiconductor (e.g. TiO2) and conductivity-enhancing additive (e.g. graphene) and surface modifier moieties with optimum chain lengths. A schematic energy level diagram of such a gel system is given in Fig. 4. The solvent-free gels should be able to withstand direct sunlight; also, if dyes/QDs with near infra-red absorption regions are used as photosensitizers, non-radiative relaxation of the thermalized excitons can heat the system and increase the charge carrier mobility of the gels. A thermoelectric device can also be introduced to the device to supply thermal energy.86
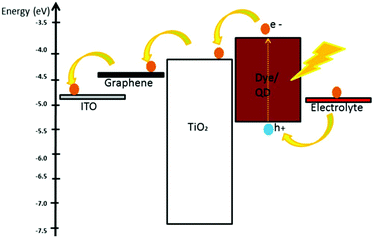 |
| Fig. 4 Schematic of the energy levels and electron flow in a graphene-based photosensitizer–photoanode gel system. | |
Theoretically, all the dye molecules reported to date as potential light absorbers in conventional DSSCs can be liquefied by optimizing their intermolecular interactions via attaching flexible, bulky alkyl chains or siloxane chains onto the dyes. However, the branching ratio of the aliphatic side chain must be tuned to enable optimum exciton diffusion, dissociation and charge separation when used in a device. In addition, it is very well known that the molecular structure of the dye and the nature of its binding mode (monodentate, bidentate or higher order dentate) on the photoanode play a crucial role in deciding the electron transfer kinetics between the dye and the photoanode130–132 and the magnitudes of JSC and VOC.133 Therefore, we can reasonably propose that the small planar conjugated organic molecules used in conventional devices can be directly embedded in a photoanode material in the gel form. Alternatively, a gel form of these sensitizer molecules (obtained by functionalization with long flexible functional aliphatic side chains) could be embedded in or mixed with the photoanode material in the gel form. In this case, because both the dye and the photoanode material bear long, flexible aliphatic side chains, we anticipate that van der Waals interactions among the side chains will lead to an intertwined, hybrid network structure of the gel phase dye–photoanode composite; however, this possibility should be verified experimentally. Regardless, the formation of such a structure could result in a distribution of the spacing between the dye and the photoanode and their relative orientation with respect to each other. This could affect the rates of electron transfer and back electron transfer between the dye and the photoanode, the extent of electronic coupling between the dye and the photoanode surface states, and, hence, the extent of charge transfer. Therefore, the former approach of directly embedding sensitizer molecules (with anchoring groups) onto a fluidic photoanode system appears to be the simplest, most workable method. However, the long functional groups used to jellify the photoanode material could increase the physical separation between the dye molecules and the photoanode material. This could decrease the efficiency of both the forward and backward electron transfer processes. This should be resolved by determining the optimum side chain length of the photoanode material. A list of liquefiable materials53 currently being used in conventional DSSCs49,135 that can potentially be applied in solvent-free flexible DSSCs/QDSSCs is given in Table 1.
Table 1 List of possible nanoliquids that can be used in the solar cell industry135
Photoanode |
Photosensitizer |
Electrolyte |
Inorganic semiconductors such as TiO2 and SnO2 |
Low-melting phthalocyanines and porphyrins |
Transition metal-based electrolytes |
Carbonaceous materials such as fullerene and graphene |
Pyrene, perylene, anthracene, coumarin, indoline, quinoline, aniline, cyanine, squaraine, carbazole and triarylamine-based dyes |
Polysulphide and disulphide/thiolate electrolytes, such as smectic 1-dodecyl-3-methyl-1H-imidazolium 1-methyl-1H-tetrazole-5-thiolate/di-5-(1-methyltetrazole) disulfide electrolytes |
Semiconductor-MOF composites, semiconductor-cyclodextrin composites |
Quantum dots and metal-based dyes, such as N719 black dye |
Cobalt complexes, such as [Co(bpy-pz)2]3+/2+(PF6)3/2 |
5. Device structure of all-flexible DSSCs/QDSSCs
A flexible solar cell whose components all consist of non-volatile, solvent-free elements can be expected to have an extended lifespan. A possible device configuration includes a photoanode nanogel-coated flexible PET/PEN sheet embedded with dye/QD and sandwiched with a flexible counter electrode; an electrolyte in gel form can be filled in between the sandwich structure. A schematic of such a device is shown in Fig. 5. The excitation and electron transport paths through the conduction band of the photoanode (such as TiO2) from the LUMO/C.B. of the oxidized dye/QD are shown in Fig. 6. Solvent-free flexible solar cells can be fabricated by making all the three components of the device, viz. photosensitizer, photoanode and electrolyte, solvent-free and flexible. An alternate strategy is to mix solvent-free gels of the electrolyte and the photoanode to form a composite; then, the photosensitizer in the solid state can be directly embedded into the composite gel. Even though these materials, after being converted to liquid/gel forms, should have significant advantages over their crystalline, rigid predecessors, employing flexible photoanodes and non-volatile electrolytes in a device without compromising their conductivity is a difficult task. Sophisticated and precise methods of synthesis are required to overcome this issue. In order to maintain fluidity while retaining the conductivity of the material, the branching ratio of the aliphatic side chains functionalized onto the nanomaterials must be tuned to an optimum level to enable optimum intermolecular interaction and charge transfer properties. To improve the performance of electrolytes in DSSCs, lithium perchlorite and pyridine-based additives are usually added to the electrolytes.134 These additives can also be introduced in gel-type electrolytes for better performance. To integrate these electrolytes into DSSC modules with precise morphologies, textures and structural integrity, direct ink-jet printing can be employed.136 It should be remembered that ink-jet printing of electrolytes based on volatile solvents such as acetonitrile is difficult due to ready evaporation of the solvent and subsequent solidification of the matrix.137 In this article, we have discussed a number of possible ways in which materials such as dye molecules, fullerenes, porphyrins, electrolytes, and TiO2 can be converted into solvent-free liquids so that they can be employed in the fabrication of flexible DSSC/QDSSC devices. Molecular-level engineering of the materials by tuning the branching ratio of the side chains can result in materials with desired morphological and electrical properties. Photoconductive solvent-free composites can be synthesized from combinations of such materials, and they can be utilized to fabricate solvent-free flexible solar cells.
