DOI:
10.1039/D1QO00727K
(Review Article)
Org. Chem. Front., 2021,
8, 4886-4913
Green strategies for transition metal-catalyzed C–H activation in molecular syntheses
Received
10th May 2021
, Accepted 2nd June 2021
First published on 2nd June 2021
Abstract
Transition metal catalyzed C–H activation has surfaced as a powerful tool to improve the efficacy of molecular synthesis. Last two decades have witnessed a considerable progress in the activation of otherwise inert C–H bonds. However, during recent years, focus has shifted to address rising concerns to achieve high levels of resource economy in molecular syntheses. Herein, we discuss the advent and recent emerging strategies to improve the sustainability and environmentally benign nature of C–H activation manifolds.
The emergence of resource-economical, environmentally-benign strategies is one of the primary objectives in the field of organic synthesis for the construction of structurally complex and diverse molecules.1 For instance, the tremendous progress in transition metal-catalyzed coupling reactions has propelled this discipline, enabling diverse applications in industries as well as in academia.2 However, the pre-functionalization of starting materials and the formation of undesired byproducts jeopardize the overall efficiency of this approach. In stark contrast, catalyzed C–H activations3 has been recognized as an increasingly powerful alternative in terms of step- and atom-economy for the assembly of complex molecules,4 with transformative applications to among others late-stage diversification,5 material sciences,6 and pharmaceutical industries.7 This approach avoids the use of pre-functionalized starting materials, such as sensitive organometallic reagents, thereby preventing additional steps for pre-functionalizations and concurrent undesired waste generation.
Recent attention has shifted to improve the overall sustainability of the C–H activation approach using greener and more resource-economical strategies thus enabling lower E-factors.8 The use of non toxic, inexpensive Earth-abundant 3d metal catalysts9 as well as alternative energy10 sources are particularly attractive for environmentally benign transformations. Therefore, in this review we are focusing on the recent developments in the design of enabling green strategies for otherwise activation of the inert C–H bonds towards improved sustainability and resource-economy.11
Environmentally-benign solvents
The tremendous progress of transition metal-catalysis is associated with an environmental disadvantage, because the use of organic solvents generates major amounts of waste, both in the chemical and pharmaceutical industries.12 Unfortunately, the commonly used organic solvents are volatile, toxic and flammable, which potentially, constitutes a major safety hazard that is considered undesirable for large production.13 Therefore, environmentally-benign solvents are in high demand for transition metal-catalyzed C–H activations as a sustainable alternative.13d,g,14
The application of environmentally-benign solvents15 in transition metal-catalyzed C–H activation is attractive, because they are readily available from biomass feedstocks and generally less toxic with high biodegradability, minimizing the environmental footprints.14b,16 Apart from bio-based solvents, water also has found applications in various C–H transformations.17
Polyethylene glycols (PEGs)
Polyethylene glycols (PEGs), available from inexpensive ethylene glycol,18 have gained considerable attention as green reaction media19 for organic transformations due to their favourable physiochemical properties and negligible toxicities. Commercially available PEGs have been recognized as promising green solvents in industries due to their non-flammable and non-corrosive nature.19
In 2009, Ackermann reported on the first transition metal-catalyzed C–H arylations in PEGs as reaction media (Scheme 1).20 Chelation-assisted C–H arylations were achieved by the combination of catalytic amounts of [RuCl3(H2O)n] and a co-catalytic amount of MesCO2H (2,4,6-trimethylbenzoic acid) to furnish the desired biaryls 3 in high yields. Various arenes 1 were arylated in a regioselective fashion by chelation-assisted ruthenium catalysis.
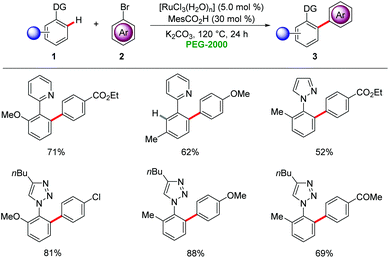 |
| Scheme 1 Ruthenium-catalyzed C–H arylation in PEG-2000. | |
In a recent study, Li disclosed a related arylation of heteroarenes via C–H bond activation in PEG-400 with recyclable and inexpensive RuCl3·xH2O21 as the catalyst.22
A green synthesis of benzimidazoisoquinolines236 was reported by Chandrasekhar via a ruthenium-catalyzed alkyne annulation with 2-aryl benzimidazoles 4 in a PEG-400/water reaction mixture at room temperature in the presence of Cu(OAc)2·H2O as oxidant (Scheme 2).24 Notably, the catalyst could be recycled without significant loss of catalytic efficiency. In contrast, when using toluene as the solvent a reaction temperature of 111 °C was required.
 |
| Scheme 2 Ruthenium-catalyzed oxidative annulation with alkynes 5 in PEG-400/H2O. | |
Bhanage employed recently a recyclable ruthenium/PEG-400 catalytic system for the oxidative annulation with internal alkynes 5via directed C–H activation for the synthesis of isoquinolinones, isocoumarins, and N-methyl isoquinolinones25 by the cleavage of C–H, N–O and O–O bonds (Scheme 3a).26 Likewise, the oxidative alkenylation of N-methoxy-N-methylamides 8 with substituted styrenes 15 was achieved (Scheme 3b). Interestingly, the recyclability test for the ruthenium catalyst and PEG-400 provided satisfactory results without loss of the catalytic efficiency. The product isolation was conducted by extraction with diethyl ether, along with column chromatography using toluene and ethyl acetate as the eluents. In a recent study, the same group extended the approach towards N-tosylhydrazones for the synthesis of isoquinolinones via C–H/N–N activation.27
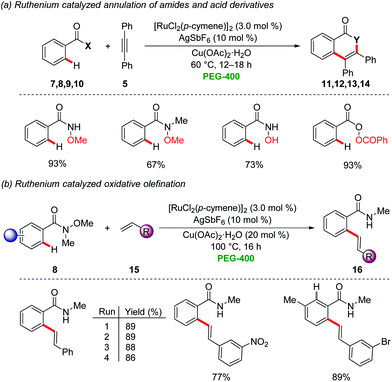 |
| Scheme 3 Ruthenium-catalyzed oxidative annulation and olefination of amides in PEG-400. | |
In a related study, Cai reported on the synthesis of phthalides by recyclable ruthenium-catalyzed oxidative C–H alkenylations in a PEG-400/water (3
:
2) mixture. Various substituted benzoic acids and electron-deficient alkenes were amenable substrates for the alkenylation to afford the desired phthalides in good yields.28
Ackermann demonstrated that PEGs are viable green reaction media for palladium-catalyzed C–H arylations. Co-catalytic amounts of MesCO2H significantly improved the reaction efficiency for the direct arylation of triazoles 17 under aerobic conditions with 5.0 mol% of Pd(OAc)2 in PEG-20000 (Scheme 4).20 The catalyst was found to be easily reusable, taking advantage of the physiochemical properties of PEG-20000 without significant loss in activity.
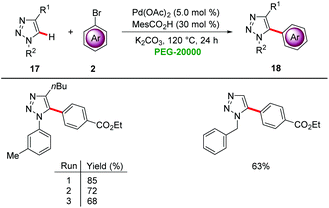 |
| Scheme 4 Palladium-catalyzed C–H arylation in PEG-20000. | |
Berteina-Raboin developed a sequential one-pot synthesis of 2,3-diarylimidazo-[1,2-a]pyridines 21 starting from easily-accessible 2-amino pyridines 19, α-bromo ketones 20 and aryl bromides 2 in PEG-400 (Scheme 5).29 The use of Pd(OAc)2 as the catalyst and KOAc as the base at 220 °C under microwave irradiation for 1 h gave the optimal results.
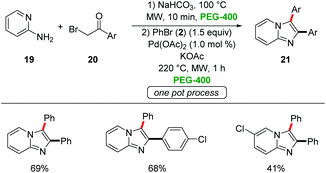 |
| Scheme 5 One pot sequential approach for palladium-catalyzed arylation in PEG-400. | |
In 2015, Ackermann showed PEG-400 to be suitable solvent for Earth-abundant 3d metal-catalyzed C–H activation. Here, a combination of inexpensive Co(OAc)2 and sacrificial oxidant Mn(OAc)2 or AgOPiv afforded isoindolinone derivatives 23 from aromatic benzamides 22 by C–H/N–H annulation with alkenes 15 under aerobic conditions (Scheme 6).30 Notably, organic solvents failed to provide the desired products in synthetically useful yields, highlighting the unique potential of PEG-400 as the reaction media. Several sensitive functional groups were well tolerated, providing versatile access to substituted isoindolinones 23.
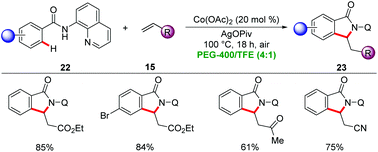 |
| Scheme 6 Cobalt-catalyzed C–H/N–H annulation in PEG-400/TFE. | |
Later, Cui employed picolinamides as a traceless directing group for the cobalt-catalyzed synthesis of isoquinonlines 25via C–H/N–H activation using oxygen as terminal oxidant (Scheme 7).31 The reaction proceeded efficiently for a broad range of substrates including terminal and internal alkynes 5 with excellent regioselectivities.
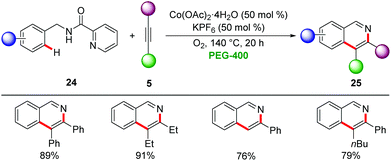 |
| Scheme 7 Cobalt-catalyzed synthesis of isoquinonlines 25 in PEG-400. | |
Furthermore, copper-catalysis was amenable for C–H alkynylation in PEG-400. Here, catalytic amounts of CuBr were used for the kinetic C–H acidity guided alkynylation of 1,3,4-oxadiazoles 26 by gem-dibromoalkenes 27 to afford the desired products 28 in moderate to good yields (Scheme 8).32
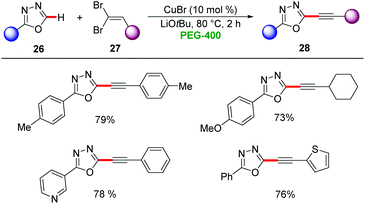 |
| Scheme 8 Copper-catalyzed alkynylation in PEG-400. | |
For the isolation of the desired products, simple extraction with ethereal solvents was carried out, followed by column chromatography.
γ-Valerolactone (GVL)
γ-Valerolactone's characteristic features include a high boiling point, a low vapour pressure, low toxicity along with high miscibility with water and most importantly it is highly biodegradable.33 This environmentally-benign aprotic solvent is produced from levulinic acid which is itself derived from lignocellilosic biomasses.34 Due to its physicochemical properties, GVL has found widespread applications as an alternative to hazardous organic solvents in transition metal-catalyzed transformations, including cross-coupling reactions,35 hydroformylations36 and aminocarbonylations.37 In contrast, step-economical catalyzed C–H activations in renewable biomass derived solvent GVL have only recently been developed.
For instance, Larini and Cravotto disclosed the palladium-catalyzed C–H arylation of heterocycles 29 with aryl halides 2 in GVL as solvent (Scheme 9a).38 Remarkably, only 0.2 mol% Pd(OAc)2 was sufficient for efficient regioselective C2 arylation of thiophenes 29 under microwave irradiation at 140 °C. The reaction conditions proved applicable to palladium-catalyzed synthesis of poly(3-hexyl)thiophene 31 by C–H (hetero)arylation polymerisation (Scheme 9b).
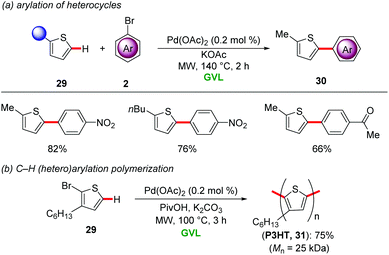 |
| Scheme 9 Palladium-catalyzed C–H arylation in GVL. | |
More cost-effective ruthenium-catalysts enabled the synthesis of phthalides 33 from aryl carboxylic acids 32 and alkenes 15 by Ackermann in biomass-derived GVL using molecular oxygen as the sole oxidant (Scheme 10).39 The oxidative double C–H functionalization was characterized by high positional selectivity and functional group tolerance, with sensitive bromo, iodo and hydroxyl groups being tolerated. The reaction mixture was extracted with H2O and n-hexane/MTBE to remove the GVL.
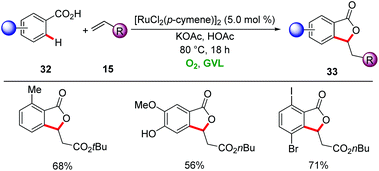 |
| Scheme 10 Ruthenium-catalyzed green synthesis of phthalides 33 in GVL. | |
In a recent study, the same group found GVL as an effective solvent for the ruthenium(II)-catalyzed distal C–H alkenylation of arylacetamides via weakly coordinating amide assistance.40
Ackermann also demonstrated GVL as a suitable solvent for Earth abundant 3d metal-catalyzed C–H activations. Thus, an ortho C–H arylation of benzamide 22 in GVL was realized (Scheme 11).41 In addition, challenging C(sp3)–H bond arylations were also achieved with arylsiloxanes in GVL as reaction media albeit at a higher temperature.
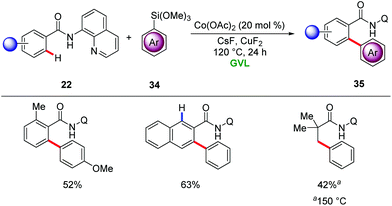 |
| Scheme 11 Cobalt-catalyzed ortho-C–H arylation of benzamide 22 in GVL. | |
Furthermore, GVL has also found applications to heterogenous palladium-catalyzed C–H activations as well as in the field of electrochemistry to enhance the sustainability in molecular synthesis (vide infra).
2-Methyltetrahydrofuran (2-MeTHF)
2-Methyltetrahydrofuran (2-MeTHF) is largely produced from renewable lignocellulosic biomasses.42 Favourable physio-chemical properties of 2-MeTHF render it as an eco-friendly alternative to THF with lower miscibility with water and higher boiling point.43 In this section, we summarize the potential of 2-MeTHF as a green reaction media for catalyzed C–H activation.
McMullin, Williams and Frost disclosed ortho-C–H alkenylation of a variety of N-aryloxazolinone 36 using [RuCl2(p-cymene)]2 as a catalyst in 2-MeTHF (Scheme 12).44 2-MeTHF showed superior efficacy to typically used organic solvents for the desired alkenylated product with high levels of monoselectivity.
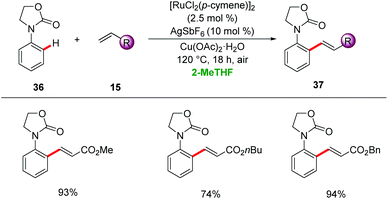 |
| Scheme 12
ortho-C–H alkenylation of a variety of N-aryloxazolinone 36 in 2-MeTHF. | |
Ackermann employed 2-MeTHF for ruthenium-catalyzed remote meta-C–H functionalization of purines 38.45 Hence, the arene-ligand free complex [Ru(OAc)2(PPh3)2] enabled the meta-C–H alkylation of purines 38via a ruthenium(II/III) manifold with excellent levels of chemo- and meta-selectivity (Scheme 13).
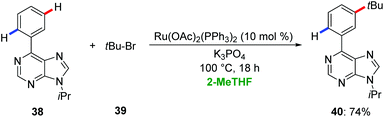 |
| Scheme 13 Ruthenium-catalyzed meta-selective C–H alkylation in 2-MeTHF. | |
Sommer demonstrated a sustainable synthesis of n-type conjugated copolymers PNDIT2 43 from 2,6-dibromonapthalene diimide 42 using palladium-catalyzed direct arylations in 2-MeTHF (Scheme 14).46 The palladium catalyst delivered the desired product PNDIT2 43 in 98% yield and with molecular weight of Mn ∼20 kDa. Later, the synthesis of benzodithiophene-alt-diketopyrrolopyrrole copolymer was achieved via a palladium-catalyzed direct heteroarylation polymerization (DHAP) in 2-MeTHF.47
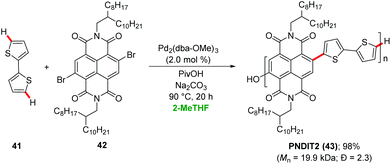 |
| Scheme 14 Palladium-catalyzed direct C–H arylation polymerization in 2-MeTHF. | |
The Cook group employed an inexpensive and non-toxic iron catalyst for the ortho-alkylation of aromatic amides with alkyl bromides in biomass-derived 2-MeTHF as a green reaction media via bidentate 8-AQ assistance. The combination of catalytic amounts of Fe(acac)3 as catalyst and dppe as ligand enabled primary and secondary alkylations in high yields and excellent regioselectivities.48
Water:micellar catalysis
Water is nontoxic, non-flammable, non-corrosive, naturally abundant and cost-efficient, providing key advantages as a sustainable, safe and environmentally-benign solvent.17 The eco-friendly nature of water emphasizes its significant potential as reaction media for organic transformations, particularly in combination with non-renewable organic solvents for the workup procedures. However, the solubility and instability of organic or organometallic compounds in water often imposes significant limitations for the use of water as a solvent.49 Therefore, surfactants have emerged as enabling tools for realizing homogenous metal catalysis in water. In this section, we discuss micellar catalysis for transition metal-catalyzed C–H activations in water.
A mixture of 2% surfactant/H2O was employed in the palladium-catalyzed C–H arylations of anilides 44 to provide diversely substituted biaryl compounds 46 (Scheme 15).50 Brij 35 was found by Lipshutz as an efficient surfactant for the C–H arylation with AgOAc as a stoichiometric additive at room temperature.
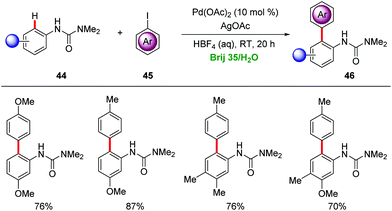 |
| Scheme 15 Palladium-catalyzed C–H arylation of anilides 44 in Brij 35/H2O. | |
Later, ortho-acylations of anilides were also achieved in an aqueous solution of a surfactant via a palladium-catalyzed cross dehydrogenative coupling between anilides and aromatic aldehydes.51
Ackermann devised a ruthenium-catalyzed C–H arylation in water through micellar catalysis.52 The presence of a single component ruthenium catalyst and K2CO3 as base gave optimal results for the C–H arylation of sensitive ferrocenes 47 through weak thioketone assistance in a solution of 2% TPGS-750 in H2O (Scheme 16).
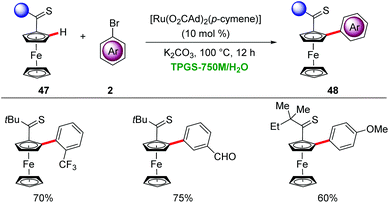 |
| Scheme 16 Ruthenium-catalyzed C–H arylation in TPGS-750M/H2O. | |
Water itself has been found applications as a reaction medium for a variety of C–H bond functionalization reactions, catalyzed by palladium,53 rhodium,54 iridium55 and ruthenium.56 Similarly, water has also been found as an amenable solvent for Earth-abundant 3d metal-catalyzed C–H functionalization.57
In this context, in 2017, decarboxylative C–H/C–O functionalizations by manganese catalysis in H2O were realized by Ackermann.58 Air- and water-tolerant manganese(I) catalysis enabled versatile C–H allylations of indole 49 with high levels of chemo- and regioselectivity (Scheme 17).
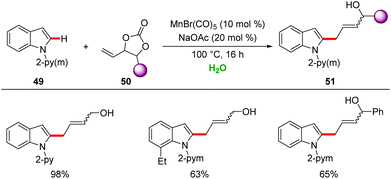 |
| Scheme 17 Manganese(I)-catalyzed C–H allylation in H2O. | |
In addition, water-tolerant manganese(I)-catalysis was easily expanded to the unprecedented C–C activation of alcohol, leading to the allylated and hydroarylated arenes.59 It is noteworthy that similar reactivity of sequential C–H and C–C/C–Het bond activation was demonstrated by Glorius under neat reaction conditions.60
Generally speaking, green solvents have emerged as viable reaction medium for C–H activation reactions. However, to unleash the full potential, toxic organic solvents should ideally be avoided during the workup procedures for the isolation and purification of the target products.
