DOI:
10.1039/D0BM00055H
(Review Article)
Biomater. Sci., 2020,
8, 2084-2101
Rational design and latest advances of polysaccharide-based hydrogels for wound healing
Received
9th January 2020
, Accepted 13th February 2020
First published on 13th February 2020
Abstract
Acute and chronic wounds cause severe physical trauma to patients and also bring an immense socio-economic burden. Hydrogels are considered to be effective wound dressings. Polysaccharides possessing distinctive properties such as biocompatibility, biodegradability, and nontoxicity are promising candidates to structure hydrogels for wound healing. Polysaccharide-based hydrogels can provide suitable moisture for the wound and act as a shield against bacteria. Adequate mechanical properties, degradability, and therapeutic agent controlled release of polysaccharide-based hydrogels have been already characterized for effective utilization. This review presented several crucial design considerations about hydrogels for wound healing, and the current state of polysaccharide (chitosan, alginate, hyaluronic acid, cellulose, dextran, and starch)-based hydrogels as wound dressings was also summarized. The commonly used crosslinking techniques, including physical, chemical, and enzymatic crosslinking, are discussed in detail. Finally, we outline the challenges and perspectives about the improvement of polysaccharide-based hydrogels.
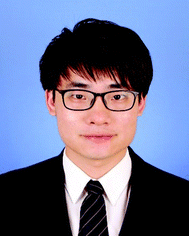 Hao Hu | Hao Hu received his PhD from the Beijing University of Chemical Technology in 2016 under the supervision of Prof. Fu-Jian Xu. In 2016, he joined the College of Materials Science and Engineering at Qingdao University as a Lecturer. His research focuses on developing smart gene/drug delivery systems for cancer therapy and smart hydrogels for wound healing. |
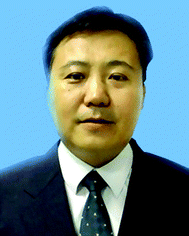 Fu-Jian Xu | Fu-Jian Xu is the Executive Director of the Beijing Laboratory of Biomedical Materials, Beijing University of Chemical Technology. His research interests focus on functional biomacromolecules. He was the recipient of the National Science Foundation for Distinguished Young Scholars (NSFC, 2013), Cheung Kong Distinguished Professor (Ministry of Education of China, 2014) and Beijing Outstanding Young Scientist Program (2018). |
1. Introduction
Timely and effective wound treatment in the wound care department is of great significance, not only for acute bleeding caused by accidents or wars but also for chronic wounds such as diabetic foot ulcers, venous leg ulcers, and pressure ulcers.1,2 The human body undergoes a coagulation process which can transform blood into stable and insoluble fibrin to accomplish hemostasis. However, in some cases such as in operating rooms or emergencies, it is not enough to rely solely on the body's biological process of hemostasis. Hydrogels are considered to be effective wound dressings for wound treatment.3–5 A microclimate could be formed between the wound bed and hydrogel which can provide a biocompatible platform, adequate moisture, and ability to isolate bacteria.6–8 Compared with traditional hemostatic materials (e.g., gauze, bandage), one notable advantage of hydrogels is that they can be easily removed from the wound due to their limited adhesion. This will avoid secondary pain for the injured person and further trauma to the healing tissue.9
Hydrogels have been the material of choice for biomedical applications because of their desirable biocompatibility, variable structures, flexible methods of synthesis, and desirable physical characteristics.10 Hydrogels possess three dimensional (3D) crosslinked porous networks and may absorb up to thousands of times their dry weight in water.11 Hydrogels can swell in fluids due to thermodynamic force but do not dissolve.12–14 The molecular chains forming hydrogels are linked by chemical or physical crosslinkers. There are different classifications of hydrogels. For example, depending on different binding modes, hydrogels can be classified as physical and chemical hydrogels. Physical hydrogels are formed by physical actions (ionic interactions,15 hydrogen bonds,16 entanglement of chains,17etc.). In general, physical hydrogels are characterized as reversible when being heated or treated with other stimulations.18–20 In contrast, chemical hydrogels are usually stable due to covalently crosslinked networks.21–23 According to the source of materials, hydrogels could be divided into synthetic hydrogels and natural ones. Synthetic hydrogels can be roughly classified into five categories according to their chemical composition: poly(hydroxyalkyl methacrylates), poly(acrylamide), poly(N-vinyl pyrrolidone), poly(acrylic acid), and poly(vinyl alcohol).12,24 The most studied natural hydrogels are polysaccharide- and peptide-based hydrogels.25–27 Polysaccharides have abundant sources and are readily available from renewable sources (marine organisms and plants).28,29 The production process of polysaccharides is convenient, low cost and feasible for large-scale production.30 Moreover, the unique physical and chemical properties of polysaccharides such as biocompatibility, biodegradability and no immune responses are the premise of their wide applications in biological materials.31–34
In recent years, polysaccharides have been recognized as excellent candidates for various biomedical applications such as drug delivery, gene delivery, and wound dressing.35–38 A series of controllable polymer-grafted polysaccharides were designed and prepared by living radical polymerization (LRP), ring-opening polymerization (ROP) and self-assembly (Fig. 1).32,39–41 The modified polysaccharides (aminated, PEGylated or zwitterionic dextran, hyaluronic acid or heparin; supramolecular assemblies which consist of β-cyclodextrin/adamantane) show excellent therapeutic effects for cancer therapy or wound healing applications.42–45 The abundant pendant functional groups in the structural unit of polysaccharides provide available reaction sites to form hydrogels, no matter whether by physical or chemical crosslinking. In addition to the unique properties of polysaccharides themselves, polysaccharide-based hydrogels also possess distinctive abilities such as high water retention capacity. Because of the tunable structure and network morphology, polysaccharide-based hydrogels are good for use in the area of controlling diffusion, wound healing, and biosensors. Fig. 2 shows the property overview of polysaccharide-based hydrogels for wound healing. Although some hydrogels for wound management have been reviewed,30,46 these reviews mainly focus on the properties and applications of hydrogels. Few reviews on the rational design of polysaccharide-based hydrogels have been reported to date. The properties required for hydrogels are complementary. Any weak aspect will affect the healing process. Therefore, it is important to prepare hydrogels with appropriate properties for wound healing by rational design.
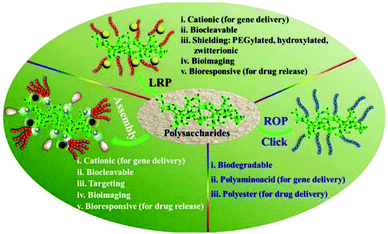 |
| Fig. 1 Versatile functionalization of polysaccharides via polymer grafts. Reprinted with permission from ref. 32. Copyright 2017 American Chemical Society. | |
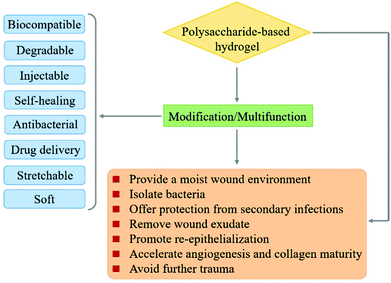 |
| Fig. 2 Property overview of polysaccharide-based hydrogels for wound healing. | |
In this review, we discuss the rational design of polysaccharide-based hydrogels as ideal wound healing materials. The advantages and drawbacks of the commonly used crosslinking techniques as well as the scope of their application are discussed in detail. We also highlight representative research studies about polysaccharide-based hydrogels used for wound healing in recent years.
2. Design considerations and required properties of polysaccharide-based hydrogels for wound healing
Polysaccharides consist of monosaccharides and their derivatives, including D-glucose, D-galactose, D-fructose, D-mannose, D-xylose, L-galactose, and L-arabinose, which are combined by glycosidic bonds.34 The polysaccharides composed of a single monosaccharide are defined as homopolysaccharides, such as starch, cellulose, and glycogen.47 The polysaccharides composed of different monosaccharides are called heteropolysaccharides such as hyaluronic acid, chondroitin, and alginate.48–50 The length and branching degree of molecular chains are also different according to various polysaccharides. For instance, starch can be divided into linear amylose and branched amylopectin, depending on the branching degree.46 The structures of commonly used polysaccharides in the preparation of hydrogels are shown in Fig. 3. The polysaccharide-based hydrogels are claimed as being effective candidates for wound treatment with high water-retaining capacity, renewability, biodegradability, biocompatibility, and nontoxicity.
