Modification of BiVO4/WO3 composite photoelectrodes with Al2O3via chemical vapor deposition for highly efficient oxidative H2O2 production from H2O†
Received
14th February 2018
, Accepted 14th May 2018
First published on 4th June 2018
Abstract
The modification of a BiVO4/WO3 photoelectrode with Al2O3 by a chemical vapor deposition (CVD) method significantly improved the faradaic efficiency for H2O2 production (FE(H2O2)) in the photoelectrochemical oxidation of H2O. The faradaic efficiency after passing 0.9 C (after photoirradiation for 15 min using simulated solar light) was 80% using a 2.0 M KHCO3 aqueous solution as an electrolyte. The initial FE(H2O2) (<0.02 C) was almost 100% on the Al2O3/BiVO4/WO3 photoelectrode. The thin Al2O3 layer prevents the oxidative decomposition of the produced H2O2 into O2. The applied bias photon to current efficiency (ABPE) was 2.57% based on H2O2 and O2 production on the photoanode and H2 production on a Pt cathode. Solar light driven simultaneous H2O2 production at the anode for H2O oxidation and the cathode for O2 reduction was also achieved by combining a noble-metal-free biomass-derived carbon cathode without applying any external bias.
1 Introduction
Since the Honda–Fujishima effect was reported, water splitting into H2 and O2 using a photoelectrochemical cell has been identified as a promising technique for an effective solar energy conversion and storage system.1–3 Such systems enable water-splitting at a lower external bias than the thermodynamic value (ΔEo = 1.23 V) by utilizing solar energy effectively. The water splitting into H2 and O2 on the cathode and photoanode is presented in eqn (1)–(3), respectively.
Cathode:
| 2H+ + 2e− → H2 (Eo(H+/H2) = 0.00 V vs. RHE) | (1) |
Photoanode:
| 2H2O → O2 + 4H+ + 4e− (Eo(O2/H2O) = +1.23 V vs. RHE) | (2) |
2(eqn (1)) + (eqn (2)):
It is favorable to utilize metal oxide films such as WO3,4–6 BiVO4,7,8 and Fe2O3
9 in photoelectrochemical reactions, having absorption in the visible light region. In particular, BiVO4 is widely known as one of the most promising metal oxide materials for H2O oxidation. It is composed of inexpensive metals, and absorbs a wide range of visible light (∼520 nm) corresponding to the narrow band gap (ca. 2.4 eV).10 The oxidation products of H2O such as O2 have been paid little attention in most photoelectrochemical water-splitting systems, while reduction products such as H2 have been regarded as new clean fuels.11 On the other hand, some researchers reported oxidative production of high value-added oxidants such as H2O2,12–17 S2O82−,18,19 ClO−,20etc. on the photoanode with simultaneous H2 production on the cathode. Additionally, it has been revealed that 2-electon oxidation of H2O to H2O2 (eqn (4)) might be concurrent with 4-electron oxidation to O2.21
| 2H2O → H2O2 + 2H+ + 2e− (Eo(H2O2/H2O) = +1.77 V vs. RHE) | (4) |
H2O2 has attracted considerable attention as a versatile and clean redox reagent for selective organic conversion and environmental purification, and as a bleaching and cleaning agent and an energy source for H2O2 fuel cells.22–24 Therefore, the development of water-splitting systems for H2 and H2O2 production (eqn (5)) would enable us to broaden the use of photoelectrode systems in various fields, while conventional water-splitting systems for H2 and O2 production (eqn (3)) do not make good use of the photo-induced oxidation power.
Eqn (1) + eqn (4):
| 2H2O → H2O2 + H2 (2-electron process) | (5) |
A small amount of H2O2 was sometimes detected in photocatalytic or photoelectrochemical H2O oxidation, indicating that H2O2 can be an intermediate species of O2 production from H2O via 4-electron oxidation. Inevitable oxidative decomposition of the produced H2O2 may disable the system for oxidative production and accumulation of H2O2, that is, the produced H2O2 might be easily decomposed into O2 by generated holes (h+) in accordance with eqn (6). Moreover, the H2O2 production from H2O via a 2-electron process (eqn (4)) should compete with the O2 production from H2O via a 4-electron process (eqn (2)), which is more preferential than H2O2 production in consideration of their redox potentials. Hence, the development of tailor-made photoanodes that can prevent the oxidative decomposition of the produced H2O2 into O2 should accelerate the photoelectrochemical H2O2 production and accumulation from H2O.
| H2O2 → O2 + 2H+ + 2e− (Eo(O2/H2O2) = +0.68 V vs. RHE) | (6) |
In our previous work, we found a unique phenomenon that the presence of the bicarbonate anion (HCO3−) in the electrolyte solution enabled us to achieve a significant improvement in the faradaic efficiency for oxidative H2O2 generation (FE(H2O2)) in the photoelectrochemical reaction using the BiVO4/WO3 photoelectrode.13–15 It was expected that the percarbonate/bicarbonate couple played an important role in effective H2O2 production and accumulation.25 Our original BiVO4/WO3 multilayer photoelectrode is composed of BiVO4 and WO3, which worked as a photocatalyst layer (light absorption layer) and an effective electron transport layer, respectively.26,27 It is also reported that the BiVO4 surface was suitable for H2O2 production in bicarbonate solution under dark conditions.16,28 The value of the applied bias photon to current efficiency (ABPE) under simulated solar light irradiation (1 Sun: AM 1.5G, 100 mW cm−2) was 2.2% by considering the production of H2O2, O2 and H2. Moreover, we have recently reported that the FE(H2O2) was drastically improved from 54% to 79% by surface modification of the BiVO4/WO3 photoelectrode with a porous and thick Al2O3 layer prepared via a metal–organic decomposition (MOD) method (hereinafter, Al2O3(MOD)).15 However, the modification obviously deteriorated the photocurrent–potential property and caused a decrease in the ABPE value from 2.2% to 1.5%. In the present study, we investigate the modification of the BiVO4/WO3 photoelectrode with a thin Al2O3 layer produced by the chemical vapor deposition method (Al2O3(CVDn) where n = the number of CVD cycles) with the aim of achieving high FE(H2O2) and ABPE values.