Heterogeneous catalytic system
Despite significant advances in C–H functionalization with homogenous catalysts, a major challenge for large-scale applications is associated with the limited recyclability of the often expensive catalysts and difficult removal of undesired trace metal impurities.61 Hence, heterogenous catalysts are in high demand for sustainable molecular synthesis. Further recyclable heterogenous catalysts provide an opportunity for innovative transformations through the design of hybrid metal catalysts.62
An early report on C–H arylations by Fagnou proved viable with the commercially available heterogeneous palladium catalyst Pd(OH)2/C.63 Pearlman's catalyst allowed for efficient intra- and intermolecular C–H arylation with aryl iodides 52 and bromides 2 (Scheme 18). Detailed experimental studies revealed in situ formation of the active homogenous palladium species. In a related work, C-2 selective arylations of NH-free pyrroles were realized with the Pearlman's catalyst.64
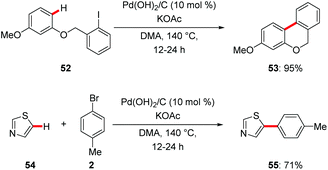 |
| Scheme 18 Heterogenous palladium-catalyzed C–H arylation. | |
In 2015, the Glorius group disclosed C–H arylations of triphenylene, napthalenes and anthracene with the combination of diaryliodonium salts and catalytic amounts of Pd/C.65
Aimed at improving catalyst recyclability, chemists have focused their attention on using different types of heterogeneous palladium sources for efficient arylation.66 Along with heterogeneous palladium sources, the addition of catalytic amounts of copper has also been beneficial for the regio- and chemoselective arylations.67
For example, a reusable copper-based catalyst system was used for the direct arylation of anilides 56 with meta-selectivity using reusable heterogeneous copper nanoparticles (Scheme 19).68
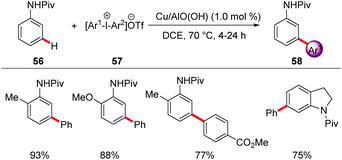 |
| Scheme 19
meta-C–H arylation of anilides 56 with heterogenous copper nanoparticles. | |
In subsequent studies, Wang further achieved the C–H arylation of various heterocycles in the presence of a heterogenous catalyst CuO nano spindles in diglyme.69 Furthermore, heterogenous MOF based copper catalysts have found suitable application for the direct C–H arylation of heteroarenes.70
Very recently, Ackermann achieved heterogeneous photo-induced copper-catalyzed C–H arylation of heteroarenes 54, 59 with aryl iodides 45 at room temperature (Scheme 20).71 This photo-catalysis strategy proved to be highly robust leading to minimal leaching and enabling the recycling of the catalyst without loss of its efficiency.
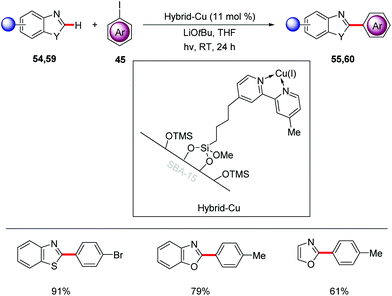 |
| Scheme 20 Heterogenous copper-catalyzed photo-induced C–H arylation. | |
While a significant number of heterogeneous catalytic based systems are based mainly on the arylation of aromatic substrates,72 recent interest on other types of C–C and C–Het bond formation reactions using recyclable heterogenous catalysis has gained considerable attention.73
In 2016, Ackermann and Vaccaro realized the heterogenous palladium catalysis for the Catellani reaction in the renewable biomass derived green solvent GVL.74 The authors employed two different heterogenous palladium sources, namely, Pd/Al2O3 and Pd EnCAt™ 30, to enable difunctionalization with a variety of alkenes 15, providing access to diverse meta-substituted arenes 62. Notably, biomass-derived GVL proved to be the superior solvent among other typical organic solvents (Scheme 21).
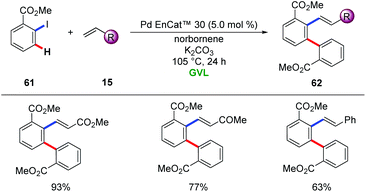 |
| Scheme 21 Heterogeneous palladium-catalyzed Catellani reaction in GVL. | |
The same groups reported heterogeneous Pd/C for the C–H arylation of 1,2,3-triazoles with aryl bromides in GVL. Co-catalytic amount of MesCO2H and CF3CO2K were used as ligand and base respectively for the direct arylation to provide access to fully decorated 1,2,3-triazoles in good to excellent yields with good functional group tolerance. Interestingly, the heterogenous palladium catalyst was also applicable for the intramolecular C–H arylation of the substrate 63 to afford isoindole motif 64 in good yield (Scheme 22a).75 Later, Ackermann and Vaccaro further extended the heterogenous palladium-catalyzed direct C–H functionalization of 1,2,3-triazoles 65 using continuous flow regime in GVL as a green reaction media (Scheme 22b).76
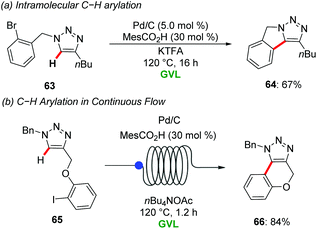 |
| Scheme 22 Heterogenous palladium-catalyzed C–H arylation in GVL. | |
The Glorius group employed Pd/Al2O3 as a heterogenous catalyst for the direct C–H thiolation and selenation reactions.77 The combination of Pd/Al2O3 and CuCl2 in dichloroethane or toluene gave optimal results for the synthesis of sulfenylated 69 and selenylated products 70 (Scheme 23).
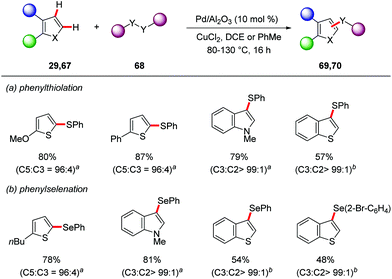 |
| Scheme 23 Heterogenous palladium-catalyzed direct C–H thiolation and selenylation. a DCE, 80 °C, b PhME, 130 °C. | |
Furthermore, C–H cyanations were achieved by heterogenous palladium(II) and magnesium–lanthanum mixed oxide.78 The combination of NH4HCO3 and DMSO served as cyano source to allow for regioselective C–H cyanations of heteroarenes 1 (Scheme 24).
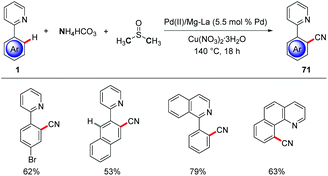 |
| Scheme 24 Heterogenous palladium-catalyzed C–H cyanation. | |
C–H halogenations were achieved using an easily synthesized Pd@MOF nano catalysts by Martín-Matute.79 The halogenation reaction was conveniently performed with the robust MOF-based heterogenous catalysts to deliver the desired products 73 and 74 under very mild conditions with excellent mono- and di-selectivities (Scheme 25). It is worth mentioning that the MOFs were recycled and reused without loss of efficacy.
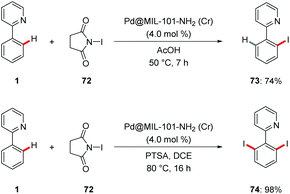 |
| Scheme 25 C–H halogenation by Pd@MOF nano catalysts. | |
meta-C–H Brominations of aryl substituted purine bases 38 were devised by Ackermann using a heterogenous ruthenium catalyst (Scheme 26).80 The robust heterogenous Ru@SiO2 catalyst proved broadly applicable for the meta-selective bromination with NBS with excellent level of positional selectivity to furnish the meta-brominated purine bases 76. Interestingly, the catalyst was easily recovered and reused without loss of catalytic efficiency.
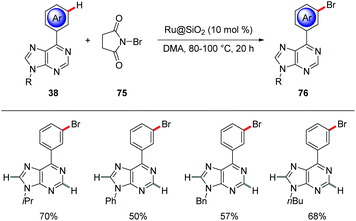 |
| Scheme 26
meta-C–H Bromination using heterogenous ruthenium catalyst. | |
In a very recent study, the same group reported the first recyclable hybrid-ruthenium catalysis for distal meta-C–H activation (Scheme 27).81 It is noteworthy to mention that meta-C–H alkylation was achievable under photoinduced conditions with the hybrid-ruthenium-catalysis manifold.
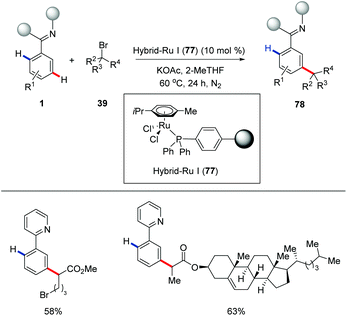 |
| Scheme 27
meta-C–H Activation by recyclable hybrid-ruthenium catalysis. | |
To extend the synthetic utility of the heterogenous catalysts, C–H oxygenation82 and C–H borylation83 were also developed with easily recyclable heterogenous palladium and iridium catalysts respectively.
Overall, significant momentum was gained in C–H activation by heterogenous catalysis to reduce the undesired trace metal impurities, and enable the efficient recycling and reuse of the transition metal catalysts.
Photocatalytic C–H activation
In the last fifteen years, photochemistry has witnessed a renaissance. Thus, photocatalysis was identified as a mild and efficient tool for challenging transformations.84 The merger of photocatalysis with transition metal-catalyzed85 cross coupling unlocked a dormant area of the chemical space.86
The generation of aryl radicals from aryldiazonium salts and subsequent addition of these radicals to various acceptors, in a Meerwein type arylation, is well established.87 In 2011, Sanford merged the versatile palladium-catalyzed C–H activation regime with photocatalytic generation of aryl radical (Scheme 28a).88 The use of Ru(bpy)3Cl2 as an effective photocatalyst for the controlled generation of the aryl radicals under mild reaction conditions was crucial. The ortho-arylation of arenes bearing various directing groups was achieved by means of catalytic amounts of Pd(OAc)2 and Ru(bpy)3Cl2 in MeOH, at room temperature under CFL (compact fluorescent lamp) irradiation.
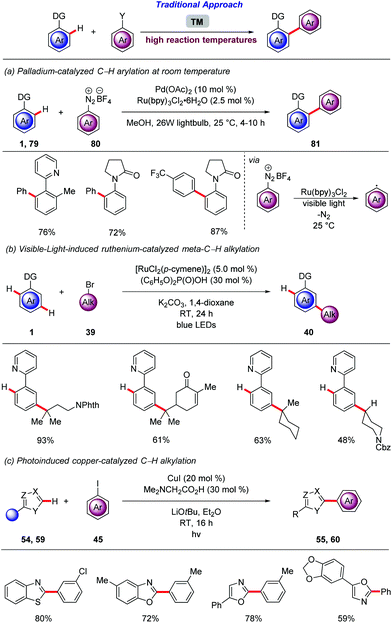 |
| Scheme 28 Redox-neutral photocatalytic C–H activation. | |
The proximity-induced regime for the functionalization of inert C–H bonds is restricted to the ortho position in relation to the directing group. To bypass this limitation many strategies have been developed89 including, use of templates,90 non-covalent interactions between the ligand and the substrate,91 mediators92 for Catellani type reaction93 and σ-activation.94 Generally, these reactions require elevated reaction temperatures that restrict the synthetic utility of those highly sought transformations. Thus, Ackermann demonstrated that the meta-C–H functionalization regime that have already shaped into a powerful strategy for remote functionalization can be further improved by the merger with photocatalysis.95 Thus, the meta-selective C–H alkylation of arenes 1 was achieved under exceedingly mild reactions conditions via visible-light irradiation at room temperature, without the aid of an exogenous photocatalyst (Scheme 28b). After extensive optimization, catalytic amounts of [RuCl2(p-cymene)]2 and (C6H5O)2P(O)OH were found to promote not only the acid-assisted C–H cycloruthenation but also the visible-light induced homolytic cleavage of the alkyl bromides 39. With the optimized conditions in hand, the robustness of the methodology was demonstrated by the ample scope. Remarkably, compared to the previously developed ruthenium-catalyzed meta-C–H that require 100–140 °C, this reaction was conducted at room temperature. Subsequently, Greaney independently reported similar reactivity under blue-light irradiation.96 The same authors exploited the visible-light irradiation for the ruthenium-catalyzed ortho-C–H arylation of heteroarenes. The robust C–H arylation occurred at ambient temperature, enabled by an unprecedented inner-sphere electron transfer from biscyclometalated ruthenacycles.97 In sharp contrast, the differentiation of C–H bonds based on their electronic environment is a common strategy. Thus azoles, bearing a relatively acidic C–H bond have been functionalized, without the assistance of a chelating group, by means of various transition metals under rather forcing reaction conditions.98 Thus, Ackermann reported on the photoinduced copper-catalyzed C–H arylation of azoles 54, 59 at room temperature, using cost-effective CuI (Scheme 28c).99 Detailed optimization studies revealed the essential nature of the N,N-dimethylglycine as a ligand and LiOtBu as a base. The mild nature and robustness of the protocol was reflected by the complete tolerance of various functional groups, the expedient and chemoselective synthesis of alkaloids. Interestingly, challenging oxazolines proved likewise to be viable substrates for this C–H arylation.
The productive merger of photocatalysis with C–H activation is not only restricted to redox-neutral reactions, but can also be expanded to oxidative reactions. The rhodium-catalyzed oxidative alkenylation of arenes bearing various directing groups is well established,100 but stoichiometric amounts of oxidants are required and generally elevated temperatures are employed in order to achieve high conversion.101 Thus, Rueping disclosed a rhodium-catalyzed Fujiwara–Moritani reaction enabled by visible light (Scheme 29a).102 The authors reasoned that the rhodium hydride species produced after β-hydride elimination can be recycled towards the active species by a photocatalytic oxidation, where O2 acts as the terminal oxidant. After extensive optimization, benzamides 8 were efficiently converted to the desired E-olefins with catalytic amounts of [Cp*RhCl2]2, AgSbF6 and [Ru(bpy)3](PF6)2 in chlorobenzene at 80 °C under air. Under the optimized reaction conditions a plethora of functional groups were tolerated on the arene moiety and various alkenes 15 were also employed.103 Subsequently, Rueping and Sundararaju employed this strategy, for high valent cobalt catalysis with photocatalysis (Scheme 29b).104 This approach represents a sustainable protocol with an earth-abundant cobalt catalyst in combination with an organic dye. Indeed, catalytic quantities of Co(acac)2 and Na2Eosin Y in combination with CF3SO2Na in TFE were effective to promote this oxidative cyclisation at room temperature.
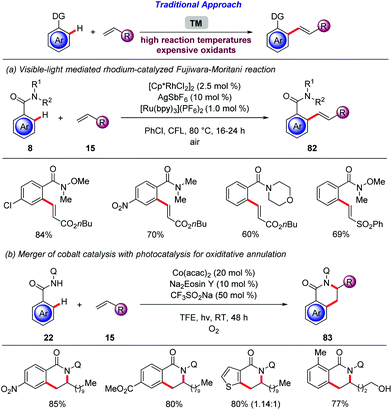 |
| Scheme 29 Oxidative photocatalytic C–H activation. | |
C–H activation in flow
A straightforward transfer of the enormous potential of C–H activation from academia to the industries is through the use of flow technology,105 because flow setups improve heat and mass transfer, are generally safer as batch processes and are well suited for the use of highly reactive intermediates. Moreover, the inherent scalability of flow procedures represents an additional asset.
In this context, Gaunt and Lapkin developed an oxidative palladium-catalyzed C–H aziridination and subsequent ring opening in flow.106 In order to transform the process from the batch conditions,107 to the desired flow setup the authors performed DFT calculations and constructed a predictive kinetic model that enabled the facile transfer to the flow conditions (Scheme 30). Under the optimized reaction conditions consisting of catalytic amounts of Pd(OAc)2 and stoichiometric PhI(OAc)2, Ac2O and AcOH, that was crucial for reducing the concentration of the off-cycle resting state of the catalyst, the desired aziridine 85 was isolated in 90% yield in only 10 minutes. In addition, the authors were able to devise a purification system in flow by connecting one column packed with a palladium scavenger and a second column with an amine scavenger. Flow technology offers unique opportunities for immobilizing a heterogeneous catalyst, enabling the facile reuse of the catalyst. Thus, after developing the directed Fujiwara–Moritani reaction catalyzed by simple palladium on carbon for batch synthesis Ackermann and Vaccaro probed the possibility of immobilizing the heterogeneous catalyst in a coil and performing the reaction in flow.108 Remarkably, their batch conditions consisting of Pd/C, benzoquinone as the oxidant, p-toluenesulfonic acid and GVL as solvent, were easily transferred to the flow setup with the catalyst was immobilised to the coil (Scheme 31). The reaction between anilides 87 and acrylate 15 was run for 29 hours to produce up to 109 g of the alkenylated product. Control experiments demonstrated that the leaching of the palladium catalyst was minimal in GVL (up to 4 ppm) compared to other commonly used polar aprotic solvents such as DMF and NMP, clearly showcasing the enhanced durability of the heterogeneous catalyst in GVL. The high surface-area-to-volume ratio typical of flow reactors is ideal for performing photochemical reactions, since photochemical reaction are very difficult to be scaled up in batch setups, thus photochemical protocols that are developed in flow are more likely to be adopted from industries. To this end, Noël and Van der Eycken reported on the photocatalytic C-2 acylation of indole derivatives 49 under palladium catalysis with aldehydes serving as acyl radical surrogates (Scheme 32).109 The authors optimized the reaction conditions for the acylation in batch, and were delighted to observe that the reaction conditions were compatible for reactions in flow as well, while observing an increased rate. With the optimized conditions in hand, consisting of catalytic amounts of Pd(OAc)2, Boc-Val-OH, fac-[Ir(ppy)3] as photocatalyst and tert-butyl hydroperoxide as terminal oxidant in acetonitrile, they expanded the scope of the acylation in batch and in flow. Interestingly, the isolated yields were comparable but the required 2 hours, which the batch protocol called for 20 hours. In addition, the mild reaction conditions enabled the acylation with α,α-disubstituted aldehydes to occur without any noticeable decarbonylation.
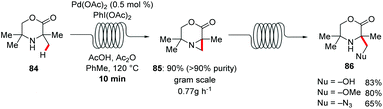 |
| Scheme 30 Continuous-flow synthesis of aziridines. | |
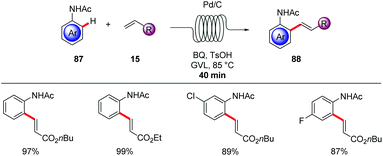 |
| Scheme 31 Heterogeneous C–H alkenylations in continuous flow. | |
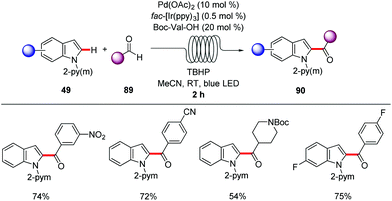 |
| Scheme 32 C–H acylation of indoles by dual photoredox/transition metal catalysis. | |
Despite the fact that the majority of C–H activation protocols has been developed with precious transition metals, C–H activation by 3d-transition metals has gained immense momentum during the last 10 years, due to its cost-effective and less-toxic nature.9,110 Thus, in 2017 Ackermann developed the manganese(I) hydroarylation of propargylic carbonates 91 in flow (Scheme 33a).111 Fine-tuning of the reaction conditions were required to bypass the inherent pathway for the β-oxygen elimination that leads to allene products, gratifyingly, the synergistic catalysis between MnBr(CO)5 and a Brønsted acid promoted the key protodemetalation in favour of the undesired β-oxygen elimination. With the synergistic regime in hand, various arenes and propargylic carbonates were efficiently coupled in only 20 minutes. In addition, metal impurities could be easily removed by implementing a scavenger column in flow. Remarkably, high levels of chemo-, site- and regioselectivities were observed in only 1 minute, albeit at higher temperatures. Whereas, manganese(I)-catalyzed C–H activation offers a wide range of reactivity, due to the fact that the mechanistic pathways are presumably redox-neutral, arylation reactions are still elusive. Hence, in 2018 Ackermann reported on the directed C–H arylation of heteroarenes 93 with the aid of weakly-coordinating amides using the cost effective MnCl2 as catalyst and neocuproine as ligand (Scheme 33b).112 The methodology features the use of a flow-setup in order to minimize safety concerns, since Grignard reagents were used. In addition, improved heat and mass transfer allowed the completion of the reaction in 100 min compared to the batch setup that required 16 h. Importantly, pyridines, pyrimidines and thiophenes were well tolerated showing the potential for medicinal chemistry.