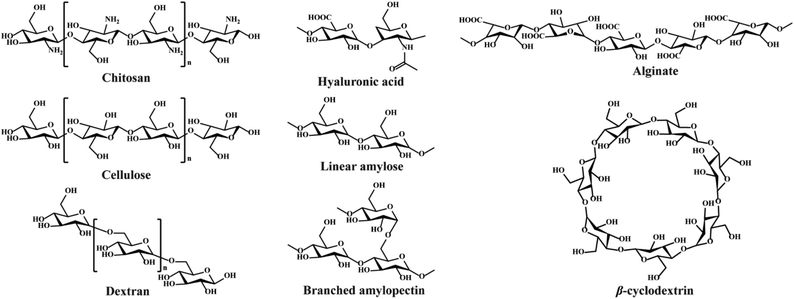 |
| Fig. 3 Structures of representative polysaccharides. | |
2.1. Wound healing process
For an ideal hemostatic material, the most important aspect is to hold blood rapidly and have no negative influence on wound healing. If hydrogels possess excellent biocompatibility, biodegradability, and stretchability, their applications will be further expanded, especially for chronic wound treatments.51 The wound healing process consists of four typical stages: exudative, inflammatory, proliferative and regenerative, as shown in Fig. 4.18,30 These cellular and biochemical cascades occur in a sequential but overlapping manner.52 During the exudative stage, or called hemostasis, blood vessels contract, and platelets aggregate and form blood clots at the wound site.53,54 The act of bleeding can wash the damaged tissue and protect the wound from microbial invasion. To prevent excessive bleeding, blood vessels contract and platelets aggregate and release clotting factors to form blood clots.55,56 Once the exudative stage is complete, the inflammatory stage follows. Neutrophils migrate to the wound site and release cytokines to activate the phagocytic activity of macrophages. The combined activity of neutrophils and macrophages will cause inflammatory responses such as heat and redness, and meanwhile, help to clear damaged and/or necrotic tissues, particulate contaminants, and microorganisms from the wound site.57–59 Later in this stage, neutrophils are eliminated from the wound site by apoptosis and phagocytosis (by macrophages). Modified monocyte macrophages start to remove apoptotic cells58,59 and stimulate angiogenesis and granulation tissue formation.60 The proliferation stage is a stage of tissue formation. The granulation tissue composed of collagen (type III) and extracellular matrices is used to fill the wound and epithelialization takes place around the wound edges.61,62 Moreover, in order to supply enough oxygen and nutrients for the active proliferating process, new blood capillaries are formed during this stage.59,63 Once the wound has closed, the regeneration process initiates. Collagen type III fibers are replaced by stronger type I fibers to increase wound strength, and a normal dermal architecture is restored.52,64 One more issue that needs to be considered during wound healing is skin and soft tissue infections (SSTIs).65 The normal wound healing process would be compromised if the immune system is not able to remove the pathogen, when infection occurs and causes the deterioration of the granulation tissue, growth factors and extracellular matrix components (collagen, elastin, and fibrin).66
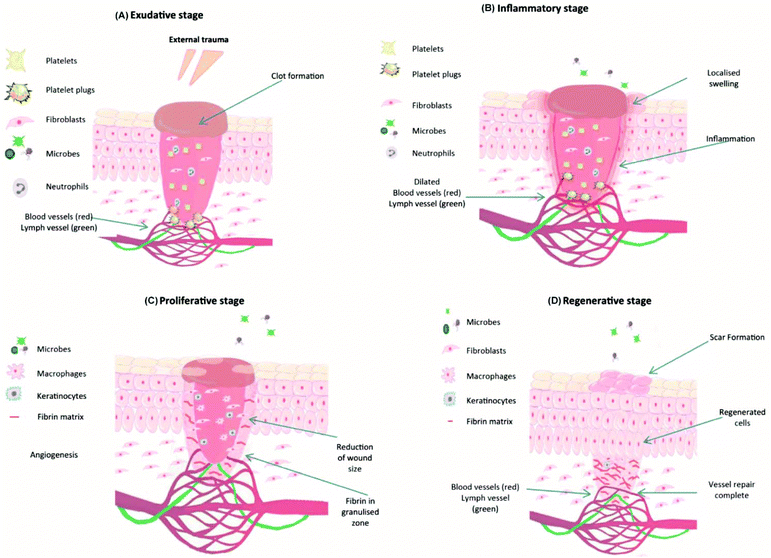 |
| Fig. 4 Typical four stages of wound healing: (A) exudative, (B) inflammatory, (C) proliferation, and (D) regenerative stages. Reprinted with permission from ref. 30. Copyright 2018 Elsevier Ltd. | |
As wound dressings, the hydrogel can prevent microbial invasion into the wound site because of its barrier action between the wound bed and external environment. Meanwhile, antimicrobial agents and wound healing drugs can be loaded into the hydrogels by being physically encapsulated or covalently crosslinked to avoid the risk of bacterial contamination and infection, and thereby improving the healing process.67,68
2.2. Design considerations
2.2.1. Biocompatibility.
Biocompatibility is usually the first consideration, as long as the materials are for biomedical applications. The hydrogels should exhibit no or limited deleterious immunological, toxic, or foreign body responses since the gels touch tissues and cells directly for wound healing. If this requirement cannot be achieved, the safety of wound treatment can be threatened, and the wound may become infected, difficult to heal, or scarred.69 Polysaccharides are generally regarded as having superior biocompatibility. However, it is important to note that the initiators, organic solvents, stabilizers, emulsifiers, unreacted monomers and crosslinkers used in the hydrogel preparation process may be hazardous to host cells or the whole system. For example, glutaraldehyde, which is a small molecule crosslinker, has been extensively used to crosslink various types of biopolymeric tissue scaffolds, hydrogels, and composites.70 The aldehyde groups of glutaraldehyde are toxic for cells and would cause severe inflammation in the body. Therefore, hazardous chemicals should be removed thoroughly, which was performed typically by solvent washing or dialysis.70,71 Utilizing the technique of in situ gelation, ideal for its minimal invasiveness, requires special caution to ensure that all components are safe and reasonably nontoxic. This indicates one highlight of physical crosslinking. No toxic chemical crosslinking agents would be introduced into hydrogels during the preparation process.
2.2.2. Degradation.
In some cases, the degradation properties of hydrogels need to be considered. The wound dressings for the wounds on the skin surface do not require degradability necessarily. However, for the chronic wound, visceral bleeding, and deep fissured wound, biodegradability is indispensable.72 The degradable hydrogel would progressively eliminate its function and be replaced by new tissue as degradation progresses. This strategy of avoiding the removal process after hemostasis is friendly to patients. Polysaccharides can be degraded gradually in vivo, but at different rates.73 Therefore, the degradation time can be controlled by altering the component ratios of each component.74 Cleavable groups or bonds can be introduced into the polymer backbone to endow the hydrogel with degradability. These groups or bonds can be cleaved via hydrolysis or external stimulation (thermal-stimulus, pH-stimulus, enzymatic digestion, etc.) to break down the hydrogel.75,76