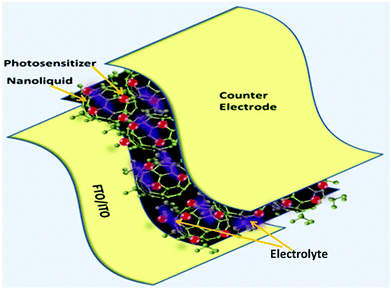 |
| Fig. 5 Schematic of a solvent-free flexible third generation solar cell. | |
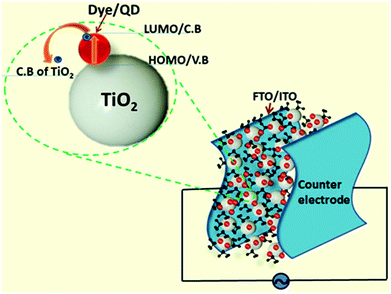 |
| Fig. 6 Schematic of the working mechanism of solvent-free flexible solar cells. | |
To recapitulate, converting the functional components of a solid state solar cell device into a non-volatile gel form is highly appealing because it can offer mechanical flexibility and longevity. However, upon transformation from solid to liquid and, finally, to gel, some desirable properties, such as charge separation, transport and recombination, may be affected. However, the materials can be chosen to offset this loss with good mechanical performance and long device lifetime. In addition, a solvent-free system can prevent contamination of the environment with the solvents that would otherwise be used in conventional devices and can potentially help decrease the hazardous “chemical footprint” of the system on the environment.
6. Conclusions and outlook
Converting well-known organic and inorganic photoanode materials, such as fullerene, carbon nanotubes, and TiO2, and conventionally used superior electrolyte materials into flexible and solvent-free liquids/gels is the initial step towards the fabrication of an all-flexible solar cell. The organic modifiers can be covalently grafted to the surfaces of nanoparticles (such as TiO2) to weaken their intermolecular attractive forces, resulting in liquid/gel-type photoanode materials. Embedding a suitable photosensitizer into a liquid phase semiconductor can create a photoanode–photosensitizer composite material in a gel/liquid phase. By modifying the natures and concentrations of the components, the viscosity of the composite product can be tuned. At low vapour pressures, liquid-like materials can be obtained by controlling the structures of the organic modifiers. In a similar way, non-volatile liquid/gel type electrolytes can also be prepared. In this perspective article, we have outlined the challenges that may arise when gel-type components are integrated into solar cell devices and how they could pragmatically be solved. Our optimism prompts us to suggest that according to market requirements, one or all of the components of third generation solar cells can be replaced by surface-modified non-volatile materials, which should be economically viable to produce. This can potentially culminate in the development of inexpensive and stable photovoltaic devices processed entirely from solvent-free components and provide scope for flexible, non-volatile organic and nano-materials in the solar cell industry.
Conflicts of interest
There are no conflicts to declare.
Acknowledgements
We acknowledge Dr S. Anas, Hon. Director, AMMRC, for the encouragement and Mahatma Gandhi University for the generous support.
References
- N. S. Lewis and D. G. Nocera, Powering the planet: Chemical challenges in solar energy utilization, Proc. Natl. Acad. Sci. U. S. A., 2006, 103, 15729–15735 CrossRef CAS PubMed.
-
A. J. McEvoy, L. Castaner and T. Markvart, Solar cells: materials, manufacture and operation, Academic Press, 2012 Search PubMed.
- W. A. Badawy, A Review on Solar Cells from Si-Single Crystals to Porous Materials and Quantum Dots, J. Adv. Res., 2015, 6, 123–132 CrossRef CAS PubMed.
-
M. Kibria, A. Ahammed, S. Sony and F. Hossain, A Review: Comparative studies on different generation solar cells technology, 5th Int. Conf. Enviro. Aspects, Bangladesh, 2014 Search PubMed.
- A. G. Aberle, Thin-film solar cells, Thin Solid Films, 2009, 517, 4706–4710 CrossRef CAS.
- S. E. Shaheen, D. S. Ginley and G. E. Jabbour, Organic-Based Photovoltaics: Toward Low-Cost Power Generation, MRS Bull., 2005, 30, 10–19 CrossRef CAS.
- B. O'Regan and M. Grätzel, A low-cost, high-efficiency solar cell based on dye-sensitized colloidal TiO2 films, Nature, 1991, 353, 737 CrossRef.
- A. T. Arjunan and T. Senthil, Review: Dye-sensitised solar cells, Mater. Technol., 2013, 28, 9–14 CrossRef.
-
Z. Yu, Liquid Redox Electrolytes for Dye-Sensitized Solar Cells, Ph.D. Thesis, KTH Royal Institute of Technology, 2012 Search PubMed.
- M. Pagliaro, R. Ciriminna and G. Palmisano, Flexible Solar Cells, ChemSusChem, 2008, 1, 880–891 CrossRef CAS PubMed.
-
M. K. Singh, in Solar Cells-New Aspects and Solutions, InTech, 2011 Search PubMed.
- M. B. Schubert and J. H. Werner, Flexible solar cells for clothing, Mater. Today, 2006, 9, 42–50 CrossRef CAS.
- Y. Dkhissi, F. Huang, Y.-B. Cheng and R. A. Caruso, Quasi-solid-state dye-sensitized solar cells on plastic substrates, J. Phys. Chem. C, 2014, 118, 16366–16374 CrossRef CAS.
