DOI:
10.1039/D4RA03095H
(Review Article)
RSC Adv., 2024,
14, 20163-20181
Molecularly imprinted polymer-based electrochemical sensors for monitoring the persistent organic pollutants chlorophenols
Received
26th April 2024
, Accepted 30th May 2024
First published on 24th June 2024
Abstract
Because of the serious risks they pose to the environment and public health, chlorophenols (CPs), a typical class of the most persistent organic pollutants, have drawn increasing attention. Monitoring CPs effectively has become a pressing and difficult problem. The rapidly increasing need for onsite and real-time CP detection has led to the consideration of electrochemical sensing as a workable solution. Molecularly imprinted polymer (MIP)-based electrochemical sensing has emerged as a promising area for environmental monitoring in response to this analytical problem. MIPs, in conjunction with miniature electrochemical transducers, provide the opportunity to detect target analytes in situ. These devices have the advantages of great chemical and physical stability, cheap production costs, good selectivity, and quick response times. Most studies suggest that these sensors use nanoparticles to improve their analytical properties, especially sensitivity. Furthermore, these sensors have successfully used real water samples without the need for time-consuming pretreatment procedures. This article provides an overview of electrochemical MIP-based sensors reported to detect CPs in water samples. To obtain the highest sensitivity, special consideration is given to the fabrication of the sensors, which includes the use of various functional monomers, sensing platforms, and materials. Several other parameters are also discussed, including the linear concentration range, limit of detection, and the types of water samples that were examined.
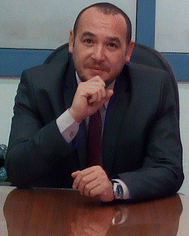 Prof. Ayman H. Kamel | Prof. Kamel completed his PhD from Ain Shams University, Cairo, Egypt, with a focus on the fabrication and characterization of electrochemical sensors for environmental, biomedical and pharmaceutical analyses. Prof. Kamel has published over 137 research articles in well-reputed, high-impact factor international journals. He is also the author of several book chapters. He has served as a guest editor, member, referee, and reviewer for many internationally renowned scientific journals. He has successfully completed several research projects as Principal Investigator. Professor Ayman H. Kamel is a leading figure in the field of sensors and their applications. His research primarily focuses on developing novel sensor technologies and exploring their potential applications in various fields, such as healthcare, environmental monitoring, and industrial automation. Prof. Kamel has made significant contributions to the development of sensors with improved sensitivity, selectivity, and reliability. His work often involves interdisciplinary collaboration, combining principles from fields such as materials science, electrical engineering, and chemistry to advance sensor technology. One of his notable achievements is in the area of biomedical sensors, where he has worked on developing sensors for early disease detection, monitoring physiological parameters, and drug delivery systems. These sensors have the potential to revolutionize healthcare by providing real-time monitoring and personalized treatment options. |
1. Introduction
Water is vital to life and the environment, and its availability is critical to agriculture because it plays a major role in boosting grain yields.1 Apart from its use in agriculture and drinking, water also serves as a solvent in biological chemistry and the natural world.2 An article by the World Health Organization (WHO) has reported that only 25% of people on the earth have access to clean drinking water, with developing nations experiencing a worse problem than developed ones.3 Wastewater recycling and reuse have received considerable attention as a means of meeting the world's growing water demand. Organic substances typically contaminate this wastewater. Water pollution is a major environmental issue that plagues many nations, as both natural and man-made activities frequently contaminate various streams and rivers. Water pollution is caused by hazardous compounds contaminating streams, bays, lakes, and/or seas that directly affect living things.4
It has been reported that wastewater contains significant concentrations of phenols and their derivatives, which are widely used compounds in daily life.5 One group of pollutants with an organic origin is chlorophenols (CPs).6 These are a class of phenolic organochlorides with one or more covalently bonded chlorine atoms. Fan et al. classified them into five groups: dichlorophenols (DCPs), trichlorophenols (TCPs), tetrachlorophenols (TeCPs), pentachlorophenols (PCPs), and mono-chlorophenols (2-, 3-, and 4-chlorophenols).7 Chlorophenols negatively impact the human nervous and respiratory systems, posing major health risks. In addition to being poisonous and carcinogenic, they are persistent in the environment, have a strong odor, and are poorly biodegradable.8–10 Table 1 lists some of the features of chlorophenols.
Table 1 Physical–chemical properties of chlorophenolsa11
Compound |
Molar mass, g mol−1 |
Boiling point (°C) |
Melting point (°C) |
Solubility in water, g L−1 at 20 °C |
pKa |
na: not available. |
2-Chlorophenol |
128.56 |
174.9 |
93 |
28 |
8.3–8.6 |
3-Chlorophenol |
128.56 |
214 |
33–34 |
26 |
8.8–9.1 |
4-Chlorophenol |
128.56 |
217–219 |
42–44 |
27 |
9.1–9.4 |
2,3-Dichlorophenol |
163.00 |
206 |
57–58 |
na |
6.4–7.8 |
2,4-Dichlorophenol |
163.00 |
210 |
45 |
4.50 |
7.5–8.1 |
2,6-Dichlorophenol |
163.00 |
219 |
68 |
na |
6.7–7.8 |
3,5-Dichlorophenol |
163.00 |
233 |
68 |
na |
6.9–8.3 |
2,3,4-Trichlorophenol |
197.45 |
Sublimes |
77–84 |
0.22 |
6.5–7.7 |
2,3,5-Trichlorophenol |
197.45 |
248–255 |
57–62 |
0.22 |
6.8–7.4 |
2,4,5-Trichlorophenol |
197.45 |
Sublimes |
67–70 |
0.948 |
7.0–7.7 |
2,4,6-Trichlorophenol |
197.45 |
243–249 |
69 |
0.434 |
6.0–7.4 |
3,4,5-Trichlorophenol |
197.45 |
271–277 |
101 |
na |
7.7–7.8 |
2,3,4,5-Tetrachlorophenol |
231.89 |
Sublimes |
116–117 |
0.166 |
6.2–7.0 |
2,3,4,6-Tetrachlorophenol |
231.89 |
150 |
70 |
0.183 |
5.3–6.6 |
2,3,5,6-Tetrachlorophenol |
231.89 |
188 |
114–116 |
0.100 |
5.2–5.5 |
1.1. Applications of chlorophenols
Chlorophenols are a significant class of organic chemicals with a wide range of application in the pharmaceutical and agricultural sectors. They are widely employed in agriculture as fungicides, herbicides, rodenticides, germicides, and other pesticides to boost crop yield and combat various invaders and diseases that often result in significant losses to cereal crops.12,13 4-Chlorophenol is the first step in producing 2-benzyl-4-chlorophenol germicides. The manufacturing of analgesics known as acetophenetidin also utilizes it. The main use of 2,4-DCP is in the formation of 4-dichlorophenoxyacetic acid, a common herbicide that is widely used in agricultural production. Chlorophenols are the building blocks for chemicals such as polychlorophenoxyphenols and extremely hazardous polychlorodibenzofurans. The production of anti-helminthic and anti-bacterial medications, such as triclosan, widely uses it.14
1.2. Risks associated with chlorophenols
Chlorophenols are one of the many organic chemicals that make up a class of extremely dangerous substances that can resist mineralization for an extended period in the natural world. Urine, adipose tissues, sediments, groundwater, animal milk, and the bodies of aquatic animals all contain these substances. Water tainted with chlorophenols has an offensive stench. The World Health Organization and the United States Environmental Protection Agency have jointly advised that the levels of chlorophenols in drinking water and industrial effluent should not surpass 0.5 μg L−1 and 100 μg L−1, respectively. According to Younis et al., the primary sources of chlorophenols in the environment are effluents from coal, oil, petrochemical refineries, leather, plastics, wood, dye and paint, insecticides, and pesticides, as well as water and pharmaceutical chlorination, textile, and paper industries. Their use as biocidal agents has a negative impact on ecosystems.15 When these chemicals evaporate from treated wood products, they begin their journey into the atmosphere. Rain and snow transport these compounds from the atmosphere to the ground. Leaching from treated wood products and spills at industrial and hazardous waste sites releases these compounds into the soil. Individuals primarily encounter these compounds, which are usually used as wood preservatives, when they touch painted objects. The majority of those affected met the contaminated environment around wood treatment facilities and municipal waste sites. Exposure to these compounds can also occur through breathing in polluted air, eating contaminated food, drinking contaminated ground water, and meeting contaminated soil and items. When they meet the body in vapor form, they have extremely harmful and burning effects on the mouth, eyes, and skin. When eaten with meals, they gradually accumulate in the liver, kidneys, spleen, fat, and plasma protein. They damage vital organs, such as the kidney, liver, stomach, small and large intestines (gastrointestinal tract); blood circulation system; and even the immune system, and their effects only become worse from there. Because they are mutagenic, carcinogenic, genotoxic, and teratogenic, they are dangerous to people, aquatic life, and wildlife. People consider them potent compounds that influence hormone levels. Once adsorbed, these substances severely impact an animal's immune system, reproductive system, endocrine system, and central nervous system. These substances are believed to make zebrafish more feminine.16 These substances can change a wide range of biological pathways in both plants and animals, and the degree of chlorine substitution in the benzene ring affects how poisonous they are. Changes in pH significantly alter their toxicities, with toxicity generally increasing as the pH of the solution decreases. The DNA helix unbinds because of its mutagenic action. According to some authors, human Syrian hamster embryo cells exhibit suppression of DNA synthesis and gene mutation. When human growth hormone levels are high, changes occur. Researchers have also linked chlorophenols to the development of non-Hodgkin lymphatic system neoplasmic changes.17 Because of their caustic and poisonous properties, lung and throat infections accompanied by coughing, wheezing, and dyspnea are extremely common, particularly in children. Increased perspiration, jerky movements, and twitching of the muscles are typical reactions to high temperatures, particularly during the summer. Additional studies indicate that prolonged exposure raises the risk of neurological illnesses and cancer.18–21
2. Molecular imprinted polymers (MIPs)
Recent years have seen the creation of new molecularly imprinted polymers (MIPs). These polymers recognize specific target molecules very well, but they are very strong, chemically stable, recyclable, and suitable for sensor applications.22,23 Additionally, electrochemical devices featuring molecular imprints offer several benefits, including recognition capacity, affordability, miniaturization, and automated equipment.12,24 Typically, a template molecule polymerizes functional monomers to form MIPs. Once the template molecules separate from the MIPs, the MIPs create holes that retain the targeted molecule. This allows strong chemisorption to find the target molecule. The literature frequently features studies that use sensors derived from a combination of electrochemical and molecular printing techniques to detect a wide variety of compounds. MIPs have several benefits, but they have certain drawbacks, such as limited conductivity and electrocatalytic activity, which reduce sensitivity. Therefore, researchers have used various nanomaterials, such as quantum dots (QDs), metal organic frameworks (MOFs), Au, Pt, and Ni nanoparticles/nanowires, as well as Pt/Au and Au/Ag bimetallic nanoparticles, to boost their conductivity.22,25 Therefore, we can conclude that MIPs enhance the selectivity of nano-sensors, while nanomaterials boost the sensitivity of MIP-based electrochemical sensors.