2.2.3. Strength, stretchability, and adhesion.
Depending on the type and site of the wound, the mechanical properties of hydrogels need to be emphasized. To treat the wound of skin trauma, especially at joints, like ankle, knee, and wrist, the traditional dressing materials are tied around the extremities, which makes the patients feel discomfort and is inconvenient. In addition, considering the frequent motion and bending of extremities, the stable adhesion between dressing materials and the wound site would enhance their reliability and availability. The mechanical properties of hydrogels can be controlled by adjusting the crosslinking index or crosslinking manner. To achieve the balanced mechanical properties between stiffness and toughness, double-network (DN) hydrogels were prepared. DN hydrogels were composed of two interpenetrating networks, wherein the first (brittle and rigid) network easily ruptures to dissipate energy, and the second (soft and ductile) network contributes to the stretchability of hydrogels.77 Wu and coworkers fabricated a series of DN hydrogels with superior elastic modulus, high tensile strength, and ultrahigh fracture energy by hybrid noncovalent–covalent crosslinking.17,37 Hydrogel adhesion properties need to be viewed dialectically. We can use hydrogels that act like glue instead of sutures to seal the wound. This requires the hydrogels to have good adhesive properties to bind the two parts of the tissue. Lu and coworkers prepared a plant catechol-chemistry-based hydrogel with high adhesiveness, toughness, and cell affinity.78 The continuous generation of catechol groups endows the hydrogel with long-term and repeatable adhesiveness. However, for skin trauma such as bruises, hydrogels are required to have limited adhesive properties and can be removed easily after healing. For example, Guo and coworkers prepared a series of antibacterial adhesive injectable hydrogels by mixing quaternized chitosan (QCS) and benzaldehyde-terminated Pluronic F127 (PF127-CHO), which exhibited suitable stretchable and compressive properties and efficient hemostatic performance (Fig. 5).7 The tensile moduli of the prepared hydrogel dressings matched the moduli of the underlying and neighbouring tissues, which made the hydrogel adhere to skin stably.79
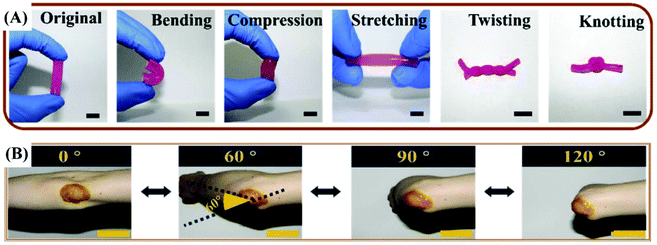 |
| Fig. 5 (A) The original, bending, compression, stretching, twisting and knotting shapes of the quaternized chitosan (QCS)/Pluronic F127 (PF) hydrogel. (B) Photograph of the curcumin loaded QCS/PF (Cur-QCS/PF) hydrogels that were applied on the human elbow. Reprinted with permission from ref. 7. Copyright 2018 Elsevier Ltd. | |
2.2.4. Injectable and self-healing properties.
An injectable hydrogel, which can be injected using an injector system, is a kind of low viscosity fluid before the injection. After injection, the sol state transfers to the gel state in situ by crosslinking. The most obvious advantage of injectable hydrogels is that they are suitable for filling irregular wounds with minimal invasiveness and pain.80 Also, therapeutic drugs or cells can be loaded into the hydrogel in the sol state, and then delivered into the body for controlled release or to repair the wound. Given that the injectable hydrogel usually would not be removed from the body after the treatment, the injectable hydrogel needs to be biodegradable and the degradable segments should be biocompatible. Self-healing ability could prolong the service time of hydrogels via repairing the deformation or damage automatically, especially for the hydrogels with weak elasticity, such as most injectable hydrogels.81,82
2.2.5. Therapeutic agent encapsulation.
Different kinds of therapeutic agents were encapsulated into hydrogels to aid the wound healing process, especially for chronic wounds.83–86 Diabetic wound is a significant cause of diabetes-related amputations. Mechanisms underlying the poor healing of diabetic wounds may involve hypoxia, impaired angiogenesis, damage from reactive oxygen species (ROS), and neuropathy.2 Stem cells and secretome have been proven to positively impact on wound healing by direct and indirect actions including residing cell stimulation, biomolecule release, inflammation control and extracellular matrix remodelling.87 Mao and coworkers prepared a multifunctional hydrogel, which was composed of Pluronic F127, oxidative hyaluronic acid, and poly-ε-L-lysine, to deliver adipose-derived mesenchymal stem cell exosomes for synergistically enhancing chronic wound healing and complete skin regeneration. The diabetic full-thickness cutaneous wounds exhibited enhanced wound closure rates, fast angiogenesis, re-epithelialization and collagen deposition within the wound site after being treated with the exosome-loaded hydrogel.88 In order to release the cargo in a controlled manner, the rate of degradation/breakdown of hydrogels should be rationally designed. Poor biodegradability may cause the drug to be released too slowly and extend the detention time of gels in the body.
2.2.6. Antibacterial activity.
Excessive inflammation may lead to a systemic infection. It is easy for bacteria to settle and breed on the infected wounds.8 Although hydrogels can be used as a barrier to avoid microorganism proliferation and invasion, the antibacterial ability would be further improved by incorporating antibacterial agents into the dressings or directly using materials with inherent antibacterial activities.89,90 In the work reported by Guo et al., a series of antibacterial hydrogels based on quaternized chitosan were developed.90 Jayakumar et al. prepared a tigecycline loaded chitosan hydrogel for the treatment of S. aureus infected wounds. This hydrogel was an effective medium for antibiotic delivery and prevented skin infections effectively.91
The properties of hydrogels discussed above or not mentioned are interrelated. For example, the environmental sensitivity (pH-sensitive, temperature-sensitive, etc.) would affect the degradation rate which in turn may affect the controlled release and mechanical properties. Therefore, all aspects need to be fully considered when designing hydrogels, though it is highly challenging to obtain an all-in-one hydrogel system as a wound dressing. One more thing is that the risk of batch-to-batch variation may occur because the raw materials as polysaccharides are mostly from natural sources.92 Thus, a permanent supplier of raw materials is suggested to maintain the stability of raw materials.
3. Preparation of polysaccharide-based hydrogels
The crosslinking techniques of hydrogels can be categorized into physical and chemical crosslinking methods. Chemical crosslinking is achieved by forming strong chemical bonds. These strong bonds could enhance the mechanical properties of hydrogels, but have negative effects on the degradability and biocompatibility.93 Physical crosslinking is not as strong as chemical crosslinking. However, the weak interactions (e.g., hydrogen bonds, ionic interactions) endow the hydrogels with self-healing and injectable properties. The weak interactions are usually reversible and could act as sacrificial portions when subjected to external forces. These sacrificial bonds could bear the stress or stretch at small deformation and preferentially rupture with original networks sustained, resulting in an efficient energy dissipation mechanism and improved mechanical performance.94 Besides, good biocompatibility is a great strength of physical crosslinking. The advantages and disadvantages of these methods are shown in Table 1.
Table 1 Advantages and disadvantages of physical, chemical, and enzymatic crosslinking methods. Reprinted with permission from ref. 93. Copyright 2017 Elsevier B.V
Crosslinking method |
Advantages |
Disadvantages |
Physical |
1. Safe |
1. Bonds are far weaker than the chemical crosslinkers |
2. Less toxic for cells than chemical agents |
2. May alter the properties of the materials |
3. Inexpensive |
3. Needs more time for crosslinking |
4. Minimum tissue reaction after crosslinking process |
4. Lack of control over the reaction kinetics of crosslinking |
|
5. Lower degrees of crosslinking |
|
Chemical |
1. Forming very strong bonds |
1. Almost toxic for the cells |
|
2. Needs washing to remove the residual cross-linker |
|
3. More expensive than physical crosslinker |
|
Enzymatic |
1. Unlike many chemical agents, enzymes are most active under mild aqueous reaction conditions |
1. The most expensive crosslinkers |
2. Crosslinking process can often be controlled by modifying temperature, pH, or ionic strength |
2. Substrate specificity |
In addition to the hydroxyl groups of polysaccharide chains, other functional groups, such as amide, amino, carboxyl, and hydroxyl, also provide versatile reaction sites for physical and chemical crosslinkings. The different types of crosslinkages and crosslinking methods would determine the physicochemical properties of hydrogels.
3.1. Physical crosslinking
Physically crosslinked 3D networks by physical interactions such as hydrogen bonding, ionic interactions, crystallization, entanglement of chains, and hydrophobic interactions can be achieved by heating or cooling of polymer solutions, freeze-thawing, lowering pH, and selection of anionic and cationic polymers.95,96 The significant advantage of physical crosslinking techniques is that no additional crosslinkers are required in the preparation of hydrogels. This avoids the potential toxicity of chemical crosslinking agents. The semipermanent crosslinked points would stabilize the 3D network and avoid the dissolution of the hydrogel. However, physically crosslinked hydrogels are not always ideal in terms of mechanical strength. Fig. 6 shows the typical interactions of physical crosslinking.