- Y. Han, J. M. Pringle and Y.-B. Cheng, Photovoltaic characteristics and stability of flexible dye-sensitized solar cells on ITO/PEN substrates, RSC Adv., 2014, 4, 1393–1400 RSC.
- K. Otte, L. Makhova, A. Braun and I. Konovalov, Flexible Cu(In,Ga)Se2 thin-film solar cells for space application, Thin Solid Films, 2006, 511, 613–622 CrossRef.
- S. S. Shin, W. S. Yang, J. H. Noh, J. H. Suk, N. J. Jeon, J. H. Park, J. S. Kim, W. M. Seong and S. I. Seok, High-performance flexible perovskite solar cells exploiting Zn2SnO4 prepared in solution below 100 °C, Nat. Commun., 2015, 6, 7410 CrossRef CAS PubMed.
- A. V. Kubarev, E. Breynaert, J. Van Loon, A. Layek, G. Fleury, S. Radhakrishnan, J. Martens and M. B. Roeffaers, Solvent Polarity-Induced Pore Selectivity in H-ZSM-5 Catalysis, ACS Catal., 2017, 7, 4248–4252 CrossRef CAS PubMed.
- J. O. Onah, J. E. Odeiani and U. Ajima, Influence of Solvent Polarity on the Physio-Chemical Properties and Quantitative Determinations of Tenofovir Disoproxil and Emtricitabine with Chloranilic Acid as Complexing Agent, Open J. Phys. Chem., 2013, 3(1), 30–39 CrossRef.
- M. I. Asghar, K. Miettunen, J. Halme, P. Vahermaa, M. Toivola, K. Aitola and P. Lund, Review of stability for advanced dye solar cells, Energy Environ. Sci., 2010, 3, 418–426 RSC.
- S. Lue, P. Lo, F. Huang, K. Cheng and Y. Tung, Correlation between Dye-Sensitized Solar Cell Performance and Internal Resistance using Electrochemical Impedance Spectroscopy, J. Phys. Chem. Biophys., 2015, 5, 1 CAS.
- H. Zhu, J. Wei, K. Wang and D. Wu, Applications of carbon materials in photovoltaic solar cells, Sol. Energy Mater. Sol. Cells, 2009, 93, 1461–1470 CrossRef CAS.
- L. Dai, D. W. Chang, J. B. Baek and W. Lu, Carbon nanomaterials for advanced energy conversion and storage, Small, 2012, 8, 1130–1166 CrossRef CAS PubMed.
- H. Li, S. S. Babu, S. T. Turner, D. Neher, M. J. Hollamby, T. Seki, S. Yagai, Y. Deguchi, H. Möhwald and T. Nakanishi, Alkylated-C60 based soft materials: regulation of self-assembly and optoelectronic properties by chain branching, J. Mater. Chem., 2013, 100, 1943–1951 Search PubMed.
- J.-X. Zhang, Y.-P. Zheng, L. Lan, S. Mo, P.-Y. Yu, W. Shi and R.-M. Wang, Direct synthesis of solvent-free multiwall carbon nanotubes/silica nonionic nanofluid hybrid material, ACS Nano, 2009, 3, 2185–2190 CrossRef CAS PubMed.
- S. S. Babu and T. Nakanishi, Nonvolatile functional molecular liquids, Chem. Commun., 2013, 49, 9373–9382 RSC.
- B. Santhosh, J. Aimi, H. Ozawa, N. Shirahata, A. Saeki, S. Seki, A. Ajayaghosh, H. Möhwald and T. Nakanishi, Solvent-Free Luminescent Organic Liquids, Angew. Chem., Int. Ed., 2012, 124, 3447–3451 CrossRef.
- T. N. Singh-Rachford and F. N. Castellano, Photon upconversion based on sensitized triplet–triplet annihilation, Coord. Chem. Rev., 2010, 254, 2560–2573 CrossRef CAS.
- P. Duan, N. Yanai and N. Kimizuka, Photon Upconverting Liquids: Matrix-Free Molecular Upconversion Systems Functioning in Air, J. Am. Chem. Soc., 2013, 135, 19056–19059 CrossRef CAS PubMed.
- N. Adachi, R. Itagaki, M. Sugeno and T. Norioka, Dispersion of Fullerene in Neat Synthesized Liquid-state Oligo(p-phenyleneethynylene)s, Chem. Lett., 2014, 43, 1770–1772 CrossRef CAS.
- S. S. Babu, Paradigms shift when solvent-less fluids come into play, Phys. Chem. Chem. Phys., 2015, 17, 3950–3953 RSC.
- S. Ghosh, V. K. Praveen and A. Ajayaghosh, The Chemistry and Applications of π-Gels, Annu. Rev. Mater. Res., 2016, 46, 235–262 CrossRef CAS.
- F. Bella, S. Galliano, C. Gerbaldi and G. Viscardi, Cobalt-Based Electrolytes for Dye-Sensitized Solar Cells: Recent Advances towards Stable Devices, Energies, 2016, 9, 384 CrossRef.
- A. Bourlinos, E. Giannelis, Q. Zhang, L. Archer, G. Floudas and G. Fytas, Surface-functionalized nanoparticles with liquid-like behavior: The role of the constituent components, Eur. Phys. J. E, 2006, 20, 109–117 CrossRef CAS PubMed.
- H. Li, J. Choi and T. Nakanishi, Optoelectronic Functional Materials Based on Alkylated-π Molecules: Self-Assembled Architectures and Nonassembled Liquids, Langmuir, 2013, 29, 5394–5406 CrossRef CAS PubMed.
- S.-Y. Gu, X.-F. Gao and Y.-H. Zhang, Synthesis and characterization of solvent-free ionic molybdenum disulphide (MoS2) nanofluids, Mater. Chem. Phys., 2015, 149, 587–593 CrossRef.