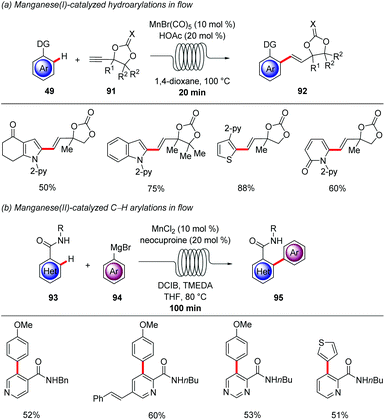 |
| Scheme 33 Manganese-catalyzed C–H activation in flow. | |
Metallaelectro-catalyzed C–H activation
Transition metal-catalyzed C–H activation has emerged as viable tool for molecular synthesis by reducing the formation of undesired byproducts.3 Yet, synthetically attractive oxidative C–H transformations pose significant limitation in terms of oxidant economy.113 The oxidative C–H activation primarily relies on stoichiometric amounts of expensive and toxic chemical oxidants including hypervalent iodine(III), copper(II), or silver(I) salts jeopardizing the overall sustainable nature of the C–H activation approach. While in few cases molecular oxygen has been used as terminal oxidant,114 this imposes additional restriction for its fixed redox potential and major safety hazard with flammable organic solvents.115 Since the pioneering studies of Kolbe116 and Shono,117 organic electrosynthesis118 has undergone a considerable recent renaissance. Particularly, the merger of transition metal catalysis with electrosynthesis11 has enormous potential for the development of environmentally-benign diverse C–H functionalizations to form C–C or C–Het bonds using electrons as formal redox reagent for the re-oxidation of the metal centre. Thereby, super stoichiometric amounts of chemical oxidants can be avoided, thus enabling lower E-factors8 for resource economical synthesis.118d,i,119
In this context, Amatore and Jutand achieved the electrochemical palladium(II)-catalyzed C–H bond olefination of N-acetylanilines 96 in AcOH in a divided cell set-up. Co-catalytic amounts of p-benzoquinone were needed as a redox mediator which can be regenerated at the anode to recycle the palladium(II) species in the catalytic cycle (Scheme 34a).120
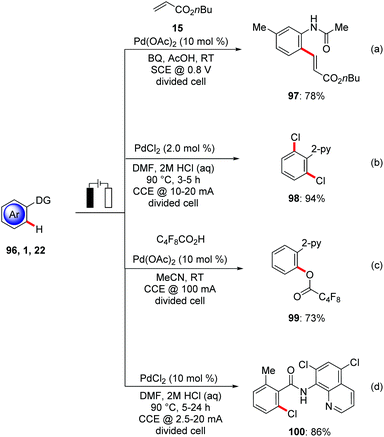 |
| Scheme 34 Palladaelectro-catalyzed C–H activation. | |
Palladium-catalyzed electrochemical oxidations with HX were accomplished by Kakiuchi.121 It is worth noting that in this protocol electricity was primarily responsible for the formation of the electrophilic Cl+ species (Scheme 34b). This protocol enhanced the atom-economy, since the lengthy and costly use of electrophilic halogenation reagents can be circumvented. Later, a related C–H perfluoroalkoxylation of phenyl pyridines 1 was achieved with perfluroalkylated acids by Budnikova (Scheme 34c).122
In a recent study, Kakiuchi used the bidentate chlorinated 8-aminoquinoline directing group for the electrochemical ortho-selective chlorination of electronically poor benzamides 22 for the synthesis of Vismodegib (Scheme 34d).123
In subsequent studies, Mei developed for the first time palladium-catalyzed C(sp3)–H oxygenation of oxime derivatives 101 using anodic oxidation instead of stoichiometric amounts of toxic metal oxidants. The combination of catalytic amounts of Pd(OAc)2, carboxylic acids and their corresponding sodium salts gave optimal results again in a divided cell set-up (Scheme 35a).124 Other commonly employed chemical oxidants, such as PhI(OAc)2, t-BuOOAc, or NaNO3/O2, provided the desired product 102 in significantly lower yields, highlighting the unique potential of electricity as green terminal oxidant. Based on their initial findings, Mei reported the palladium-catalyzed oxidative C(sp2)–H methylation 105 and benzoylation 107 of oximes with methyltrifluoroborates 104 and benzoyl acetic acids 106 respectively (Scheme 35b and c).125
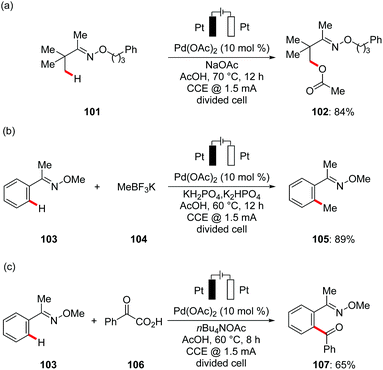 |
| Scheme 35 Palladaelectro-catalyzed C–H activation using oxime as directing group. | |
All palladaelectrocatalytic C–H transformations require a strong N-coordination and a divided cell set up. However, organic electrochemical C–H activation is not limited to the strong nitrogen-centred directing groups. In recent years, ruthenium,126 rhodium127 and iridium128 catalysts have enabled the efficient electrooxidative C–H transformations with weakly coordinating substrates. Very recently, Ackermann reported on the first transition metal-catalyzed electrocatalytic organometallic C–H activation via weakly co-ordinating oxygen based carboxylic acids.126h Thus, an inexpensive ruthenium(II) catalyst in combination with NaOPiv, enabled the C–H/O–H functionalization of synthetically meaningful benzoic acids 32 for alkyne 5 annulations with high levels of efficacy, thereby avoiding the use of sacrificial metal oxidants, namely Cu(OAc)2·H2O129 (Scheme 36a).
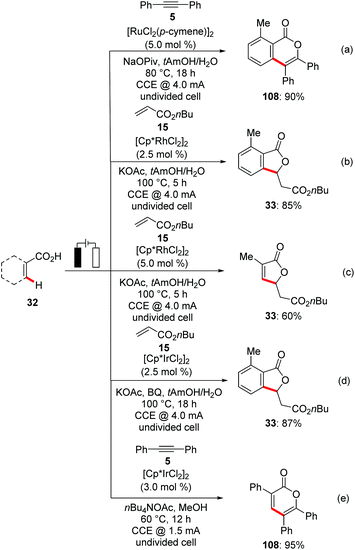 |
| Scheme 36 Metallaelectro-catalyzed C–H/O–H annulations with acids. | |
Along the same lines, Ackermann devised an unprecedented rhodium(III)-catalyzed electrooxidative C–H/O–H alkenylation between benzoic acids 32 and acrylates 15via weakly co-ordinating O-chelation with H2 as the sole by-product (Scheme 36b and c).127f
Furthermore, the same group demonstrated the first electrocatalytic iridium-catalyzed C–H/O–H annulation of benzoic acids 32 and acrylates 15.128b Catalytic amounts of p-benzoquinone were exploited as a redox catalyst to increase the turnover number (Scheme 36d). In a recent study Mei extended the iridium-catalyzed electrochemical vinylic C–H/O–H annulation with alkynes 5 and synthetically useful acrylic acids to afford α-pyrones 108 in good to excellent yields (Scheme 36e).128a The authors showed that under otherwise identical reaction conditions, commonly used chemical oxidants in combination with cobalt or ruthenium catalysis yielded poor regioselectivities with unsymmetrical internal alkynes, which demonstrates the advantage of this strategy over previously reported annulation protocols.129,130
While electrocatalysis has largely relied on precious and toxic 4d and 5d transition metals, in recent years, momentum has been gained by identifying Earth-abundant and less-toxic 3d metals as viable catalysts for molecular C–H transformations.131
In 2017, Ackermann reported on the first electrochemical C–H activation by Earth-abundant metal catalysis.132 Here, the authors employed Cp*-free Co(OAc)2·4H2O as an inexpensive precatalyst for the electrochemical C–H oxygenation of benzamides 111 with alcohols (Scheme 37a). The reaction proceeded with a broad range of substrates and high levels of functional group tolerance at room temperature. In contrast, previous studies by Song and Niu required high reaction temperatures in the presence of stoichiometric amounts of silver(I) chemical oxidants.133 Intrigued by this report, the electrooxidative134 direct C–H amination of otherwise inert C–H bonds of aromatic benzamides 32 was subsequently reported.135 Ackermann hence demonstrated for the use of biomass-derived, renewable solvent GVL in electrocatalysis for the amination of benzamides 109 with secondary amines 112 at a low temperature of 40 °C with H2 as the stoichiometric byproduct by cathodic reduction (Scheme 37b), representing a notable advancement towards sustainability, since previous amination protocols were largely restricted to the use of superstoichiometric chemical oxidant in organic solvent at higher temperature.136 Concurrently, Lei utilized 8-AQ as directing group for the C–H amination with cyclic secondary amines 112 in acetonitrile media at an elevated reaction temperature of 65 °C (Scheme 37c).137 The Ackermann group realized an electrochemical annulation of C–H and N–H bond with alkynes 5 using electricity as the sole oxidant (Scheme 37d).138 This environmentally-benign cobalt catalysis was performed in non-toxic H2O as the reaction medium at room temperature. Benzamides, heterocycles and alkenes bearing pyridine N-oxide were found as amenable substrates for the electro-chemical annulation to gain access to isoquinolone 115 motifs. Later along these lines, Lei extended this approach towards the C–H/N–H annulation of ethylene and ethyne by amides 32 (Scheme 37e).139 The electrochemical C–H/N–H annulation was not limited to terminal alkynes. Ackermann rationalized electro-oxidative internal alkyne annulation with electro-reductive removable benzhydrazide 110, demonstrating the unique potential of electricity (Scheme 37f).140 It is noteworthy that the authors employed an electroreductive hydrazide cleavage approach by the use of catalytic amounts of SmI2 to cleave the benzhydrazides in a traceless manner. Considering the unique key structural motifs of allenes, Ackermann delineated the first direct use of allenes 118 for electrochemical C–H functionalization.141 An unprecedented electrochemical cobalt-catalyzed C–H activation with allenes 118 was realized with high regio- and site-selectivity under exceedingly mild reaction conditions in the absence of toxic chemical oxidants (Scheme 37g). Later, the oxidative C–H/N–H carbonylation with carbon monoxide as a readily available inexpensive C1 building block proved viable. In addition to carbon monoxide, Ackermann likewise showed the versatility of cobalt catalysis by using synthetically useful isocynaides (Scheme 37h).142 Mei and Ackermann disclosed C–H/N–H activations of hydrazides 110 with 1,3-diynes 5 using a robust earth-abundant cobalt catalyst to furnish the desired products 117 in good to excellent yields (Scheme 37i).143 In a very recent study by the Ackermann group the potential of cobalt catalysts in an electrocatalytic C–H allylation of benzamides 32 with nonactivated alkenes 15 was disclosed in bio-mass derived green solvent GVL (Scheme 37j).144 In stark contrast, previous methods for C–H allylations were limited to the use of silver(I) chemical oxidants in organic solvents, jeapordizing the innate sustainability of C–H activation approach.145
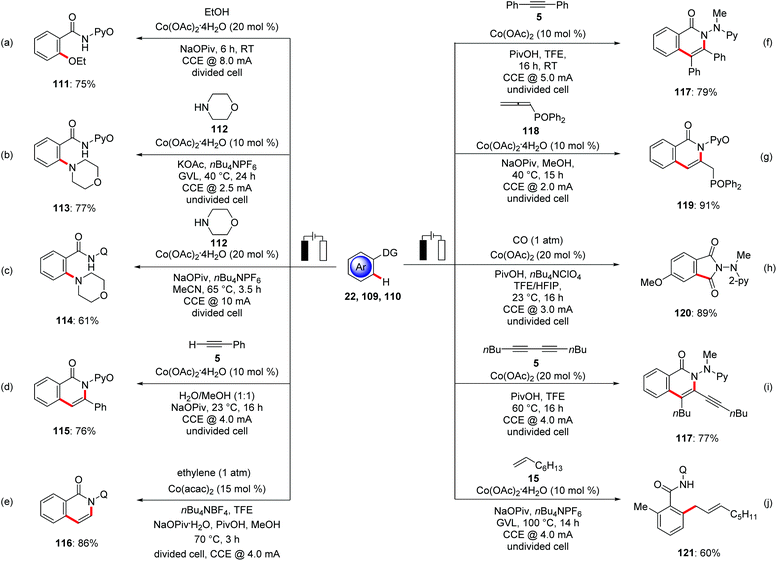 |
| Scheme 37 Cobaltaelectro-catalyzed C–H activation. | |
In a recent proof-of-concept study, Ackermann demonstrated the utilization of renewable solar and wind energy for electrocatalytic oxidative C–H activations. As a model study, the authors performed the cobaltaelectro-catalyzed C–H/N–H annulation of amide 109 with alkyne 5 in biomass derived glycerol as reaction media. The reaction was powered by either a commercially available photovoltaic module or a commercially available wind turbine to drive to the desired transformation (Scheme 38).146
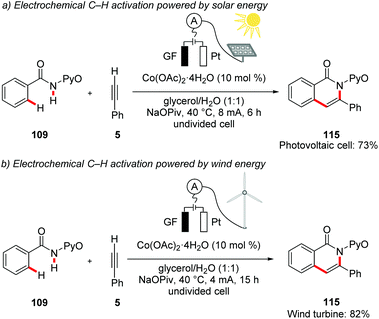 |
| Scheme 38 Electrochemical C–H activation powered by renewable electricity. | |
With notable progress in Earth-abundant cobalt catalysis for sustainable electrocatalysis, there has been strong interest in further developing metallaelectro-catalyzed C–H transformations. Ackermann devised less toxic, cost-effective nickel complexes as efficient catalysts for the electrochemical C–H activations.147 In 2018, Ackermann reported first electrochemical nickel-catalyzed C–H amination of benzamides 22 (Scheme 39a).148 The nickelaelectro-catalyzed chemo- and position-selective amination proceeded with a broad range of functional group tolerance. Along this line, the same group reported on nickelaelectro-oxidative C–H alkoxylations with challenging secondary alcohols 122 with H2 as the sole by product (Scheme 39b).149 It is worth noting that the chemical oxidants AgOAc, Cu(OAc)2, molecular oxygen, PhI(OAc)2, or K2S2O8 provided significantly lower yield of the desired product. Detailed mechanistic studies revealed an oxidation-induced reductive elimination at a nickel(III) center. Furthermore, the nickel-catalyzed C–H alkylation of benzamide 22 was achieved with broad functional group tolerance at room temperature in user-friendly undivided cell setup (Scheme 39c).150 In contrast, previous studies were largely limited to the use of strong bases and considerably higher reaction temperatures for nickel-catalyzed C–H alkylations.151
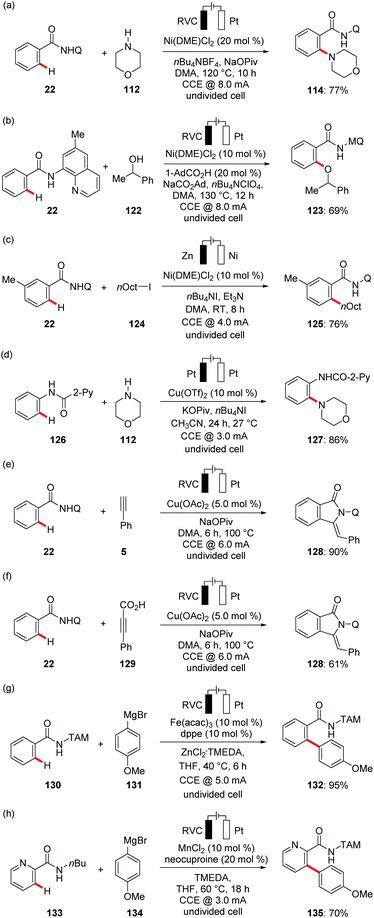 |
| Scheme 39 3d metal-catalyzed C–H activation. | |
Recently, Mei exploited copper catalysis for the electrochemical C–H aminations of electron-rich anilides 126 with electricity as terminal oxidant (Scheme 39d).152 In a related work, Nicholls and coworkers reported electro-oxidative amination of anilides with amines producing H2 as the sole byproduct.153 Concurrently, the Ackermann group disclosed cupraelectro-catalyzed C–H alkynylations of electron-rich and electron-deficient benzamides 22 for the synthesis of synthetically meaningful isoindolones 128 (Scheme 39e).154 Notably, the authors also demonstrated C–H/C–C functionalizations in a decarboxylative fashion with alkynyl carboxylic acids to afford the desired isoindolone 128 products (Scheme 39f).
Recently, iron catalysis has found considerable applications in molecular synthesis due to its low cost, low toxicity and as the most naturally abundant transition metal on Earth.155 Despite the recent attention iron-catalyzed C–H activation has gained, superstoichoimetric use of corrosive, expensive chemical oxidants pose a major limitation to organometallic iron catalysis.156 To overcome these challenges, Ackermann reported the unprecedented ferraelectro-catalyzed C–H arylations at mild temperature using electricity as a green oxidant (Scheme 39g).157 Interestingly, the electro-oxidative arylations proceeded efficiently in the biomass-derived solvent 2-MeTHF. Ackermann enhanced the versatility of metallaelectrocatalysis by the merger of electrocatalysis with environmentally-benign manganese catalysis. Non-toxic MnCl2 was utilized for the electrochemical C–H arylation of amides 133 in the absence of any zinc additives (Scheme 39f).157
Despite significant advances of metalla-electrocatalysis, reports on enantioselective electrochemical transformations are scarce.158 Recently, the Ackermann group reported on the first asymmetric metallaelectro-catalyzed C–H activation.159 The authors disclosed the unprecedented use of transient directing groups for the asymmetric palladaelectro-catalyzed C–H olefinations for the synthesis of enantiomerically-enriched axially chiral biaryls and heterobiaryls 137 scaffolds under mild conditions (Scheme 40). Late-stage diversification of the products enabled the synthesis of highly enantioenriched BINOLs, dicarboxylic acids and helicenes.
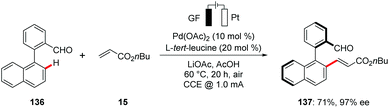 |
| Scheme 40 Atroposelective palladaelectro-catalyzed C–H activation. | |
Conclusions
During the last decade transition metal-catalyzed C–H activation has emerged as a transformative platform for molecular syntheses. However, despite significant advances in C–H activations, the quest for sustainable and environmentally-benign strategies continues to be of central importance for resource economical C–H activations. Herein, we have highlighted key aspects for eco-friendly metal-catalyzed C–H activation (Scheme 41). The strategies discussed herein provide guidance towards more sustainable C–H transformations. Each approach, by itself, does not necessarily ensure ideal greenness, but their combination will lead to improved levels of sustainability. Namely, the following five key trends have been summarized: (1) the use of biomass-derived solvents is an important aspect to reduce the consumption of toxic organic solvents. This is particularly the case in industrial scale, since typical isolation methods, such as column chromatography generally employed in academic settings, are replaced by more sustainable methods. Particularly, in many cases similar trends have been observed for GVL compared to commonly employed polar aprotic solvents, such as DMF, DMA, and NMP. Similarly, biomass-derived 2-MeTHF is a safer alternative to flammable THF in many C–H activation processes. These empirical observations can encourage the practitioner to use these class of solvents more frequently. (2) The facile recyclability of the catalyst is another key aspect, potentially even for large scale applications. In recent years, heterogenous catalysts have emerged for various C–C and C–heteroatom bond forming reactions with efficient recycling of the catalysts. (3) Furthermore, the merger of transition metal catalysis with photocatalysis has considerably enhanced the reactivity under exceedingly mild conditions. (4) The recent use of flow technologies for metal-catalyzed C–H activation reactions has shown enormous potential for the straightforward scale-up with safer setups and shorter reaction times. (5) Last but not least, metallaelectrocatalysis avoids the use of toxic chemical oxidants or metal promoters by electricity as traceless inexpensive oxidant, representing a significant advance for resource-economical C–H activations. Very recently, metallaelectrocatalysis has been realized with 3d metal catalysts as well as in green reaction medium, showcasing the high sustainability of the process. In view of current metal-catalyzed C–H activation portfolio, it is evident that the exponential growth for sustainable strategies in organic synthesis has gained considerable attention for resource economical transformations. Given the topical interest in resource-economical C–H activation, future exciting advances are expected in this rapidly evolving research arena, including, enantioselective heterogeneous catalysis and Earth-abundant electrocatalysis.