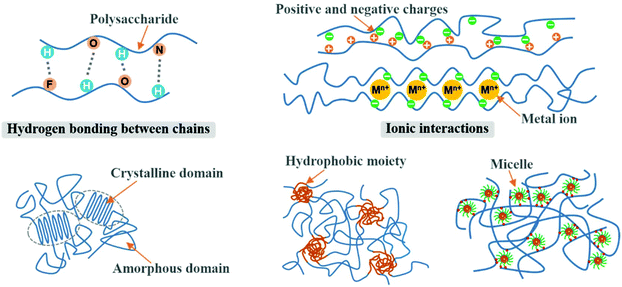 |
| Fig. 6 Typical interactions of physical crosslinkings. | |
3.1.1. Hydrogen bonding between chains.
Hydrogen bonding plays a crucial role in the fabrication of polysaccharide-based hydrogels. Plenty of hydrogen donors and acceptors (e.g., –OH, –COOH, –NH2) in polysaccharides provide the conditions for the formation of hydrogen bonds. The hydrogen bonding is a weak interaction. Dynamic destruction and reconstruction of hydrogen bonds occur in response to the change in temperature, pH, or solvent. The hydrogels crosslinked by hydrogen bonding alone usually cannot meet the mechanical strength requirements for wound healing. Therefore, it is a good choice to combine with other types of crosslinking to enhance the properties of hydrogels. In the work reported by Han et al., the hydrogels reinforced with cellulose nanocrystals were fabricated from xanthan solutions and chitosan through electrostatic and hydrogen bonding interactions.97 A kind of polysaccharide-templated hydrogel, composed of oxidized sodium alginate (OSA) and poly(acrylamide) (PAM), exhibited excellent self-healing properties due to the presence of dynamic Schiff-base and hydrogen bonding interactions.98Fig. 7 shows the possible schematic explanation for the different mechanical properties of the OSA–PAM hydrogels.
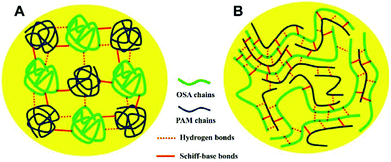 |
| Fig. 7 A possible schematic explanation for the different mechanical properties of oxidized sodium alginate (OSA)–poly(acrylamide) (PAM) hydrogels based on the (A) random coil model and (B) polysaccharide-template model. Reprinted with permission from ref. 98. Copyright 2017 Elsevier B.V. | |
3.1.2. Ionic interactions.
There are two main kinds of ionic interactions used to prepare hydrogels, (1) ionic interaction between polyanionic polymers and metal ions; (2) polyelectrolyte complexation of polyanions with polycations.99–101 The ionic interaction strategy is the most useful method to crosslink sodium alginate. Sodium alginate can form join points upon contact with divalent ions (e.g., Ca2+, Cu2+) which has been described as an “egg box structure” between four G residues.102 A series of N-carboxymethyl chitosan- and alginate-based hydrogels were prepared via both electrostatic interaction and divalent chelation with an epidermal growth factor (EGF) payload to promote the cell proliferation and wound healing (Fig. 8).42 The hydrogels crosslinked via divalent chelation alone probably cannot provide sufficient swelling capacity or mechanical properties for wound healing due to the leaking of the divalent ions from the hydrogel into the surrounding medium.103
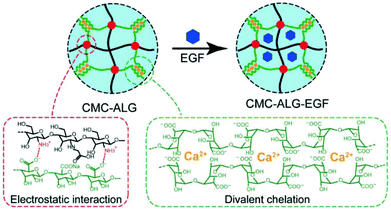 |
| Fig. 8 Schematic illustration of the N-carboxymethyl chitosan–alginate (CMC–ALG) hydrogel and epidermal growth factor loaded hydrogels (CMC–ALG–EGF) via ionic interactions. Reprinted with permission from ref. 42. Copyright 2018 WILEY-VCH Verlag GmbH & Co. KGaA, Weinheim. | |
3.1.3. Crystallization.
Microcrystals can act as join points to form 3D networks. The freeze–thaw technique is a common method to obtain microcrystals. As shown in Fig. 9, phase separation is caused by the freezing of water during the freeze–thaw process, which results in the formation of microcrystals.104 Repeated freeze–thaw cycles would strengthen the existing crystals in the structure and provide higher crystallinity and stability.105 Polysaccharide-based hydrogels can be fabricated using either polysaccharides alone or with other polymers such as polyvinyl alcohol (PVA). Xiao et al. prepared a series of complex hydrogels of PVA and sodium carboxymethyl cellulose where PVA crystalline regions served as physical crosslinks; the interaction between carboxymethyl cellulose and PVA resulted in intramolecular entanglements.106 Almost all polysaccharides can form gels by a freeze–thaw technique. This method is simple to operate, but time-consuming. Besides, the crosslinking degree and the crosslinking distribution are closely related to freezing temperature, time of the freezing period and freeze–thaw cycles.
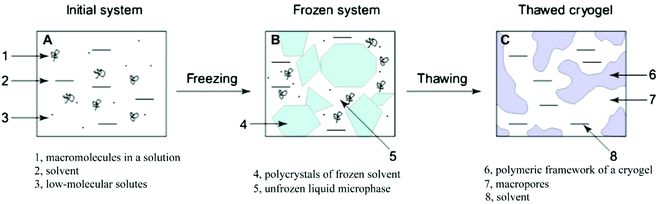 |
| Fig. 9 The main process of forming hydrogels by a freeze–thaw technique. Reprinted with permission from ref. 104. Copyright 2013 Elsevier Ltd. | |
3.1.4. Hydrophobic interactions.
The amphiphilic polymer from hydrophobic interaction self-assembles into gels in aqueous solution due to the thermodynamic incompatibility of hydrophilic and hydrophobic moieties. Two main methods were involved in the preparation of hydrogels by hydrophobic interaction. The first one is to utilize micelles as crosslinkers. Micelles can be loaded with hydrophobic drugs while serving as crosslinking points. The loading capacity and dispersion of hydrophobic drugs in hydrogels could be significantly increased via this approach. Yan and coworkers used simvastatin (a hydrophobic drug for bone regeneration) loaded aldehyde modified micelles as crosslinkers to fix the polysaccharide network (Fig. 10).107 The gelation time could be easily adjusted by controlling the oxidation degree of maltodextrin. The second method is to graft hydrophobic moieties onto the hydrophilic precursor. Bhuniya et al. synthesized a nontoxic hydrogel by grafting a hydrophobic moiety dioctylamine onto a hydrophilic precursor carboxymethyl cellulose through an amide bond formation.108 The incorporation of hydrophobic groups onto hydrophilic polysaccharide chains provides amphiphilic polysaccharides with adaptive properties.
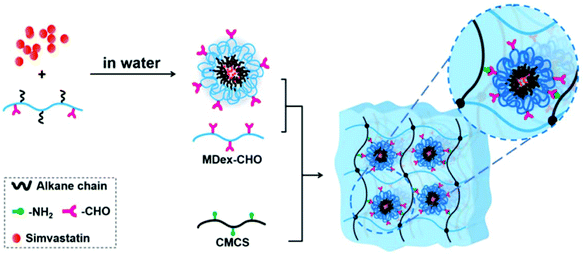 |
| Fig. 10 Schematic illustration for the preparation of simvastatin-loaded micelle crosslinked carboxymethyl chitosan (CMCS)/maltodextrin (MDex) hydrogel. Reprinted with permission from ref. 107. Copyright 2018 American Chemical Society. | |
3.2. Chemical crosslinking
Chemical crosslinking agents and techniques were utilized to enhance the morphological and biomechanical characteristics of polysaccharide-based hydrogels. Hydrogels with permanent covalent linkages usually exhibit high mechanical strength, but the stretchability and self-healing ability are limited. Chemical crosslinking would be achieved by chemical reactions of complementary groups, radiation techniques, and enzyme mediated crosslinking.
3.2.1. Crosslinking by chemical reactions of complementary groups.
The functional groups like –OH, –COOH, and –NH2 on the backbone of polysaccharides could be utilized to structure the network of hydrogels. The complementary groups can be on the chains of another component, or the additional small molecule crosslinkers were used. 3D networks would be formed via the chemical reactions of the complementary reactions, such as the –NH2/–COOH reaction, –OH/–NH2 reaction, and Schiff-base formation.109 The Schiff-base reaction is the most commonly used method to fabricate chitosan-based hydrogels.110,111 Schiff-base (imine) linkages would be hydrolyzed under acidic conditions which relates to the degradation of hydrogels and the controlled release of drugs. It should be noted that aldehyde groups are toxic for cells and would cause severe inflammation in the body. If small molecule crosslinking agents (e.g., glutaraldehyde) containing an aldehyde group are introduced during the preparation process, the residual crosslinking agents should be removed thoroughly. The carboxyl groups on hyaluronic acid could be crosslinked via condensation reactions between –COOH/–OH or –COOH/–NH2. Ester or amide bonds act as linkages to crosslink hyaluronic acid chains.112 The hyaluronic acid could also be modified in advance to form other chemically reactive groups. For instance, dopamine could be grafted onto hyaluronic acid in the presence of 1-(3-dimethylaminopropyl)-3-ethylcarbodiimide hydrochloride (EDC) and N-hydroxysuccinimide (NHS) converting part of carboxy groups to catechol groups.113 Alginate is commonly crosslinked by ionic interactions, but the carboxyl group on its backbone can also be modified.114 Cellulose, dextran, and starch are crosslinked mainly by the reaction of hydroxyl groups. The vicinal glycols in dextran could be specifically oxidized by periodate to form aldehyde groups that could subsequently react with amine groups.115