2.1. MIP definition
Biological recognition mechanisms, such as receptors, enzymes, and antibodies, which are useful only under moderate conditions, have traditionally inspired MIPs, as demonstrated by the groundbreaking molecular imprinting investigations.26,27 Extreme pH and temperature, along with the denaturation of proteins in organic solvents, adversely affect biomolecules. They must also remain immobile on the electrode surface, which makes covering various surfaces, including transducer surfaces, challenging. Furthermore, the production of these biomolecules takes a long time and is often expensive. Researchers have created artificial receptors to overcome these restrictions.28,29 MIPs are a unique class of polymers shaped by the volume, form, and molecular structure of the template molecule.26 It has numerous benefits, including the ability to recognize a large range of targets due to its custom character, excellent chemical and physical stability, compatibility with organic media, potential for reusability, ease of engineering, and low cost. MIPs are used in many ways because of these properties. They are used in solid-phase extraction, affinity separation, immunoassays, biochemical sensing, controlled drug release, direct synthesis, and catalysis.27 MIPs are also easy to make and do not cost too much. They are very selective and affine to the molecule used in the imprinting process, and their longer degradation times allow the recognition sites to stay active for longer periods at room temperature. MIPs have garnered significant attention due to these advantages, leading to their application in various settings, including biomolecule processing. Moreover, researchers continue to explore MIPs in various processing applications, such as separation science, solid-phase extraction, chromatography, sensors, and catalysis.30 The process of molecular imprinting is simple: a cross-linker and an initiator copolymerize a functional monomer or a series of monomer units in the presence of template molecules. The functional monomer has unique functional groups that allow it to bind to the template molecules. After the polymerization process, the corresponding MIPs have stable linking moieties. Eliminating the template molecules entirely creates a space similar to the template in size, shape, and chemical functional groups, enabling the MIP to capture the analyte.31–33 The polymer matrix's microporous structure and microcavities that resemble those in the template molecule are the most important parts of molecular recognition.34–36
Three types of imprinting procedures, covalent, non-covalent, and semi covalent, can be distinguished based on how the functional monomer in the polymer and the target molecule rearrange themselves.37,38 Non-covalent imprinting is the most widely used technique for producing MIPs. Non-covalent interactions, such as hydrogen bonding, van der Waals forces, and electrostatic forces, form the complex of the template and functional monomer in situ. Rebinding template molecules to MIPs also occurs via the same non-covalent interactions between template molecules and MIPs. Several benefits of this approach include quick processing of template/monomer complexes, quick template extraction from polymers, easy template binding to MIPs, and applicability to a broad range of target molecules.39 Another method of generating MIPs is covalent imprinting. First, the formation of covalent bonds between a functional monomer and a template forms the complex before polymerization occurs. The established MIPs use the same kind of covalent collaboration to rebind template molecules after a chemical reaction eliminates the template. The flexibility to use a wide range of polymerization settings and the creation of stable and stoichiometric monomer/template complexes are the main benefits of this technology. Unfortunately, the limited applicability of monomer/template complexes is due to their laborious and expensive production, as well as the delayed release and binding of templates.40 Furthermore, reversible covalent interactions with polymerizable monomers are rare and usually require an acid hydrolysis process to break the covalent bonds between the template and the functional monomer because there are very few reversible covalent interactions and potential templates.41
The third method, referred to as semi-covalent imprinting, is a hybrid that combines covalent and noncovalent imprinting. Although the polymers are made in a manner akin to that of covalent imprinting, guest binding takes advantage of non-covalent interactions. Consequently, semi-covalent imprinting combines the key benefits of the two previously stated strategies: quick guest binding generated by non-covalent imprinting and the stable and stoichiometric complex produced by covalent imprinting.40,42
MIPs can be prepared in suspension, precipitation, emulsion, bulk, or multi-step polymerization processes using free radical polymerization (FRP), controlled radical polymerization (CRP), or electropolymerization. Table 2 presents the advantages and disadvantages of polymerization techniques for MIP synthesis.
Table 2 Benefits and drawbacks of MIP polymerization techniques41–48
Methods of polymerization |
Advantages |
Disadvantages |
Bulk polymerization |
Ease of preparation and simplicity |
Mechanical grinding and sieving procedures are time consuming |
Irregularities in the sizes or shapes of the generated particles |
MIP loading capacity decreased because of grinding |
Most of the binding sites inside the polymer matrix are inaccessible |
Suspension polymerization |
Uniform and consistent dispersion of MIP beads |
Special surfactant polymers are required due to in compatibility with water |
Ease (a single step) |
Emulsion polymerization |
Creation of uniform particle size spherical MIPs |
Using surfactants and water together can speed up interferences during polymerization |
Lack of need for expensive post-processing procedures, such as screening or grinding |
High efficiency of usage |
Quick polymerization coupled with a steady dispersion mechanism |
Precipitation polymerization |
It generates homogenous particle size spherical MIPs |
Overuse of solvents |
Increased response time |
Surfactants are not required |
The challenge of getting the solvent out of the polymer |
Multi-step swelling polymerization |
Uniformly distributed beads with regulated diameter |
Time-consuming and difficult processes (requiring particular reaction conditions) |
Manufacturing of consistent beads |
Outstanding particle for HPLC |
Core–shell polymerization |
Rapid extraction of template molecules |
Restricted ability to bind |
A larger surface area |
Postponed mass transfer |
Smaller particles |
Unfit for use in chemical sensing devices |
Quicker mass transfer |
2.2. Integration of MIPs in electrochemical sensors
2.2.1. In situ MIP sensors. It has been suggested that the in situ polymerization of imprinted thin films can be used to address the integration and compatibility between the MIP and the transducer. The objective is to enhance the diffusion kinetics and/or encourage the establishment of specific binding sites closer to the sensor surface.49 Moreover, improved film adhesion to the transducer surface and improved film thickness control are offered by in situ synthesis. Out of all the methods that have been suggested, we consider two here: electro-polymerization and grafting.For grafting, some researchers have suggested attaching initiators or polymerizable groups using various chemistries as an effective method for creating ultra-thin MIPs.50 Utilizing self-assembled monolayers (SAMs) of diazonium salts, silanes, or thiols on gold electrodes is the main way in which grafting is done. We then carry out the polymerization reaction directly on the electrode surface by dropping a solution that contains the template, monomer(s), cross-linker(s), or initiator depending on the immobilized agent. For example, Khlifi et al. discussed adding 4-benzoylphenyl groups electrochemically to the right diazonium salt. This made the MIP bind to it strongly and helped start the photosynthesis of a MIP for melamine detection.51 Kidakova et al. used a similar method to show for the first time that they could make a synthetic receptor based on MIP that could bind brain-derived neurotrophic factor (BDNF, Fig. 1) more strongly than other proteins.52
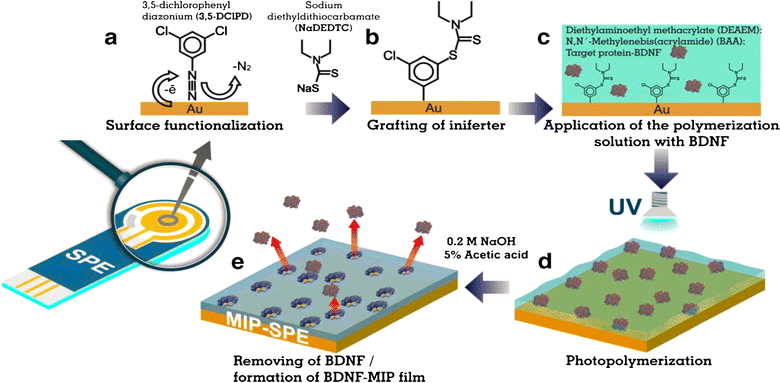 |
| Fig. 1 Schematic of the steps involved in surface-initiated polymerization.52 | |
The electropolymerization technique can accomplish the in situ synthesis of MIPs. This method offers better film adhesion to the transducer surface and control over film thickness. We have already shown an earlier example using oPD as monomer.53 Similarly, Ozcan synthesized a voltammetric paracetamol sensor by electrochemically combining pyrrole, the template, and electrolyte support to create a polypyrrole MIP film.54 This method has even synthesized MIPs directed towards bacteria (such as S. aureus, Fig. 2).55 Ma et al. proposed an intriguing method that involved coating a GCE with graphene nanoplatelets and gold nanoparticles, followed by modifying it with an MIP (Fig. 3) 56 to determine a prostate-specific antigen (PSA) by presenting a covalent bond between the protein (template) and glutaraldehyde through an imine reaction.