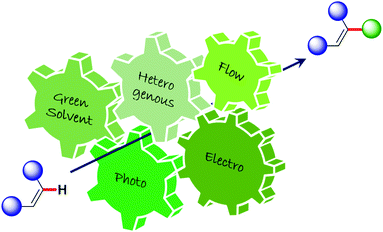 |
| Scheme 41 Green strategies for sustainable C–H activation. | |
Conflicts of interest
There are no conflicts to declare.
Acknowledgements
Generous support by the DFG (Gottfried-Wilhelm-Leibniz award), the DAAD (fellowship to U. D.) and the Onassis Foundation (fellowship to N. K.) is gratefully acknowledged.
Notes and references
-
(a) R. A. Sheldon, Metrics of Green Chemistry and Sustainability: Past, Present, and Future, ACS Sustainable Chem. Eng., 2018, 6, 32–48 Search PubMed;
(b) P. A. Wender, Toward the ideal synthesis and molecular function through synthesis-informed design, Nat. Prod. Rep., 2014, 31, 433–440 Search PubMed;
(c) C. C. C. Johansson Seechurn, M. O. Kitching, T. J. Colacot and V. Snieckus, Palladium-Catalyzed Cross-Coupling: A Historical Contextual Perspective to the 2010 Nobel Prize, Angew. Chem., Int. Ed., 2012, 51, 5062–5085 Search PubMed;
(d) P. T. Anastas and M. M. Kirchhoff, Origins, Current Status, and Future Challenges of Green Chemistry, Acc. Chem. Res., 2002, 35, 686–694 Search PubMed;
(e) B. M. Trost, Atom Economy—A Challenge for Organic Synthesis: Homogeneous Catalysis Leads the Way, Angew. Chem., Int. Ed. Angl., 1995, 34, 259–281 Search PubMed;
(f) B. M. Trost, The atom economy–a search for synthetic efficiency, Science, 1991, 254, 1471–1477 Search PubMed.
-
(a) A. Suzuki, Cross-Coupling Reactions Of Organoboranes: An Easy Way To Construct C–C Bonds (Nobel Lecture), Angew. Chem., Int. Ed., 2011, 50, 6722–6737 Search PubMed;
(b) E.-i. Negishi, Magical Power of Transition Metals: Past, Present, and Future (Nobel Lecture), Angew. Chem., Int. Ed., 2011, 50, 6738–6764 Search PubMed;
(c) A. Zapf and M. Beller, The development of efficient catalysts for palladium-catalyzed coupling reactions of aryl halides, Chem. Commun., 2005, 431–440 Search PubMed;
(d) K. C. Nicolaou, P. G. Bulger and D. Sarlah, Palladium-Catalyzed Cross-Coupling Reactions in Total Synthesis, Angew. Chem., Int. Ed., 2005, 44, 4442–4489 Search PubMed;
(e)
A. de Meijere and F. Diederich, Metal–Catalyzed Cross–Coupling Reactions, WILEY-VCH, 2nd edn, 2004 Search PubMed.
-
(a) S. Rej, Y. Ano and N. Chatani, Bidentate Directing Groups: An Efficient Tool in C–H Bond Functionalization Chemistry for the Expedient Construction of C–C Bonds, Chem. Rev., 2020, 120, 1788–1887 Search PubMed;
(b) A. Dey, S. K. Sinha, T. K. Achar and D. Maiti, Accessing Remote meta- and para-C(sp2)−H Bonds with Covalently Attached Directing Groups, Angew. Chem., Int. Ed., 2019, 58, 10820–10843 Search PubMed;
(c) Y. Xu and G. Dong, sp3 C–H activation via exo-type directing groups, Chem. Sci., 2018, 9, 1424–1432 Search PubMed;
(d) C. Sambiagio, D. Schönbauer, R. Blieck, T. Dao-Huy, G. Pototschnig, P. Schaaf, T. Wiesinger, M. F. Zia, J. Wencel-Delord, T. Besset, B. U. W. Maes and M. Schnürch, A comprehensive overview of directing groups applied in metal-catalysed C–H functionalisation chemistry, Chem. Soc. Rev., 2018, 47, 6603–6743 Search PubMed;
(e) P. Gandeepan and L. Ackermann, Transient Directing Groups for Transformative C–H Activation by Synergistic Metal Catalysis, Chem, 2018, 4, 199–222 Search PubMed;
(f) H. Yi, G. Zhang, H. Wang, Z. Huang, J. Wang, A. K. Singh and A. Lei, Recent Advances in Radical C–H Activation/Radical Cross-Coupling, Chem. Rev., 2017, 117, 9016–9085 Search PubMed;
(g) Y. Park, Y. Kim and S. Chang, Transition Metal-Catalyzed C–H Amination: Scope, Mechanism, and Applications, Chem. Rev., 2017, 117, 9247–9301 Search PubMed;
(h) J. R. Hummel, J. A. Boerth and J. A. Ellman, Transition-Metal-Catalyzed C–H Bond Addition to Carbonyls, Imines, and Related Polarized π Bonds, Chem. Rev., 2017, 117, 9163–9227 Search PubMed;
(i) J. He, M. Wasa, K. S. L. Chan, Q. Shao and J.-Q. Yu, Palladium-Catalyzed Transformations of Alkyl C–H Bonds, Chem. Rev., 2017, 117, 8754–8786 Search PubMed;
(j) H. M. L. Davies and D. Morton, Collective Approach to Advancing C–H Functionalization, ACS Cent. Sci., 2017, 3, 936–943 Search PubMed;
(k) T. Gensch, M. N. Hopkinson, F. Glorius and J. Wencel-Delord, Mild metal-catalyzed C–H activation: examples and concepts, Chem. Soc. Rev., 2016, 45, 2900–2936 Search PubMed;
(l) P. Gandeepan and C.-H. Cheng, Transition-Metal-Catalyzed π-Bond-Assisted C–H Bond Functionalization: An Emerging Trend in Organic Synthesis, Chem. – Asian J., 2015, 10, 824–838 Search PubMed;
(m) O. Daugulis, J. Roane and L. D. Tran, Bidentate, Monoanionic Auxiliary-Directed Functionalization of Carbon–Hydrogen Bonds, Acc. Chem. Res., 2015, 48, 1053–1064 Search PubMed;
(n) C. S. Yeung and V. M. Dong, Catalytic Dehydrogenative Cross-Coupling: Forming Carbon−Carbon Bonds by Oxidizing Two Carbon−Hydrogen Bonds, Chem. Rev., 2011, 111, 1215–1292 Search PubMed;
(o) L. Ackermann, Carboxylate-Assisted Transition-Metal-Catalyzed C−H Bond Functionalizations: Mechanism and Scope, Chem. Rev., 2011, 111, 1315–1345 Search PubMed;
(p) I. A. I. Mkhalid, J. H. Barnard, T. B. Marder, J. M. Murphy and J. F. Hartwig, C−H Activation for the Construction of C−B Bonds, Chem. Rev., 2010, 110, 890–931 Search PubMed;
(q) T. W. Lyons and M. S. Sanford, Palladium-Catalyzed Ligand-Directed C−H Functionalization Reactions, Chem. Rev., 2010, 110, 1147–1169 Search PubMed;
(r) A. Gunay and K. H. Theopold, C−H Bond Activations by Metal Oxo Compounds, Chem. Rev., 2010, 110, 1060–1081 Search PubMed;
(s) D. A. Colby, R. G. Bergman and J. A. Ellman, Rhodium-Catalyzed C−C Bond Formation via Heteroatom-Directed C−H Bond Activation, Chem. Rev., 2010, 110, 624–655 Search PubMed;
(t) L. Ackermann, R. Vicente and A. R. Kapdi, Transition-Metal-Catalyzed Direct Arylation of (Hetero)Arenes by C–H Bond Cleavage, Angew. Chem., Int. Ed., 2009, 48, 9792–9826 Search PubMed;
(u) R. G. Bergman, C–H activation, Nature, 2007, 446, 391–393 Search PubMed;
(v) L. Ackermann, Catalytic Arylations with Challenging Substrates: From Air-Stable HASPO Preligands to Indole Syntheses and C-H-Bond Functionalizations, Synlett, 2007, 507–526 Search PubMed.
-
(a) E. R. Welin, A. Ngamnithiporn, M. Klatte, G. Lapointe, G. M. Pototschnig, M. S. J. McDermott, D. Conklin, C. D. Gilmore, P. M. Tadross, C. K. Haley, K. Negoro, E. Glibstrup, C. U. Grünanger, K. M. Allan, S. C. Virgil, D. J. Slamon and B. M. Stoltz, Concise total syntheses of (–)-jorunnamycin A and (–)-jorumycin enabled by asymmetric catalysis, Science, 2019, 363, 270 Search PubMed;
(b) A. Çapcı, M. M. Lorion, H. Wang, N. Simon, M. Leidenberger, M. C. Borges Silva, D. R. M. Moreira, Y. Zhu, Y. Meng, J. Y. Chen, Y. M. Lee, O. Friedrich, B. Kappes, J. Wang, L. Ackermann and S. B. Tsogoeva, Artemisinin–(Iso)quinoline Hybrids by C−H Activation and Click Chemistry: Combating Multidrug-Resistant Malaria, Angew. Chem., Int. Ed., 2019, 58, 13066–13079 Search PubMed.
-
(a) J. Börgel and T. Ritter, Late-Stage Functionalization, Chem, 2020, 6, 1877–1887 Search PubMed;
(b) W. Wang, M. M. Lorion, J. Shah, A. R. Kapdi and L. Ackermann, Late-Stage Peptide Diversification by Position-Selective C−H Activation, Angew. Chem., Int. Ed., 2018, 57, 14700–14717 Search PubMed;
(c) A. F. M. Noisier and M. A. Brimble, C–H Functionalization in the Synthesis of Amino Acids and Peptides, Chem. Rev., 2014, 114, 8775–8806 Search PubMed.
-
(a) J.-R. Pouliot, F. Grenier, J. T. Blaskovits, S. Beaupré and M. Leclerc, Direct (Hetero)arylation Polymerization: Simplicity for Conjugated Polymer Synthesis, Chem. Rev., 2016, 116, 14225–14274 Search PubMed;
(b) D. J. Schipper and K. Fagnou, Direct Arylation as a Synthetic Tool for the Synthesis of Thiophene-Based Organic Electronic Materials, Chem. Mater., 2011, 23, 1594–1600 Search PubMed.
-
(a) S. D. Friis, M. J. Johansson and L. Ackermann, Cobalt-catalysed C–H methylation for late-stage drug diversification, Nat. Chem., 2020, 12, 511–519 Search PubMed;
(b) D. C. Blakemore, L. Castro, I. Churcher, D. C. Rees, A. W. Thomas, D. M. Wilson and A. Wood, Organic synthesis provides opportunities to transform drug discovery, Nat. Chem., 2018, 10, 383–394 Search PubMed;
(c) M. Seki, A New Catalytic System for Ru-Catalyzed C–H Arylation Reactions and Its Application in the Practical Syntheses of Pharmaceutical Agents, Org. Process Res. Dev., 2016, 20, 867–877 Search PubMed;
(d) T. Cernak, K. D. Dykstra, S. Tyagarajan, P. Vachal and S. W. Krska, The medicinal chemist's toolbox for late stage functionalization of drug-like molecules, Chem. Soc. Rev., 2016, 45, 546–576 Search PubMed;
(e) L. Ackermann, Robust Ruthenium(II)-Catalyzed C–H Arylations: Carboxylate Assistance for the Efficient Synthesis of Angiotensin-II-Receptor Blockers, Org. Process Res. Dev., 2015, 19, 260–269 Search PubMed.
- R. A. Sheldon, The E factor 25 years on: the rise of green chemistry and sustainability, Green Chem., 2017, 19, 18–43 Search PubMed.
-
(a) S. M. Khake and N. Chatani, Nickel-Catalyzed C−H Functionalization Using A Non-directed Strategy, Chem, 2020, 6, 1056–1081 Search PubMed;
(b) Ł. Woźniak and N. Cramer, Enantioselective CH Bond Functionalizations by 3d Transition-Metal Catalysts, Trends Chem., 2019, 1, 471–484 Search PubMed;
(c) J. Loup, U. Dhawa, F. Pesciaioli, J. Wencel-Delord and L. Ackermann, Enantioselective C−H Activation with Earth-Abundant 3d Transition Metals, Angew. Chem., Int. Ed., 2019, 58, 12803–12818 Search PubMed;
(d) S. M. Khake and N. Chatani, Chelation-Assisted Nickel-Catalyzed C–H Functionalizations, Trends Chem., 2019, 1, 524–539 Search PubMed;
(e) P. Gandeepan, T. Müller, D. Zell, G. Cera, S. Warratz and L. Ackermann, 3d Transition Metals for C–H Activation, Chem. Rev., 2019, 119, 2192–2452 Search PubMed;
(f) P. M. Edwards and L. L. Schafer, Early transition metal-catalyzed C–H alkylation: hydroaminoalkylation for Csp3−Csp3 bond formation in the synthesis of selectively substituted amines, Chem. Commun., 2018, 54, 12543–12560 Search PubMed;
(g) T. Yoshino and S. Matsunaga, (Pentamethylcyclopentadienyl)cobalt(III)-Catalyzed C–H Bond Functionalization: From Discovery to Unique Reactivity and Selectivity, Adv. Synth. Catal., 2017, 359, 1245–1262 Search PubMed;
(h) M. Moselage, J. Li and L. Ackermann, Cobalt-Catalyzed C–H Activation, ACS Catal., 2016, 6, 498–525 Search PubMed;
(i) W. Liu and L. Ackermann, Manganese-Catalyzed C–H Activation, ACS Catal., 2016, 6, 3743–3752 Search PubMed;
(j) G. Cera and L. Ackermann, Iron-Catalyzed C–H Functionalization Processes, Top. Curr. Chem., 2016, 374, 57 Search PubMed;
(k) K. Gao and N. Yoshikai, Low-Valent Cobalt Catalysis: New Opportunities for C–H Functionalization, Acc. Chem. Res., 2014, 47, 1208–1219 Search PubMed;
(l) C.-L. Sun, B.-J. Li and Z.-J. Shi, Direct C−H Transformation via Iron Catalysis, Chem. Rev., 2011, 111, 1293–1314 Search PubMed;
(m) A. A. Kulkarni and O. Daugulis, Direct Conversion of Carbon-Hydrogen into Carbon-Carbon Bonds by First-Row Transition-Metal Catalysis, Synthesis, 2009, 4087–4109 Search PubMed.
-
(a) J. L. Howard, Q. Cao and D. L. Browne, Mechanochemistry as an emerging tool for molecular synthesis: what can it offer?, Chem. Sci., 2018, 9, 3080–3094 Search PubMed;
(b) C. Bolm and J. G. Hernández, From Synthesis of Amino Acids and Peptides to Enzymatic Catalysis: A Bottom-Up Approach in Mechanochemistry, ChemSusChem, 2018, 11, 1410–1420 Search PubMed;
(c) N. A. Romero and D. A. Nicewicz, Organic Photoredox Catalysis, Chem. Rev., 2016, 116, 10075–10166 Search PubMed;
(d) M. D. Kärkäs, J. A. Porco and C. R. J. Stephenson, Photochemical Approaches to Complex Chemotypes: Applications in Natural Product Synthesis, Chem. Rev., 2016, 116, 9683–9747 Search PubMed.
- T. H. Meyer, I. Choi, C. Tian and L. Ackermann, Powering the Future: How Can Electrochemistry Make a Difference in Organic Synthesis?, Chem, 2020, 6, 2484–2496 Search PubMed.
-
(a) C. J. Clarke, W.-C. Tu, O. Levers, A. Bröhl and J. P. Hallett, Green and Sustainable Solvents in Chemical Processes, Chem. Rev., 2018, 118, 747–800 Search PubMed;
(b) C. Jimenez-Gonzalez, C. S. Ponder, Q. B. Broxterman and J. B. Manley, Using the Right Green Yardstick: Why Process Mass Intensity Is Used in the Pharmaceutical Industry To Drive More Sustainable Processes, Org. Process Res. Dev., 2011, 15, 912–917 Search PubMed.
-
(a) L. Soh and M. J. Eckelman, Green Solvents in Biomass Processing, ACS Sustainable Chem. Eng., 2016, 4, 5821–5837 Search PubMed;
(b) F. P. Byrne, S. Jin, G. Paggiola, T. H. M. Petchey, J. H. Clark, T. J. Farmer, A. J. Hunt, C. Robert McElroy and J. Sherwood, Tools and techniques for solvent selection: green solvent selection guides, Sustainable Chem. Processes, 2016, 4, 7 Search PubMed;
(c) M. Tobiszewski, S. Tsakovski, V. Simeonov, J. Namieśnik and F. Pena-Pereira, A solvent selection guide based on chemometrics and multicriteria decision analysis, Green Chem., 2015, 17, 4773–4785 Search PubMed;
(d) D. Prat, O. Pardigon, H.-W. Flemming, S. Letestu, V. Ducandas, P. Isnard, E. Guntrum, T. Senac, S. Ruisseau, P. Cruciani and P. Hosek, Sanofi's Solvent Selection Guide: A Step Toward More Sustainable Processes, Org. Process Res. Dev., 2013, 17, 1517–1525 Search PubMed;
(e) L. Moity, M. Durand, A. Benazzouz, C. Pierlot, V. Molinier and J.-M. Aubry, Panorama of sustainable solvents using the COSMO-RS approach, Green Chem., 2012, 14, 1132–1145 Search PubMed;
(f) P. J. Dunn, The importance of Green Chemistry in Process Research and Development, Chem. Soc. Rev., 2012, 41, 1452–1461 Search PubMed;
(g) P. G. Jessop, Searching for green solvents, Green Chem., 2011, 13, 1391–1398 Search PubMed;
(h) K. Alfonsi, J. Colberg, P. J. Dunn, T. Fevig, S. Jennings, T. A. Johnson, H. P. Kleine, C. Knight, M. A. Nagy, D. A. Perry and M. Stefaniak, Green chemistry tools to influence a medicinal chemistry and research chemistry based organisation, Green Chem., 2008, 10, 31–36 Search PubMed.
-
(a) T. Dalton, T. Faber and F. Glorius, C–H Activation: Toward Sustainability and Applications, ACS Cent. Sci., 2021, 7, 245–261 Search PubMed;
(b) S. Santoro, A. Marrocchi, D. Lanari, L. Ackermann and L. Vaccaro, Towards Sustainable C−H Functionalization Reactions: The Emerging Role of Bio-Based Reaction Media, Chem. – Eur. J., 2018, 24, 13383–13390 Search PubMed;
(c) C. M. Alder, J. D. Hayler, R. K. Henderson, A. M. Redman, L. Shukla, L. E. Shuster and H. F. Sneddon, Updating and further expanding GSK's solvent sustainability guide, Green Chem., 2016, 18, 3879–3890 Search PubMed;
(d) C. Fischmeister and H. Doucet, Greener solvents for ruthenium and palladium-catalysed aromatic C–H bond functionalisation, Green Chem., 2011, 13, 741–753 Search PubMed;
(e) R. K. Henderson, C. Jiménez-González, D. J. C. Constable, S. R. Alston, G. G. A. Inglis, G. Fisher, J. Sherwood, S. P. Binks and A. D. Curzons, Expanding GSK's solvent selection guide – embedding sustainability into solvent selection starting at medicinal chemistry, Green Chem., 2011, 13, 854–862 Search PubMed;
(f) D. Prat, A. Wells, J. Hayler, H. Sneddon, C. R. McElroy, S. Abou-Shehada and P. J. Dunn, CHEM21 selection guide of classical- and less classical-solvents, Green Chem., 2016, 18, 288–296 Search PubMed;
(g) J. H. Clark and S. J. Tavener, Alternative Solvents: Shades of Green, Org. Process Res. Dev., 2007, 11, 149–155 Search PubMed;
(h) L. J. Diorazio, D. R. J. Hose and N. K. Adlington, Toward a More Holistic Framework for Solvent Selection, Org. Process Res. Dev., 2016, 20, 760–773 Search PubMed.