When BiVO4 was used as a photoanode, it was necessary to apply an external bias for inducing H2 production on the counter electrode because the potential of the bottom of the conduction band (ca. +0.02 V vs. RHE)29 was more positive than the standard potential for H2 production (H+/H2: 0.00 V vs. RHE). On the other hand, the standard potential for reductive H2O2 production from O2 (O2/H2O2: +0.68 V vs. RHE) was more positive than that for H2 production and the bottom of its conduction band. Therefore, a BiVO4 photoanode may cause H2O2 production on the counter electrode without applying an external bias. In fact, we reported that a photoelectrochemical cell composed of an Au cathode and the BiVO4/WO3 photoanode achieved H2O2 production without applying any external bias under simulated solar light irradiation, as shown in eqn (7)via the 2-electron process.14
Eqn (4) − eqn (6):
| 2H2O + O2 → 2H2O2 (2-electron process) | (7) |
However, it is preferable not to use expensive noble metals such as Au. Recently, we have reported that carbon-paper cathodes composed of a biomass-derived W- or Mo-carbide/nitride composite on carbon paper showed high performance for electrochemical H2 evolution at pH 1.30,31 Here we investigate the activity of the reductive H2O2 production from O2 in a bicarbonate solution using these biomass-derived electrocatalysts over carbon-based cathodes. Moreover, a new photoelectrochemical cell composed of an Al2O3(CVD5)/BiVO4/WO3 photoanode and a W-based cathode successfully produced solar light driven H2O2 on both electrodes without applying any external bias or using noble metals.
2 Experimental section
2.1 Preparation of electrodes
2.1.1 BiVO4/WO3 and Al2O3/BiVO4/WO3 photoelectrodes.
The BiVO4/WO3 photoelectrode was prepared on a conductive glass substrate (FTO: F-doped SnO2) by the spin-coating method as we have previously reported.13 The precursor of the WO3 layer was loaded by spin-coating (1000 rpm, 15 s, 200 μL per 12 cm2) on FTO and then calcined at 773 K for 30 min. The spin-coating of the WO3 layer was repeated twice using N,N-dimethylformamide (DMF) solutions of tungsten hexachloride (WCl6) adjusted to 504 mM and 252 mM, respectively. The BiVO4 layer was also fabricated by spin-coating (500 rpm, 15 s, 400 μL per 12 cm2) on the WO3 layer and calcined at 773 K for 30 min. The BiVO4 precursor solution, adjusted to 100 mM, was prepared by dissolving a bismuth oxide (BiO1.5) solution (Symmetrix Co., USA), and a vanadium oxide (VO2.5) solution (Symmetrix Co., USA) in butyl acetate with a Bi/V molar ratio of 1.0. A 10 wt% butyl acetate solution of ethyl cellulose was added to the BiVO4 solution as a thickening agent with a (BiVO4 solution)/(ethyl cellulose solution) volume ratio of 1.5. The surface area of the photoanode was changed in order to evaluate the produced H2O2 accurately and to minimize the effect of successive H2O2 decomposition.
The BiVO4/WO3 photoelectrode was modified with Al2O3 by two methods: a conventional wet process using the MOD method15 and a dry process based on chemical vapor deposition (CVD). The surface modification by CVD was performed using a SAL3000Plus (SUGA Co., Japan). The temperature of the CVD unit was kept stable at 423 K, and vapor trimethylaluminum (TMA) and H2O were alternately introduced into the heated sample chamber at 30 s intervals per cycle. In the case of the MOD method, the optimized conditions in the previous work were used.15 The BiVO4/WO3 photoelectrode modified with Al2O3 using the CVD method and the MOD method is denoted as the Al2O3(CVDn)/BiVO4/WO3 (where, n = the number of CVD cycles) and the Al2O3(MOD)/BiVO4/WO3 photoelectrode, respectively. The prepared photoelectrodes were characterized by X-ray fluorescence (XRF, Rigaku, ZSX mini, measured in a vacuum with a wavelength dispersive spectrometer, analysis area: 3 cm2) with Pd–K or –L radiation, X-ray photoelectron spectroscopy (XPS, ULVAC, PHI) with Al-Kα radiation, scanning electron microscopy (SEM, HITACHI High-Technologies Co. S-4800), X-ray diffraction (XRD, PANalytical, IC Vario) with Cu-Kα, and UV-Vis spectroscopy (JASCO, V570).