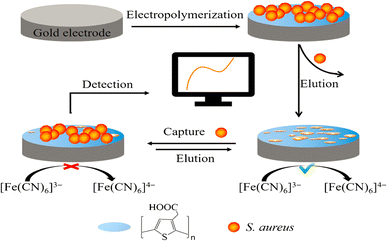 |
| Fig. 2 Schematic of the sensing approach for S. aureus sensing based on an electropolymerized MIP.55 | |
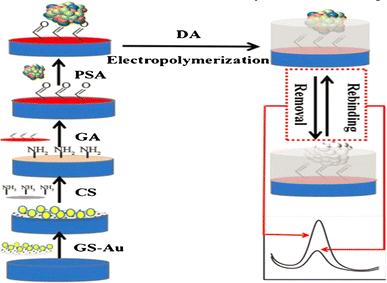 |
| Fig. 3 Schematic of the fabrication and detection principle of the voltammetric MIP-based sensor towards PSA.56 | |
2.2.2. Ex situ MIP sensors. Separating the synthesis and immobilization processes allows for the synthesis and characterization of the MIP particles, followed by their integration into the transducer under optimal conditions. We can simultaneously add electro-catalytic materials to enhance the electrochemical response. Typical methods usually involve physically entrapping the materials onto the electrode surface using a membrane or polymeric substances, such as polystyrene, sol–gel, or Nafion®, or incorporating them into a composite material that serves as the working electrode by combining them prior to their creation.Multiple reports indicate that the usual strategy is likely to incorporate MIP particles onto the electrode by entrapment. Sol–gel, Nafion®, chitosan, agarose, and glutaraldehyde, among others, are the most widely used methods to accomplish these. This method's primary benefit is that it makes it easy and quick to incorporate multiple materials at once; for example, MIP beads reinforced with graphene oxide sheets or other electrocatalysts. The concept is to maintain the particles within polymeric frameworks while preserving their characteristics and structure. To ensure homogeneous distribution, the sensing platform can employ various techniques, such as drop-casting and spin coating (Fig. 4).
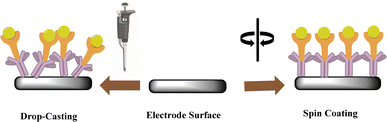 |
| Fig. 4 Schematic representation of the immobilization of the MIP particles. | |
2.3. Transduction mechanisms
Conductometry, capacitive impedance spectroscopy, potentiometry, chemical (ion-selective) field effect, amperometry, and voltammetry are the transduction methods that have been employed to create MIP-based electrochemical sensors. The recognition element should be sufficiently stiff to prevent deformation of the recognition sites under operating conditions because several of these approaches need vigorous, continuous agitation to achieve a steady regime.
2.3.1. Voltammetry. Voltammetric techniques include square wave voltammetry, differential pulse voltammetry, linear sweep voltammetry (LSV), and CV (SWV). These procedures subject the detecting system to the analyte's electroactivity. For electroactive molecules, the current is immediately monitored, and the optimal signal outputs are the faradaic currents produced by the oxidation or reduction of the analytes after binding to the MIP. In this instance, under carefully monitored circumstances, a correlation between the analyte concentration and the observed current can be made.57 Using redox probes, such as [Fe(CN)6]3−/4−, the signal can be generated when non-electroactive targets are involved.58
2.3.2. Amperometry. The concentration of electroactive species and the current measured at a constant voltage must be linearly related to performing amplification measurements. It can also be used to describe non-electroactive species that participate in an electrochemical reaction and a displacement step. The formation of a current requires the diffusion of species towards the working electrode and the outward diffusion of reaction byproducts; otherwise, the surface becomes passivated. Therefore, it is crucial to have porosity in the MIP layer (regardless of the type of recognition element) to provide the channels needed for species diffusion and electron transfer reactions at an exposed electrode surface. Following the template's selective extraction for measurement, electrons are subsequently exchanged with the electrode surface.
2.3.3. Potentiometry. To measure potential differences between two solutions containing charged species with differing activities, a membrane must be positioned between them. It is crucial to understand that a membrane potential can be created without the template being removed from the membrane for the fabrication of MIP sensors. This is advantageous because removing the template to leave binding-ready recognition sites is frequently a source of confusion or a sensitivity-limiting factor. The species do not need to diffuse through the membrane; hence, there are no size limits on the template compound in potentiometry. Despite these benefits, this form of transduction has received much attention in the literature (Fig. 5).
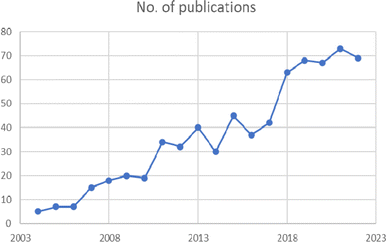 |
| Fig. 5 Plot of no. of publications versus year regarding MIP-based potentiometric sensors (Scopus database). | |
2.3.4. Chemical (ion-selective) field effect. Potentiometric systems can be used to create ion-sensitive field-effect transistors (ISFETs). A film can be applied to a semiconductor substrate to make it sensitive to changes in surface potential caused by chemical reactions or changes in charge at the film's location on the gate of a field-effect transducer. The current that circulates between the transistor's source and drain electrodes changes as a result. These devices have a high level of interest due to their ease of miniaturization, which calls for wafer-level preparation, strong adherence to substrates, and thickness control.
2.3.5. Capacitive impedance spectroscopy. Changes in the system's capacitance or impedance may accompany interfacial events. A completely impermeable, thin dielectric coating is typically required on gold substrates. Because the growth of the film is easy to control and electropolymerized polymers, such as phenol (non-conductive), are an excellent alternative, chemisorption of alkylthiols on a gold surface is necessary as an additional step to fill pores.59 The herbicide desmetryn and creatinine can both be detected using a photografting technique that uses acrylate derivatives as identification components.60,61 An imprinted SAM of mercaptans on a gold substrate has also been used for capacitance measurements.62
2.3.6. Conductometry. Conductometry relies on the movement of ions with opposing charges to establish the current flow when an electric field is created between two electrodes submerged in an electrolyte solution. The MIP must then be made into a membrane before an MIP-based conductometric sensor can be created. Because conductivity is cumulative and cannot distinguish between two ions, this transduction is the least sensitive of the electrochemical techniques. Ionic limiting equivalent conductance variations are too modest to distinguish across species. A very high concentration of one ion may also obscure the presence of other ions.
3. MIP-based sensors for chlorophenol (CP) detection
3.1. 4-Chlorophenol
An important industrial chemical, 4-chlorophenol (4-CP), is found in many colors, insecticides, biocides, clofibrate, and refined mineral oil solvents.63 However, 4-CP irritates the respiratory system and has negative effects on the skin, mucous membranes, and eyes. European Decision 2455/2001/EC has classified it as a persistent organic pollutant due to its high toxicity and propensity to accumulate in the environment64 and the Clean Water Act of the US Environment Protection Authority.65 Thus far, MIPs have been extensively employed in the development of highly selective sensors.66,67 The MIPs for 4-CP have recently been created,68,69 used for 4-CP solid-phase extraction on-line,70 and sampled for 4-CP in water.71 However, studies on the application of a 4-CP MIP in the creation of electro-chemical sensors are limited, as presented in Table 3.72,73
Table 3 Comparison of the corresponding efficiency levels of the modified electrodes based on MIPs for voltammetric 4-CP measurementa
Modified electrode |
Method detection |
LOD (μM) |
Linear range (μM) |
Ref. |
DPV, differential pulse voltammetry; SWV, square wave voltammetry. |
GCE/ZnO/GNPs/MIP |
DPV |
0.04 |
0.2–170 |
72 |
PDDA-G/MIP/GCE |
SWV |
0.3 |
0.8–100 |
73 |
Using a modified glassy carbon electrode (GCE), Al-Ammari et al. 72 considered an electrochemical sensor that could find 4-chlorophenol (4-CP). Graphene nanoplatelets (GNPs), zinc oxide nanoparticles, and a poly(o-phenylenediamine) polymer nanocomposite (GCE/ZnO/GNPs/MIP) supplemented with a graphene composite electrode (GCE) (Fig. 6). The nanocomposite improved the sensitivity and electrochemical responsiveness of the sensor for the detection of 4-CP. They used cyclic voltammetry and electrochemical impedance spectroscopy to check how the GCE/ZnO/GNP/MIP nanocomposite-based sensor worked in an electric field. They measured the 4-CP levels in real water samples using an electrochemical sensor that demonstrated good reproducibility, sensitivity, and selectivity. They proposed the GCE/ZnO/GNP/MIP sensor as a quick, easy, dependable, practical, and inexpensive technique for potential online 4-CP monitoring in the water.
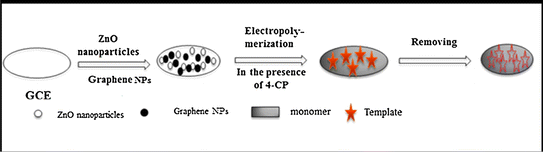 |
| Fig. 6 Fabrication of the GCE/ZnO/GNPs/MIP.72 | |
An electrochemical sensor for the selective detection of 4-chlorophenol (4-CP) was created by combining a molecularly imprinted polymer (MIP) of 4-chlorophenol (4-CP) with poly(diallyldimethylammonium chloride) (PDDA)-functionalized graphene (PDDA-G) (Fig. 7).73 The obtained MIP/PDDA-G/GCE demonstrated strong 4-CP sensing capabilities. Under optimal conditions, the sensor responded linearly to the 4-CP concentration throughout a wide range from 0.8 to 100 M, with a detection limit (3S/N) of 0.3 M. Furthermore, the imprinted sensor showed outstanding specific recognition for 4-CP, avoiding interference from other phenolic chemicals with similar structures. The sensor successfully identified 4-CP in real water samples.
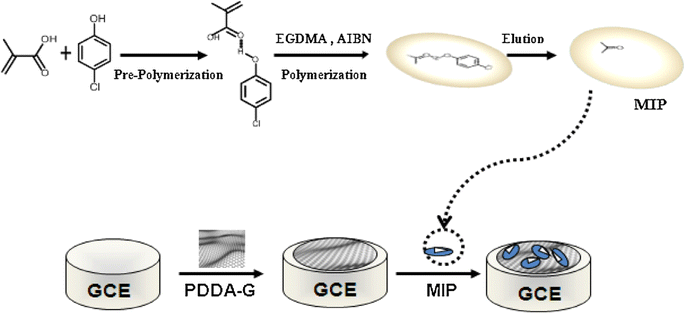 |
| Fig. 7 Schematic illustration of the fabrication of MIP/PDDA-G/GCE.73 | |
3.2. 2,4-Dichlorophenol
The organochlorine pesticide 2,4-DCP is used to make colors, antifungal agents, preservatives, and insecticides.74 Frequently found in the adipose tissue of aquatic life, these chemicals eventually make their way into the human body through bioaccumulation and biomagnification. When it accumulates, it becomes difficult to eliminate, and a mother may even transfer some of it to the fetus. We need to continuously evaluate these ubiquitously dangerous pollutants, known as polychlorinated aromatic compounds, to protect people and animals from exposure. In tissues, these compounds have a half-life ranging from five to thirty years. The US Environmental Protection Agency and the European Union have classified 2,4-DCP as a priority pollutant, with the maximum allowable amount in the water being 0.5 ng mL−1. This hazardous pollutant has negative effects on both humans and animals, even at nanogram levels. These effects include itching, skin irritation, anemia, and cancer.75 Table 4 presents a comparison of the analytical performance parameters for 2,4-DCP quantification using various reported modified electrodes based on MIPs.