3.2.2. Radiation crosslinking.
Radiation crosslinking could be carried out in two main ways, ultraviolet (UV) light irradiation116,117 and high energy radiation (e.g., γ-irradiation118–120 and electron beam121). On exposure to radiation, radicals may be produced on polymer chains which could initiate the polymerization of unsaturated bonds, or inert groups are converted to other active groups. The radiation technique is a green approach to fabricate hydrogels since it is additive-free, and gel formation and sterilization occur simultaneously. Note that radiation can cause damage to or denaturation of polysaccharides. The balance between radiation-induced denaturation and radiation-induced crosslinking is a critical factor in the preparation of polysaccharide-based hydrogels.70 A significant advantage of the radiation technique in preparing wound dressings is controllable and rapid gelation. For example, Ouyang et al. designed a hyaluronic acid/gelatin-based hydrogel that can undergo rapid gelling and fixation to adhere and seal bleeding arteries and cardiac walls after UV light irradiation.117 Nevertheless, high cost and equipment requirements limit the widespread use of this technique.
3.2.3. Enzyme mediated crosslinking.
As an efficient method, enzymatic crosslinking has been used for the preparation of polysaccharide-based hydrogels under mild reaction conditions.111,122 However, high cost and substrate specificity limit the application of the enzymatic crosslinking strategy. Horseradish peroxidase (HRP) can catalyze the coupling of phenol or aniline derivatives via the decomposition of hydrogen peroxide.123 Microbial transglutaminase (MTGase) catalyzes an acyl-transfer reaction between the γ-carbonyl group of a glutamine residue and the ε-amino group of a lysine residue.122 Polysaccharides need to be modified first to suit the substrate requirements of specific enzymes.
It is clear that just using one type of crosslinking method is difficult to meet the performance requirements of wound dressings. The combined utilization of two or more types of crosslinking methods may achieve complementary advantages of each other. For example, chemical and physical linkages could be assigned into one hydrogel where chemical linkages are responsible for the stiffness of the hydrogel, and the physical linkages are responsible for toughness. A delicate equilibrium between each interaction requires precise regulation. In recent years, double-network hydrogels, which consist of two types of polymer components with opposite physical natures, were proposed.124–126 The minor components as the first network are crosslinked rigid skeletons, and the major components as the second network are poorly cross-linked ductile substances.27,127 New techniques make it possible for hydrogels to exhibit both mechanically robust and versatile performance.
4. Polysaccharide-based hydrogels in wound healing
4.1. Chitosan-based hydrogels
Chitosan, which is widely found in the exoskeleton of crustaceans, is derived from the deacetylation of chitin which is a linear polymer of N-acetylglucosamine. Chitosan, the only basic polysaccharide among natural polysaccharides, is positively charged because of a large number of amino groups along the molecular chains. Considering its unique properties, such as antimicrobial activity and non-antigenicity, chitosan has been widely studied for drug delivery, anti-bacteria, and tissue engineering.128,129 The antibacterial properties of chitosan are an advantage that can make the hydrogel multi-functional. Traditional dressings often encapsulate antibacterial agents to achieve antibacterial ability. Compared with dressings with releasable antibacterial agents, chitosan-based dressings with inherent antibacterial activity may present persistent antibacterial activity and reduced cytotoxicity to tissues. Ma and coworkers reported an injectable antibacterial conductive hydrogel based on glycidyl methacrylate functionalized quaternized chitosan (QCSG) and carbon nanotubes (CNTs) for lethal noncompressible hemorrhage hemostasis and wound healing (Fig. 11A). The as-prepared hydrogel presented robust mechanical strength, rapid blood-triggered shape recovery and absorption speed, and high blood uptake capacity.130 Tao and Wang proposed that the hydrogels constructed through dynamic chemical linkages (Schiff-base, disulfide, acetal, hydrazine, ester, etc.) can automatically change the shape without external stimuli, only following the natural forces of surface tension and gravity. A self-healing hydrogel based on chitosan (CP) and telechelic difunctional poly(ethylene glycol) (DF-PEG) was prepared as shown in Fig. 11B. In a healing process of the rat-liver laceration, the liver capsule treated with a thrombin-loaded hydrogel (CPT) appeared with a smooth surface and healthy color, suggesting this self-adapting hydrogel as an excellent drug carrier for in vivo wound treatment.131 Yao et al. used thermo-sensitive chitosan-based hydrogels to deliver exogenous recombinant human stromal cell-derived factor-1 alpha (SDF-1 alpha) for injured eyes. This therapeutic strategy accelerated the reconstitution of the corneal epithelium, giving it more natural structural and functional properties, and increased local expression of growth factors critical to corneal epithelial repair.132
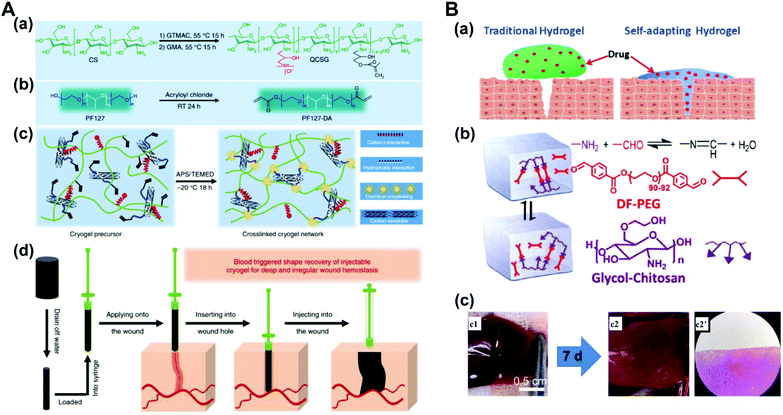 |
| Fig. 11 (A) Schematic representation of quaternized chitosan (QCSG)/carbon nanotube (CNT) cryogel synthesis. (a) Synthesis of the QCSG copolymer. (b) Synthesis of the PF127-DA copolymer. (c) Preparation of the QCSG/CNT cryogel. (d) Scheme representation of the hemostatic application of the injectable shape memory cryogel hemostatic in a deep and irregularly shaped wound model. Reprinted with permission from ref. 130. Copyright 2018 Springer Nature. (B) A self-healing hydrogel that is constructed through dynamic chemical linkages. (a) Illustration of the enhanced wound-healing by a self-adapting hydrogel. (b) Schematic illustration of the fluid properties of the solid hydrogel. (c) Photographs and microscopic evaluation of rat liver laceration with different treatments. (c1) The cross-shaped incision wound. (c2, c2′) Wound-healing after 7 days by the treatment of the CPT hydrogel. Reprinted with permission from ref. 131. Copyright 2018 American Chemical Society. | |
4.2. Alginate-based hydrogels