- T. Michinobu, K. Okoshi, Y. Murakami, K. Shigehara, K. Ariga and T. Nakanishi, Structural requirements for producing solvent-free room temperature liquid fullerenes, Langmuir, 2013, 29, 5337–5344 CrossRef CAS PubMed.
- T. Michinobu, T. Nakanishi, J. P. Hill, M. Funahashi and K. Ariga, Room Temperature Liquid Fullerenes: An Uncommon Morphology of C60 Derivatives, J. Am. Chem. Soc., 2006, 128, 10384–10385 CrossRef CAS PubMed.
- Y. PY and Z. YP, The Synthesis of Solvent-Free TiO2 Nanofluids through Surface Modification, Soft Nanosci. Lett., 2011, 2, 45–49 Search PubMed.
- A. B. Bourlinos, A. Stassinopoulos, D. Anglos, R. Herrera, S. H. Anastasiadis, D. Petridis and E. P. Giannelis, Functionalized ZnO nanoparticles with liquid like behavior and their photoluminescence properties, Small, 2006, 2, 513–516 CrossRef CAS PubMed.
- N. Agnieszka, G. Dorota and T. Daniel, Meso-Substituted Liquid Porphyrins, Chem. – Asian J., 2010, 5, 904–909 CrossRef PubMed.
- S. Yang, Y. Tan, X. Yin, S. Chen, D. Chen, L. Wang, Y. Zhou and C. Xiong, Preparation and characterization of monodisperse solvent-free silica nanofluids, J. Dispersion Sci. Technol., 2017, 38, 425–431 CrossRef CAS.
- Y. Zheng, J. Zhang, L. Lan, P. Yu, R. Rodriguez, R. Herrera, D. Wang and E. P. Giannelis, Preparation of Solvent-Free Gold Nanofluids with Facile Self-Assembly Technique, ChemPlusChem, 2010, 11, 61–64 CAS.
- M. Hagen, E. Meijer, G. Mooij and D. Frenkel, Does C60 have a liquid phase?, Nature, 1993, 365, 425–426 CrossRef CAS.
- F. Lu and T. Nakanishi, Alkyl-π engineering in state control toward versatile optoelectronic soft materials, Sci. Technol. Adv. Mater., 2015, 16, 014805 CrossRef PubMed.
- M. J. Hollamby and T. Nakanishi, The power of branched chains: optimising functional molecular materials, J. Mater. Chem. C, 2013, 1, 6178–6183 RSC.
- A. Çelik, A. Demir and Y. Bozkurt, Recent Developments in Flexible Solar cells, Nonlinear Opt., Quantum Opt., 2008, 38, 175–190 Search PubMed.
- Y. Zhou, J. Pei, Q. Dong, X. Sun, Y. Liu and W. Tian, Donor−Acceptor Molecule as the Acceptor for Polymer-Based Bulk Heterojunction Solar Cells, J. Phys. Chem. C, 2009, 113, 7882–7886 CrossRef CAS.
- G. Conibeer, Third-generation photovoltaics, Mater. Today, 2007, 10, 42–50 CrossRef CAS.
- M. Grätzel, Dye-Sensitized Solar Cells, J. Photochem. Photobiol., C, 2003, 4, 145–153 CrossRef.
- P. Choubey, A. Oudhia and R. Dewangan, A review: Solar cell current scenario and future trends, Recent Res. Sci. Technol., 2012, 4, 99–101 Search PubMed.
- S. S. Babu, M. J. Hollamby, J. Aimi, H. Ozawa, A. Saeki, S. Seki, K. Kobayashi, K. Hagiwara, M. Yoshizawa and H. Möhwald, Nonvolatile liquid anthracenes for facile full-colour luminescence tuning at single blue-light excitation, Nat. Commun., 2013, 4, 1969 CrossRef PubMed.
- S. A. Rice and J. Jortner, Do Exciton States Exist in the Liquid Phase?, J. Chem. Phys., 1966, 44, 4470–4472 CrossRef CAS.
- A. Ghosh and T. Nakanishi, Frontiers of solvent-free functional molecular liquids, Chem. Commun., 2017, 53, 10344–10357 RSC.
- T. Meng, K.-H. Young, D. F. Wong and J. Nei, Ionic Liquid-Based Non-Aqueous Electrolytes for Nickel/Metal Hydride Batteries, Batteries, 2017, 3, 4–15 CrossRef.
- J.-C. Ribierre, T. Aoyama, T. Muto, Y. Imase and T. Wada, Charge transport properties in liquid carbazole, Org. Electron., 2008, 9, 396–400 CrossRef CAS.
- K. Kubota, S. Hirata, Y. Shibano, O. Hirata, M. Yahiro and C. Adachi, Liquid carbazole substituted with a poly(ethylene oxide) group and its application for liquid organic light-emitting diodes, Chem. Lett., 2012, 41, 934–936 CrossRef CAS.
- J.-C. Ribierre, L. Zhao, M. Inoue, P.-O. Schwartz, J.-H. Kim, K. Yoshida, A. S. Sandanayaka, H. Nakanotani, L. Mager and S. Méry, Low threshold amplified spontaneous emission and ambipolar charge transport in non-volatile liquid fluorene derivatives, Chem. Commun., 2016, 52, 3103–3106 RSC.
- B. A. Kamino, T. P. Bender and R. A. Klenkler, Hole Mobility of a Liquid Organic Semiconductor, J. Phys. Chem. Lett., 2012, 3, 1002–1006 CrossRef CAS PubMed.