Table 4 A review of MIP-based sensors for 2,4-DCP electrochemical detectiona
Modified electrode |
Method of detection |
LOD (μM) |
Linear range (μM) |
Ref. |
DPV, differential pulse voltammetry; SWV, square wave voltammetry; CFP, carbon fiber paper electrodes; PDA, polydopamine; CV, cyclic voltammetry; PRGO, Carica papaya reduced graphene oxide; DVB, divinylbenzene; AM, acrylamide; EGDMA, ethyleneglycoldimethacrylate. |
MIP/GO/GCE |
DPV |
0.5 × 10−3 |
0.004–10.0 |
75 |
MIP-PEDOT/CFP |
DVP |
0.07 × 10−3 |
0.21 × 10−3–0.3 |
76 |
MIP/PDA-rGO/GCE |
CV |
0.8 × 10−3 |
1.0 × 10−2–0.1 |
77 |
MIPs/Fe3O4/GCE |
SWV |
0.01 |
0.04 to 2.0 |
78 |
MIP/chitosan/Nafion/GCE |
Amperometry |
1.6 |
5.0–100 |
79 |
MIPs/PRGO microgel/GCE |
CV |
0.04 |
0.02 to 2.0 |
80 |
MIP/AM/DVB |
Potentiometry |
56.0 |
85.0–7.4 × 103 |
81 |
MIP/MAA/DVB |
59.0 |
85.0–7.4 × 103 |
MIP/EMA/EGDMA |
51.0 |
85.0–7.4 × 103 |
To detect the pesticide component 2,4-dichlorophenol (2,4-DCP), a selective electrochemical sensor was built using a molecularly imprinted conductive polymer.76 The 2,4-dichlorophenol-imprinted polymer films were produced by electropolymerizing 3,4-ethylenedioxythiophene (EDOT) on the surface of the carbon fiber paper electrodes (CFP) in the presence of 2,4-dichlorophenol. Electrochemical over-oxidation was employed to facilitate the controlled release of 2,4-DCP templates and to generate imprinting sites. The voltammetric sensor responded linearly ranging from 0.21 nM to 300 nM, with a detection limit of 0.07 nM (Fig. 8). The imprinted sensor was more selective and had a higher affinity for the target 2,4-DCP, even though it had the same amount of structural interference as the non-imprinted sensor. The MIP sensor effectively detected 2,4-DCP selectively in real water samples.
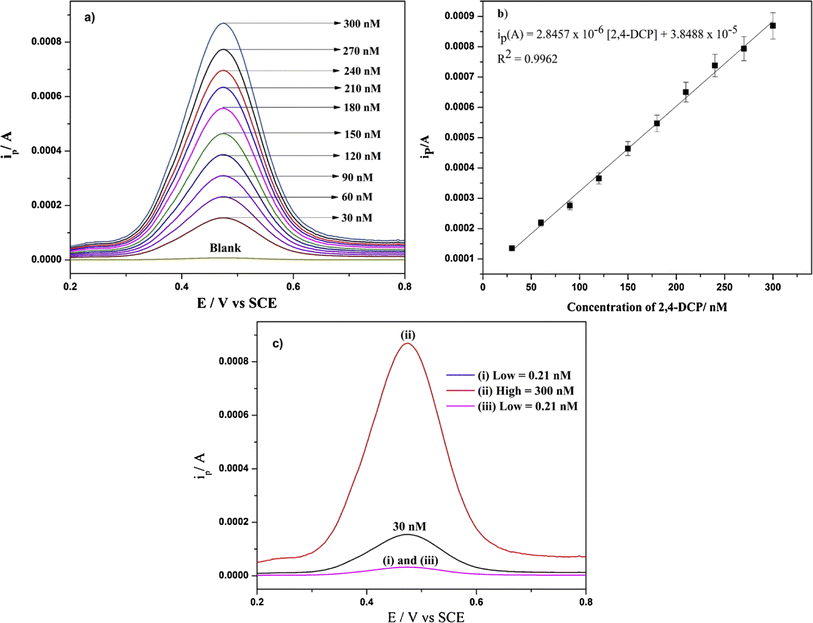 |
| Fig. 8 DPVs of 2,4-DCP at different concentrations ranging from 30 nM to 300 nM in the potential ranging from +0.2 V to +0.8 V vs. SCE in PBS (pH 9.0) (a), calibration plot for determining 2,4-DCP under the same experimental conditions (b) and DPVs of 2,4-DCP at low-high-low concentrations under similar experimental conditions (c). Each error bar represents the standard deviation for n = 3.76 | |
Liu et al. 77 developed a glassy carbon electrode modified with molecularly imprinted polymers (MIP) and polydopamine-reduced graphene oxide (PDA-rGO) as the basis for an electrochemical sensor for the detection of 2,4-DCP (Fig. 9). PDA-rGO was created when dopamine was auto-polymerized in an alkaline graphene oxide solution. Electropolymerizing the o-phenylenediamine (o-PD) monomer with the 2,4-dichlorophenol (2,4-DCP) template produced the MIP film on the surface of the PDA-rGO-modified electrode. The relative current intensity of ferro/ferricyanide, a signal transduction probe, decreased linearly as the 2,4-DCP concentration increased, with a detection limit of 0.8 nM (S/N = 3). The MIP sensor exhibited a notably greater affinity for 2,4-DCP in contrast to other analogs. The recommended method works well for measuring 2,4-DCP in real water samples.
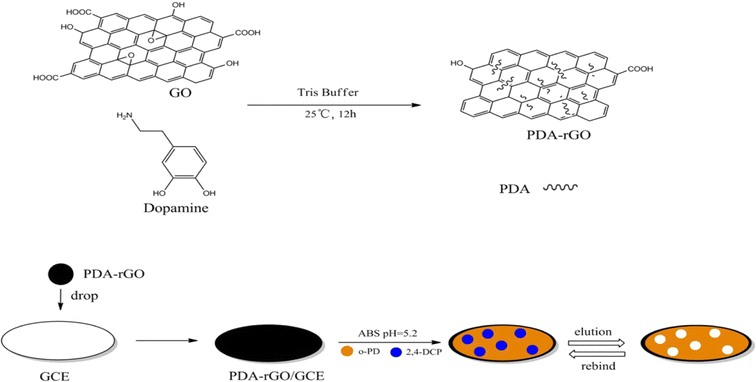 |
| Fig. 9 Synthesis procedure for molecularly imprinted polymer and the modified electrode construction process.77 | |
To produce a molecularly imprinted polymer-based electrochemical sensor, pyrrole was electropolymerized on a glassy carbon electrode modified with Fe3O4 nanoparticles (Fig. 10).78 The sensor showed a high level of catalytic activity in terms of 2,4-dichlorophenol oxidation (2,4-DCP). Square-wave voltage monitoring was used to measure the 2,4-DCP. At the 0.01 μM detection limit, the oxidation peak currents were proportionate to the 2.4% DCP concentrations, ranging from 0.04 to 2.0 μM. The amount of 2,4-DCP found in water samples was measured using the proposed sensor.
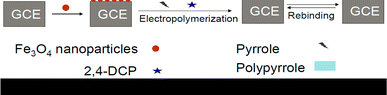 |
| Fig. 10 The process involves producing a modified glassy carbon electrode with molecularly imprinted polymers (MIPs, Fe3O4/GCE). | |
Liang et al. made an electrochemical sensor to test 2,4-dichlorophenol (2,4-DCP). It is based on a modified glassy carbon electrode (GCE) that has a molecularly imprinted polymer/graphene oxide (MIP/GO) on it (Fig. 11).75 To make MIP, azodiisobutyronitrile (AIBN) was used as an initiator, ethylene glycoldimethacrylate (EGDMA) was used as a cross-linking agent, methacrylic acid (MAA) was used as the functional monomer, and 2,4-DCP was used as a template. The MIP/GO/GCE demonstrated high recognition and electrochemical activity for 2,4-DCP. A straight-line link was found between the oxidation peak current and the amount of 2,4-DCP ranging from 0.004 M to 10.0 M, with a detection limit of 0.5 nM. The proposed sensor was successful in measuring 2,4-DCP in a real water sample.
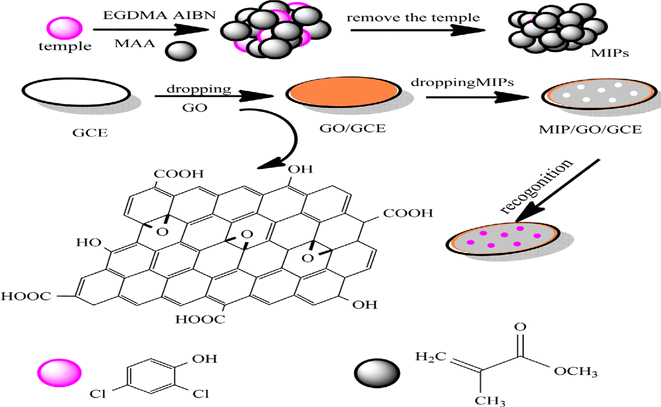 |
| Fig. 11 Construction of the improved electrode and synthesis of the molecularly imprinted polymer.75 | |
A unique molecularly imprinted polymer (MIP) was developed and used as a recognition element of an amperometric sensor to detect 2,4-dichlorophenol (2,4-DCP).79 An MIP with a clear structure could copy the natural enzyme chloroperoxidase's ability to dehalogenate 2,4-DCP. It was necessary to drop-coat the 2,4-DCP imprinted microgel solution and chitosan/Nafion mixture to build the imprinted sensor on a glassy carbon electrode surface (Fig. 12). Under optimal operating conditions, the sensor displayed a linear response in the range of 5.0–100 μM with a 1.6 μM detection limit. Additionally, compared to a sensor that was not imprinted, the one that was imprinted had a stronger affinity for the target 2,4-DCP than competing chlorophenolic compounds. It also demonstrated good stability and acceptable repeatability. With recoveries between 96.2% and 111.8%, the proposed sensor holds great promise for practical applications in quantifying 2,4-DCP in water samples.