2.1.2 Biomass-derived carbon cathodes (WSoy/GnP-CP, MoSoy-CP, and CB-CP).
Biomass-derived W- and Mo-based electrocatalysts (WSoy and MoSoy) were synthesized following the previous reports.30,31 WSoy/GnP, a tungsten carbide/nitride composite supported on graphene nanoplatelets (GnP), was prepared by a sintering process. A mixture of 100 mg of GnP (XG Sciences C-750) and 100 mg of ground soybean (Whole Food Market Inc., USA) was added to a 0.64 mM aqueous solution (50 mL) of ammonium tungstate tetrahydrate (Sigma-Aldrich Co., USA). The suspension was thoroughly dispersed by ultrasonication for 30 min and dried at 353 K overnight under ambient pressure in a heating oven. The dried powder was calcined at 1123 K in an Ar atmosphere for 2 h in a quartz tube furnace. MoSoy was synthesized by a similar method using mixtures of 100 mg of ammonium molybdate tetrahydrate (Sigma-Aldrich Co., USA) and 100 mg of soybean, and sintered at 1073 K for 2 h. A mixture of 1.5 mg (standard amount) of the electrocatalyst (WSoy/GnP, MoSoy, or carbon black (CB, VULCAN XC-72, CABOT Co., USA)) and 0.75 mg of a 20 wt% Nafion solution (Sigma-Aldrich Co., USA) was dispersed in 0.5 mL ethanol and loaded on one side of water-repellent treated carbon paper (CP, 1.0 cm2, TORAY, TGP-H-090).
2.2 Photoelectrochemical reaction for H2O2 production and accumulation
H2O2 production was performed using a two-chamber cell with a Nafion membrane (Nafion NRE-212, Sigma-Aldrich Co., USA) separating the Al2O3-modified BiVO4/WO3 photoanode (6 cm2) and a Pt-mesh electrode as a cathode and a quasi-reference electrode. An aqueous solution of KHCO3 was utilized as an electrolyte solution (35 mL each for the anode and cathode chambers), and CO2 gas was continuously bubbled into the solution to keep the pH value at 7.0–7.8 during the reaction. The solution was cooled in an ice bath (273–278 K) to prevent the thermal decomposition of the produced H2O2. A solar simulator (XES-151S, SAN-EI ELECTRIC Co.,) calibrated to AM 1.5G (1 SUN, 100 mW cm−2, JIS-A-class) was used as the light source. The anodic photocurrent was kept at 1.0 mA by using an electrochemical analyzer (ALS660E, BAS. Inc.). The amount of H2O2 produced was quantified by colorimetry using 0.1 M FeCl2 in 1.0 M HCl aqueous solution, as we reported previously.13 In addition, produced O2 in the gas and liquid phase was measured with an O2 meter (PyroScience, Firesting O2) in the sealed reaction cell. The faradaic efficiency for H2O2 production (FE(H2O2)) was calculated according to eqn (8). | FE(H2O2) = [amount of produced H2O2 (μmol)] × 100/[passed electrons (μmol)/2] | (8) |
2.3 Photoelectrochemical properties and calculation of applied bias photon-to-current conversion efficiency (ABPE)
The current–potential (I–V) curves of the fabricated photoelectrodes were measured using a three-electrode system composed of a photoanode (irradiation area: 0.28 cm2, with a white board behind the photoelectrode), a Pt wire cathode, and an Ag/AgCl reference electrode. The applied bias photon-to-current conversion efficiency (ABPE) for the H2O2 and O2 production on the photoanode and H2 production on the cathode was calculated using the photocurrent–potential properties and faradaic efficiencies of H2O2 and O2 after passing 0.9 C, as shown in eqn (9),32,33 | ABPE (%) = [Jopt × (1.77 V − Eopt)/Int] × FE(H2O2) + [Jopt × (1.23 V − Eopt)/Int] × FE(O2) | (9) |
where Jopt is the photocurrent density at Eopt, Eopt is the applied external voltage under optimal conditions between working and counter electrodes, Int is the intensity of incident simulated solar light (100 mW cm−2), and FE(H2O2) and FE(O2) are the faradaic efficiencies of H2O2 and O2, respectively. The standard potentials of H+/H2, O2/H2O and H2O2/H2O are also applied to this equation.
2.4 Investigation of reductive H2O2 production and accumulation
The current–potential (I–V) curves for H2O2 production using various cathodes were measured with an electrochemical analyzer (BAS Inc., ALS660E) using a three-electrode system composed of a cathode electrode, a Pt-mesh anode and an Ag/AgCl reference electrode in a 2.0 M KHCO3 aqueous solution (35 mL each for the anode and cathode chambers). H2O2 production was performed using a three-electrode system in a two-chamber cell separated by a Nafion membrane between the cathode chamber containing a cathode electrode (standard amount of catalyst loading: 1.5 mg cm−2; area: 1 cm2) and an Ag/AgCl reference electrode and the anode chamber containing a Pt-mesh counter electrode. An aqueous solution of 2.0 M KHCO3 with O2 bubbling at a flow rate of 50 mL min−1 was utilized as an electrolyte (pH 8.6), and the solution was cooled in an ice bath (273–278 K) to prevent the thermal decomposition of the produced H2O2. A constant external bias of +0.5 V (vs. RHE) was applied to the cathode during the reaction.
2.5 Simultaneous H2O2 production on both the photoanode and cathode without external bias
The simultaneous production of H2O2 from H2O oxidation at the photoanode and O2 reduction at the cathode was performed without applying any external bias using a two-electrode system composed of an Al2O3(CVD5)/BiVO4/WO3 photoanode (12 cm2) and a WSoy/GnP-CP cathode (catalyst loading: 1.5 mg cm−2, area: 12 cm2). A 2.0 M KHCO3 aqueous solution was used as an electrolyte (35 mL each for the anode and cathode chamber), and CO2 and O2 gases were bubbled into the anode and cathode chambers of the two-component cell, respectively. It is known that the electrochemical reaction should be accelerated when the pH value of the anolyte solution is higher than that of the catholyte solution.34 In the case of the present study, the difference in pH values between them (anolyte: 7.9 and catholyte: 8.6) will be a little disadvantageous for simultaneous H2O2 production on both the photoanode and cathode without external bias. The solution was cooled in an ice bath (273–278 K) to prevent the thermal decomposition of the produced H2O2. A solar simulator calibrated to AM 1.5G (1SUN, 100 mW cm−2) was used as the light source.