-
J. B. Hasted, Aqueous dielectrics, Chapman and Hall, 1973 Search PubMed.
- T. Grancha, J. s. Ferrando-Soria, J. Cano, P. Amorós, B. Seoane, J. Gascon, M. Bazaga-García, E. R. Losilla, A. Cabeza and D. Armentano, Insights into the Dynamics of Grotthuss Mechanism in a Proton-Conducting Chiral bioMOF, Chem. Mater., 2016, 28, 4608–4615 CrossRef CAS.
- S. Tan, Z. Zhao, C. Wang and Y. Wu, A thiolate/disulfide liquid crystalline electrolyte for dye-sensitized solar cells: Promotion of the Grotthuss-type charge transport through lamellar nanostructures, Electrochim. Acta, 2018, 288, 165–172 CrossRef CAS.
- W. Xu and C. A. Angell, Solvent-free electrolytes with aqueous solution-like conductivities, Science, 2003, 302, 422–425 CrossRef CAS PubMed.
- S. García-Garabal, J. Vila, E. Rilo, M. Domínguez-Pérez, L. Segade, E. Tojo, P. Verdía, L. Varela and O. Cabeza, Transport Properties for 1-Ethyl-3-methylimidazolium n-alkyl Sulphates: Possible Evidence of Grothuss Mechanism, Electrochim. Acta, 2017, 231, 94–102 CrossRef.
- B. O'regan and M. Grätzel, A low-cost, high-efficiency solar cell based on dye-sensitized colloidal TiO2 films, Nature, 1991, 353, 737 CrossRef.
- J. Gong, K. Sumathy, Q. Qiao and Z. Zhou, Review on dye-sensitized solar cells (DSSCs): Advanced techniques and research trends, Renewable Sustainable Energy Rev., 2017, 68, 234–246 CrossRef CAS.
-
K. Surana and R. M. Mehra, Quantum Dot Sensitized Solar Cells (QDSSCs), Nanomaterials and Their Applications, Springer, Singapore, 2018, pp. 315–321 Search PubMed.
- I. Obotowo, I. Obot and U. Ekpe, Organic sensitizers for dye-sensitized solar cell (DSSC): Properties from computation, progress and future perspectives, J. Mol. Struct., 2016, 1122, 80–87 CrossRef CAS.
- A. Zrenner, A close look on single quantum dots, J. Chem. Phys., 2000, 112, 7790–7798 CrossRef CAS.
- P. Kambhampati, Unraveling the Structure and Dynamics of Excitons in Semiconductor Quantum Dots, Acc. Chem. Res., 2010, 44, 1–13 CrossRef PubMed.
- M. Cheng, X. Yang, F. Zhang, J. Zhao and L. Sun, Tuning the HOMO and LUMO Energy Levels of Organic Dyes with N-Carboxomethylpyridinium as Acceptor To Optimize the Efficiency of Dye-Sensitized Solar Cells, J. Phys. Chem. C, 2013, 117, 9076–9083 CrossRef CAS.
- S. Nakade, T. Kanzaki, W. Kubo, T. Kitamura, Y. Wada and S. Yanagida, Role of Electrolytes on Charge Recombination in Dye-Sensitized TiO2 Solar Cell (1): The Case of Solar Cells Using the I−/I3− Redox Couple, J. Phys. Chem. B, 2005, 109, 3480–3487 CrossRef CAS PubMed.
- B. C. O'Regan, I. López-Duarte, M. V. Martínez-Díaz, A. Forneli, J. Albero, A. Morandeira, E. Palomares, T. Torres and J. R. Durrant, Catalysis of Recombination and Its Limitation on Open Circuit Voltage for Dye Sensitized Photovoltaic Cells Using Phthalocyanine Dyes, J. Am. Chem. Soc., 2008, 130, 2906–2907 CrossRef PubMed.
- X. Liu, J. Fang, Y. Liu and T. Lin, Progress in nanostructured photoanodes for dye-sensitized solar cells, Front. Mater. Sci., 2016, 10, 225–237 CrossRef.
- R. Mori, T. Ueta, K. Sakai, Y. Niida, Y. Koshiba, L. Lei, K. Nakamae and Y. Ueda, Organic solvent based TiO2 dispersion paste for dye-sensitized solar cells prepared by industrial production level procedure, J. Mater. Sci., 2011, 46, 1341–1350 CrossRef CAS.
- G. Chen, L. Wang, Y. Zou, X. Sheng, H. Liu, X. Pi, D. Yang and CdSe, Quantum Dots Sensitized Mesoporous TiO2 Solar Cells with CuSCN as Solid-State Electrolyte, J. Nanomater., 2011, 2011, 40 Search PubMed.
- J. T. Margraf, A. s. Ruland, V. Sgobba, D. M. Guldi and T. Clark, Quantum-dot-sensitized solar cells: Understanding linker molecules through theory and experiment, Langmuir, 2013, 29, 2434–2438 CrossRef CAS PubMed.
- Y.-L. Lee, C.-L. Chen, L.-W. Chong, C.-H. Chen, Y.-F. Liu and C.-F. Chi, A platinum counter electrode with high electrochemical activity and high transparency for dye-sensitized solar cells, Electrochem. Commun., 2010, 12, 1662–1665 CrossRef CAS.
- C. H. Yoon, R. Vittal, J. Lee, W.-S. Chae and K.-J. Kim, Enhanced performance of a dye-sensitized solar cell with an electrodeposited-platinum counter electrode, Electrochim. Acta, 2008, 53, 2890–2896 CrossRef CAS.
- M.-H. Yeh, C.-P. Lee, C.-Y. Chou, L.-Y. Lin, H.-Y. Wei, C.-W. Chu, R. Vittal and K.-C. Ho, Conducting polymer-based counter electrode for a quantum-dot-sensitized solar cell (QDSSC) with a polysulfide electrolyte, Electrochim. Acta, 2011, 57, 277–284 CrossRef CAS.