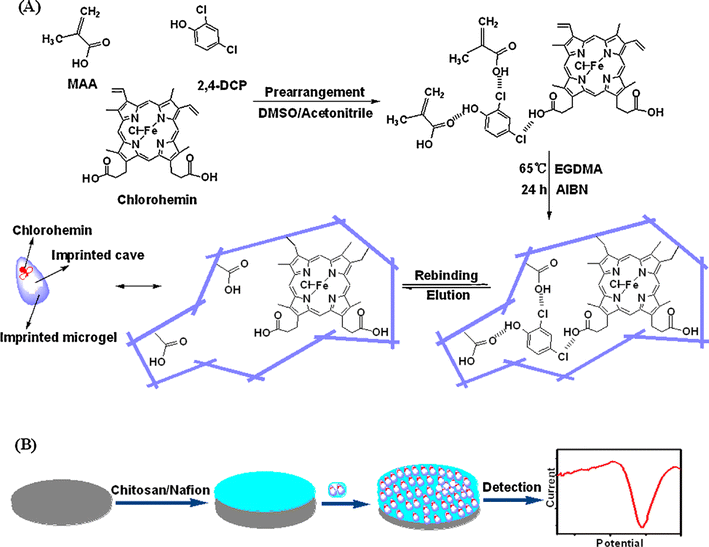 |
| Fig. 12 Diagram showing the synthesis pathway of the 2,4-DCP imprinted microgel (A) and the fabrication of the imprinted sensor (B).79 | |
It was possible to synthesize reduced graphene oxide (rGO) in an economical and environmentally beneficial manner by employing Carica papaya fruit extract as a reducing agent. The synthesized rGO and molecularly imprinted polymers (MIPs) were used to create a composite.80 Using Carica papaya reduced graphene oxide (PRGO) as the functional monomer and 2,4-DCP (2,4-dichlorophenol) as a template, a straightforward procedure was used to create this MIP microgel (Fig. 13). The cyclic voltammetry method was used to conduct electrochemical investigations. With a quantification limit of 0.04 μmol L−1, the modified electrode demonstrated a strong linear relationship for 2,4-DCP in concentrations ranging from 0.02 to 2.0 μmol L−1. It was discovered that this created sensor could detect 2,4-DCP in actual water samples taken from agricultural areas.
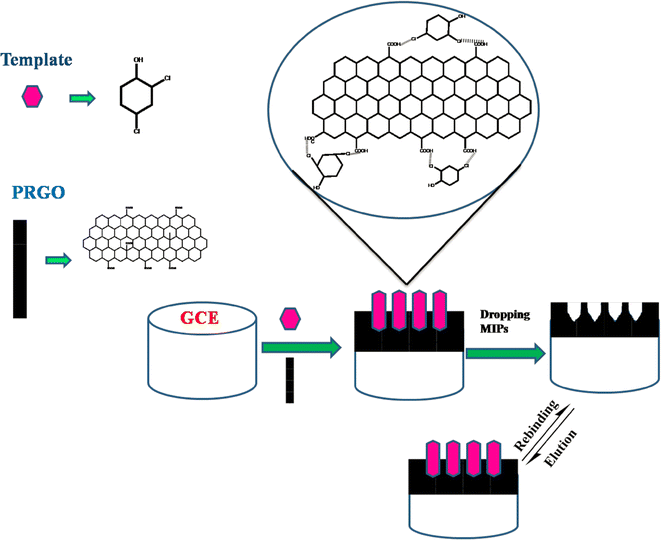 |
| Fig. 13 Schematic representation for the fabrication of the MIP/PRGO microgel/GCE modified surface.80 | |
Host-tailored polymers were created and used as recognition components in potentiometric transducers to specifically quantify 2,4-dichlorophenol (DCP).81 The polymer beads were made with acrylamide (AM), methacrylic acid (MAA), and ethyl methacrylate (EMA) as functional monomers; divinylbenzene (DVB) and ethylene glycol dimethacrylate (EGDMA) as cross-linkers; and DCP as a template molecule. The sensors were made by combining MIPs with a plasticized polyvinyl chloride (PVC) matrix. The response characteristics of the proposed MIP/AM/DVB, MIP/MAA/DVB, and MIP/EMA/EGDMA membrane-based sensors revealed anionic slopes of −59.2, −49.7, and −80.6 mV dec−1, with detection limits of 5.6 × 10−5, 5.9 × 10−5, and 13.2 × 10−5 M, respectively. The presented sensors revealed high potentiometric selectivity towards 2,4-DCP over many phenolic compounds (Fig. 14). The method worked well for routinely analyzing fish and fish farm water samples for food taint.
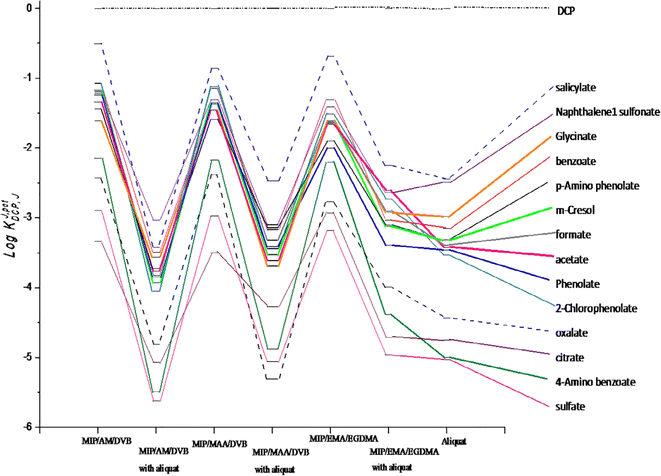 |
| Fig. 14 Potentiometric selectivity coefficients log KpotDCP, J of DCP membrane-based sensors.81 | |
3.3. 2,4,6-Trichlorophenol
The US EPA prohibits 2,4,6-trichlorophenol (TCP) because of its substantial carcinogenicity, probable toxicity, and stability. These elements have the potential to negatively impact living things and the environment over time.82,83 In the aquatic environment, it contains a diverse range of metabolites.84 Its detection is essential for assessing pollution because it may indicate the concentration of CPs in ambient air. The use of 2,4,6-trichlorophenol (TCP) to disinfect barrels is still practiced by wineries.85 TCP's slight acidity makes it easy for the skin to absorb. Current rules establish the maximum acceptable concentration (MAC) of 2,4,6-TCP in drinking water as 5.0 μg L−1 (10.1 nM). Table 5 presents a comparison of the analytical performance features for the quantification of 2,4,6-TCP using different reported modified electrodes based on MIPs.
Table 5 Comparing the modified electrodes based on the MIP for the electrochemical quantification of 2,4,6-TCPa
Modified electrode |
Method of detection |
LOD (μM) |
Linear range (μM) |
Ref. |
SPCE, screen printed carbon electrode; AuNPs, gold nanoparticles; PDA, polydopamine; GCE, glassy carbon electrode; CS, carbon sphere; LSV, linear sweep voltammetry. |
GC/MWCNTs/MIP |
Potentiometry |
0.5 |
8.0–100 |
85 |
SPCE/Gr/AuNPs/MIP |
SWV |
0.0067 |
0.02–1000 |
86 |
MIPs/AgNPs-PDA-GR/GCE |
DPV |
0.0007 |
0.002–0.01 0.01–0.1 |
87 |
CS@Ag@GO/GCE |
LSV |
0.0097 |
0.03–35 |
88 |
An electrochemical sensor with a conductive interface and MIP modification was created.86 The electrochemical sensor was modified by covalently linking layer-by-layer self-assembly with the imprinted polymer film. The addition of these two conductive functional materials raises the conductivity of the electrodes and supplies interface support materials, resulting in a high specific surface area (Fig. 15). Using 2,4,6-trichlorophenol as a model, we demonstrated that the created conductive sensor was significantly more sensitive than the conventional MIP sensor.
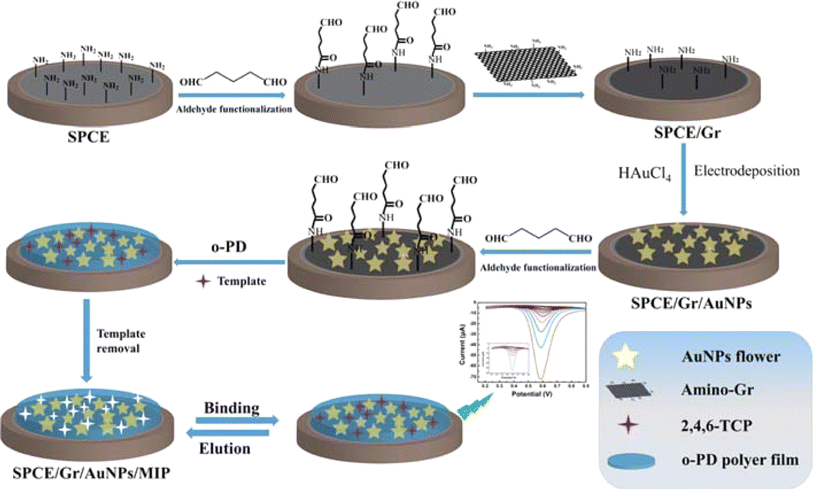 |
| Fig. 15 An illustration of the steps involved in fabricating the SPCE/Gr/AuNP/MIP electrode.86 | |
A molecularly imprinted electrochemical sensor for the high identification of 2,4,6-trichlorophenol was created by Wang et al. using molecularly imprinted polymers (MIPs).87 The sensitive and selective layer was an Ag nanoparticle-polydopamine-reduced graphene oxide-modified glassy carbon electrode (AgNP-PDA-GR/GCE). Next, we electro-polymerized o-phenylenediamine (o-PD) on the surface of AgNP-PDA-GR/GCE using 2,4,6-trichlorophenol as a template molecule (Fig. 16). Because AgNP-PDA-GR composites have a faster electron transfer rate, they can specifically recognize imprinted cavities, and PDA-graphene and 2,4,6-TCP have strong π–π stacking and hydrogen bonding, while MIPs/AgNPs-PDA-GR/GCE showed very selective and sensitive identification for 2,4,6-TCP. The imprinted sensor's electrochemical responses exhibit two dynamic linear relationships throughout the range of 2.0–10.0 nM and 10.0–100.0 nM, with a detection limit of 0.7 nM. Additionally, the 2,4,6-TCP concentration in water was measured using MIPs/AgNPs-PDA-GR/GCE, and the recovery ranged from 96.4 to 103.3%.