Alginate, extracted from seaweed, is a linear polysaccharide with homopolymeric blocks of α-L-guluronate (G) and β-D-mannuronate (M) residues. Alginate has been widely used as hydrogels for wound healing due to biocompatibility and flexible modifiability.133 The most important characteristic of alginate used to prepare hydrogels is that the G-blocks can be crosslinked with divalent cations (e.g., Ca2+, Mg2+, and Ba2+) to form hydrogels.134 Therefore, the M/G ratio, G-block length, sequence, and molecular weight are crucial to the physical properties of alginate-based hydrogels. Chang and coworkers reported a bioglass (BG)/oxidized sodium alginate (OSA) composite hydrogel with adipic acid dihydrazide (ADH)-modified γ-polyglutamic acid (γ-PGA) as the cross-linking agent. As shown in Fig. 12, this hydrogel realizes wound closure and wound healing in three ways: (a) the release of alkaline ions from BG could facilitate imine linkage formation between the hydrogel and the tissues, (b) the release of Ca2+ from BG could promote adhesion between the hydrogel and implantable biomaterials via chelation between the carboxyl groups in the alginate structure, and (c) the released Si ions would accelerate vascularization in the wound area.135 Sui and coworkers fabricated a nanoporous, ultrastrong, and gradient chitosan/sodium alginate complex hydrogel film driven by diffusing low molecular weight chitosan into high molecular weight sodium alginate solution. The as-prepared film can provide superfast temperature, ionic strength, and pH-triggered programmable deformations.101 Hytönen et al. described a nanocellulose reinforced alginate-based hydrogel suitable for 3D printing. This hydrogel can well avoid collapsing of the printing paste to produce shape fidelity of the print pattern. The 3D printable hydrogel is a novel platform for wound healing.136 Doping different ions in alginate-based hydrogels can endow them with a variety of properties. The antibacterial properties of alginate-based hydrogels were investigated where Rb+, Cu2+, Zn2+, and Sr2+ were doped in gels.137,138
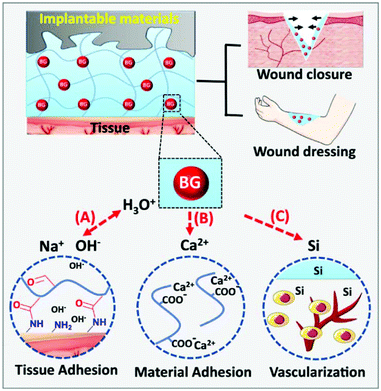 |
| Fig. 12 Dual-adhesive and bioactive bioglass (BG)/oxidized sodium alginate (OSA) hydrogels. (A) The rapid ion exchange on the BG surface makes an alkaline environment to promote imine linkage formation between the tissues and the hydrogel. (B) The BG promotes adhesion between the hydrogel and materials via chelation between the carboxyl groups and Ca2+ released from BG. (C) The released Si ions promote vascularization in the wound area. Reprinted with permission from ref. 135. Copyright 2019, Springer Nature. | |
4.3. Hyaluronic acid-based hydrogels
Hyaluronic acid is composed of alternating units of N-acetyl-D-glucosamine and D-glucuronic acid linked by β-1,4- and β-1,3-glucosidic bonds. Hyaluronic acid is the main component of connective tissues such as the extracellular matrix and synovial fluid, which play an important physiological function of retaining water, maintaining extracellular space, adjusting osmotic pressure and lubricating.48 A large number of carboxyl and hydroxyl groups are contained in hyaluronic acid, which makes it ready to form intramolecular and intermolecular hydrogen bonds in aqueous solution. Hyaluronic acid-based hydrogels are considered as a wonderful platform for wound healing due to their biodegradability, biocompatibility, nontoxicity, non-immunogenicity and non-inflammatory properties. For instance, Cho et al. developed a hyaluronic acid-based hydrogel patch as a ready-to-use tissue tape for versatile biomedical applications (Fig. 13).139 As reported by Li et al., the hydrazine conjugated on the hyaluronic acid chain via the reaction of carboxyl and amino groups. Then, hydrazine-modified hyaluronic acid reacted with benzaldehyde modified F127 to form micelle containing acylhydrazone bonds. The hydrogel was formed utilizing dynamic acylhydrazone bonding and micellization crosslinking.140 Ossipov et al. designed a kind of moldable hyaluronic acid-based hydrogel enabled by dynamic metal–bisphosphonate coordination chemistry for wound healing. Hyaluronic acid was modified with pendant bisphosphonate (BP) ligands first. After the addition of silver (Ag+) ions to the modified hyaluronic acid solution, the hydrogel was formed immediately owing to coordination crosslinking between Ag+ ions and BP groups linked to the hyaluronic acid backbone. The hydrogel exhibited excellent antimicrobial properties to both Gram-positive and Gram-negative bacterial strains, enabling the prevention of infections in wound care.141 Hyaluronic acid-based hydrogels can also be functionalized by loading drugs, factors, or cells. A pH controllable H2S donor, JK1 (synthesized from phenylphosphonothioic dichloride142), was encapsulated in hyaluronic acid-based hydrogels for wound repair via in situ polarization of M2 macrophages. The in vivo studies on dermal wounds showed that the hyaluronic acid/JK1 hybrid hydrogel significantly accelerated the wound regeneration process through enhanced re-epithelialization, collagen deposition, angiogenesis, and cell proliferation.143 An injectable hydrogel was fabricated from hyperbranched multi-acrylated poly(ethylene glycol) macromers (HP-PEGs) and thiolated hyaluronic acid (HA-SH) for a stem cell delivery and retention platform.144 Thornton et al. reported a redox-responsive hyaluronic acid-based hydrogel by selecting aminoethyl disulfide (AED) as a glutathione (GSH)-sensitive crosslinker. The redox-responsive hydrogel offers a very low-cost method for the visual detection of a target analyte that varies and is dependent on the status of cells and tissues (wound detection).145
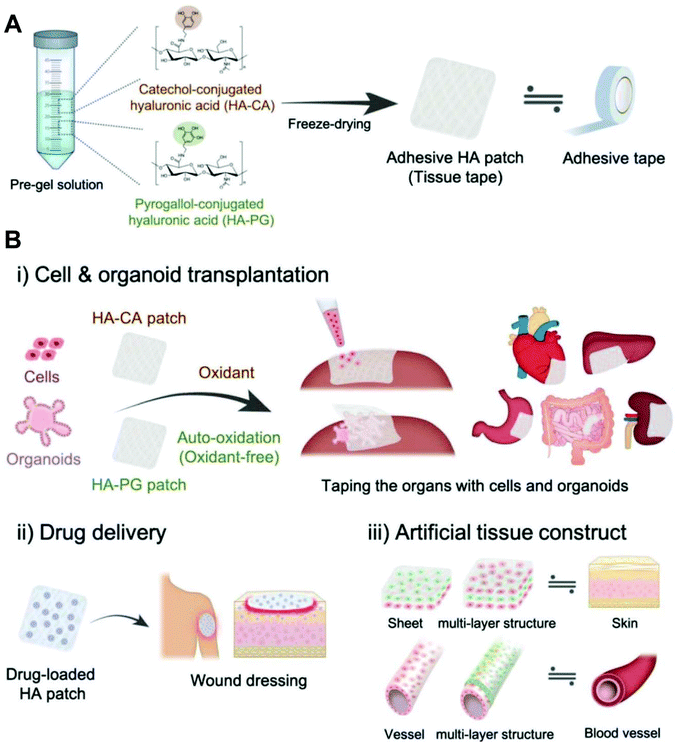 |
| Fig. 13 Adhesive hyaluronic acid patch platform for versatile biomedical applications. (A) Fabrication of catechol-modified hyaluronic acid (HA-CA) and pyrogallol-modified hyaluronic acid (HA-PG) patches as a tissue tape. (B) Various applications of oxidant-dependent HA-CA patch and oxidant-free HA-PG patch; (i) transplantation of single cells and massive cell clusters (organoids) to various types of organ, (ii) sustained drug delivery system for wound healing, and (iii) construction of multilayered tissue structures such as the skin and blood vessel. Reprinted with permission from ref. 139. Copyright 2019 WILEY-VCH Verlag GmbH & Co. KGaA, Weinheim. | |
4.4. Cellulose-based hydrogels