- W. Hong, Y. Xu, G. Lu, C. Li and G. Shi, Transparent graphene/PEDOT–PSS composite films as counter electrodes of dye-sensitized solar cells, Electrochem. Commun., 2008, 10, 1555–1558 CrossRef CAS.
- J. Wu, Q. Li, L. Fan, Z. Lan, P. Li, J. Lin and S. Hao, High-performance polypyrrole nanoparticles counter electrode for dye-sensitized solar cells, J. Power Sources, 2008, 181, 172–176 CrossRef CAS.
- Y. Xiao, J. Wu, G. Yue, J. Lin, M. Huang and Z. Lan, Low temperature preparation of a high performance Pt/SWCNT counter electrode for flexible dye-sensitized solar cells, Electrochim. Acta, 2011, 56, 8545–8550 CrossRef CAS.
- X. Xu, D. Huang, K. Cao, M. Wang, S. M. Zakeeruddin and M. Grätzel, Electrochemically reduced graphene oxide multilayer films as efficient counter electrode for dye-sensitized solar cells, Sci. Rep., 2013, 3, 1489 CrossRef PubMed.
- C.-P. Lee, C.-T. Li and K.-C. Ho, Use of organic materials in dye-sensitized solar cells, Mater. Today, 2017, 20, 267–283 CrossRef CAS.
- S. Yun, Y. Liu, T. Zhang and S. Ahmad, Recent advances in alternative counter electrode materials for Co-mediated dye-sensitized solar cells, Nanoscale, 2015, 7, 11877–11893 RSC.
- J. Burschka, V. Brault, S. Ahmad, L. Breau, M. K. Nazeeruddin, B. Marsan, S. M. Zakeeruddin and M. Grätzel, Influence of the counter electrode on the photovoltaic performance of dye-sensitized solar cells using a disulfide/thiolate redox electrolyte, Energy Environ. Sci., 2012, 5, 6089–6097 RSC.
- Y. Shibata, T. Kato, T. Kado, R. Shiratuchi, W. Takashima, K. Kaneto and S. Hayase, Quasi-solid dye sensitised solar cells filled with ionic liquid—increase in efficiencies by specific interaction between conductive polymers and gelators, Chem. Commun., 2003, 2730–2731 RSC.
- G. Boschloo and A. Hagfeldt, Characteristics of the Iodide/Triiodide Redox Mediator in Dye-Sensitized Solar Cells, Acc. Chem. Res., 2009, 42, 1819–1826 CrossRef CAS PubMed.
- Y.-L. Lee and C.-H. Chang, Efficient polysulfide electrolyte for CdS quantum dot-sensitized solar cells, J. Power Sources, 2008, 185, 584–588 CrossRef CAS.
- J.-H. Yum, E. Baranoff, F. Kessler, T. Moehl, S. Ahmad, T. Bessho, A. Marchioro, E. Ghadiri, J.-E. Moser and C. Yi, A cobalt complex redox shuttle for dye-sensitized solar cells with high open-circuit potentials, Nat. Commun., 2012, 3, 631 CrossRef PubMed.
- A. Yella, H.-W. Lee, H. N. Tsao, C. Yi, A. K. Chandiran, M. K. Nazeeruddin, E. W.-G. Diau, C.-Y. Yeh, S. M. Zakeeruddin and M. Grätzel, Porphyrin-sensitized solar cells with cobalt (II/III)-based redox electrolyte exceed 12 percent efficiency, Science, 2011, 334, 629–634 CrossRef CAS PubMed.
- J. Wu, Z. Lan, J. Lin, M. Huang, Y. Huang, L. Fan and G. Luo, Electrolytes in Dye-Sensitized Solar Cells, Chem. Rev., 2015, 115, 2136–2173 CrossRef CAS PubMed.
- P. Chawla and M. Tripathi, Nanocomposite Polymer Electrolyte for Enhancement in Stability of Betacyanin Dye Sensitized Solar Cells, Electrochem. Solid-State Lett., 2015, 4, Q21–Q23 CrossRef CAS.
- S. Yun, J. N. Freitas, A. F. Nogueira, Y. Wang, S. Ahmad and Z.-S. Wang, Dye-sensitized solar cells employing polymers, Prog. Polym. Sci., 2016, 59, 1–40 CrossRef CAS.
- C. L. Boldrini, N. Manfredi, F. M. Perna, V. Trifiletti, V. Capriati and A. Abbotto, Dye-Sensitized Solar Cells that use an Aqueous Choline Chloride-Based Deep Eutectic Solvent as Effective Electrolyte Solution, Energy Technol., 2017, 5, 345–353 CrossRef CAS.
- S.-H. Park, I. Y. Song, J. Lim, Y. S. Kwon, J. Choi, S. Song, J.-R. Lee and T. Park, A novel quasi-solid state dye-sensitized solar cell fabricated using a multifunctional network polymer membrane electrolyte, Energy Environ. Sci., 2013, 6, 1559–1564 RSC.
- J. Gong, K. Sumathy and J. Liang, Polymer electrolyte based on polyethylene glycol for quasi-solid state dye sensitized solar cells, Renewable Energy, 2012, 39, 419–423 CrossRef CAS.
- H. Yang, M. Huang, J. Wu, Z. Lan, S. Hao and J. Lin, The polymer gel electrolyte based on poly(methyl methacrylate) and its application in quasi-solid-state dye-sensitized solar cells, Mater. Chem. Phys., 2008, 110, 38–42 CrossRef CAS.
- A. Arof, I. Noor, M. Buraidah, T. Bandara, M. Careem, I. Albinsson and B.-E. Mellander, Polyacrylonitrile Gel Polymer Electrolyte Based Dye Sensitized Solar Cells for a Prototype Solar Panel, Electrochim. Acta, 2017, 251, 223–234 CrossRef CAS.