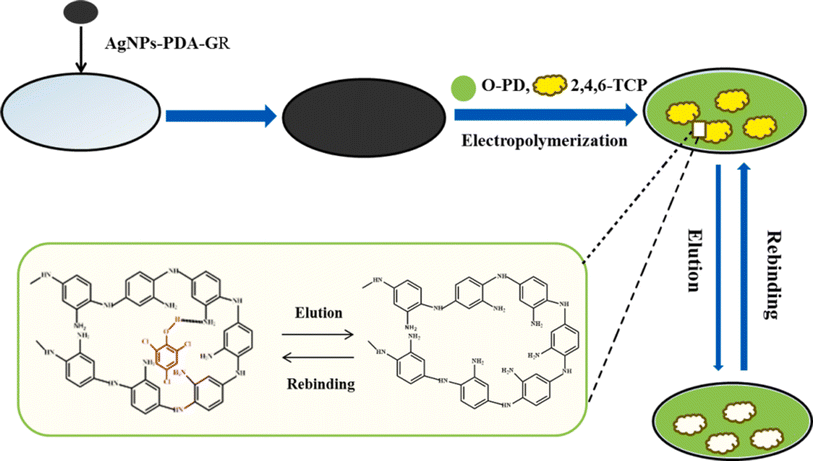 |
| Fig. 16 MIP/AgNP-PDA-GR/GCE fabrication.87 | |
A template-activated method was developed to produce a carbon sphere/silver composite with a core/shell structure based on a one-pot hydrothermal treatment.88 Graphene oxide (GO) nano-layers mechanically shielded the homogeneous, three-dimensional, interconnected microstructure of the CS@Ag, thereby increasing its surface area and catalytic activity. This one-of-a-kind structure made the CS@Ag more electrically conductive and effectively stopped AgNP from oxidizing and sticking together. The CS@Ag@GO, immobilized on the electrode surface, facilitated the sensitive determination of chlorinated phenols, such as 2-chlorophenol, 4-chlorophenol, 2,4-dichlorophenol, and 2,4,6-trichlorophenol (Fig. 17). CS@Ag@GO is a promising electrode material for practical phenol sensing applications due to its tailored structure, speed of electron transfer, and ease of manufacture.
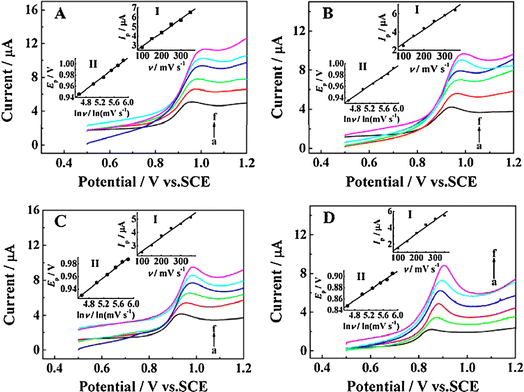 |
| Fig. 17 Linear sweep voltammograms of 0.5 mM 2-CP (A), 4-CP (B), 2,4-DCP (C) and 2,4,6-TCP (D) in pH 2.56 BR buffer acquired at CS@Ag@GO/GCE with different scan rates (ν) of 100 (a), 150 (b), 200 (c), 250 (d), 300 (e) and 350 (f) mV s−1. Insets: (I) peak current (Ip) versus ν. (II) Peak potential (Ep) versus ln ν.88 | |
To measure 2,4,6-trichlorophenol (2,4,6-TCP) potentiometrically,85 a solid-contact electrode (SCE) is made and talked about. Potentiometry uses trichlorophenol polymer (MIP) beads with molecular imprints as recognition receptors to quantify the amount of this persistent organic pollutant. The synthesis of MIPs uses 4-vinyl pyridine (4-VP) as a building block, while N,N′-methylene bis(acrylamide) (N,N-MBAA) links the molecules (Fig. 18). They are surrounded by a selective polyvinyl chloride (PVC) membrane on top of multi-walled carbon nanotubes (MWCNTs), which serve as an electron-moving route for ions. As shown in the table, it has an R2 value of 0.999, a detection limit of 5.0 × 10–7 M (0.98 μg mL−1), a Nernstian response that works between 8.0 × 10–6 M and 1.0 × 10–4 M, and a reaction time of less than 10 s. The proposed SCE has multiple applications for identifying TCP in various environmental samples.
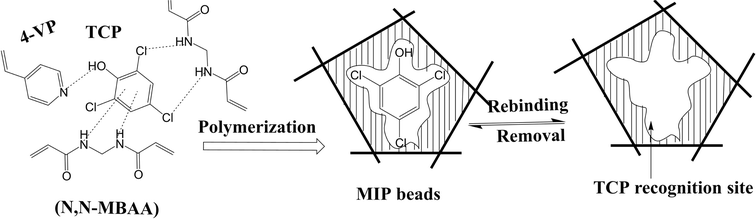 |
| Fig. 18 Schematic diagram of the imprinting process of TCP/MIP.85 | |
3.4. Pentachlorophenols
Pentachlorophenol, or PCP, has been a widely used pesticide for a long time. Nevertheless, because of its strong carcinogenic and mutagenic potential, PCP is categorized as a priority pollutant in the USA, EU, and China.89 Because of its extensive use, aquatic products eventually contain traces of it, and the environmental water system eventually becomes badly damaged. Consequently, numerous techniques have been described to identify and remove PCP residues from fish and water samples 90 and to break down the accumulated PCP in water samples.91 However, the use of a PCP MIP in the creation of electro-chemical sensors has not been the subject of many investigations.
Xia et al. developed a specific type of magnetic molecularly imprinted microsphere (MIM) for pentachlorophenol.92 An enzyme-labeled tracer was made simultaneously using strep avidinated horseradish peroxidase and pentachloroaniline (Fig. 19). Using a standard microplate, the two reagents were used to establish a semi-homogeneous approach for pentachlorophenol detection in fish samples. The use of biotinylated horseradish peroxidase as a signal amplification agent increased the procedure's sensitivity by 375 times when compared to the traditional method, which used a horseradish peroxidase-labeled tracer with a detection limit of 0.8 fg g−1. Comparatively speaking, this method outperformed the previous immunoassays and the molecularly imprinted polymer-based method for pentachlorophenol detection. This study introduced a novel semi-homogeneous approach for detecting small molecules on microspheres: magnetic molecular imprinting. This method has the potential to frequently determine the remaining amount of pentachlorophenol in fish samples.
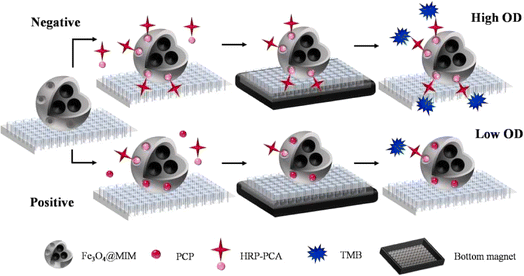 |
| Fig. 19 Fe3O4@MIM-based semi-homogeneous method's schematic depiction and the molecular structures of the chemicals employed in this study.92 | |
Pentachlorophenol (PCP) was detected using a visible light photo-electrochemical (PEC) sensing platform that was built.93 Using the molecular imprinting process in conjunction with a microfluidic paper-based analytical device (μ-PAD) and gold nanoparticles (AuNPs) functionalized with polypyrrole, the working electrode was a piece of paper decorated with AuNPs (Fig. 20). Ascorbic acid (AA) was employed as an efficient and non-toxic electron donor in a mild solution medium to scavenge photogenerated holes and encourage the development of a continuous photocurrent. The microfluidic molecularly imprinted polymer-based PEC analytical origami device aims to identify PCP linearly ranging from 0.01 ng mL−1 to 100 ng mL−1 while maintaining a low detection limit of 4 pg L−1. This disposable microfluidic PEC origami device enables a novel platform for sensitive, targeted, and multiplex analysis in public health, environmental monitoring, and the developing world.
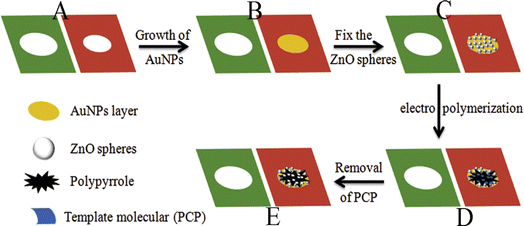 |
| Fig. 20 Paper-based PEC sensor's manufacturing process.93 | |
4. Mechanism of electrochemical reactions of chlorophenols
(i) Electrochemical oxidation can effectively treat 4-CP, 2,4-DCP, and 2,4,6-TCP aqueous wastes. In all cases, the electrochemical oxidation process fully converted the organic contaminants into carbon dioxide and volatile chlorinated organic molecules, specifically chloroform.
(ii) Hydroquinone, fumaric, maleic, and oxalic acids were the primary intermediates produced during the oxidation processes. Low concentrations of very tiny amounts of chlorinated aromatic intermediates, such as chlorohydroquinone and chlororesorcinol, as well as other carboxylic acids, such as formic and trichloroacetic, were also found. During the electrolytic process, it was discovered that CPs released chloride ions, which were oxidized to species with higher oxidation states.