-
(a) F. G. Calvo-Flores, M. J. Monteagudo-Arrebola, J. A. Dobado and J. Isac-García, Green and Bio-Based Solvents, Top. Curr. Chem., 2018, 376, 18 Search PubMed;
(b) M. Sarmah, M. Mondal and U. Bora, Agro-Waste Extract Based Solvents: Emergence of Novel Green Solvent for the Design of Sustainable Processes in Catalysis and Organic Chemistry, ChemistrySelect, 2017, 2, 5180–5188 Search PubMed;
(c) S. Santoro, F. Ferlin, L. Luciani, L. Ackermann and L. Vaccaro, Biomass-derived solvents as effective media for cross-coupling reactions and C–H functionalization processes, Green Chem., 2017, 19, 1601–1612 Search PubMed;
(d) G. C. Arlene, W. P. Márcio and S. S. Ricardo, Application of Bio-Based Solvents in Catalysis, Curr. Org. Synth., 2015, 12, 675–695 Search PubMed;
(e) Y. Gu and F. Jérôme, Bio-based solvents: an emerging generation of fluids for the design of eco-efficient processes in catalysis and organic chemistry, Chem. Soc. Rev., 2013, 42, 9550–9570 Search PubMed.
- P. Gandeepan, N. Kaplaneris, S. Santoro, L. Vaccaro and L. Ackermann, Biomass-Derived Solvents for Sustainable Transition Metal-Catalyzed C–H Activation, ACS Sustainable Chem. Eng., 2019, 7, 8023–8040 Search PubMed.
-
(a) T. Kitanosono, K. Masuda, P. Xu and S. Kobayashi, Catalytic Organic Reactions in Water toward Sustainable Society, Chem. Rev., 2018, 118, 679–746 Search PubMed;
(b) B. Li and P. H. Dixneuf, sp2 C–H bond activation in water and catalytic cross-coupling reactions, Chem. Soc. Rev., 2013, 42, 5744–5767 Search PubMed;
(c) A. Chanda and V. V. Fokin, Organic Synthesis “On Water”, Chem. Rev., 2009, 109, 725–748 Search PubMed;
(d) C. I. Herrerías, X. Yao, Z. Li and C.-J. Li, Reactions of C−H Bonds in Water, Chem. Rev., 2007, 107, 2546–2562 Search PubMed;
(e) C.-J. Li, Organic Reactions in Aqueous Media with a Focus on Carbon−Carbon Bond Formations: A Decade Update, Chem. Rev., 2005, 105, 3095–3166 Search PubMed.
-
(a) Y. Zhu, C. Romain and C. K. Williams, Sustainable polymers from renewable resources, Nature, 2016, 540, 354–362 Search PubMed;
(b) B. Pereira, H. Zhang, M. De Mey, C. G. Lim, Z.-J. Li and G. Stephanopoulos, Engineering a novel biosynthetic pathway in Escherichia coli for production of renewable ethylene glycol, Biotechnol. Bioeng., 2016, 113, 376–383 Search PubMed.
-
(a) M. Vafaeezadeh and M. M. Hashemi, Polyethylene glycol (PEG) as a green solvent for carbon–carbon bond formation reactions, J. Mol. Liq., 2015, 207, 73–79 Search PubMed;
(b) E. Colacino, J. Martinez, F. Lamaty, L. S. Patrikeeva, L. L. Khemchyan, V. P. Ananikov and I. P. Beletskaya, PEG as an alternative reaction medium in metal-mediated transformations, Coord. Chem. Rev., 2012, 256, 2893–2920 Search PubMed;
(c) J. Chen, S. K. Spear, J. G. Huddleston and R. D. Rogers, Polyethylene glycol and solutions of polyethylene glycol as green reaction media, Green Chem., 2005, 7, 64–82 Search PubMed.
- L. Ackermann and R. Vicente, Catalytic Direct Arylations in Polyethylene Glycol (PEG): Recyclable Palladium(0) Catalyst for C−H Bond Cleavages in the Presence of Air, Org. Lett., 2009, 11, 4922–4925 Search PubMed.
-
(a) L. Ackermann, A. Althammer and R. Born, [RuCl3(H2O)n]-catalyzed direct arylations, Tetrahedron, 2008, 64, 6115–6124 Search PubMed;
(b) L. Ackermann, A. Althammer and R. Born, [RuCl3(H2O)n]-Catalyzed Direct Arylations with Bromides as Electrophiles, Synlett, 2007, 2833–2836 Search PubMed.
- L. Jian, H.-Y. He, J. Huang, Q.-H. Wu, M.-L. Yuan, H.-Y. Fu, X.-L. Zheng, H. Chen and R.-X. Li, Combination of RuCl3·xH2O with PEG – a simple and recyclable catalytic system for direct arylation of heteroarenes via C–H bond activation, RSC Adv., 2017, 7, 23515–23522 Search PubMed.
- S. I. Kozhushkov and L. Ackermann, Ruthenium-catalyzed direct oxidative alkenylation of arenes through twofold C–H bond functionalization, Chem. Sci., 2013, 4, 886–896 Search PubMed.
- N. Kavitha, G. Sukumar, V. P. Kumar, P. S. Mainkar and S. Chandrasekhar, Ruthenium-catalyzed benzimidazoisoquinoline synthesis via oxidative coupling of 2-arylbenzimidazoles with alkynes, Tetrahedron Lett., 2013, 54, 4198–4201 Search PubMed.
-
(a) C. Kornhaaß, J. Li and L. Ackermann, Cationic Ruthenium Catalysts for Alkyne Annulations with Oximes by C–H/N–O Functionalizations, J. Org. Chem., 2012, 77, 9190–9198 Search PubMed;
(b) L. Ackermann and S. Fenner, Ruthenium-Catalyzed C–H/N–O Bond Functionalization: Green Isoquinolone Syntheses in Water, Org. Lett., 2011, 13, 6548–6551 Search PubMed.
- S. L. Yedage and B. M. Bhanage, Ru(II)/PEG-400 as a highly efficient and recyclable catalytic media for annulation and olefination reactions via C–H bond activation, Green Chem., 2016, 18, 5635–5642 Search PubMed.
- D. S. Deshmukh and B. M. Bhanage, N-Tosylhydrazone directed annulation via C–H/N–N bond activation in Ru(II)/PEG-400 as homogeneous recyclable catalytic system: a green synthesis of isoquinolines, Org. Biomol. Chem., 2018, 16, 4864–4873 Search PubMed.
- H. Zhao, T. Zhang, T. Yan and M. Cai, Recyclable and Reusable [RuCl2(p-cymene)]2/Cu(OAc)2/P EG-400/H2O System for Oxidative C–H Bond Alkenylations: Green Synthesis of Phthalides, J. Org. Chem., 2015, 80, 8849–8855 Search PubMed.
- M.-A. Hiebel, Y. Fall, M.-C. Scherrmann and S. Berteina-Raboin, Straightforward Synthesis of Various 2,3-Diarylimidazo[1,2-a]pyridines in PEG400 Medium through One-Pot Condensation and C–H Arylation, Eur. J. Org. Chem., 2014, 4643–4650 Search PubMed.
- W. Ma and L. Ackermann, Cobalt(II)-Catalyzed Oxidative C–H Alkenylations: Regio- and Site-Selective Access to Isoindolin-1-one, ACS Catal., 2015, 5, 2822–2825 Search PubMed.
- C. Kuai, L. Wang, B. Li, Z. Yang and X. Cui, Cobalt-Catalyzed Selective Synthesis of Isoquinolines Using Picolinamide as a Traceless Directing Group, Org. Lett., 2017, 19, 2102–2105 Search PubMed.
- G. C. Reddy, P. Balasubramanyam, N. Salvanna and B. Das, Copper-Mediated C–H Activation of 1,3,4-Oxadiazoles with 1,1-Dibromo-1-alkenes Using PEG-400 as a Solvent Medium: Distinct Approach for the Alkynylation of 1,3,4-Oxadiazoles, Eur. J. Org. Chem., 2012, 471–474 Search PubMed.
- I. T. Horváth, H. Mehdi, V. Fábos, L. Boda and L. T. Mika, γ-Valerolactone—a sustainable liquid for energy and carbon-based chemicals, Green Chem., 2008, 10, 238–242 Search PubMed.
-
(a) Z. Zhang, Synthesis of γ-Valerolactone from Carbohydrates and its Applications, ChemSusChem, 2016, 9, 156–171 Search PubMed;
(b) F. Liguori, C. Moreno-Marrodan and P. Barbaro, Environmentally Friendly Synthesis of γ-Valerolactone by Direct Catalytic Conversion of Renewable Sources, ACS Catal., 2015, 5, 1882–1894 Search PubMed;
(c) A. Farrán, C. Cai, M. Sandoval, Y. Xu, J. Liu, M. J. Hernáiz and R. J. Linhardt, Green Solvents in Carbohydrate Chemistry: From Raw Materials to Fine Chemicals, Chem. Rev., 2015, 115, 6811–6853 Search PubMed;
(d) D. M. Alonso, S. G. Wettstein and J. A. Dumesic, Gamma-valerolactone, a sustainable platform molecule derived from lignocellulosic biomass, Green Chem., 2013, 15, 584–595 Search PubMed.
-
(a) G. Strappaveccia, L. Luciani, E. Bartollini, A. Marrocchi, F. Pizzo and L. Vaccaro,
γ-Valerolactone as an alternative biomass-derived medium for the Sonogashira reaction, Green Chem., 2015, 17, 1071–1076 Search PubMed;
(b) G. Strappaveccia, E. Ismalaj, C. Petrucci, D. Lanari, A. Marrocchi, M. Drees, A. Facchetti and L. Vaccaro, A biomass-derived safe medium to replace toxic dipolar solvents and access cleaner Heck coupling reactions, Green Chem., 2015, 17, 365–372 Search PubMed;
(c) E. Ismalaj, G. Strappaveccia, E. Ballerini, F. Elisei, O. Piermatti, D. Gelman and L. Vaccaro,
γ-Valerolactone as a Renewable Dipolar Aprotic Solvent Deriving from Biomass Degradation for the Hiyama Reaction, ACS Sustainable Chem. Eng., 2014, 2, 2461–2464 Search PubMed.
-
(a) P. Pongrácz, B. Bartal, L. Kollár and L. T. Mika, Rhodium-catalyzed hydroformylation in γ-valerolactone as a biomass-derived solvent, J. Organomet. Chem., 2017, 847, 140–145 Search PubMed;
(b) P. Pongrácz, L. Kollár and L. T. Mika, A step towards hydroformylation under sustainable conditions: platinum-catalysed enantioselective hydroformylation of styrene in gamma-valerolactone, Green Chem., 2016, 18, 842–847 Search PubMed.
- D. Marosvölgyi-Haskó, B. Lengyel, J. M. Tukacs, L. Kollár and L. T. Mika, Application of γ-Valerolactone as an Alternative Biomass-Based Medium for Aminocarbonylation Reactions, ChemPlusChem, 2016, 81, 1224–1229 Search PubMed.
- S. Tabasso, E. Calcio Gaudino, L. Rinaldi, A. Ledoux, P. Larini and G. Cravotto, Microwave-assisted, ligand-free, direct C–H arylation of thiophenes in biomass-derived γ-valerolactone, New J. Chem., 2017, 41, 9210–9215 Search PubMed.
- A. Bechtoldt, M. E. Baumert, L. Vaccaro and L. Ackermann, Ruthenium(II) oxidase catalysis for C–H alkenylations in biomass-derived γ-valerolactone, Green Chem., 2018, 20, 398–402 Search PubMed.
- Q. Bu, T. Rogge, V. Kotek and L. Ackermann, Distal Weak Coordination of Acetamides in Ruthenium(II)-Catalyzed C−H Activation Processes, Angew. Chem., Int. Ed., 2018, 57, 765–768 Search PubMed.
- Q. Bu, E. Gońka, K. Kuciński and L. Ackermann, Cobalt-Catalyzed Hiyama-Type C−H Activation with Arylsiloxanes: Versatile Access to Highly ortho-Decorated Biaryls, Chem. – Eur. J., 2019, 25, 2213–2216 Search PubMed.
-
(a) R. Mariscal, P. Maireles-Torres, M. Ojeda, I. Sádaba and M. López Granados, Furfural: a renewable and versatile platform molecule for the synthesis of chemicals and fuels, Energy Environ. Sci., 2016, 9, 1144–1189 Search PubMed;
(b) C. M. Cai, T. Zhang, R. Kumar and C. E. Wyman, Integrated furfural production as a renewable fuel and chemical platform from lignocellulosic biomass, J. Chem. Technol. Biotechnol., 2014, 89, 2–10 Search PubMed.
- D. F. Aycock, Solvent Applications of 2-Methyltetrahydrofuran in Organometallic and Biphasic Reactions, Org. Process Res. Dev., 2007, 11, 156–159 Search PubMed.
- J. A. Leitch, P. B. Wilson, C. L. McMullin, M. F. Mahon, Y. Bhonoah, I. H. Williams and C. G. Frost, Ruthenium(II)-Catalyzed C–H
Functionalization Using the Oxazolidinone Heterocycle as a Weakly Coordinating Directing Group: Experimental and Computational Insights, ACS Catal., 2016, 6, 5520–5529 Search PubMed.
- F. Fumagalli, S. Warratz, S.-K. Zhang, T. Rogge, C. Zhu, A. C. Stückl and L. Ackermann, Arene-Ligand-Free Ruthenium(II/III) Manifold for meta-C−H Alkylation: Remote Purine Diversification, Chem. – Eur. J., 2018, 24, 3984–3988 Search PubMed.
- R. Matsidik, A. Luzio, S. Hameury, H. Komber, C. R. McNeill, M. Caironi and M. Sommer, Effects of PNDIT2 end groups on aggregation, thin film structure, alignment and electron transport in field-effect transistors, J. Mater. Chem. C, 2016, 4, 10371–10380 Search PubMed.
- T. Bura, S. Beaupré, O. A. Ibraikulov, M.-A. Légaré, J. Quinn, P. Lévêque, T. Heiser, Y. Li, N. Leclerc and M. Leclerc, New Fluorinated Dithienyldiketopyrrolopyrrole Monomers and Polymers for Organic Electronics, Macromolecules, 2017, 50, 7080–7090 Search PubMed.
-
(a) B. M. Monks, E. R. Fruchey and S. P. Cook, Iron-Catalyzed C(sp2)–H Alkylation of Carboxamides with Primary Electrophiles, Angew. Chem., Int. Ed., 2014, 53, 11065–11069 Search PubMed;
(b) E. R. Fruchey, B. M. Monks and S. P. Cook, A Unified Strategy for Iron-Catalyzed ortho-Alkylation of Carboxamides, J. Am. Chem. Soc., 2014, 136, 13130–13133 Search PubMed.
-
(a) N. Krause, New surfactants for chemistry in water, Curr. Opin. Green Sustainable Chem., 2017, 7, 18–22 Search PubMed;
(b) S. Narayan, J. Muldoon, M. G. Finn, V. V. Fokin, H. C. Kolb and K. B. Sharpless, “On Water”: Unique Reactivity of Organic Compounds in Aqueous Suspension, Angew. Chem., Int. Ed., 2005, 44, 3275–3279 Search PubMed;
(c) J. E. Klijn and J. B. F. N. Engberts, Fast reactions ‘on water’, Nature, 2005, 435, 746–747 Search PubMed.
- T. Nishikata, A. R. Abela and B. H. Lipshutz, Room Temperature C–H Activation and Cross-Coupling of Aryl Ureas in Water, Angew. Chem., Int. Ed., 2010, 49, 781–784 Search PubMed.
- F. Szabó, J. Daru, D. Simkó, T. Z. Nagy, A. Stirling and Z. Novák, Mild Palladium-Catalyzed Oxidative Direct ortho-C–H Acylation of Anilides under Aqueous Conditions, Adv. Synth. Catal., 2013, 355, 685–691 Search PubMed.
- S. R. Yetra, T. Rogge, S. Warratz, J. Struwe, W. Peng, P. Vana and L. Ackermann, Micellar Catalysis for Ruthenium(II)-Catalyzed C−H Arylation: Weak-Coordination-Enabled C−H Activation in H2O, Angew. Chem., Int. Ed., 2019, 58, 7490–7494 Search PubMed.
-
(a) W. Liu, D. Wang, Y. Zhao, F. Yi and J. Chen, Palladium-Catalyzed Mono-Selective ortho C–H Arylation of Aryl Sulfonamides in Water: A Concise Access to Biaryl Sulfoamide Derivatives, Adv. Synth. Catal., 2016, 358, 1968–1974 Search PubMed;
(b) F. Chen, Q.-Q. Min and X. Zhang, Pd-Catalyzed Direct Arylation of Polyfluoroarenes on Water under Mild Conditions Using PPh3 Ligand, J. Org. Chem., 2012, 77, 2992–2998 Search PubMed;
(c) L. Joucla, N. Batail and L. Djakovitch, “On Water” Direct and Site-Selective Pd-Catalysed C–H Arylation of (NH)-Indoles, Adv. Synth. Catal., 2010, 352, 2929–2936 Search PubMed;
(d) S. A. Ohnmacht, P. Mamone, A. J. Culshaw and M. F. Greaney, Direct arylations on water: synthesis of 2,5-disubstituted oxazoles balsoxin and texaline, Chem. Commun., 2008, 1241–1243 Search PubMed;
(e) G. L. Turner, J. A. Morris and M. F. Greaney, Direct Arylation of Thiazoles on Water, Angew. Chem., Int. Ed., 2007, 46, 7996–8000 Search PubMed.
-
(a) S. Wu, X. Wu, C. Fu and S. Ma, Rhodium(III)-Catalyzed
C–H Functionalization in Water for Isoindolin-1-one Synthesis, Org. Lett., 2018, 20, 2831–2834 Search PubMed;
(b) S. Kim, S. Han, J. Park, S. Sharma, N. K. Mishra, H. Oh, J. H. Kwak and I. S. Kim, Cp*Rh(III)-catalyzed C(sp3)–H alkylation of 8-methylquinolines in aqueous media, Chem. Commun., 2017, 53, 3006–3009 Search PubMed;
(c) L. Shi and B. Wang, Tandem Rh(III)-Catalyzed C–H Amination/Annulation Reactions: Synthesis of Indoloquinoline Derivatives in Water, Org. Lett., 2016, 18, 2820–2823 Search PubMed;
(d) S. P. Midya, M. K. Sahoo, V. G. Landge, P. R. Rajamohanan and E. Balaraman, Reversed reactivity of anilines with alkynes in the rhodium-catalysed C–H activation/carbonylation tandem, Nat. Commun., 2015, 6, 8591 Search PubMed.
- S. Debbarma, M. R. Sk, B. Modak and M. S. Maji, On-Water Cp*Ir(III)-Catalyzed C–H Functionalization for the Synthesis of Chromones through Annulation of Salicylaldehydes with Diazo-Ketones, J. Org. Chem., 2019, 84, 6207–6216 Search PubMed.
-
(a) W.-J. Han, F. Pu, C.-J. Li, Z.-W. Liu, J. Fan and X.-Y. Shi, Carboxyl-Directed Conjugate Addition of C−H Bonds to α,β-Unsaturated Ketones in Air and Water, Adv. Synth. Catal., 2018, 360, 1358–1363 Search PubMed;
(b) L. Ackermann, L. Wang, R. Wolfram and A. V. Lygin, Ruthenium-Catalyzed Oxidative C–H Alkenylations of Anilides and Benzamides in Water, Org. Lett., 2012, 14, 728–731 Search PubMed;
(c) L. Ackermann and A. V. Lygin, Cationic Ruthenium(II) Catalysts for Oxidative C–H/N–H Bond Functionalizations of Anilines with Removable Directing Group: Synthesis of Indoles in Water, Org. Lett., 2012, 14, 764–767 Search PubMed;
(d) L. Ackermann and J. Pospech, Ruthenium-Catalyzed Oxidative C–H Bond Alkenylations in Water: Expedient Synthesis of Annulated Lactones, Org. Lett., 2011, 13, 4153–4155 Search PubMed;
(e) P. B. Arockiam, C. Fischmeister, C. Bruneau and P. H. Dixneuf, C–H Bond Functionalization in Water Catalyzed by Carboxylato Ruthenium(II) Systems, Angew. Chem., Int. Ed., 2010, 49, 6629–6632 Search PubMed;
(f) L. Ackermann, Phosphine Oxides as Preligands in Ruthenium-Catalyzed Arylations via C−H Bond Functionalization Using Aryl Chlorides, Org. Lett., 2005, 7, 3123–3125 Search PubMed.