-
M. G. Kang, N.-G. Park, K.-M. Kim, K. S. Ryu, S. H. Chang and K.-J. Kim, Highly efficient polymer gel electrolytes for dye-sensitized solar cells, Proc. 3rd World Conference on Photovoltaic Energy Conversion, 2003 Search PubMed.
- N. Mohmeyer, P. Wang, H.-W. Schmidt, S. M. Zakeeruddin and M. Grätzel, Quasi-solid-state dye sensitized solar cells with 1,3:2,4-di-O-benzylidene-D-sorbitol derivatives as low molecular weight organic gelators, J. Mater. Chem., 2004, 14, 1905–1909 RSC.
- L. Tao, Z. Huo, S. Dai, Y. Ding, J. Zhu, C. Zhang, B. Zhang, J. Yao, M. K. Nazeeruddin and M. Grätzel, Stable Quasi-Solid-State Dye-Sensitized Solar Cells Using Novel Low Molecular Mass Organogelators and Room-Temperature Molten Salts, J. Phys. Chem. C, 2014, 118, 16718–16726 CrossRef CAS.
- J. Wu, Z. Lan, S. Hao, P. Li, J. Lin, M. Huang, L. Fang and Y. Huang, Progress on the electrolytes for dye-sensitized solar cells, Pure Appl. Chem., 2008, 80, 2241–2258 CAS.
- Z. Seidalilir, R. Malekfar, H.-P. Wu, J.-W. Shiu and E. W.-G. Diau, High-Performance and Stable Gel-State Dye-Sensitized Solar Cells Using Anodic TiO2 Nanotube Arrays and Polymer-Based Gel Electrolytes, ACS Appl. Mater. Interfaces, 2015, 7, 12731–12739 CrossRef CAS PubMed.
- S.-Y. Shen, R.-X. Dong, P.-T. Shih, V. Ramamurthy, J.-J. Lin and K.-C. Ho, Novel Polymer Gel Electrolyte with Organic Solvents for Quasi-Solid-State Dye-Sensitized Solar Cells, ACS
Appl. Mater. Interfaces, 2014, 6, 18489–18496 CrossRef CAS PubMed.
- T. V. Burova, N. V. Grinberg, A. S. Dubovik, E. A. Olenichenko, V. N. Orlov and V. Y. Grinberg, Interpolyelectrolyte complexes of lysozyme with short poly[di(carboxylatophenoxy)phosphazene]. Binding energetics and protein conformational stability, Polymer, 2017, 108, 97–104 CrossRef CAS.
- C. Fiedler, B. Luerssen, B. Lucht and J. Janek, Synthesis and characterization of polyphosphazene electrolytes including cyclic ether side groups, J. Power Sources, 2018, 384, 165–171 CrossRef CAS.
- A. J. Pearse, T. E. Schmitt, E. J. Fuller, F. El-Gabaly, C.-F. Lin, K. Gerasopoulos, A. C. Kozen, A. A. Talin, G. Rubloff and K. E. Gregorczyk, Nanoscale Solid State Batteries Enabled by Thermal Atomic Layer Deposition of a Lithium Polyphosphazene Solid State Electrolyte, Chem. Mater., 2017, 29, 3740–3753 CrossRef CAS.
- S.-H. A. Lee, A.-M. S. Jackson, A. Hess, S.-T. Fei, S. M. Pursel, J. Basham, C. A. Grimes, M. W. Horn, H. R. Allcock and T. E. Mallouk, Influence of Different Iodide Salts on the Performance of Dye-Sensitized Solar Cells Containing Phosphazene-Based Nonvolatile Electrolytes, J. Phys. Chem. C, 2010, 114, 15234–15242 CrossRef CAS.
- N. Manfredi, A. Bianchi, V. Causin, R. Ruffo, R. Simonutti and A. Abbotto, Electrolytes for quasi solid-state dye-sensitized solar cells based on block copolymers, J. Polym. Sci., Part A: Polym. Chem., 2014, 52, 719–727 CrossRef CAS.
- T. M. Bandara, H. Fernando, M. Furlani, I. Albinsson, M. Dissanayake and B.-E. Mellander, Performance enhancers for gel polymer electrolytes based on LiI and RbI for quasi-solid-state dye sensitized solar cells, RSC Adv., 2016, 6, 103683–103691 RSC.
- L.-Y. Chang, C.-P. Lee, C.-T. Li, M.-H. Yeh, K.-C. Ho and J.-J. Lin, Synthesis of a novel amphiphilic polymeric ionic liquid and its application in quasi-solid-state dye-sensitized solar cells, J. Mater. Chem. A, 2014, 2, 20814–20822 RSC.
- K. Prabakaran, A. K. Palai, S. Mohanty and S. K. Nayak, Aligned carbon nanotube/polymer hybrid electrolytes for high performance dye sensitized solar cell applications, RSC Adv., 2015, 5, 66563–66574 RSC.
- S. M. Zakeeruddin and M. Grätzel, Solvent-Free Ionic Liquid Electrolytes for Mesoscopic Dye-Sensitized Solar Cells, Adv. Funct. Mater., 2009, 19, 2187–2202 CrossRef CAS.
-
C.-P. Lee, P.-Y. Chen and K.-C. Ho, Ionic Liquid Based Electrolytes for Dye-Sensitized Solar Cells, in Ionic liquids: Theory, properties, new approaches, InTech, 2011 Search PubMed.
-
C.-P. Lee, T.-C. Chu, L.-Y. Chang, J.-J. Lin and K.-C. Ho, Solid-State Ionic Liquid Based Electrolytes for Dye-Sensitized Solar Cells, in Ionic Liquids-New Aspects for the Future, InTech, 2013 Search PubMed.
- P. Wang, S. M. Zakeeruddin, I. Exnar and M. Grätzel, High efficiency dye-sensitized nanocrystalline solar cells based on ionic liquid polymer gel electrolyte, Chem. Commun., 2002, 2972–2973 RSC.