3 Results and discussion
3.1 H2O2 production from H2O on the BiVO4/WO3 photoelectrode modified with Al2O3
Fig. 1 shows the concentration of H2O2 produced in a 0.5 M KHCO3 aqueous solution during the photoelectrochemical H2O oxidation over an Al2O3(CVDn)/BiVO4/WO3 (n = 0–10) photoanode under simulated solar light irradiation. The amount of H2O2 produced improved with an increasing number of CVD cycles, and the H2O2 concentration reached 88 μM (FE(H2O2) = 67%) on the Al2O3(CVD5)/BiVO4/WO3 photoelectrode after passing 0.9 C, while a bare photoelectrode (i.e., no Al2O3 coating) produced 55 μM (FE(H2O2) = 41%). The amount of Al2O3 loaded on the BiVO4/WO3 photoelectrode obviously increased with the number of CVD cycles. These results indicate that the modification of the BiVO4/WO3 photoelectrode with Al2O3 might change its surface properties corresponding to photoelectrochemical H2O2 production. The concentration of bicarbonate ions (HCO3−) in the electrolyte solution affected the H2O2 production, and H2O2 was produced at a FE(H2O2) of 80% over the Al2O3(CVD5)/BiVO4/WO3 photoelectrode when 2.0 M KHCO3 aqueous solution was used as the electrolyte solution (Fig. S1†). The amount of H2O2 produced over the Al2O3(CVD5)/BiVO4/WO3 photoelectrode, which was the best CVD cycle, was commensurate with the case of the Al2O3(MOD)/BiVO4/WO3 photoelectrode reported in our previous paper (Fig. S2(A)†).15 The applied external bias on the Al2O3(CVD5)/BiVO4/WO3 was the same as that of the BiVO4/WO3 photoelectrode (Fig S2(B)†). Moreover, the effect of Al2O3 modification with the CVD method remained even in a prolonged reaction to 50 C (Fig. S3†). The FE values of H2O2 production on photoelectrodes were not influenced by the magnitude of the applied bias (Fig. S4†). Fig. 2 shows the I–V curves of each photoelectrode: (a) Al2O3(CVD5)/BiVO4/WO3, (b) Al2O3(MOD)/BiVO4/WO3, and (c) bare BiVO4/WO3 measured in 2.0 M KHCO3 aqueous solution. The photoelectrode modified with Al2O3(MOD) exhibited lower photocurrent density than the bare BiVO4/WO3 photoelectrode, indicating that thick Al2O3 layers fabricated by the MOD method might disrupt the electrical contact between the BiVO4 and the electrolyte.15 In contrast to this, the Al2O3 layer loaded by the CVD method did not influence the photocurrent density. Accordingly, modification of BiVO4/WO3 with Al2O3(CVD) enabled superior faradaic efficiency for H2O2 production while maintaining good photocurrent–potential properties.
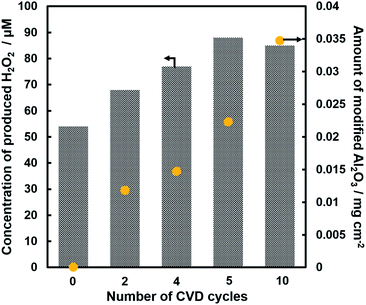 |
| Fig. 1 Concentration of H2O2 produced after 0.9 C of electric charge was passed (left) and the amount of loaded Al2O3 measured by XRF (right). A two-electrode photoelectrochemical cell with an Al2O3(CVD)/BiVO4/WO3 photoanode (6 cm2) and a Pt wire as a cathode electrode was used in a 0.5 M KHCO3 aqueous solution under CO2 bubbling and simulated solar irradiation by controlling the constant current of 1.0 mA. | |
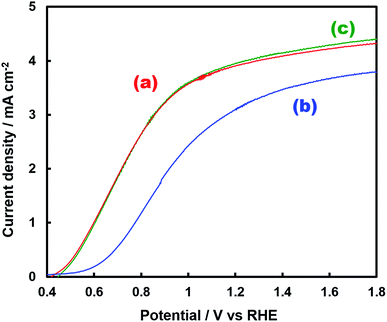 |
| Fig. 2 Current density of the fabricated photoanodes (0.28 cm−2): (a) Al2O3(CVD5)/BiVO4/WO3, (b) Al2O3(MOD)/BiVO4/WO3, and (c) BiVO4/WO3. Three-electrode cell with various photoelectrodes (a–c), a Pt wire as a cathode and an Ag/AgCl reference electrode are used in a 2.0 M KHCO3 aqueous solution under CO2 bubbling and simulated solar irradiation. | |
The distinctive peaks corresponding to BiVO4, WO3, and SnO2 were clearly observed in XRD patterns (Fig. S5†). The BiVO4/WO3 photoelectrode modified by both the MOD method and the CVD method did not exhibit diffraction peaks corresponding to Al2O3, indicating that the prepared Al2O3 layer on the surface of the photoelectrode should be amorphous-like in structure. XRF analysis revealed that the amount of modified Al2O3 on the photoelectrode through 5 CVD cycles was 2.2 × 10−2 mg cm−2 (Fig. 1), and it was less than that on the photoelectrode modified by the MOD method (5.5 × 10−2 mg cm−2). The top-view and side-view SEM images of the bare and Al2O3-modified photoelectrodes are shown in Fig. 3(A) and (B), respectively. The 100–150 nm thick BiVO4 worm-like particle layer (Fig. 3(Aa)) was loaded on the 100–120 nm thick WO3 layer, which has a rather smooth surface (Fig. 3(Ad)) on the FTO substrate. In the case of the MOD method, the Al2O3 layer almost thoroughly covered the BiVO4/WO3 photoelectrode (Fig. 3(Ab) and (Bb)), whereas a mesoporous structure corresponding to pinholes or cracks was partially observed (Fig. 3(Ab)). It is speculated that H2O2 was produced on the surface of BiVO4 layers through the mesoporous structure of Al2O3.15 On the other hand, in the case of the CVD method, the worm-like shape of BiVO4 particles was clearly observed despite the presence of Al2O3, similar to the bare photoelectrode (Fig. 3(Aa) and (Ac)). The location of Al2O3 loaded by the CVD method could not be identified from SEM images. The apparent surface elemental ratio of each photoelectrode, calculated from XPS spectra, is shown in Fig. 4. Both BiVO4 and WO3 were covered with Al2O3 thoroughly by the MOD method (Fig. 4(b)). On the other hand, a tiny part of BiVO4 was covered with Al2O3 introduced by the CVD method, while the majority of WO3 and SnO2 were covered by Al2O3 (Fig. 4(c)). It is speculated that the precursor of Al2O3 (precursor gas: TMA) was more easily absorbed or hydrolyzed on the WO3 and SnO2 layers compared to the BiVO4 layer. As shown in Fig. S6,† the surface elemental ratio of WO3 in the Al2O3(CVD)/BiVO4/WO3 photoelectrode significantly decreased with increasing number of CVD cycles, whereas that of BiVO4 did not decrease within 5 cycles of CVD. It can be estimated that Al2O3 was deposited on the exposed part of the WO3 layer preferentially by the CVD method. FE(H2O2) was clearly improved by Al2O3(CVD) modification in accordance with the decrease in the exposed WO3 ratio. When the amount of Al2O3 loaded by the MOD method (XRF based: 2.0 × 10−2 mg cm−2, Fig. 4(d)) was the same as that in the CVD method (5 cycles, 2.2 × 10−2 mg cm−2), the surface of BiVO4 was thoroughly covered by Al2O3 introduced by the MOD method. The value of ABPE, indicating the conversion efficiency of the solar light energy to chemical energy, was decreased using the Al2O3(MOD)/BiVO4/WO3 photoelectrode in comparison with the bare photoelectrode (Fig. 5), because the photocurrent–potential property was significantly deteriorated by Al2O3 modification with the MOD method, which seems to completely cover the BiVO4 layer (Fig. 2). On the other hand, we achieved the highest ABPE value of 2.57% by using the Al2O3(CVD5)/BiVO4/WO3 photoelectrode, which enabled high FE(H2O2) and superior photocurrent–potential properties simultaneously.
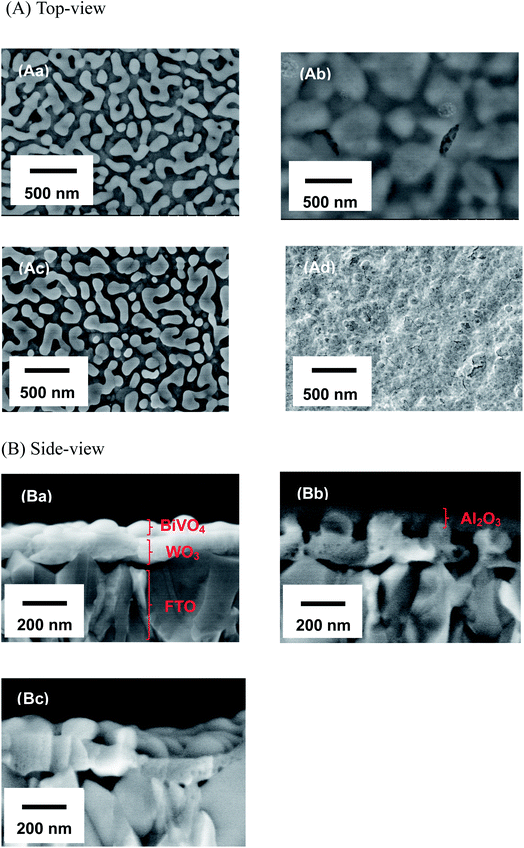 |
| Fig. 3 SEM images of top-views (A) and side-view (B) of the fabricated photoelectrodes: (a) BiVO4/WO3, (b) Al2O3(MOD)/BiVO4/WO3, (c) Al2O3/BiVO4/WO3, and (Ad) WO3 photoelectrodes. | |
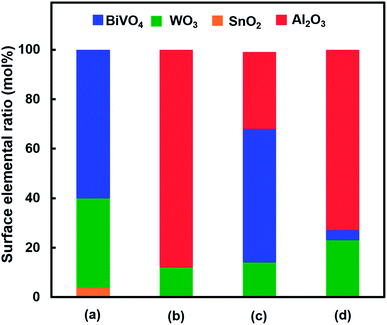 |
| Fig. 4 Surface elemental ratio of the fabricated photoelectrodes: (a) BiVO4/WO3, (b) Al2O3(MOD)/BiVO4/WO3 (Al2O3: 5.5 × 10−2 mg cm−2), (c) Al2O3(CVD5)/BiVO4/WO3 (Al2O3: 2.2 × 10−2 mg cm−2), and (d) Al2O3(MOD)/BiVO4/WO3 (Al2O3: 2.0 × 10−2 mg cm−2), calculated by XPS spectroscopy. | |
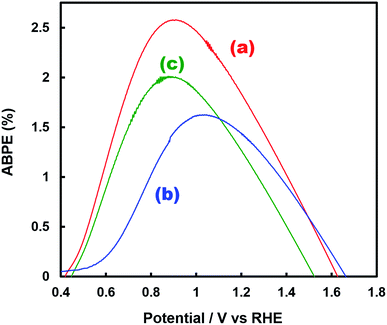 |
| Fig. 5 ABPE values for H2O2, O2, and H2 production after 0.9 C of electric charge was passed in the photoelectrochemical reaction using (a) Al2O3(CVD5)/BiVO4/WO3 (FE(H2O2) = 80%), (b) Al2O3(MOD)/BiVO4/WO3 (FE(H2O2) = 79%), and (c) bare BiVO4/WO3 (FE(H2O2) = 54%) photoelectrodes. FE(H2O2) measured in a two-electrode photoelectrochemical cell (Fig. S2†) and current densities obtained using a three-electrode photoelectrochemical cell (Fig. 2) were applied to the calculation. | |
Fig. 6 exhibits the amount of H2O2 produced in the photoelectrochemical H2O oxidation using three kinds of fabricated photoelectrodes. It is noteworthy that the initial FE(H2O2) at less than 0.02 C was close to 100% for all photoelectrodes regardless of the Al2O3 modification; on the other hand, the FE(H2O2) decreased with time due to oxidative decomposition of H2O2 into O2. The faradaic efficiency of O2 production (FE(O2)) as shown in Fig. S7,† and the sum of FE(H2O2) and FE(O2) were found to be nearly 100% under the reaction conditions (Fig. S7†). The decrease in FE(H2O2) for the Al2O3(CVD)- and Al2O3(MOD)-modified photoelectrodes