-
(a) J. Xu, L. Qiao, J. Shen, K. Chai, C. Shen and P. Zhang, Nickel(II)-Catalyzed Site-Selective C–H Bond Trifluoromethylation of Arylamine in Water through a Coordinating Activation Strategy, Org. Lett., 2017, 19, 5661–5664 Search PubMed;
(b) L.-Y. Xie, Y.-J. Li, J. Qu, Y. Duan, J. Hu, K.-J. Liu, Z. Cao and W.-M. He, A base-free, ultrasound accelerated one-pot synthesis of 2-sulfonylquinolines in water, Green Chem., 2017, 19, 5642–5646 Search PubMed;
(c) H. Qiao, S. Sun, F. Yang, Y. Zhu, J. Kang, Y. Wu and Y. Wu, Merging Photoredox Catalysis with Iron(III) Catalysis: C5-H Bromination and Iodination of 8-Aminoquinoline Amides in Water, Adv. Synth. Catal., 2017, 359, 1976–1980 Search PubMed;
(d) H. Fang, Y. Dou, J. Ge, M. Chhabra, H. Sun, P. Zhang, Y. Zheng and Q. Zhu, Regioselective and Direct Azidation of Anilines via Cu(II)-Catalyzed C–H Functionalization in Water, J. Org. Chem., 2017, 82, 11212–11217 Search PubMed;
(e) C.-J. Wu, J.-J. Zhong, Q.-Y. Meng, T. Lei, X.-W. Gao, C.-H. Tung and L.-Z. Wu, Cobalt-Catalyzed Cross-Dehydrogenative Coupling Reaction in Water by Visible Light, Org. Lett., 2015, 17, 884–887 Search PubMed.
- H. Wang, M. M. Lorion and L. Ackermann, Air-Stable Manganese(I)-Catalyzed C−H Activation for Decarboxylative C−H/C−O Cleavages in Water, Angew. Chem., Int. Ed., 2017, 56, 6339–6342 Search PubMed.
- H. Wang, I. Choi, T. Rogge, N. Kaplaneris and L. Ackermann, Versatile and robust C–C activation by chelation-assisted manganese catalysis, Nat. Catal., 2018, 1, 993–1001 Search PubMed.
- Q. Lu, F. J. R. Klauck and F. Glorius, Manganese-catalyzed allylation via sequential C–H and C–C/C–Het bond activation, Chem. Sci., 2017, 8, 3379–3383 Search PubMed.
- S. Santoro, S. I. Kozhushkov, L. Ackermann and L. Vaccaro, Heterogeneous catalytic approaches in C–H activation reactions, Green Chem., 2016, 18, 3471–3493 Search PubMed.
- A. A. Latimer, A. R. Kulkarni, H. Aljama, J. H. Montoya, J. S. Yoo, C. Tsai, F. Abild-Pedersen, F. Studt and J. K. Nørskov, Understanding trends in C–H bond activation in heterogeneous catalysis, Nat. Mater., 2017, 16, 225–229 Search PubMed.
- M. Parisien, D. Valette and K. Fagnou, Direct Arylation Reactions Catalyzed by Pd(OH)2/C: Evidence for a Soluble Palladium Catalyst, J. Org. Chem., 2005, 70, 7578–7584 Search PubMed.
- F. Jafarpour, S. Rahiminejadan and H. Hazrati, Triethanolamine-Mediated Palladium-Catalyzed Regioselective C-2 Direct Arylation of Free NH-Pyrroles, J. Org. Chem., 2010, 75, 3109–3112 Search PubMed.
- K. D. Collins, R. Honeker, S. Vásquez-Céspedes, D.-T. D. Tang and F. Glorius, C–H arylation of triphenylene, naphthalene and related arenes using Pd/C, Chem. Sci., 2015, 6, 1816–1824 Search PubMed.
-
(a) Y.-B. Huang, M. Shen, X. Wang, P. Huang, R. Chen, Z.-J. Lin and R. Cao, Water-medium C–H activation over a hydrophobic perfluoroalkane-decorated metal-organic framework platform, J. Catal., 2016, 333, 1–7 Search PubMed;
(b) M. Cao, D. Wu, W. Su and R. Cao, Palladium nanocrystals stabilized by cucurbit[6]uril as efficient heterogeneous catalyst for direct C–H functionalization of polyfluoroarenes, J. Catal., 2015, 321, 62–69 Search PubMed;
(c) J. Malmgren, A. Nagendiran, C.-W. Tai, J.-E. Bäckvall and B. Olofsson, C-2 Selective Arylation of Indoles with Heterogeneous Nanopalladium and Diaryliodonium Salts, Chem. – Eur. J., 2014, 20, 13531–13535 Search PubMed;
(d) V. A. Zinovyeva, M. A. Vorotyntsev, I. Bezverkhyy, D. Chaumont and J.-C. Hierso, Highly Dispersed Palladium–Polypyrrole Nanocomposites: In-Water Synthesis and Application for Catalytic Arylation of Heteroaromatics by Direct C–H Bond Activation, Adv. Funct. Mater., 2011, 21, 1064–1075 Search PubMed;
(e) L. Wang, W.-b. Yi and C. Cai, Fluorous silica gel-supported perfluoro-tagged palladium nanoparticles: an efficient and reusable catalyst for direct C-2 arylation of indoles, Chem. Commun., 2011, 47, 806–808 Search PubMed;
(f) T.-H. Park, A. J. Hickman, K. Koh, S. Martin, A. G. Wong-Foy, M. S. Sanford and A. J. Matzger, Highly Dispersed Palladium(II) in a Defective Metal–Organic Framework: Application to C–H Activation and Functionalization, J. Am. Chem. Soc., 2011, 133, 20138–20141 Search PubMed;
(g) Y. Huang, Z. Lin and R. Cao, Palladium Nanoparticles Encapsulated in a Metal–Organic Framework as Efficient Heterogeneous Catalysts for Direct C2 Arylation of Indoles, Chem. – Eur. J., 2011, 17, 12706–12712 Search PubMed;
(h) S. Hernández, I. Moreno, R. SanMartin, G. Gómez, M. T. Herrero and E. Domínguez, Toward Safer Processes for C−C Biaryl Bond Construction: Catalytic Direct C−H Arylation and Tin-Free Radical Coupling in the Synthesis of Pyrazolophenanthridines, J. Org. Chem., 2010, 75, 434–441 Search PubMed.
-
(a) S. Keshipour and A. Shaabani, Copper(I) and palladium nanoparticles supported on ethylenediamine-functionalized cellulose as an efficient catalyst for the 1,3-dipolar cycloaddition/direct arylation sequence, Appl. Organomet. Chem., 2014, 28, 116–119 Search PubMed;
(b) D.-T. D. Tang, K. D. Collins and F. Glorius, Completely Regioselective Direct C–H Functionalization of Benzo[b]thiophenes Using a Simple Heterogeneous Catalyst, J. Am. Chem. Soc., 2013, 135, 7450–7453 Search PubMed.
- E. Y. Lee and J. Park, Recyclable Copper Catalyst for meta-Selective C–H Bond Arylation, ChemCatChem, 2011, 3, 1127–1129 Search PubMed.
- W. Zhang, Q. Zeng, X. Zhang, Y. Tian, Y. Yue, Y. Guo and Z. Wang, Ligand-Free CuO Nanospindle Catalyzed Arylation of Heterocycle C–H Bonds, J. Org. Chem., 2011, 76, 4741–4745 Search PubMed.
-
(a) H. T. N. Le, T. T. Nguyen, P. H. L. Vu, T. Truong and N. T. S. Phan, Ligand-free direct C-arylation of heterocycles with aryl halides over a metal-organic framework Cu2(BPDC)2(BPY) as an efficient and robust heterogeneous catalyst, J. Mol. Catal. A: Chem., 2014, 391, 74–82 Search PubMed;
(b) F. Jeremias, D. Fröhlich, C. Janiak and S. K. Henninger, Advancement of sorption-based heat transformation by a metal coating of highly-stable, hydrophilic aluminium fumarate MOF, RSC Adv., 2014, 4, 24073–24082 Search PubMed.
- I. Choi, V. Müller, G. Lole, R. Köhler, V. Karius, W. Viöl, C. Jooss and L. Ackermann, Photoinduced Heterogeneous C−H Arylation by a Reusable Hybrid Copper Catalyst, Chem. – Eur. J., 2020, 26, 3509–3514 Search PubMed.
- H. Miura, K. Wada, S. Hosokawa and M. Inoue, Recyclable Solid Ruthenium Catalysts for the Direct Arylation of Aromatic C–H Bonds, Chem. – Eur. J., 2010, 16, 4186–4189 Search PubMed.
-
(a) W. Liang, T. Zhang, Y. Liu, Y. Huang, Z. Liu, Y. Liu, B. Yang, X. Zhou and J. Zhang, Polydimethylsiloxane Sponge-Supported Nanometer Gold: Highly Efficient Recyclable Catalyst for Cross-Dehydrogenative Coupling in Water, ChemSusChem, 2018, 11, 3586–3590 Search PubMed;
(b) Y. Zhang, Y. Zhao, Y. Luo, L. Xiao, Y. Huang, X. Li, Q. Peng, Y. Liu, B. Yang, C. Zhu, X. Zhou and J. Zhang, Directed Aromatic C–H Activation/Acetoxylation Catalyzed by Pd Nanoparticles Supported on Graphene Oxide, Org. Lett., 2017, 19, 6470–6473 Search PubMed;
(c) V. Panwar, P. Kumar, A. Bansal, S. S. Ray and S. L. Jain, PEGylated magnetic nanoparticles (PEG@Fe3O4) as cost effective alternative for oxidative cyanation of tertiary amines via CH activation, Appl. Catal., A, 2015, 498, 25–31 Search PubMed;
(d) L. He, K. Natte, J. Rabeah, C. Taeschler, H. Neumann, A. Brückner and M. Beller, Heterogeneous Platinum-Catalyzed C–H Perfluoroalkylation of Arenes and Heteroarenes, Angew. Chem., Int. Ed., 2015, 54, 4320–4324 Search PubMed;
(e) H. Duan, M. Li, G. Zhang, J. R. Gallagher, Z. Huang, Y. Sun, Z. Luo, H. Chen, J. T. Miller, R. Zou, A. Lei and Y. Zhao, Single-Site Palladium(II) Catalyst for Oxidative Heck Reaction: Catalytic Performance and Kinetic Investigations, ACS Catal., 2015, 5, 3752–3759 Search PubMed;
(f) J. Chen, K. Natte and X.-F. Wu, Pd/C-catalyzed carbonylative C–H activation with DMF as the CO source, Tetrahedron Lett., 2015, 56, 6413–6416 Search PubMed;
(g) C. Bai, X. Yao and Y. Li, Easy Access to Amides through Aldehydic C–H Bond Functionalization Catalyzed by Heterogeneous Co-Based Catalysts, ACS Catal., 2015, 5, 884–891 Search PubMed;
(h) J. Chen, L. He, K. Natte, H. Neumann, M. Beller and X.-F. Wu, Palladium@Cerium(IV) Oxide-Catalyzed Oxidative Synthesis of N-(2-Pyridyl)indoles via C–H Activation Reaction, Adv. Synth. Catal., 2014, 356, 2955–2959 Search PubMed;
(i) R. Kishore, M. L. Kantam, J. Yadav, M. Sudhakar, S. Laha and A. Venugopal, Pd/Mg–La mixed oxide catalyzed oxidative sp2 CH bond acylation with alcohols, J. Mol. Catal. A: Chem., 2013, 379, 213–218 Search PubMed;
(j) S. Verma, S. L. Jain and B. Sain, Starch Immobilized Ruthenium Trichloride Catalyzed Oxidative Cyanation of Tertiary Amines with Hydrogen Peroxide, ChemCatChem, 2011, 3, 1329–1332 Search PubMed;
(k) L. L. Chng, J. Zhang, J. Yang, M. Amoura and J. Y. Ying, C–C Bond Formation via C–H Activation and C–N Bond Formation via Oxidative Amination Catalyzed by Palladium- Polyoxometalate Nanomaterials Using Dioxygen as the Terminal Oxidant, Adv. Synth. Catal., 2011, 353, 2988–2998 Search PubMed;
(l) H. Miura, K. Wada, S. Hosokawa and M. Inoue, Catalytic Addition of Aromatic C–H Bonds to Vinylsilanes in the Presence of Ru/CeO2, ChemCatChem, 2010, 2, 1223–1225 Search PubMed;
(m) S. Haldar and S. Koner, Iron-Containing Mesoporous Aluminosilicate: A Highly Active and Reusable Heterogeneous Catalyst for Hydroarylation of Styrenes, J. Org. Chem., 2010, 75, 6005–6008 Search PubMed.
- D. Rasina, A. Kahler-Quesada, S. Ziarelli, S. Warratz, H. Cao, S. Santoro, L. Ackermann and L. Vaccaro, Heterogeneous palladium-catalysed Catellani reaction in biomass-derived γ-valerolactone, Green Chem., 2016, 18, 5025–5030 Search PubMed.
- X. Tian, F. Yang, D. Rasina, M. Bauer, S. Warratz, F. Ferlin, L. Vaccaro and L. Ackermann, C–H arylations of 1,2,3-triazoles by reusable heterogeneous palladium catalysts in biomass-derived γ-valerolactone, Chem. Commun., 2016, 52, 9777–9780 Search PubMed.
- F. Ferlin, L. Luciani, S. Santoro, A. Marrocchi, D. Lanari, A. Bechtoldt, L. Ackermann and L. Vaccaro, A continuous flow approach for the C–H functionalization of 1,2,3-triazoles in γ-valerolactone as a biomass-derived medium, Green Chem., 2018, 20, 2888–2893 Search PubMed.
- S. Vásquez-Céspedes, A. Ferry, L. Candish and F. Glorius, Heterogeneously Catalyzed Direct C–H Thiolation of Heteroarenes, Angew. Chem., Int. Ed., 2015, 54, 5772–5776 Search PubMed.
- R. Kishore, J. Yadav, B. Venu, A. Venugopal and M. Lakshmi Kantam, A Pd(II)/Mg–La mixed oxide catalyst for cyanation of aryl C–H bonds and tandem Suzuki–cyanation reactions, New J. Chem., 2015, 39, 5259–5264 Search PubMed.
- V. Pascanu, F. Carson, M. V. Solano, J. Su, X. Zou, M. J. Johansson and B. Martín-Matute, Selective Heterogeneous C−H Activation/Halogenation Reactions Catalyzed by Pd@MOF Nanocomposites, Chem. – Eur. J., 2016, 22, 3729–3737 Search PubMed.
- S. Warratz, D. J. Burns, C. Zhu, K. Korvorapun, T. Rogge, J. Scholz, C. Jooss, D. Gelman and L. Ackermann,
meta-C−H Bromination on Purine Bases by Heterogeneous Ruthenium Catalysis, Angew. Chem., Int. Ed., 2017, 56, 1557–1560 Search PubMed.
- I. Choi, V. Müller, Y. Wang, K. Xue, R. Kuniyil, L. B. Andreas, V. Karius, J. G. Alauzun and L. Ackermann, Recyclable Ruthenium Catalyst for Distal meta-C−H Activation, Chem. – Eur. J., 2020, 26, 15290–15297 Search PubMed.
- H. Fei and S. M. Cohen, Metalation of a Thiocatechol-Functionalized Zr(IV)-Based Metal–Organic Framework for Selective C–H Functionalization, J. Am. Chem. Soc., 2015, 137, 2191–2194 Search PubMed.
-
(a) F. Wu, Y. Feng and C. W. Jones, Recyclable Silica-Supported Iridium Bipyridine Catalyst for Aromatic C–H Borylation, ACS Catal., 2014, 4, 1365–1375 Search PubMed;
(b) R. Murakami, K. Tsunoda, T. Iwai and M. Sawamura, Stereoselective C–H Borylations of Cyclopropanes and Cyclobutanes with Silica-Supported Monophosphane–Ir Catalysts, Chem. – Eur. J., 2014, 20, 13127–13131 Search PubMed;
(c) T. Iwai, R. Murakami, T. Harada, S. Kawamorita and M. Sawamura, Silica-Supported Tripod Triarylphosphane: Application to Transition Metal-Catalyzed C(sp3)–H Borylations, Adv. Synth. Catal., 2014, 356, 1563–1570 Search PubMed;
(d) W. R. Grüning, G. Siddiqi, O. V. Safonova and C. Copéret, Bipyridine Periodic Mesoporous Organosilica: A Solid Ligand for the Iridium-Catalyzed Borylation of C–H Bonds, Adv. Synth. Catal., 2014, 356, 673–679 Search PubMed;
(e) S. Kawamorita, R. Murakami, T. Iwai and M. Sawamura, Synthesis of Primary and Secondary Alkylboronates through Site-Selective C(sp3)–H Activation with Silica-Supported Monophosphine–Ir Catalysts, J. Am. Chem. Soc., 2013, 135, 2947–2950 Search PubMed;
(f) T. Iwai, T. Harada, K. Hara and M. Sawamura, Threefold Cross-Linked Polystyrene–Triphenylphosphane Hybrids: Mono-P-Ligating Behavior and Catalytic Applications for Aryl Chloride Cross-Coupling and C(sp3)–H Borylation, Angew. Chem., Int. Ed., 2013, 52, 12322–12326 Search PubMed;
(g) S. Kawamorita, T. Miyazaki, T. Iwai, H. Ohmiya and M. Sawamura, Rh-Catalyzed Borylation of N-Adjacent C(sp3)–H Bonds with a Silica-Supported Triarylphosphine Ligand, J. Am. Chem. Soc., 2012, 134, 12924–12927 Search PubMed;
(h) S. Kawamorita, T. Miyazaki, H. Ohmiya, T. Iwai and M. Sawamura, Rh-Catalyzed Ortho-Selective C–H Borylation of N-Functionalized Arenes with Silica-Supported Bridgehead Monophosphine Ligands, J. Am. Chem. Soc., 2011, 133, 19310–19313 Search PubMed;
(i) K. Yamazaki, S. Kawamorita, H. Ohmiya and M. Sawamura, Directed Ortho Borylation of Phenol Derivatives Catalyzed by a Silica-Supported Iridium Complex, Org. Lett., 2010, 12, 3978–3981 Search PubMed;
(j) T. Tagata, M. Nishida and A. Nishida, Continuous-Flow C–H Borylation of Arene Derivatives, Adv. Synth. Catal., 2010, 352, 1662–1666 Search PubMed;
(k) S. Kawamorita, H. Ohmiya and M. Sawamura, Ester-Directed Regioselective Borylation of Heteroarenes Catalyzed by a Silica-Supported Iridium Complex, J. Org. Chem., 2010, 75, 3855–3858 Search PubMed;
(l) T. Tagata, M. Nishida and A. Nishida, Development of recyclable iridium catalyst for C–H borylation, Tetrahedron Lett., 2009, 50, 6176–6179 Search PubMed;
(m) S. Kawamorita, H. Ohmiya, K. Hara, A. Fukuoka and M. Sawamura, Directed Ortho Borylation of Functionalized Arenes Catalyzed by a Silica-Supported Compact Phosphine−Iridium System, J. Am. Chem. Soc., 2009, 131, 5058–5059 Search PubMed;
(n) Z. Yinghuai, K. Chenyan, A. T. Peng, A. Emi, W. Monalisa, L. Kui-Jin Louis, N. S. Hosmane and J. A. Maguire, Catalytic Phenylborylation Reaction by Iridium(0) Nanoparticles Produced from Hydridoiridium Carborane, Inorg. Chem., 2008, 47, 5756–5761 Search PubMed.