Cellulose, the most abundant renewable polymer on the Earth, consists of D-glucopyranose polymerized through the β-1,4-glucosidic bond. Cellulose and its derivatives have been widely used as medical materials whether for the laboratory study or clinical application due to their biocompatibility, biodegradability, low toxicity and relatively low cost.146,147 As shown in Fig. 14A, Mansur et al. synthesized a series of eco-friendly hydrogels based on carboxymethyl-functionalized cellulose derivatives chemically crosslinked by citric acid (CA) and modified with PEG using a green aqueous process.148 Tan et al. reported a kind of pH-sensitive chitosan–cellulose composite hydrogel for drug delivery and wound dressing. The adjacent hydroxyl groups on carboxymethyl cellulose were oxidized to aldehyde groups in advance. Then the aldehyde groups reacted with amine groups on carboxymethyl chitosan via the Schiff-base reaction to form gels. Silver sulfadiazine, an antimicrobial model drug, was loaded into the hydrogels and undergo controlled release in both pH 5.5 and pH 9.5.149 A quaternized hydroxyethyl cellulose/mesocellular silica foam (MCF) hydrogel sponge (QHM) was prepared via one-pot radical graft copolymerization. The hydrogel sponge could be expanded instantly triggered by water to thereby accelerate blood component concentration. Additionally, the QHM (amount of MCF, 9.8%) could activate the coagulation factors to facilitate hemostasis and wound healing.150 Cellulose-based hydrogels are also a great carrier. A bacterial cellulose/acrylic acid (BC/AA) hydrogel was loaded with human epidermal keratinocytes and human dermal fibroblasts for burn wound healing.151 The role of nanocellulose in hydrogel preparation is remarkable as well. When cellulose fibers were hydrolyzed, the fibers produced defect-free, rod-like crystalline residues which were named cellulose nanocrystals (CNs). CNs have physical and chemical properties such as high tensile strength and elastic modulus (130–150 GPa), high specific surface area (up to several hundreds of m2 g−1), low density (1.6 g cm−3), reactive surfaces, and unique morphology.47,152 The quintessential properties qualify CNs as excellent reinforcing agents while acting as crosslinking agents to structure hydrogels. Chen et al. designed a CN reinforced hydrogel crosslinked by dynamic Schiff-base linkages between amines from water-soluble carboxymethyl chitosan (CMC) and aldehydes from rigid rod-like dialdehyde-modified cellulose nanocrystals (DACNC, Fig. 14B). DACNC, on one hand, served as active junctions which can be readily broken and reformed, allowing the hydrogel to rapidly self-heal. On the other hand, DACNC acted as reinforcing fillers to improve the hydrogel strength.153
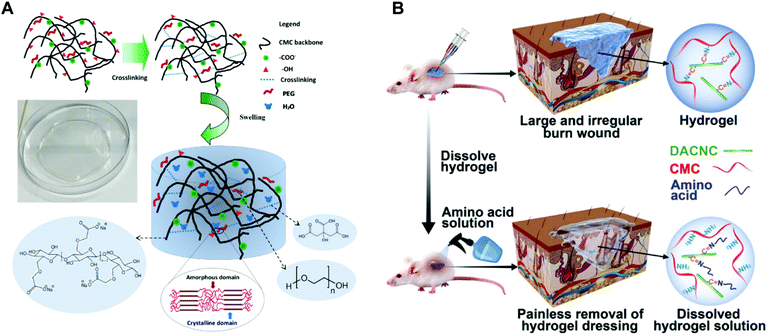 |
| Fig. 14 (A) Superabsorbent crosslinked carboxymethyl cellulose–PEG hydrogels. Swollen structure after immersion in water (not to scale). Reprinted with permission from ref. 148. Copyright 2017 Elsevier B.V. (B) On-demand dissolvable self-healing hydrogel based on carboxymethyl chitosan (CMC) and cellulose nanocrystal (DACNC) for deep partial thickness burn wound healing Reprinted with permission from ref. 153. Copyright 2018 American Chemical Society. | |
4.5. Dextran-based hydrogels
Dextran consists primarily of α-1,6-linked D-glucopyranose residues with a few percent of α-1,2-, α-1,3- or α-1,4-linked side chains.154 Like other polysaccharides, dextran has good biocompatibility and plenty of pendant hydroxyl groups which could be easily modified. It is a promising candidate for biomedical applications.155–158 Dextran-based hydrogels have been verified to facilitate neovascularization and skin regeneration remarkably in vivo.159,160 To benefit tissue infiltration, neovascularization, and hydrogel degradation, Gerecht et al. developed a dextran-based hydrogel by controlling the ratio of dextran–allyl isocyanate–ethylamine (Dex-AE)/polyethylene glycol diacrylate (PEGDA) (Fig. 15A). As shown in Fig. 15B, a 3-week comparative study indicated that the as-prepared hydrogel (Dex-AE/PEGDA = 80/20) promoted dermal regeneration with complete skin appendages.159 An ingenious method was used to load resveratrol (Res) and vascular endothelial growth factor (VEGF) plasmid into a chemically modified dextran (Dex)/hyaluronic acid (HA)/β-cyclodextrin (β-CD) compound hydrogel. Res, an anti-inflammatory substance, was embedded into the lipophilic central cavity of β-CD and plasmid DNA encoded with vascular endothelial growth factor (pDNA-VEGF) condensing by polyethyleneimine (PEI) was doped in the hydrogel. The sophisticated hydrogel speeded the burn wound healing, particularly by inhibiting inflammation response and promoting microvascular formation.161 Different kinds of antibacterial dextran-based hydrogels were prepared to load Ag nanoparticles162 or sanguinarine,163 respectively. A series of antibacterial hydrogels were also obtained by combining dextran with polyamines.164
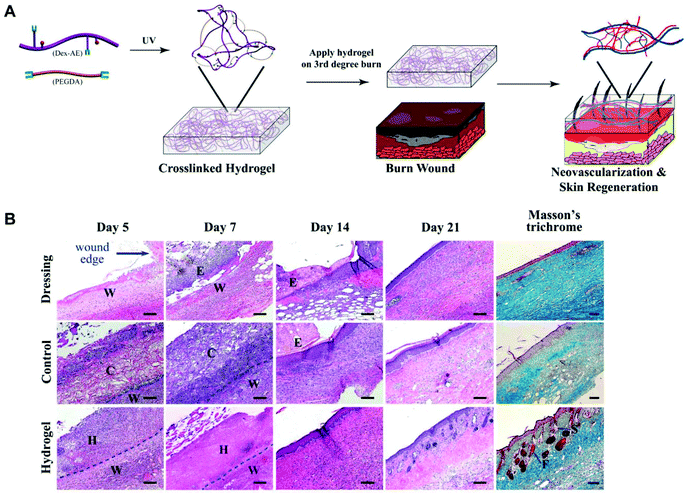 |
| Fig. 15 Dextran hydrogel as a therapeutic modality. (A) Dextran-based hydrogel promotes neovascularization: precise structure manipulation allows us to achieve rapid, efficient, and functional neovascularization. (B) Representative images of H&E-stained histological sections at time intervals show that the dextran hydrogel promoted wound healing with complete skin appendage regeneration. Masson's trichrome staining indicates distinct collagen structures formed in the dermal layer by day 21. Wound edge indicates the excision rim. W, wound area; H, hydrogel scaffold; C, control scaffold; E, eschar; F, follicle; S, sebaceous gland. Scale bars, 100 μm. Reprinted with permission from ref. 159. Copyright 2011 National Academy of Sciences. | |
4.6. Starch-based hydrogels
Starch is the second most abundant biomass material in nature. It consists of two glucosidic macromolecules: amylose and branched amylopectin. Only α-1,4-glycosidic bonds are participating in forming the amylose, but both α-1,4-glycosidic bonds and α-1,6-glycosidic bonds are there in amylopectin.46 Starch, especially modified starch, has been widely applied as a biomaterial.165,166 Starch-based hemostatic powder has been used for hemostasis in clinical practice. However, to fabricate hydrogels, starch is not as popular as the polysaccharides mentioned above. This may be since starch itself has no unique properties other than good biocompatibility. Usually, starch is blended with other polymers to develop hydrogels for wound healing. Polyvinyl alcohol is a good partner to form hydrogels due to good hydrophilicity, biocompatibility, and easy modification.167–169 Functional components can be encapsulated in starch-based hydrogels to achieve multifunctional hydrogels. For example, antibacterial ingredients like copper nanoparticles,170 Ag nanoparticles,169 zeolite nanoparticles,171 and turmeric167 have been loaded into the starch-based hydrogel to endow wound dressings with antibacterial activity.
The commonly used polysaccharides to prepare hydrogels for wound healing in recent years are summarized above. Generally, single-component hydrogels cannot meet the requirements of wound treatment. Composite hydrogels would achieve complementary advantages of each component and improve the overall performance of hydrogels. Polysaccharides with distinctive properties are more likely to be studied, such as chitosan, which has antibacterial properties, and alginate, which is easily crosslinked with divalent cations. According to the specific wound site and the required properties of the wound dressing, it is necessary to choose suitable polysaccharides as raw materials to design the desired hydrogel in a suitable manner.