was much slower than that for the bare photoelectrode, indicating that the Al2O3 layer loaded on the photoelectrode might suppress the decomposition of the produced H2O2 into O2. Fig. S8(a)–(c)† show the decomposition profiles of H2O2 in the presence of photoelectrodes without applying an external bias. H2O2 was barely decomposed under dark conditions for 60 min. However, H2O2 was drastically decomposed under simulated solar light irradiation for all photoelectrodes, indicating that photo-generated carriers (electrons and holes) on the photoelectrodes caused the decomposition of H2O2. The decomposition rates of H2O2 were obviously suppressed by the modification of photoelectrodes with the CVD (Fig. S8(a)†) and MOD (Fig. S8(b)†) methods. The decomposition rate over a BiVO4 photoelectrode without WO3 (Fig. S8(d)†) was much slower than that over a WO3 photoelectrode (Fig. S8(e)†) under simulated solar light irradiation, whereas the amounts of photo-generated carriers for WO3 were much smaller than those for BiVO4 considering the light harvesting efficiencies (LHEs) in Fig. S9.† We also confirmed that the modification of WO3 with Al2O3 by the CVD method clearly inhibits the H2O2 decomposition under the same conditions as Fig. S8.† These results suggested that H2O2 was easily decomposed on WO3, and the modification of the BiVO4/WO3 photoelectrode with Al2O3 effectively suppressed the decomposition of the produced H2O2 by blocking contact with WO3 as shown in Fig. S10.† Moreover, an Al2O3 layer fabricated by the MOD method on the photoelectrode, which thoroughly covers the BiVO4 layer, caused the deterioration of the photocurrent–potential property (Fig. 2). On the other hand, Al2O3 loading by the CVD method, masking WO3 and SnO2 preferentially, achieves an improvement in the FE(H2O2) without degrading the photocurrent–potential property. Accordingly, high ABPE values were obtained in the present study.
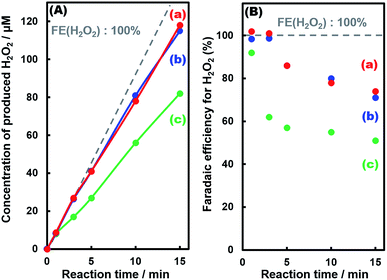 |
| Fig. 6 Time courses of (A) concentration of H2O2 produced and (B) FE(H2O2) in the photoelectrochemical reaction using (a) Al2O3(CVD5)/BiVO4/WO3, (b) Al2O3(MOD)/BiVO4/WO3, and (c) bare BiVO4/WO3. A two-electrode photoelectrochemical cell with a photoanode (6 cm2) and a Pt wire as a cathode electrode was used in a 2.0 M KHCO3 aqueous solution under CO2 bubbling and simulated solar irradiation by controlling the constant current of 1.0 mA. | |
3.2 Reductive H2O2 production from O2 on the cathode
Fig. 7 shows the cathodic current density for O2 reduction on various cathodes such as MoSoy-CP, WSoy/GnP-CP, CB-CP, Au wire, and bare CP in 2.0 M KHCO3 aqueous solution. The cathodic currents corresponding to O2 reduction over these fabricated cathodes were much higher than that over the Au cathode. Moreover, the onset potentials of MoSoy-CP and WSoy/GnP-CP were more positive than those of CB-CP and Au. Among them, the WSoy/Gn-CP composite cathode showed the highest cathodic current density for the electrochemical reduction of O2 in an aqueous KHCO3 electrolyte solution. Table 1 shows the total current density for O2 reduction, FE(H2O2) after 1.0 C of electric charge was passed, and current density for H2O2 production in the electrochemical reactions at +0.5 V (vs. RHE) using various cathodes. CB and Au showed high FE(H2O2), while the total current density and the current density for H2O2 production of both cathodes were low. On the other hand, WSoy/GnP showed large current density and a relatively high FE(H2O2) of 39% (the rest might be H2O production from O2.) simultaneously, indicating that the CP cathode with WSoy/GnP showed the highest H2O2 production performance (cathodic current density for H2O2 production: −1.1 mA cm−2). Moreover, this catalyst was stable under these reaction conditions. Fig. S11† shows the comparison of the I–V curve and the FE(H2O2) on the WSoy/GnP loaded cathode before/after 100 cycles of CV measurements operated under the same conditions as Fig. 7. Both the I–V curve and FE(H2O2) were unchanged after 100 cycles of CV measurements. It is noteworthy that these biomass-derived electrocatalysts reported for H2 production could be utilized also for H2O2 production from O2.