(iii) The findings of this investigation are compatible with a basic mechanistic model (Fig. 21).94 This model says that when CP aqueous wastes are treated electrochemically, they first make non-chlorinated aromatic intermediates, and chlorine is released at both the anode and cathode ends of the process. The process produces C4, C2, and C1 dicarboxylic acids after aromatic ring cleavage. The anode transforms the chlorine from the CP HDH into a more oxidized species, which can then undergo a haloform reaction with unsaturated C4 carboxylic acid to produce trichloroacetic acid. In the end, the trichloroacetic acid oxidizes to carbon dioxide and volatile organo-chlorinated molecules, whereas the non-chlorinated organic acids oxidize to carbon dioxide.
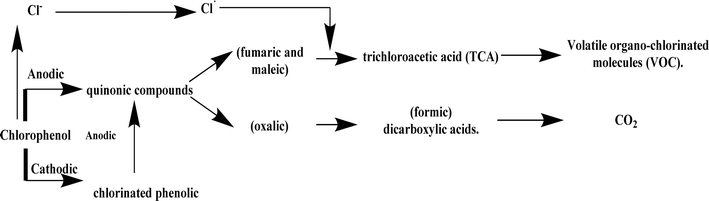 |
| Fig. 21 A diagram illustrating the basic mechanistic model suggested to describe the primary procedures involved in the electrochemical treatment of CP wastes. | |
(iv) The electrochemical treatment of CPs involves both mediated and direct electrochemical oxidation processes. Inorganic chemicals electrogenerated at the electrode surface, such as peroxodisulfates and hypochlorite, aid in global oxidation. A basic mechanistic model can be developed by identifying the intermediates in the galvanostatic electrolysis of CPs, including the compounds and their appearance timings, and combining this information with data from voltammetric studies (Fig. 21).
5. Conclusion and future outlook
Because PCPs are very common and harmful, they are among the most important pollutants to be monitored in the environment. This review discusses the pros and cons of using MIPs as synthetic selective receptors combined with electrochemical sensors to find and measure PCPs at low concentrations that are important for the environment. It is hard to imprint these parts because they do not have any functional groups, are not very plentiful, and are toxic. However, these problems can be solved with very creative imprinting methods, such as using dummy templates, sacrificial spacer synthesis, and adding silica, magnetic, carbon, and nanotubes as helper materials to improve electrochemical sensitivity. A very important step in the development of these hard MIP matrices is the effective use of advanced molecular modeling methods and spectroscopic tools for in-depth analysis. This helps in the creation of high-quality MIPs for these constituents and opens the door to the use of logical design strategies rather than trial-and-error experiments. The ability to find multiple PCPs at once using group-selective MIPs is emphasized. This eliminates the need to make multiple MIPs that are each specifically designed for a single molecule. It is clear from this review that dummy templates are frequently used in the synthesis of polymers that are selective for PCPs. This seems to be helpful for the larger-scale synthesis of these MIPs. Finally, because these materials can be reused, the commercialization of sensors and SPE cartridges based on these polymers would make the analysis of PCPs in environmental monitoring scenarios more affordable. Additionally, MIP-based sensors allow for on-site detection, which streamlines sample pretreatment.
Data availability
Data will be made available on request.
Author contributions
Ayman H. Kamel: conceptualization, supervision, data curation, formal analysis, visualization, methodology, data curation, validation, writing – original draft. Hisham S. M. Abd-Rabboh: methodology, data curation, funding acquisition, project administration, supervision, writing – review & editing, resource, and investigation. A. Hefnawy: methodology, data curation, formal analysis, visualization, writing – review & editing.
Conflicts of interest
The authors declare that they have no known competing financial interests or personal relationships that could have appeared to influence the work reported in this paper.
Acknowledgements
The authors extend their appreciation to the Deanship of Research and Graduate studies at King Khalid University for funding this work through Large Groups Project under grant number (RGP.2/288/45).
References
- M. Rastogi, S. M. Kolur, A. Burud, T. Sadineni, M. Sekhar, R. Kumar and A. Rajput, J. Geogr. Environ. Earth Sci. Int., 2024, 28, 41–53 CrossRef.
- A. Yadav and A. Dubey, Asian J. Pharm. Res. Dev., 2024, 12, 35–41 CrossRef.
- A. A. Hamad, M. Sharaf, M. A. Hamza, S. Selim, H. F. Hetta and W. El-Kazzaz, Microbiol. Spectrum, 2022, 10, e01516–e01521 CrossRef CAS PubMed.
- I. Qayoom, A. Abubakr, A. Gopinath, S. A. Dar and K. Khurshid, Aquatic pollution: Concerns and Apatement, CRC Press, 1st edn, 2025 Search PubMed.
- E. H. Khader, R. H. Khudhur, T. J. Mohammed, O. S. Mahdy, A. A. Sabri, A. S. Mahmood and T. M. Albayati, Desalin. Water Treat., 2024, 317, 100091 CrossRef.
- H.-S. Bae, T. Yamagishi and Y. Suwa, Microbiology, 2002, 148, 221–227 CrossRef CAS PubMed.
- C. Fan, N. Li and X. Cao, Food Chem., 2015, 174, 446–451 CrossRef CAS PubMed.
- P. M. Armenante, D. Kafkewitz, G. A. Lewandowski and C.-J. Jou, Water Res., 1999, 33, 681–692 CrossRef CAS.
- V. K. Gupta, P. J. M. Carrott, M. M. L. Ribeiro Carrott and Suhas, Crit. Rev. Environ. Sci. Technol., 2009, 39, 783–842 CrossRef.
- I. A. W. Tan, A. L. Ahmad and B. H. Hameed, J. Hazard. Mater., 2009, 164, 473–482 CrossRef CAS PubMed.
- M. Czaplicka, Sci. Total Environ., 2004, 322, 21–39 CrossRef CAS PubMed.
- R. Li, Y. Feng, G. Pan and L. Liu, Sensors, 2019, 19, 177 CrossRef PubMed.
- P. Nasr-Esfahani, A. A. Ensafi and B. Rezaei, Sens. Actuators, B, 2019, 296, 126682 CrossRef CAS.
- V. Rastogi, M. Porwal, A. Verma, U. Khan, P. Chauhan and P. Kumar, Int. J. Pharm. Invest., 2024, 14, 327 CrossRef.
- S. A. Younis, E. A. Motawea, Y. M. Moustafa, J. Lee and K.-H. Kim, J. Hazard. Mater., 2020, 397, 122792 CrossRef CAS PubMed.
- H. Cheng, Y. Zou, B. Lu, J. Wang, J. T. Magnuson, B. Xu, R. Xuan and W. Qiu, available at SSRN 4789103.
- A. G. Smith, in Neurotransmitters and Toxicology, ed. K. N. Woodward and T. Marrs, Royal Society of Chemistry, 2024, ch. 5, vol. 48, pp. 129–178 Search PubMed.
- Y. F. Mustafa, J. Mol. Struct., 2024, 1309, 138193 CrossRef CAS.
- S. Yadav, S. Kumar and A. K. Haritash, J. Environ. Manage., 2023, 342, 118254 CrossRef CAS PubMed.
- W.-J. Yang, H.-B. Wu, C. Zhang, Q. Zhong, M.-J. Hu, J.-L. He, G.-A. Li, Z.-Y. Zhu, J.-L. Zhu and H.-H. Zhao, Environ. Sci. Pollut. Res., 2021, 28, 61329–61343 CrossRef CAS PubMed.
- S. Rojas and P. Horcajada, Chem. Rev., 2020, 120, 8378–8415 CrossRef CAS PubMed.
- H. Rao, X. Zhao, X. Liu, J. Zhong, Z. Zhang, P. Zou, Y. Jiang, X. Wang and Y. Wang, Biosens. Bioelectron., 2018, 100, 341–347 CrossRef CAS PubMed.
- J. Miao, A. Liu, L. Wu, M. Yu, W. Wei and S. Liu, Anal. Chim. Acta, 2020, 1095, 82–92 CrossRef CAS PubMed.
- S. Liu, J. Pan, H. Zhu, G. Pan, F. Qiu, M. Meng, J. Yao and D. Yuan, Chem. Eng. J., 2016, 290, 220–231 CrossRef CAS.
- H. Pandey, P. Khare, S. Singh and S. P. Singh, Mater. Chem. Phys., 2020, 239, 121966 CrossRef CAS.
- N. S. Abdalla, M. A. Youssef, H. Algarni, N. S. Awwad and A. H. Kamel, Molecules, 2019, 24, 712 CrossRef CAS PubMed.
- S. Beyazit, B. T. S. Bui, K. Haupt and C. Gonzato, Prog. Polym. Sci., 2016, 62, 1–21 CrossRef CAS.
- K. Haupt and K. Mosbach, Chem. Rev., 2000, 100, 2495–2504 CrossRef CAS PubMed.
- K. Haupt, Anal. Chem., 2003, 75, 376A–383A CrossRef CAS PubMed.
- K. F. Pratama, M. E. R. Manik, D. Rahayu and A. N. Hasanah, Chem. Pharm. Bull., 2020, 68, 1013–1024 CrossRef CAS PubMed.
- G. Pan, S. Shinde, S. Y. Yeung, M. Jakštaitė, Q. Li, A. G. Wingren and B. Sellergren, Angew. Chem., Int. Ed., 2017, 56, 15959–15963 CrossRef CAS PubMed.
- W. Chen, Y. Ma, J. Pan, Z. Meng, G. Pan and B. Sellergren, Polymers, 2015, 7, 1689–1715 CrossRef CAS.
- Y. Yin, J. Pan, J. Cao, Y. Ma, G. Pan, R. Wu, X. Dai, M. Meng and Y. Yan, Chem. Eng. J., 2016, 286, 485–496 CrossRef CAS.
- N. Maouche, M. Guergouri, S. Gam-Derouich, M. Jouini, B. Nessark and M. M. Chehimi, J. Electroanal. Chem., 2012, 685, 21–27 CrossRef CAS.
- K. Kotova, M. Hussain, G. Mustafa and P. A. Lieberzeit, Sens. Actuators, B, 2013, 189, 199–202 CrossRef CAS.