-
(a) G. E. M. Crisenza, D. Mazzarella and P. Melchiorre, Synthetic Methods Driven by the Photoactivity of Electron Donor–Acceptor Complexes, J. Am. Chem. Soc., 2020, 142, 5461–5476 Search PubMed;
(b) K. L. Skubi, T. R. Blum and T. P. Yoon, Dual Catalysis Strategies in Photochemical Synthesis, Chem. Rev., 2016, 116, 10035–10074 Search PubMed;
(c) M. H. Shaw, J. Twilton and D. W. C. MacMillan, Photoredox Catalysis in Organic Chemistry, J. Org. Chem., 2016, 81, 6898–6926 Search PubMed;
(d) D. Ravelli, S. Protti and M. Fagnoni, Carbon–Carbon Bond Forming Reactions via Photogenerated Intermediates, Chem. Rev., 2016, 116, 9850–9913 Search PubMed;
(e) S. Poplata, A. Tröster, Y.-Q. Zou and T. Bach, Recent Advances in the Synthesis of Cyclobutanes by Olefin [2 + 2] Photocycloaddition Reactions, Chem. Rev., 2016, 116, 9748–9815 Search PubMed;
(f) E. C. Gentry and R. R. Knowles, Synthetic Applications of Proton-Coupled Electron Transfer, Acc. Chem. Res., 2016, 49, 1546–1556 Search PubMed.
- L. Guillemard and J. Wencel-Delord, When metal-catalyzed C–H functionalization meets visible-light photocatalysis, Beilstein J. Org. Chem., 2020, 16, 1754–1804 Search PubMed.
-
(a) J. Twilton, C. Le, P. Zhang, M. H. Shaw, R. W. Evans and D. W. C. MacMillan, The merger of transition metal and photocatalysis, Nat. Rev. Chem., 2017, 1, 52 Search PubMed;
(b) Z. Zuo, D. T. Ahneman, L. Chu, J. A. Terrett, A. G. Doyle and D. W. C. MacMillan, Merging photoredox with nickel catalysis: Coupling of α-carboxyl sp3-carbons with aryl halides, Science, 2014, 345, 437–440 Search PubMed;
(c) J. C. Tellis, D. N. Primer and G. A. Molander, Single-electron transmetalation in organoboron cross-coupling by photoredox/nickel dual catalysis, Science, 2014, 345, 433–436 Search PubMed;
(d) M. Osawa, H. Nagai and M. Akita, Photo-activation of Pd-catalyzed Sonogashira coupling using a Ru/bipyridine complex as energy transfer agent, Dalton Trans., 2007, 827–829 Search PubMed.
-
(a) Y.-F. Liang, R. Steinbock, L. Yang and L. Ackermann, Continuous Visible-Light Photoflow Approach for a Manganese-Catalyzed (Het)Arene C−H Arylation, Angew. Chem., Int. Ed., 2018, 57, 10625–10629 Search PubMed;
(b) M. K. Sahoo, S. P. Midya, V. G. Landge and E. Balaraman, A unified strategy for silver-, base-, and oxidant-free direct arylation of C–H bonds, Green Chem., 2017, 19, 2111–2117 Search PubMed;
(c) H. P. L. Gemoets, I. Kalvet, A. V. Nyuchev, N. Erdmann, V. Hessel, F. Schoenebeck and T. Noël, Mild and selective base-free C–H arylation of heteroarenes: experiment and computation, Chem. Sci., 2017, 8, 1046–1055 Search PubMed;
(d) J. Zoller, D. C. Fabry and M. Rueping, Unexpected Dual Role of Titanium Dioxide in the Visible Light Heterogeneous Catalyzed C–H Arylation of Heteroarenes, ACS Catal., 2015, 5, 3900–3904 Search PubMed;
(e) D. Cantillo, C. Mateos, J. A. Rincon, O. de Frutos and C. O. Kappe, Light-Induced C–H Arylation of (Hetero)arenes by In Situ Generated Diazo Anhydrides, Chem. – Eur. J., 2015, 21, 12894–12898 Search PubMed;
(f) I. Ghosh, T. Ghosh, J. I. Bardagi and B. König, Reduction of aryl halides by consecutive visible light-induced electron transfer processes, Science, 2014, 346, 725–728 Search PubMed;
(g) P. Schroll, D. P. Hari and B. König, Photocatalytic Arylation of Alkenes, Alkynes and Enones with Diazonium Salts, ChemistryOpen, 2012, 1, 130–133 Search PubMed;
(h) D. P. Hari, P. Schroll and B. König, Metal-Free, Visible-Light-Mediated Direct C–H Arylation of Heteroarenes with Aryl Diazonium Salts, J. Am. Chem. Soc., 2012, 134, 2958–2961 Search PubMed.
- D. Kalyani, K. B. McMurtrey, S. R. Neufeldt and M. S. Sanford, Room-Temperature C–H Arylation: Merger of Pd-Catalyzed C–H Functionalization and Visible-Light Photocatalysis, J. Am. Chem. Soc., 2011, 133, 18566–18569 Search PubMed.
- G. Meng, N. Y. S. Lam, E. L. Lucas, T. G. Saint-Denis, P. Verma, N. Chekshin and J.-Q. Yu, Achieving Site-Selectivity for C–H Activation Processes Based on Distance and Geometry: A Carpenter's Approach, J. Am. Chem. Soc., 2020, 142, 10571–10591 Search PubMed.
-
(a) S. Bag, T. Patra, A. Modak, A. Deb, S. Maity, U. Dutta, A. Dey, R. Kancherla, A. Maji, A. Hazra, M. Bera and D. Maiti, Remote para-C–H Functionalization of Arenes by a D-Shaped Biphenyl Template-Based Assembly, J. Am. Chem. Soc., 2015, 137, 11888–11891 Search PubMed;
(b) L. Wan, N. Dastbaravardeh, G. Li and J.-Q. Yu, Cross-Coupling of Remote meta-C–H Bonds Directed by a U-Shaped Template, J. Am. Chem. Soc., 2013, 135, 18056–18059 Search PubMed;
(c) D. Leow, G. Li, T.-S. Mei and J.-Q. Yu, Activation of remote meta-C–H bonds assisted by an end-on template, Nature, 2012, 486, 518–522 Search PubMed.
-
(a) H. J. Davis, M. T. Mihai and R. J. Phipps, Ion Pair-Directed Regiocontrol in Transition-Metal Catalysis: A Meta-Selective C–H Borylation of Aromatic Quaternary Ammonium Salts, J. Am. Chem. Soc., 2016, 138, 12759–12762 Search PubMed;
(b) Y. Kuninobu, H. Ida, M. Nishi and M. Kanai, A meta-selective C–H borylation directed by a secondary interaction between ligand and substrate, Nat. Chem., 2015, 7, 712–717 Search PubMed.
-
(a) X.-C. Wang, W. Gong, L.-Z. Fang, R.-Y. Zhu, S. Li, K. M. Engle and J.-Q. Yu, Ligand-enabled meta-C–H activation using a transient mediator, Nature, 2015, 519, 334–338 Search PubMed;
(b) Z. Dong, J. Wang and G. Dong, Simple Amine-Directed Meta-Selective C–H Arylation via Pd/Norbornene Catalysis, J. Am. Chem. Soc., 2015, 137, 5887–5890 Search PubMed.
- M. Catellani, E. Motti and N. Della Ca’, Catalytic Sequential Reactions Involving Palladacycle-Directed Aryl Coupling Steps, Acc. Chem. Res., 2008, 41, 1512–1522 Search PubMed.
-
(a) K. Korvorapun, R. Kuniyil and L. Ackermann, Late-Stage Diversification by Selectivity Switch in meta-C–H Activation: Evidence for Singlet Stabilization, ACS Catal., 2020, 10, 435–440 Search PubMed;
(b) K. Korvorapun, N. Kaplaneris, T. Rogge, S. Warratz, A. C. Stückl and L. Ackermann, Sequential meta-/ortho-C–H Functionalizations by One-Pot Ruthenium(II/III) Catalysis, ACS Catal., 2018, 8, 886–892 Search PubMed;
(c) J. Li, S. Warratz, D. Zell, S. De Sarkar, E. E. Ishikawa and L. Ackermann, N-Acyl Amino Acid Ligands for Ruthenium(II)-Catalyzed meta-C–H tert-Alkylation with Removable Auxiliaries, J. Am. Chem. Soc., 2015, 137, 13894–13901 Search PubMed;
(d) N. Hofmann and L. Ackermann, meta-Selective C–H Bond Alkylation with Secondary Alkyl Halides, J. Am. Chem. Soc., 2013, 135, 5877–5884 Search PubMed;
(e) O. Saidi, J. Marafie, A. E. W. Ledger, P. M. Liu, M. F. Mahon, G. Kociok-Köhn, M. K. Whittlesey and C. G. Frost, Ruthenium-Catalyzed Meta Sulfonation of 2-Phenylpyridines, J. Am. Chem. Soc., 2011, 133, 19298–19301 Search PubMed.
- P. Gandeepan, J. Koeller, K. Korvorapun, J. Mohr and L. Ackermann, Visible-Light-Enabled Ruthenium-Catalyzed meta-C−H Alkylation at Room Temperature, Angew. Chem., Int. Ed., 2019, 58, 9820–9825 Search PubMed.
- A. Sagadevan and M. F. Greaney,
meta-Selective C−H Activation of Arenes at Room Temperature Using Visible Light: Dual-Function Ruthenium Catalysis, Angew. Chem., Int. Ed., 2019, 58, 9826–9830 Search PubMed.
- K. Korvorapun, J. Struwe, R. Kuniyil, A. Zangarelli, A. Casnati, M. Waeterschoot and L. Ackermann, Photo-Induced Ruthenium-Catalyzed C−H Arylations at Ambient Temperature, Angew. Chem., 2020, 59, 18103–18109 Search PubMed.
- I. V. Seregin and V. Gevorgyan, Direct transition metal-catalyzed functionalization of heteroaromatic compounds, Chem. Soc. Rev., 2007, 36, 1173–1193 Search PubMed.
- F. Yang, J. Koeller and L. Ackermann, Photoinduced Copper-Catalyzed C−H Arylation at Room Temperature, Angew. Chem., Int. Ed., 2016, 55, 4759–4762 Search PubMed.
-
(a) G. Shan, J. Flegel, H. Li, C. Merten, S. Ziegler, A. P. Antonchick and H. Waldmann, C−H Bond Activation for the Synthesis of Heterocyclic Atropisomers Yields Hedgehog Pathway Inhibitors, Angew. Chem., Int. Ed., 2018, 57, 14250–14254 Search PubMed;
(b) T. Piou and T. Rovis, Electronic and Steric Tuning of a Prototypical Piano Stool Complex: Rh(III) Catalysis for C–H Functionalization, Acc. Chem. Res., 2018, 51, 170–180 Search PubMed;
(c) N. Guimond and K. Fagnou, Isoquinoline Synthesis via Rhodium-Catalyzed Oxidative Cross-Coupling/Cyclization of Aryl Aldimines and Alkynes, J. Am. Chem. Soc., 2009, 131, 12050–12051 Search PubMed.
-
(a) F. W. Patureau and F. Glorius, Rh Catalyzed Olefination and Vinylation of Unactivated Acetanilides, J. Am. Chem. Soc., 2010, 132, 9982–9983 Search PubMed;
(b) N. Umeda, K. Hirano, T. Satoh and M. Miura, Rhodium-Catalyzed Mono- and Divinylation of 1-Phenylpyrazoles and Related Compounds via Regioselective C−H Bond Cleavage, J. Org. Chem., 2009, 74, 7094–7099 Search PubMed;
(c) K. Ueura, T. Satoh and M. Miura, An Efficient Waste-Free Oxidative Coupling via Regioselective C−H Bond Cleavage: Rh/Cu-Catalyzed Reaction of Benzoic Acids with Alkynes and Acrylates under Air, Org. Lett., 2007, 9, 1407–1409 Search PubMed.
- D. C. Fabry, J. Zoller, S. Raja and M. Rueping, Combining Rhodium and Photoredox Catalysis for C−H Functionalizations of Arenes: Oxidative Heck Reactions with Visible Light, Angew. Chem., Int. Ed., 2014, 53, 10228–10231 Search PubMed.
-
(a) D. C. Fabry and M. Rueping, Merging Visible Light Photoredox Catalysis with Metal Catalyzed C–H Activations: On the Role of Oxygen and Superoxide Ions as Oxidants, Acc. Chem. Res., 2016, 49, 1969–1979 Search PubMed;
(b) D. C. Fabry, M. A. Ronge, J. Zoller and M. Rueping, C−H Functionalization of Phenols Using Combined Ruthenium and Photoredox Catalysis: In Situ Generation of the Oxidant, Angew. Chem., Int. Ed., 2015, 54, 2801–2805 Search PubMed.
- D. Kalsi, N. Barsu, S. Chakrabarti, P. Dahiya, M. Rueping and B. Sundararaju, C–H and N–H bond annulation of aryl amides with unactivated olefins by merging cobalt(III) and photoredox catalysis, Chem. Commun., 2019, 55, 11626–11629 Search PubMed.
-
(a) S. Govaerts, A. Nyuchev and T. Noel, Pushing the boundaries of C–H bond functionalization chemistry using flow technology, J. Flow Chem., 2020, 10, 13–71 Search PubMed;
(b) S. Santoro, F. Ferlin, L. Ackermann and L. Vaccaro, C–H functionalization reactions under flow conditions, Chem. Soc. Rev., 2019, 48, 2767–2782 Search PubMed;
(c) M. B. Plutschack, B. Pieber, K. Gilmore and P. H. Seeberger, The Hitchhiker's Guide to Flow Chemistry, Chem. Rev., 2017, 117, 11796–11893 Search PubMed;
(d) J. Britton and T. F. Jamison, A Unified Continuous Flow Assembly-Line Synthesis of Highly Substituted Pyrazoles and Pyrazolines, Angew. Chem., Int. Ed., 2017, 56, 8823–8827 Search PubMed;
(e) D. Cambié, C. Bottecchia, N. J. W. Straathof, V. Hessel and T. Noël, Applications of Continuous-Flow Photochemistry in Organic Synthesis, Material Science, and Water Treatment, Chem. Rev., 2016, 116, 10276–10341 Search PubMed.
- J. Zakrzewski, A. P. Smalley, M. A. Kabeshov, M. J. Gaunt and A. A. Lapkin, Continuous-Flow Synthesis and Derivatization of Aziridines through Palladium-Catalyzed C(sp3)−H Activation, Angew. Chem., Int. Ed., 2016, 55, 8878–8883 Search PubMed.
- A. P. Smalley and M. J. Gaunt, Mechanistic Insights into the Palladium-Catalyzed Aziridination of Aliphatic Amines by C–H Activation, J. Am. Chem. Soc., 2015, 137, 10632–10641 Search PubMed.
- F. Ferlin, S. Santoro, L. Ackermann and L. Vaccaro, Heterogeneous C–H alkenylations in continuous-flow: oxidative palladium-catalysis in a biomass-derived reaction medium, Green Chem., 2017, 19, 2510–2514 Search PubMed.
- U. K. Sharma, H. P. L. Gemoets, F. Schröder, T. Noël and E. V. Van der Eycken, Merger of Visible-Light Photoredox Catalysis and C–H Activation for the Room-Temperature C-2 Acylation of Indoles in Batch and Flow, ACS
Catal., 2017, 7, 3818–3823 Search PubMed.
- A. Baccalini, S. Vergura, P. Dolui, G. Zanoni and D. Maiti, Recent advances in cobalt-catalysed C–H functionalizations, Org. Biomol. Chem., 2019, 17, 10119–10141 Search PubMed.
- H. Wang, F. Pesciaioli, J. C. A. Oliveira, S. Warratz and L. Ackermann, Synergistic Manganese(I) C−H Activation Catalysis in Continuous Flow: Chemoselective Hydroarylation, Angew. Chem., Int. Ed., 2017, 56, 15063–15067 Search PubMed.
- C. Zhu, J. C. A. Oliveira, Z. Shen, H. Huang and L. Ackermann, Manganese(II/III/I)-Catalyzed C–H Arylations in Continuous Flow, ACS Catal., 2018, 8, 4402–4407 Search PubMed.
- T. H. Meyer, L. H. Finger, P. Gandeepan and L. Ackermann, Resource Economy by Metallaelectrocatalysis: Merging Electrochemistry and C H Activation, Trends Chem., 2019, 1, 63–76 Search PubMed.
-
(a) S. Warratz, C. Kornhaaß, A. Cajaraville, B. Niepötter, D. Stalke and L. Ackermann, Ruthenium(II)-Catalyzed C−H Activation/Alkyne Annulation by Weak Coordination with O2 as the Sole Oxidant, Angew. Chem., Int. Ed., 2015, 54, 5513–5517 Search PubMed;
(b) J. Piera and J.-E. Bäckvall, Catalytic Oxidation of Organic Substrates by Molecular Oxygen and Hydrogen Peroxide by Multistep Electron Transfer—A Biomimetic Approach, Angew. Chem., Int. Ed., 2008, 47, 3506–3523 Search PubMed;
(c) S. S. Stahl, Palladium Oxidase Catalysis: Selective Oxidation of Organic Chemicals by Direct Dioxygen-Coupled Turnover, Angew. Chem., Int. Ed., 2004, 43, 3400–3420 Search PubMed;
(d) R. Mei, H. Wang, S. Warratz, S. A. Macgregor and L. Ackermann, Cobalt-Catalyzed Oxidase C−H/N−H Alkyne Annulation: Mechanistic Insights and Access to Anticancer Agents, Chem. – Eur. J., 2016, 22, 6759–6763 Search PubMed.
- P. M. Osterberg, J. K. Niemeier, C. J. Welch, J. M. Hawkins, J. R. Martinelli, T. E. Johnson, T. W. Root and S. S. Stahl, Experimental Limiting Oxygen Concentrations for Nine Organic Solvents at Temperatures and Pressures Relevant to Aerobic Oxidations in the Pharmaceutical Industry, Org. Process Res. Dev., 2015, 19, 1537–1543 Search PubMed.
-
(a) H. Kolbe and E. Lautemann, Ueber die Constitution und Basicität der Salicylsäure, Justus Liebigs Ann. Chem., 1860, 115, 157–206 Search PubMed;
(b) M. Faraday, XX. On new compounds of carbon and hydrogen, and on certain other products obtained during the decomposition of oil by heat, Philos. Trans. R. Soc. London, 1825, 115, 440–466 Search PubMed.
- T. Shono, Electroorganic chemistry in organic synthesis, Tetrahedron, 1984, 40, 811–850 Search PubMed.
-
(a) D. Pollok and S. R. Waldvogel, Electro-organic synthesis – a 21st century technique, Chem. Sci., 2020, 11, 12386–12400 Search PubMed;
(b) J. Liu, L. Lu, D. Wood and S. Lin, New Redox Strategies in Organic Synthesis by Means of Electrochemistry and Photochemistry, ACS Cent. Sci., 2020, 6, 1317–1340 Search PubMed;
(c) R. D. Little, A Perspective on Organic Electrochemistry, J. Org. Chem., 2020, 85, 13375–13390 Search PubMed;
(d) K.-J. Jiao, Y.-K. Xing, Q.-L. Yang, H. Qiu and T.-S. Mei, Site-Selective C–H Functionalization via Synergistic Use of Electrochemistry and Transition Metal Catalysis, Acc. Chem. Res., 2020, 53, 300–310 Search PubMed;
(e) P. Xiong and H.-C. Xu, Chemistry with Electrochemically Generated N-Centered Radicals, Acc. Chem. Res., 2019, 52, 3339–3350 Search PubMed;
(f) S. Tang, Y. Liu and A. Lei, Electrochemical Oxidative Cross-coupling with Hydrogen Evolution: A Green and Sustainable Way for Bond Formation, Chem, 2018, 4, 27–45 Search PubMed;
(g) K. D. Moeller, Using Physical Organic Chemistry To Shape the Course of Electrochemical Reactions, Chem. Rev., 2018, 118, 4817–4833 Search PubMed;
(h) M. Yan, Y. Kawamata and P. S. Baran, Synthetic Organic Electrochemical Methods Since 2000: On the Verge of a Renaissance, Chem. Rev., 2017, 117, 13230–13319 Search PubMed;
(i) A. Jutand, Contribution of Electrochemistry to Organometallic Catalysis, Chem. Rev., 2008, 108, 2300–2347 Search PubMed;
(j) H. Kolbe, Untersuchungen über die Elektrolyse organischer Verbindungen, Justus Liebigs Ann. Chem., 1849, 69, 257–294 Search PubMed;
(k) M. Faraday, Sechste Reihe von Experimental-Untersuchungen über Elektricität, Ann. Phys., 1834, 109, 149–189 Search PubMed.