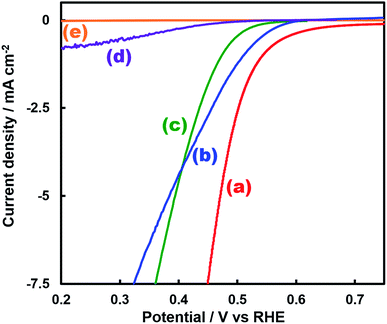 |
| Fig. 7 Current density of various cathodes (loading of catalyst: 1.5 mg cm−2; electrode area: 1 cm2): (a) WSoy/GnP, (b) MoSoy, (c) carbon black (CB), (d) Au wire, and (e) bare CP. A three-electrode cell containing various cathodes (a–d), a Pt wire as an anode and an Ag/AgCl reference electrode was used in 2.0 M KHCO3 aqueous solution under O2 bubbling. | |
Table 1 Total current density of various cathodes at +0.5 V (vs. RHE), FE(H2O2) after 1.0 C of the electric charge was passed, and the calculated current density for H2O2 production (total current density × FE(H2O2)) in the electrochemical O2 reductiona
Cathode material |
Total current density (mA cm−2) |
FE(H2O2) at 1 C (%) |
Current density for H2O2 production (mA cm−2) |
Measurement conditions: a three-electrode cell with a Pt wire as a counter electrode, various cathodes as working electrodes and an Ag/AgCl reference electrode were used with a 2.0 M KHCO3 aqueous solution under O2 bubbling.
|
Bare CP |
5.4 × 10−4 |
— |
— |
Au wire |
−4.5 × 10−2 |
57 |
−2.5 × 10−2 |
CB |
−4.5 × 10−1 |
83 |
−3.7 × 10−1 |
MoSoy |
−1.5 |
19 |
−2.9 × 10−1 |
WSoy/GnP |
−2.9 |
39 |
−1.1 |
Finally, we have demonstrated H2O2 production with the combination of the WSoy/GnP-CP cathode (loading: 1.5 mg cm−2, 12 cm2) and the Al2O3(CVD5)/BiVO4/WO3 photoelectrode (12 cm2) under simulated solar light irradiation in 2.0 M KHCO3 aqueous solution (Fig. 8(A) and (B)). The open circuit voltage was ∼0.30 V. Notably, approximately 0.7 mA photocurrent was observed without applying an external bias. H2O2 was produced on the cathode and the photoanode with an FE(H2O2) of 44% and 60%, respectively, after 1.8 C of electric charge was passed. Accordingly, a new photoelectrochemical cell was successfully developed by using the Al2O3-modified BiVO4/WO3 photoelectrode and the biomass-derived carbon cathode, which enabled us to achieve direct conversion of solar light into chemical energy (H2O2, eqn (7)) without applying an external bias.
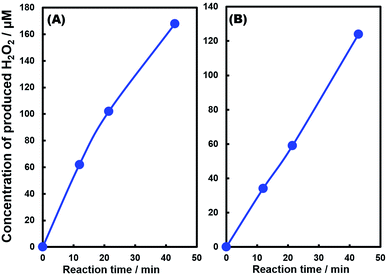 |
| Fig. 8 Time course of concentration of H2O2 produced on (A) the Al2O3(CVD5)/BiVO4/WO3 photoelectrode (12 cm2) and (B) the WSoy/GnP-CP cathode (loading: 1.5 mg cm−2) without applying external bias. A two-electrode photoelectrochemical cell with an Al2O3(CVD5)/BiVO4/WO3 photoelectrode and a WSoy/GnP-CP cathode was used in 2.0 M KHCO3 aqueous solution under CO2 or O2 bubbling and simulated solar irradiation. | |
4 Summary
The modification of BiVO4/WO3 photoelectrodes with Al2O3 using the chemical vapor deposition (CVD) method exhibited superior faradaic efficiency for H2O2 production while maintaining favorable photocurrent–potential properties, where we achieved the highest ABPE value of 2.57% by using the Al2O3(CVD5)/BiVO4/WO3 photoelectrode among the photoelectrodes we investigated. In the case of the metal–organic decomposition (MOD) method, an Al2O3 layer fabricated on the photoelectrode thoroughly covered the BiVO4 layer, causing the deterioration of the photocurrent–potential property. On the other hand, with the CVD method, the Al2O3 layer fabricated on the photoelectrode, masking WO3 and SnO2 preferentially, suppressed the decomposition of produced H2O2 on WO3 without degrading the photocurrent–potential property. Furthermore, we discovered that the CP cathode with a biomass-derived W-based electrocatalyst (WSoy/GnP-CP) exhibited superior activity for the reductive H2O2 production from O2 over an Au cathode. As a consequence, a newly developed photoelectrode cell, composed of an Al2O3(CVD)/BiVO4/WO3 photoanode and a biomass-derived carbon cathode, enables us to achieve simultaneous production of H2O2 on both the photoanode and cathode without applying external bias and employing a noble metal.
Conflicts of interest
There are no conflicts to declare.