- P.-Y. Chen, R. Vittal, P.-C. Nien, G.-S. Liou and K.-C. Ho, Talanta, 2010, 80, 1145–1151 CrossRef CAS PubMed.
- S. Farooq, J. Nie, Y. Cheng, Z. Yan, J. Li, S. A. S. Bacha, A. Mushtaq and H. Zhang, Analyst, 2018, 143, 3971–3989 RSC.
- M. Gao, Y. Gao, G. Chen, X. Huang, X. Xu, J. Lv, J. Wang, D. Xu and G. Liu, Sci. Total Environ., 2024, 927, 172309 CrossRef PubMed.
- A. Sarafraz-Yazdi and N. Razavi, TrAC, Trends Anal. Chem., 2015, 73, 81–90 CrossRef CAS.
- E. Turiel and A. Martín-Esteban, Anal. Chim. Acta, 2010, 668, 87–99 CrossRef CAS PubMed.
- H. Yan and K. Row, Int. J. Mol. Sci., 2006, 7, 155–178 CrossRef CAS.
- A. J. Kadhem, G. J. Gentile and M. M. Fidalgo de Cortalezzi, Molecules, 2021, 26, 6233 CrossRef CAS PubMed.
- M. Włoch and J. Datta, Compr. Anal. Chem., 2019, 86, 17 Search PubMed.
- K. Matyjaszewski, Eur. Polym. J., 2024, 211, 113001 CrossRef CAS.
- M. Frasco, L. Truta, M. Sales and F. Moreira, Sensors, 2017, 17, 523 CrossRef PubMed.
- Y. O. Yaman, N. Başaran, K. Karayagiz, Z. Vatansever, C. Yegin, Ö. Haluk Tekbaş and M. M. Sari, Adv. Mol. Imprinting Mater., 2016, 217–282 Search PubMed.
- G. Zhao, J. Liu, M. Liu, X. Han, Y. Peng, X. Tian, J. Liu and S. Zhang, Appl. Sci., 2020, 10, 2868 CrossRef CAS.
- A. A. Ensafi and P. Nasr-Esfahani, in Molecularly Imprinted Polymer Composites, Elsevier, 2021, pp. 5–20 Search PubMed.
- C. Malitesta, E. Mazzotta, R. A. Picca, A. Poma, I. Chianella and S. A. Piletsky, Anal. Bioanal. Chem., 2012, 402, 1827–1846 CrossRef CAS PubMed.
- D. J. Dyer, Surface-Initiated Polym. I, 2006, pp. 47–65 Search PubMed.
- A. Khlifi, S. Gam-Derouich, M. Jouini, R. Kalfat and M. M. Chehimi, Food Control, 2013, 31, 379–386 CrossRef CAS.
- A. Kidakova, J. Reut, R. Boroznjak, A. Öpik and V. Syritski, Proc. Est. Acad. Sci., 2019, 68, 158–167 CrossRef.
- C. Malitesta, I. Losito and P. G. Zambonin, Anal. Chem., 1999, 71, 1366–1370 CrossRef CAS PubMed.
- L. Özcan and Y. Şahin, Sens. Actuators, B, 2007, 127, 362–369 CrossRef.
- R. Wang, L. Wang, J. Yan, D. Luan, J. Wu and X. Bian, Talanta, 2021, 226, 122135 CrossRef CAS PubMed.
- Y. Ma, X.-L. Shen, Q. Zeng and L.-S. Wang, Microchim. Acta, 2017, 184, 4469–4476 CrossRef CAS.
- L. Chen, X. Wang, W. Lu, X. Wu and J. Li, Chem. Soc. Rev., 2016, 45, 2137–2211 RSC.
- D. Merli, E. Lio, S. Protti, R. Coccia, A. Profumo and G. Alberti, Anal. Chim. Acta, 2024, 1288, 342151 CrossRef CAS PubMed.
- T. L. Panasyuk, V. M. Mirsky, S. A. Piletsky and O. S. Wolfbeis, Anal. Chem., 1999, 71, 4609–4613 CrossRef CAS.
- T. Panasyuk-Delaney, V. M. Mirsky, M. Ulbricht and O. S. Wolfbeis, Anal. Chim. Acta, 2001, 435, 157–162 CrossRef CAS.
- T. Panasyuk-Delaney, V. M. Mirsky and O. S. Wolfbeis, Electroanalysis, 2002, 14, 221 CrossRef CAS.
- S. A. Piletsky, E. V Piletskaya, T. A. Sergeyeva, T. L. Panasyuk and A. V El'Skaya, Sens. Actuators, B, 1999, 60, 216–220 CrossRef CAS.
- Z. Dong, X. Le, C. Dong, W. Zhang, X. Li and J. Ma, Appl. Catal., B, 2015, 162, 372–380 CrossRef CAS.
- M. Abecassis-Wolfovich, M. V Landau, A. Brenner and M. Herskowitz, J. Catal., 2007, 247, 201–213 CrossRef CAS.
- L. Keith and W. Telliard, Environ. Sci. Technol., 1979, 13, 416–423 CrossRef.
- G. Yang, F. Zhao and B. Zeng, Biosens. Bioelectron., 2014, 53, 447–452 CrossRef CAS PubMed.
- Y. Wang, D. Zang, S. Ge, L. Ge, J. Yu and M. Yan, Electrochim. Acta, 2013, 107, 147–154 CrossRef CAS.
- T. Pasang and C. Ranganathaiah, Ind. Eng. Chem. Res., 2013, 52, 7445–7452 CrossRef CAS.
- T. Ye, S. Y. Lu, Q. Q. Hu, X. Jiang, G. F. Wei, J. J. Wang and J. Q. Lu, Chin. Chem. Lett., 2011, 22, 1253–1256 CAS.
- E. Caro, R. M. Marcé, P. A. G. Cormack, D. C. Sherrington and F. Borrull, J. Chromatogr. A, 2003, 995, 233–238 CrossRef CAS PubMed.
- J. Dong, H. Fan, D. Sui, L. Li and T. Sun, Anal. Chim. Acta, 2014, 822, 69–77 CrossRef CAS PubMed.
- R. H. Al-Ammari, A. A. Ganash and M. A. Salam, Synth. Met., 2019, 254, 141–152 CrossRef CAS.
- B. Wang, O. K. Okoth, K. Yan and J. Zhang, Sens. Actuators, B, 2016, 236, 294–303 CrossRef CAS.
- R. Jayaraj, P. Megha and P. Sreedev, Interdiscip. Toxicol., 2016, 9, 90–100 CrossRef CAS PubMed.
- Y. Liang, L. Yu, R. Yang, X. Li, L. Qu and J. Li, Sens. Actuators, B, 2017, 240, 1330–1335 CrossRef CAS.
- A. Maria C G, A. K B, S. Rison, A. Varghese and L. George, Synth. Met., 2020, 261, 116309 CrossRef.
- Y. Liu, Y. Liang, R. Yang, J. Li and L. Qu, Talanta, 2019, 195, 691–698 CrossRef CAS PubMed.
- B. Liu, H. Cang and J. Jin, Polymers, 2016, 8, 309 CrossRef PubMed.
- J. Zhang, J. Lei, H. Ju and C. Wang, Anal. Chim. Acta, 2013, 786, 16–21 CrossRef CAS PubMed.
- S. Ramanathan, E. Elanthamilan, A. Obadiah, A. Durairaj, P. Santhoshkumar, J. P. Merlin, S. Ramasundaram and S. Vasanthkumar, J. Mater. Sci.: Mater. Electron., 2019, 30, 7150–7162 CrossRef CAS.
- A. M. El-Kosasy, A. H. Kamel, L. A. Hussin, M. F. Ayad and N. V. Fares, Food Chem., 2018, 250, 188–196 CrossRef CAS PubMed.
- E. Ramos-Ramírez, F. Tzompantzi-Morales, N. Gutiérrez-Ortega, H. G. Mojica-Calvillo and J. Castillo-Rodríguez, Catalysts, 2019, 9, 454 CrossRef.
- X.-Q. Lin, Z.-L. Li, B. Liang, H.-L. Zhai, W.-W. Cai, J. Nan and A.-J. Wang, Water Res., 2019, 162, 236–245 CrossRef CAS PubMed.
- L. Ji, S. Ji, C. Wang and K. P. Kepp, Environ. Sci. Technol., 2018, 52, 4422–4431 CrossRef CAS PubMed.
- H. H. Shanaah, Z. Ameen, K. Jaafar, A. Hefnawy, H. S. M. Abd-Rabboh and A. H. Kamel, ChemElectroChem, 2023, 10, 202300153 CrossRef.
- Z. Xu, X. Jin, Y. Li, M. Zhang, W. Yin, Y. Yang, W. Jia and D. Xie, RSC Adv., 2024, 14, 3834–3840 RSC.
- L. Wang, Y. Liu, R. Yang, J. Li and L. Qu, Microchem. J., 2020, 159, 105567 CrossRef CAS.
- T. Gan, Z. Lv, J. Sun, Z. Shi and Y. Liu, J. Hazard. Mater., 2016, 302, 188–197 CrossRef CAS PubMed.
- Y. Yang, Y. Xiao, M. Li, F. Ji, C. Hu and Y. Cui, PLoS One, 2017, 12, e0170092 CrossRef PubMed.
- F. Khan, D. Prakash and R. K. Jain, BMC Chem. Biol., 2011, 11, 1–6 CrossRef PubMed.
- J. W. Liu, R. Han, H. T. Wang, Y. Zhao, W. J. Lu, H. Y. Wu, T. F. Yu and Y. X. Zhang, J. Mol. Catal. A: Chem., 2011, 344, 145–152 CrossRef CAS.
- W. Qiu Xia, S. Han Wang, Y. Liang Wu and J. Ping Wang, Microchem. J., 2023, 189, 108512 CrossRef.
- G. Sun, P. Wang, S. Ge, L. Ge, J. Yu and M. Yan, Biosens. Bioelectron., 2014, 56, 97–103 CrossRef CAS PubMed.
- P. Canizares, J. Garcia-Gomez, C. Saez and M. A. Rodrigo, J. Appl. Electrochem., 2003, 33, 917–927 CrossRef CAS.
|
This journal is © The Royal Society of Chemistry 2024 |