-
(a) R. C. Samanta, T. H. Meyer, I. Siewert and L. Ackermann, Renewable resources for sustainable metallaelectro-catalysed C–H activation, Chem. Sci., 2020, 11, 8657–8670 Search PubMed;
(b) L. Ackermann, Metalla-electrocatalyzed C–H Activation by Earth-Abundant 3d Metals and Beyond, Acc. Chem. Res., 2020, 53, 84–104 Search PubMed;
(c) Q.-L. Yang, P. Fang and T.-S. Mei, Recent Advances in Organic Electrochemical C−H Functionalization, Chin. J. Chem, 2018, 36, 338–352 Search PubMed;
(d) N. Sauermann, T. H. Meyer, Y. Qiu and L. Ackermann, Electrocatalytic C–H Activation, ACS Catal., 2018, 8, 7086–7103 Search PubMed;
(e) C. Ma, P. Fang and T.-S. Mei, Recent Advances in C–H Functionalization Using Electrochemical Transition Metal Catalysis, ACS Catal., 2018, 8, 7179–7189 Search PubMed.
- C. Amatore, C. Cammoun and A. Jutand, Electrochemical Recycling of Benzoquinone in the Pd/Benzoquinone-Catalyzed Heck-Type Reactions from Arenes, Adv. Synth. Catal., 2007, 349, 292–296 Search PubMed.
- F. Kakiuchi, T. Kochi, H. Mutsutani, N. Kobayashi, S. Urano, M. Sato, S. Nishiyama and T. Tanabe, Palladium-Catalyzed Aromatic C−H Halogenation with Hydrogen Halides by Means of Electrochemical Oxidation, J. Am. Chem. Soc., 2009, 131, 11310–11311 Search PubMed.
- Y. B. Dudkina, D. Y. Mikhaylov, T. V. Gryaznova, A. I. Tufatullin, O. N. Kataeva, D. A. Vicic and Y. H. Budnikova, Electrochemical Ortho Functionalization of 2-Phenylpyridine with Perfluorocarboxylic Acids Catalyzed by Palladium in Higher Oxidation States, Organometallics, 2013, 32, 4785–4792 Search PubMed.
- M. Konishi, K. Tsuchida, K. Sano, T. Kochi and F. Kakiuchi, Palladium-Catalyzed ortho-Selective C–H Chlorination of Benzamide Derivatives under Anodic Oxidation Conditions, J. Org. Chem., 2017, 82, 8716–8724 Search PubMed.
- Q.-L. Yang, Y.-Q. Li, C. Ma, P. Fang, X.-J. Zhang and T.-S. Mei, Palladium-Catalyzed C(sp3)−H Oxygenation via Electrochemical Oxidation, J. Am. Chem. Soc., 2017, 139, 3293–3298 Search PubMed.
-
(a) Q.-L. Yang, C.-Z. Li, L.-W. Zhang, Y.-Y. Li, X. Tong, X.-Y. Wu and T.-S. Mei, Palladium-Catalyzed Electrochemical C–H Alkylation of Arenes, Organometallics, 2019, 38, 1208–1212 Search PubMed;
(b) C. Ma, C.-Q. Zhao, Y.-Q. Li, L.-P. Zhang, X.-T. Xu, K. Zhang and T.-S. Mei, Palladium-catalyzed C–H activation/C–C cross-coupling reactions via electrochemistry, Chem. Commun., 2017, 53, 12189–12192 Search PubMed.
-
(a) L. Yang, R. Steinbock, A. Scheremetjew, R. Kuniyil, L. H. Finger, A. M. Messinis and L. Ackermann, Azaruthena(II)-bicyclo[3.2.0]heptadiene: Key Intermediate for Ruthenaelectro(II/III/I)-catalyzed Alkyne Annulations, Angew. Chem., 2020, 59, 11130–11135 Search PubMed;
(b) X. Tan, X. Hou, T. Rogge and L. Ackermann, Ruthenaelectro-Catalyzed Domino Three-Component Alkyne Annulation Expedient Isoquinoline Assembly, Angew. Chem., 2020, 60, 4619–4624 Search PubMed;
(c) L. Massignan, X. Tan, T. H. Meyer, R. Kuniyil, A. M. Messinis and L. Ackermann, C−H Oxygenation Reactions Enabled by Dual Catalysis with Electrogenerated Hypervalent Iodine Species and Ruthenium Complexes, Angew. Chem., 2020, 59, 3184–3189 Search PubMed;
(d) Z.-Q. Wang, C. Hou, Y.-F. Zhong, Y.-X. Lu, Z.-Y. Mo, Y.-M. Pan and H.-T. Tang, Electrochemically Enabled Double C–H Activation of Amides: Chemoselective Synthesis of Polycyclic Isoquinolinones, Org. Lett., 2019, 21, 9841–9845 Search PubMed;
(e) M.-J. Luo, T.-T. Zhang, F.-J. Cai, J.-H. Li and D.-L. He, Decarboxylative [4 + 2] annulation of arylglyoxylic acids with internal alkynes using the anodic ruthenium catalysis, Chem. Commun., 2019, 55, 7251–7254 Search PubMed;
(f) M.-J. Luo, M. Hu, R.-J. Song, D.-L. He and J.-H. Li, Ruthenium(II)-catalyzed electrooxidative [4 + 2] annulation of benzylic alcohols with internal alkynes: entry to isocoumarins, Chem. Commun., 2019, 55, 1124–1127 Search PubMed;
(g) F. Xu, Y.-J. Li, C. Huang and H.-C. Xu, Ruthenium-Catalyzed Electrochemical Dehydrogenative Alkyne Annulation, ACS Catal., 2018, 8, 3820–3824 Search PubMed;
(h) Y. Qiu, C. Tian, L. Massignan, T. Rogge and L. Ackermann, Electrooxidative Ruthenium-Catalyzed C−H/O−H Annulation by Weak O-Coordination, Angew. Chem., Int. Ed., 2018, 57, 5818–5822 Search PubMed;
(i) R. Mei, J. Koeller and L. Ackermann, Electrochemical ruthenium-catalyzed alkyne annulations by C–H/Het–H activation of aryl carbamates or phenols in protic media, Chem. Commun., 2018, 54, 12879–12882 Search PubMed.
-
(a) W.-J. Kong, Z. Shen, L. H. Finger and L. Ackermann, Electrochemical Access to Aza-Polycyclic Aromatic Hydrocarbons: Rhoda-Electrocatalyzed Domino Alkyne Annulations, Angew. Chem., 2020, 59, 5551–5556 Search PubMed;
(b) Z.-J. Wu, F. Su, W. Lin, J. Song, T.-B. Wen, H.-J. Zhang and H.-C. Xu, Scalable Rhodium(III)-Catalyzed Aryl C−H Phosphorylation Enabled by Anodic Oxidation Induced Reductive Elimination, Angew. Chem., Int. Ed., 2019, 58, 16770–16774 Search PubMed;
(c) W.-J. Kong, L. H. Finger, J. C. A. Oliveira and L. Ackermann, Rhodaelectrocatalysis for Annulative C−H Activation: Polycyclic Aromatic Hydrocarbons through Versatile Double Electrocatalysis, Angew. Chem., Int. Ed., 2019, 58, 6342–6346 Search PubMed;
(d) W.-J. Kong, L. H. Finger, A. M. Messinis, R. Kuniyil, J. C. A. Oliveira and L. Ackermann, Flow Rhodaelectro-Catalyzed Alkyne Annulations by Versatile C–H Activation: Mechanistic Support for Rhodium(III/IV), J. Am. Chem. Soc., 2019, 141, 17198–17206 Search PubMed;
(e) Y. Qiu, A. Scheremetjew and L. Ackermann, Electro-Oxidative C–C Alkenylation by Rhodium(III) Catalysis, J. Am. Chem. Soc., 2019, 141, 2731–2738 Search PubMed;
(f) Y. Qiu, W.-J. Kong, J. Struwe, N. Sauermann, T. Rogge, A. Scheremetjew and L. Ackermann, Electrooxidative Rhodium-Catalyzed C−H/C−H Activation: Electricity as Oxidant for Cross-Dehydrogenative Alkenylation, Angew. Chem., Int. Ed., 2018, 57, 5828–5832 Search PubMed.
-
(a) Q.-L. Yang, Y.-K. Xing, X.-Y. Wang, H.-X. Ma, X.-J. Weng, X. Yang, H.-M. Guo and T.-S. Mei, Electrochemistry-Enabled Ir-Catalyzed Vinylic C–H Functionalization, J. Am. Chem. Soc., 2019, 141, 18970–18976 Search PubMed;
(b) Y. Qiu, M. Stangier, T. H. Meyer, J. C. A. Oliveira and L. Ackermann, Iridium-Catalyzed Electrooxidative C−H Activation by Chemoselective Redox-Catalyst Cooperation, Angew. Chem., Int. Ed., 2018, 57, 14179–14183 Search PubMed.
- L. Ackermann, J. Pospech, K. Graczyk and K. Rauch, Versatile Synthesis of Isocoumarins and α-Pyrones by Ruthenium-Catalyzed Oxidative C–H/O–H Bond Cleavages, Org. Lett., 2012, 14, 930–933 Search PubMed.
- R. Mandal and B. Sundararaju, Cp*Co(III)-Catalyzed Annulation of Carboxylic Acids with Alkynes, Org. Lett., 2017, 19, 2544–2547 Search PubMed.
- P. Gandeepan, L. H. Finger, T. H. Meyer and L. Ackermann, 3d metallaelectrocatalysis for resource economical syntheses, Chem. Soc. Rev., 2020, 49, 4254–4272 Search PubMed.
- N. Sauermann, T. H. Meyer, C. Tian and L. Ackermann, Electrochemical Cobalt-Catalyzed C–H Oxygenation at Room Temperature, J. Am. Chem. Soc., 2017, 139, 18452–18455 Search PubMed.
- L.-B. Zhang, X.-Q. Hao, S.-K. Zhang, Z.-J. Liu, X.-X. Zheng, J.-F. Gong, J.-L. Niu and M.-P. Song, Cobalt-Catalyzed C(sp2)−H Alkoxylation of Aromatic and Olefinic Carboxamides, Angew. Chem., Int. Ed., 2015, 54, 272–275 Search PubMed.
-
(a) R. Mei, U. Dhawa, R. C. Samanta, W. Ma, J. Wencel-Delord and L. Ackermann, Cobalt-Catalyzed Oxidative C−H Activation: Strategies and Concepts, ChemSusChem, 2020, 13, 3306–3356 Search PubMed;
(b) N. Sauermann, T. H. Meyer and L. Ackermann, Electrochemical Cobalt-Catalyzed C−H Activation, Chem. – Eur. J., 2018, 24, 16209–16217 Search PubMed.
- N. Sauermann, R. Mei and L. Ackermann, Electrochemical C−H Amination by Cobalt Catalysis in a Renewable Solvent, Angew. Chem., Int. Ed., 2018, 57, 5090–5094 Search PubMed.
- L.-B. Zhang, S.-K. Zhang, D. Wei, X. Zhu, X.-Q. Hao, J.-H. Su, J.-L. Niu and M.-P. Song, Cobalt(II)-Catalyzed C–H Amination of Arenes with Simple Alkylamines, Org. Lett., 2016, 18, 1318–1321 Search PubMed.
- X. Gao, P. Wang, L. Zeng, S. Tang and A. Lei, Cobalt(II)-Catalyzed Electrooxidative C–H Amination of Arenes with Alkylamines, J. Am. Chem. Soc., 2018, 140, 4195–4199 Search PubMed.
- C. Tian, L. Massignan, T. H. Meyer and L. Ackermann, Electrochemical C−H/N−H Activation by Water-Tolerant Cobalt Catalysis at Room Temperature, Angew. Chem., Int. Ed., 2018, 57, 2383–2387 Search PubMed.
- S. Tang, D. Wang, Y. Liu, L. Zeng and A. Lei, Cobalt-catalyzed electrooxidative C-H/N-H [4 + 2] annulation with ethylene or ethyne, Nat. Commun., 2018, 9, 798 Search PubMed.
- R. Mei, N. Sauermann, J. C. A. Oliveira and L. Ackermann, Electroremovable Traceless Hydrazides for Cobalt-Catalyzed Electro-Oxidative C–H/N–H Activation with Internal Alkynes, J. Am. Chem. Soc., 2018, 140, 7913–7921 Search PubMed.
-
(a) R. Mei, X. Fang, L. He, J. Sun, L. Zou, W. Ma and L. Ackermann, Cobaltaelectro-catalyzed oxidative allene annulation by electro-removable hydrazides, Chem. Commun., 2020, 56, 1393–1396 Search PubMed;
(b) T. H. Meyer, J. C. A. Oliveira, S. C. Sau, N. W. J. Ang and L. Ackermann, Electrooxidative Allene Annulations by Mild Cobalt-Catalyzed C–H Activation, ACS Catal., 2018, 8, 9140–9147 Search PubMed.
- S. C. Sau, R. Mei, J. Struwe and L. Ackermann, Cobaltaelectro-Catalyzed C−H Activation with Carbon Monoxide or Isocyanides, ChemSusChem, 2019, 12, 3023–3027 Search PubMed.
- R. Mei, W. Ma, Y. Zhang, X. Guo and L. Ackermann, Cobaltaelectro-Catalyzed Oxidative C–H/N–H Activation with 1,3-Diynes by Electro-Removable Hydrazides, Org. Lett., 2019, 21, 6534–6538 Search PubMed.
- U. Dhawa, C. Tian, W. Li and L. Ackermann, Cobalta-Electrocatalyzed C–H Allylation with Unactivated Alkenes, ACS Catal., 2020, 10, 6457–6462 Search PubMed.
-
(a) T. Yamaguchi, Y. Kommagalla, Y. Aihara and N. Chatani, Cobalt-catalyzed chelation assisted C–H allylation of aromatic amides with unactivated olefins, Chem. Commun., 2016, 52, 10129–10132 Search PubMed;
(b) R. Manoharan, G. Sivakumar and M. Jeganmohan, Cobalt-catalyzed C–H olefination of aromatics with unactivated alkenes, Chem. Commun., 2016, 52, 10533–10536 Search PubMed;
(c) S. Maity, R. Kancherla, U. Dhawa, E. Hoque, S. Pimparkar and D. Maiti, Switch to Allylic Selectivity in Cobalt-Catalyzed Dehydrogenative Heck Reactions with Unbiased Aliphatic Olefins, ACS Catal., 2016, 6, 5493–5499 Search PubMed.
- T. H. Meyer, G. A. Chesnokov and L. Ackermann, Cobalta-Electrocatalyzed C−H Activation in Biomass-Derived Glycerol: Powered by Renewable Wind and Solar Energy, ChemSusChem, 2020, 13, 668–671 Search PubMed.
- S.-K. Zhang, R. C. Samanta, A. Del Vecchio and L. Ackermann, Evolution of High-Valent Nickela-Electrocatalyzed C−H Activation: From Cross(-Electrophile)-Couplings to Electrooxidative C−H Transformations, Chem. – Eur. J., 2020, 26, 10936–10947 Search PubMed.
- S.-K. Zhang, R. C. Samanta, N. Sauermann and L. Ackermann, Nickel-Catalyzed Electrooxidative C−H Amination: Support for Nickel(IV), Chem. – Eur. J., 2018, 24, 19166–19170 Search PubMed.
- S.-K. Zhang, J. Struwe, L. Hu and L. Ackermann, Nickela-electrocatalyzed C−H Alkoxylation with Secondary Alcohols: Oxidation-Induced Reductive Elimination at Nickel(III), Angew. Chem., 2020, 59, 3178–3183 Search PubMed.
- R. C. Samanta, J. Struwe and L. Ackermann, Nickela-electrocatalyzed Mild C−H Alkylations at Room Temperature, Angew. Chem., 2020, 59, 14154–14159 Search PubMed.
-
(a) Y. Aihara, M. Tobisu, Y. Fukumoto and N. Chatani, Ni(II)-Catalyzed Oxidative Coupling between C(sp2)–H in Benzamides and C(sp3)–H in Toluene Derivatives, J. Am. Chem. Soc., 2014, 136, 15509–15512 Search PubMed;
(b) Z. Ruan, S. Lackner and L. Ackermann, A General Strategy for the Nickel-Catalyzed C−H Alkylation of Anilines, Angew. Chem., Int. Ed., 2016, 55, 3153–3157 Search PubMed;
(c) T. Uemura, M. Yamaguchi and N. Chatani, Phenyltrimethylammonium Salts as Methylation Reagents in the Nickel-Catalyzed Methylation of C−H Bonds, Angew. Chem., Int. Ed., 2016, 55, 3162–3165 Search PubMed.
- Q.-L. Yang, X.-Y. Wang, J.-Y. Lu, L.-P. Zhang, P. Fang and T.-S. Mei, Copper-Catalyzed Electrochemical C–H Amination of Arenes with Secondary Amines, J. Am. Chem. Soc., 2018, 140, 11487–11494 Search PubMed.
- S. Kathiravan, S. Suriyanarayanan and I. A. Nicholls, Electrooxidative Amination of sp2 C–H Bonds: Coupling of Amines with Aryl Amides via Copper Catalysis, Org. Lett., 2019, 21, 1968–1972 Search PubMed.
- C. Tian, U. Dhawa, A. Scheremetjew and L. Ackermann, Cupraelectro-Catalyzed Alkyne Annulation: Evidence for Distinct C–H Alkynylation and Decarboxylative C–H/C–C Manifolds, ACS Catal., 2019, 9, 7690–7696 Search PubMed.
-
(a) A. Fürstner, Iron Catalysis in Organic Synthesis: A Critical Assessment of What It Takes To Make This Base Metal a Multitasking Champion, ACS Cent. Sci., 2016, 2, 778–789 Search PubMed;
(b) I. Bauer and H.-J. Knölker, Iron Catalysis in Organic Synthesis, Chem. Rev., 2015, 115, 3170–3387 Search PubMed;
(c) S. Enthaler, K. Junge and M. Beller, Sustainable Metal Catalysis with Iron: From Rust to a Rising Star?, Angew. Chem., Int. Ed., 2008, 47, 3317–3321 Search PubMed;
(d) A. Correa, O. García Mancheño and C. Bolm, Iron-catalysed carbon–heteroatom and heteroatom–heteroatom bond forming processes, Chem. Soc. Rev., 2008, 37, 1108–1117 Search PubMed.
- R. Shang, L. Ilies and E. Nakamura, Iron-Catalyzed C–H Bond Activation, Chem. Rev., 2017, 117, 9086–9139 Search PubMed.
- C. Zhu, M. Stangier, J. C. A. Oliveira, L. Massignan and L. Ackermann, Iron-Electrocatalyzed C−H Arylations: Mechanistic Insights into Oxidation-Induced Reductive Elimination for Ferraelectrocatalysis, Chem. – Eur. J., 2019, 25, 16382–16389 Search PubMed.
-
(a) K. E. Poremba, S. E. Dibrell and S. E. Reisman, Nickel-Catalyzed Enantioselective Reductive Cross-Coupling Reactions, ACS Catal., 2020, 10, 8237–8246 Search PubMed;
(b) X. Chang, Q. Zhang and C. Guo, Asymmetric Electrochemical Transformations, Angew. Chem., 2020, 59, 12612–12622 Search PubMed;
(c) Q. Lin, L. Li and S. Luo, Asymmetric Electrochemical Catalysis, Chem. – Eur. J., 2019, 25, 10033–10044 Search PubMed;
(d) M. Ghosh, V. S. Shinde and M. Rueping, A review of asymmetric synthetic organic electrochemistry and electrocatalysis: concepts, applications, recent developments and future directions, Beilstein J. Org. Chem., 2019, 15, 2710–2746 Search PubMed.
- U. Dhawa, C. Tian, T. Wdowik, J. C. A. Oliveira, J. Hao and L. Ackermann, Enantioselective Pallada-Electrocatalyzed C−H Activation by Transient Directing Groups: Expedient Access to Helicenes, Angew. Chem., Int. Ed., 2020, 59, 13451–13457 Search PubMed.
|
This journal is © the Partner Organisations 2021 |
Click here to see how this site uses Cookies. View our privacy policy here.