- K. B. Aribia, T. Moehl, S. M. Zakeeruddin and M. Grätzel, Tridentate cobalt complexes as alternative redox couples for high-efficiency dye-sensitized solar cells, Chem. Sci., 2013, 4, 454–459 RSC.
- F. Zhu, P. Zhang, X. Wu, L. Fu, J. Zhang and D. Xu, The Origin of Higher Open-Circuit Voltage in Zn-Doped TiO2 Nanoparticle-Based Dye-Sensitized Solar Cells, ChemPhysChem, 2012, 13, 3731–3737 CrossRef CAS PubMed.
- K. Murakoshi, G. Kano, Y. Wada, S. Yanagida, H. Miyazaki, M. Matsumoto and S. Murasawa, Importance of binding states between photosensitizing molecules and the TiO2 surface for efficiency in a dye-sensitized solar cell, J. Electroanal. Chem., 1995, 396, 27–34 CrossRef.
- J. Li, W. Wu, J. Yang, J. Tang, Y. Long and J. Hua, Effect of chenodeoxycholic acid (CDCA) additive on phenothiazine dyes sensitized photovoltaic performance, Sci. China: Chem., 2011, 54, 699–706 CAS.
- A. K. Biswas, A. Das and B. Ganguly, The influence of noncovalent interactions in metal-free organic dye molecules to augment the efficiency of dye sensitized solar cells: A computational study, Int. J. Quantum Chem., 2017, 117, e25415 CrossRef.
- N. Koumura, Z.-S. Wang, S. Mori, M. Miyashita, E. Suzuki and K. Hara, Alkyl-functionalized organic dyes for efficient molecular photovoltaics, J. Am. Chem. Soc., 2006, 128, 14256–14257 CrossRef CAS PubMed.
- Y. Tan, Y. Zheng, N. Wang and A. Zhang, Controlling the Properties of Solvent-free Fe3O4 Nanofluids by Corona Structure, Nano-Micro Lett., 2012, 4, 208–214 CrossRef CAS.
- K. Zhu, T. B. Vinzant, N. R. Neale and A. J. Frank, Removing structural disorder from oriented TiO2 nanotube arrays: reducing the dimensionality of transport and recombination in dye-sensitized solar cells, Nano Lett., 2007, 7, 3739–3746 CrossRef CAS PubMed.
- P.-Y. Yu, Y.-P. Zheng and L. Lan, The Synthesis of Solvent-Free TiO2 Nanofluids through Surface Modification, Soft Nanosci. Lett., 2011, 1, 45–49 Search PubMed.
- X. Wu, X. Zhou, Q. Wu and W. Yan, Facile fabrication of thermal-control ionic liquid compound based on undecatungstophosphoindic polyoxometalate with fast ionic conductivity, New J. Chem., 2016, 40, 7923–7927 RSC.
- J. Ding, C. Yu, N. Yuan, Y. Liu and Y. Fan, Electrical conductivity of waterborne polyurethane/graphene composites prepared by solution mixing, J. Compos. Mater., 2012, 46, 747–752 CrossRef CAS.
- X. Du, I. Skachko, A. Barker and E. Y. Andrei, Approaching ballistic transport in suspended graphene, Nat. Nanotechnol., 2008, 3, 491–495 CrossRef CAS PubMed.
- I. Martini, J. H. Hodak and G. V. Hartland, Effect of Structure on Electron Transfer Reactions between Anthracene Dyes and TiO2 Nanoparticles, J. Phys. Chem. B, 1998, 102, 9508–9517 CrossRef CAS.
- R. Ernstorfer, L. Gundlach, S. Felber, W. Storck, R. Eichberger and F. Willig, Role of Molecular Anchor Groups in Molecule-to-Semiconductor Electron Transfer, J. Phys. Chem. B, 2006, 110, 25383–25391 CrossRef CAS PubMed.
- P. Persson, M. J. Lundqvist, R. Ernstorfer, W. Goddard and F. Willig, Quantum Chemical Calculations of the Influence of Anchor-Cum-Spacer Groups on Femtosecond Electron Transfer Times in Dye-Sensitized Semiconductor Nanocrystals, J. Chem. Theory Comput., 2006, 2, 441–451 CrossRef CAS PubMed.
- E. Ronca, M. Pastore, L. Belpassi, F. Tarantelli and F. De Angelis, Influence of the dye molecular structure on the TiO2 conduction band in dye-sensitized solar cells: disentangling charge transfer and electrostatic effects, Energy Environ. Sci., 2013, 6, 183–193 RSC.
- O. Bagheri, H. Dehghani and M. Afrooz, Pyridine derivatives; new efficient additives in bromide/tribromide electrolyte for dye sensitized solar cells, RSc. Adv., 2015, 105, 86191–86198 RSC.
- A. Hagfeldt, G. Boschloo, L. Sun, L. Kloo and H. Pettersson, Dye-Sensitized Solar Cells, Chem. Rev., 2010, 110, 6595–6663 CrossRef CAS PubMed.
- S. G. Hashmi, M. Ozkan, J. Halme, K. D. Misic, S. M. Zakeeruddin, J. Paltakari, M. Grätzel and P. D. Lund, High performance dye-sensitized solar cells with inkjet printed ionic liquid electrolyte, Nano Energy, 2015, 17, 206–215 CrossRef CAS.
- K. Miettunen, J. Vapaavuori, A. Poskela, A. Tiihonen and P. D. Lund, Recent progress in flexible dye solar cells, Wiley Interdiscip. Rev.: Energy Environ., 2018, 7, e302 CrossRef PubMed.
|
This journal is © The Royal Society of Chemistry and Owner Societies 2019 |
Click here to see how this site uses Cookies. View our privacy policy here.