Acknowledgements
We thank Dr Wei-Fu Chen and Dr Fanke Meng for the preparation of MoSoy and WSoy/GnP, respectively, and Dr James Muckerman for a careful reading of the manuscript. The present work was partially supported by the International Joint Research Program for Innovative Energy Technology. The work at BNL was carried out with support from the U.S. Department of Energy, Office of Science, Division of Chemical Sciences, Geosciences & Biosciences, and Office of Basic Energy Sciences under contract DE-SC0012704.
References
- A. Fujishima and K. Honda, Nature, 1972, 238, 37 CrossRef PubMed.
- M. Grätzel, Nature, 2001, 414, 338 CrossRef PubMed.
- R. Abe, J. Photochem. Photobiol., C, 2010, 11, 179 CrossRef.
- G. Hodes, D. Cahen and J. Manassen, Nature, 1976, 260, 312 CrossRef.
- C. Santato, M. Ulmann and J. Augustynski, J. Phys. Chem. B, 2001, 105(5), 936 CrossRef.
- B. Yang, P. R. F. Barnes, Y. Zhang and V. Luca, Catal. Lett., 2007, 118, 280 CrossRef.
- K. Sayama, K. Nomura, Z. Zou, R. Abe, Y. Abe and H. Arakawa, Chem. Commun., 2003, 23, 2908 RSC.
- T. W. Kim, Y. Ping, G. A. Galli and K. S. Choi, Nat. Commun., 2015, 6, 8769 CrossRef PubMed.
- J. H. Kennedy and N. Anderman, J. Electrochem. Soc., 1983, 130, 848 CrossRef.
- A. Kudo, K. Omori and H. Kato, J. Am. Chem. Soc., 1999, 121, 11459 CrossRef.
- I. Dincer and C. Acar, Int. J. Hydrogen Energy, 2015, 40, 11094 CrossRef.
- K. Ueno and H. Misawa, NPG Asia Mater., 2013, 5, e61 CrossRef.
- K. Fuku and K. Sayama, Chem. Commun., 2016, 52, 5406 RSC.
- K. Fuku, Y. Miyase, Y. Miseki, T. Funaki, T. Gunji and K. Sayama, Chem.–Asian J., 2017, 12, 1111 CrossRef PubMed.
- K. Fuku, Y. Miyase, Y. Miseki, T. Gunji and K. Sayama, RSC Adv., 2017, 7, 47619 RSC.
- X. Shi, S. Siahrostami, G. L. Li, Y. Zhang, P. Chakthranont, F. Studt, T. J. Jaramillo, X. Zheng and J. K. Nǿrskov, Nat. Commun., 2017, 8, 701 CrossRef PubMed.
- Z. Lu, G. Chen, S. Siahrostami, Z. Chen, K. Liu, J. Xie, L. Liao, D. Lin, T. F. Jaramillo and J. K. Nǿrskov, Nat. Catal., 2018, 1, 156 CrossRef.
- Q. Mi, A. Zhanaidarova, B. S. Brunschwig, H. B. Gray and N. S. Lewis, Energy Environ. Sci., 2012, 5, 5694 Search PubMed.
- K. Fuku, N. Wang, Y. Miseki, T. Funaki and K. Sayama, ChemSusChem, 2015, 8, 1593 CrossRef PubMed.
- S. Iguchi, Y. Miseki and K. Sayama, Sustainable Energy Fuels, 2018, 2, 155 Search PubMed.
- R. Nakamura and Y. Nakato, J. Am. Chem. Soc., 2004, 126, 1290 CrossRef PubMed.
- R. A. Sheldon and J. Dakka, Catal. Today, 1994, 19, 215 CrossRef.
- R. Noyori, M. Aoki and K. Sato, Chem. Commun., 2003, 1977 RSC.
- K. Otsuka and I. Yamanaka, Electrochim. Acta, 1990, 35, 319 CrossRef.
- D. E. Richardson, H. Yao, K. M. Frank and D. A. Bennet, J. Am. Chem. Soc., 2000, 122, 1729 CrossRef.
- R. Saito, Y. Miseki and K. Sayama, Chem. Commun., 2012, 48, 3833 RSC.
- I. Fujimoto, N. Wang, R. Saito, Y. Miseki, T. Gunji and K. Sayama, Int. J. Hydrogen Energy, 2014, 39, 2454 CrossRef.
- K. Fuku, Y. Miyase, Y. Miseki, T. Gunji and K. Sayama, ChemistrySelect, 2016, 1, 5721 CrossRef.
- S. J. Hong, S. Lee, J. S. Jang and J. S. Lee, Energy Environ. Sci., 2011, 4, 1781 Search PubMed.
- W. Chen, S. Iyer, S. Iyer, K. Sasaki, C. H. Wang, Y. Zhu, J. T. Muckerman and E. Fujita, Energy Environ. Sci., 2013, 6, 1818 Search PubMed.
- F. Meng, E. Hu, L. Zhang, K. Sasaki, J. T. Muckerman and E. Fujita, J. Mater. Chem. A, 2015, 3, 18572 Search PubMed.
- K. Sayama, A. Nomura, T. Arai, T. Sugita, R. Abe, M. Yanagida, T. Oi, Y. Iwasaki, Y. Abe and H. Sugihara, J. Phys. Chem. B, 2006, 110, 11352 CrossRef PubMed.
- P. R. Mishra, P. K. Shukla and O. N. Srivastava, Int. J. Hydrogen Energy, 2007, 32, 1680 CrossRef.
- A. Kaplan, E. Kolin, S. Halevy and A. Bettlheim, Electrochem. Commun., 2015, 60, 97 CrossRef.
Footnote |
† Electronic supplementary information (ESI) available. See DOI: 10.1039/c8se00070k |
|
This journal is © The Royal Society of Chemistry 2018 |
Click here to see how this site uses Cookies. View our privacy policy here.