5. Conclusions and perspectives
Acute and chronic wounds not only cause severe physical trauma to patients, but also bring an immense socio-economic burden. Although a series of wound dressings are commercially available, some issues concerning biological safety, therapeutic efficacy, and high cost limit their wide use. Hydrogels being used for wound healing should be capable of holding blood rapidly, promoting tissue regeneration, and they exhibit specific performances depending on the type of wound. Polysaccharides possess distinctive properties such as biocompatibility, biodegradability, and nontoxicity, and can be easily modified to subjoin versatile properties. Polysaccharide-based hydrogels as advanced wound dressings have exhibited excellent hemostatic and wound healing effects. Plenty of polysaccharide-based hydrogels are available on the market today, aiming at promoting wound healing. They include Restore® Hydrogels and Regenecare® HA (hyaluronic acid-based hydrogels by Hollister Wound Care and BioTime Inc., respectively), Intrasite® Gel and Regranex® Gel (carboxymethyl cellulose-based hydrogels by Smith & Nephew Inc.), Purilon® Gel and Nu-Gel™ Hydrogel with alginate (alginate-based hydrogels by Coloplast Ltd and Systagenix Wound Management Ltd, respectively) and Woulgan® (a glucan-based hydrogel by Biotec BetaGlucans). In light of the evidence from clinical applications of proprietary polysaccharide-based hydrogels products in acute and chronic wound management, it can be concluded that polysaccharide-based hydrogels are outstanding candidates for wound healing.
This review presented several design considerations about hydrogels for wound healing and described the current state of polysaccharide-based hydrogels as wound dressings. The commonly used crosslinking techniques, including physical, chemical, and enzymatic crosslinking, are discussed in detail. Polysaccharide-based hydrogels can provide suitable moisture for wounds and act as a shield against bacteria. Adaptable mechanical properties, degradability, and therapeutic agent controlled release of polysaccharide-based hydrogels have already been designed and prepared for effective utilization. Even so, there are still some obstacles needed to be overcome. Because the raw materials for polysaccharides are mostly from natural sources, enough attention should be paid to the potential risk of batch-to-batch variation. Besides, some properties are contradictory to the requirements of crosslinking techniques. Therefore, to meet the requirements of all aspects, a delicate equilibrium between each interaction requires precise regulation. Furthermore, in some cases, in order to improve the performance of hydrogels, the usage of crosslinking agents or other additives is unavoidable. We need to make sure that all introduced components are not toxic and the degraded components of hydrogel dressings have no negative influence on the system.
For future exploration, further improving the comprehensive properties of polysaccharide-based hydrogels is the first issue to be addressed. This development will rely on new crosslinking techniques and modification strategies of polysaccharides. Cooperation with other research areas, such as molecular biology, stem cell science, bioelectrochemistry, and angiogenesis, could be an effective way to design smart hydrogels that meet the current clinical desires.
Based on laboratory research, there are many alternative ways to prepare hydrogels with ideal performance, and hydrogels can be endowed with plenty of functions through a variety of methods. However, taking into account industrial production, how to reduce manufacturing costs and simplify the production process with minimal pollution also needs to be considered. All the properties mentioned above are complementary, called the bucket effect. Any weak factor will affect the treatment of wound dressings. Therefore, the design of hydrogels for wound healing should be considered holistically. It is conceivable that polysaccharide-based hydrogels with promising performance and therapeutic effects which are fabricated in green and effective techniques will be soon applied in clinics.
Conflicts of interest
There are no conflicts to declare.
Acknowledgements
This work is financially supported by the National Natural Science Foundation of China (51703105 and 51829301), the Natural Science Foundation of Shandong Province (ZR2017BEM012), the China Postdoctoral Science Foundation (2018M630752), the Beijing Outstanding Young Scientist Program (BJJWZYJH01201910010024), and the Fundamental Research Funds for the Central Universities (XK1802-2).
References
- R. G. Frykberg and J. Banks, Adv. Wound Care, 2015, 4, 560–582 CrossRef PubMed.
- B. K. Sun, Z. Siprashvili and P. A. Khavari, Science, 2014, 346, 941–945 CrossRef PubMed.
- Y. Zhu, J. Zhang, J. Song, J. Yang, Z. Du, W. Zhao, H. Guo, C. Wen, Q. Li, X. Sui and L. Zhang, Adv. Funct. Mater., 2019, 1905493 Search PubMed.
- X. Zhao, H. Wu, B. Guo, R. Dong, Y. Qiu and P. X. Ma, Biomaterials, 2017, 122, 34–47 CrossRef CAS PubMed.
- Z. Fan, B. Liu, J. Wang, S. Zhang, Q. Lin, P. Gong, L. Ma and S. Yang, Adv. Funct. Mater., 2014, 24, 3933–3943 CrossRef CAS.
- B. Singh and A. Dhiman, RSC Adv., 2015, 5, 44666–44678 RSC.
- J. Qu, X. Zhao, Y. Liang, T. Zhang, P. X. Ma and B. Guo, Biomaterials, 2018, 183, 185–199 CrossRef CAS.
- H. Liu, C. Y. Wang, C. Li, Y. G. Qin, Z. H. Wang, F. Yang, Z. H. Li and J. C. Wang, RSC Adv., 2018, 8, 7533–7549 RSC.
- M. Madaghiele, C. Demitri, A. Sannino and L. Ambrosio, Burns Trauma, 2014, 2, 153–161 CrossRef.
- B. V. Slaughter, S. S. Khurshid, O. Z. Fisher, A. Khademhosseini and N. A. Peppas, Adv. Mater., 2009, 21, 3307–3329 CrossRef CAS.
- A. S. Hoffman, Adv. Drug Delivery Rev., 2012, 64, 18–23 CrossRef.
- A. Mathur, S. Moorjani and A. Scranton, J. Macromol. Sci., Polym. Rev., 1996, 36, 405–430 CrossRef.
- N. Peppas, J. Hilt, A. Khademhosseini and R. Langer, Adv. Mater., 2006, 18, 1345–1360 CrossRef CAS.
- K. Campbell, R. Stilhano and E. Silva, Biomaterials, 2018, 179, 109–121 CrossRef.
- A. Dodero, L. Pianella, S. Vicini, M. Alloisio, M. Ottonelli and M. Castellano, Eur. Polym. J., 2019, 118, 586–594 CrossRef.
- H. J. Zhang, T. L. Sun, A. K. Zhang, Y. Ikura, T. Nakajima, T. Nonoyama, T. Kurokawa, O. Ito, H. Ishitobi and J. P. Gong, Adv. Mater., 2016, 28, 4884–4890 CrossRef PubMed.
- Y. Yang, X. Wang, F. Yang, H. Shen and D. Wu, Adv. Mater., 2016, 28, 7178–7184 CrossRef.
- D. Stern and H. Cui, Adv. Healthcare Mater., 2019, 8, 1900104 CrossRef.
- H. Cong, L. Zhou, Q. Meng, Y. Zhang, B. Yu, Y. Shen and H. Hu, Biomater. Sci., 2019, 7, 3918–3925 RSC.
- E. Krieg, E. Shirman, H. Weissman, E. Shimoni, S. Wolf, I. Pinkas and B. Rybtchinski, J. Am. Chem. Soc., 2009, 131, 14365–14373 CrossRef CAS PubMed.
- J.-Y. Sun, X. Zhao, W. Illeperuma, O. Chaudhuri, K. H. Oh, D. Mooney, J. Vlassak and Z. Suo, Nature, 2012, 489, 133–136 CrossRef CAS PubMed.
- L. Wang, X. Zhang, K. Yang, Y. V. Fu, T. Xu, S. Li, D. Zhang, L. N. Wang and C. S. Lee, Adv. Funct. Mater., 2020, 30, 1904156 CrossRef.
- Y. K. Chang and H. R. Allcock, Adv. Mater., 2003, 15, 537–541 CrossRef.
- A. Olad, M. Eslamzadeh and A. Mirmohseni, J. Appl. Polym. Sci., 2019, 136, 48025 CrossRef.
- J. L. Li, R. R. Xing, S. Bai and X. H. Yan, Soft Matter, 2019, 15, 1704–1715 RSC.
- Z. Wei, J. H. Yang, Z. Q. Liu, F. Xu, J. X. Zhou, M. Zrínyi, Y. Osada and Y. M. Chen, Adv. Funct. Mater., 2015, 25, 1352–1359 CrossRef.
- P. Matricardi, C. Di Meo, T. Coviello, W. E. Hennink and F. Alhaique, Adv. Funct. Mater., 2013, 65, 1172–1187 Search PubMed.
- S. Kumar, I. Marrero-Berrios, M. Kabat and F. Berthiaume, Curr. Pharm. Des., 2019, 25, 1236–1248 CrossRef.
- I. Younes and M. Rinaudo, Mar. Drugs, 2015, 13, 1133–1174 CrossRef.
- A. Gupta, M. Kowalczuk, W. Heaselgrave, S. T. Britland, C. Martin and I. Radecka, Eur. Polym. J., 2019, 111, 134–151 CrossRef.
- X. Yang, W. Liu, N. Li, M. Wang, B. Liang, I. Ullah, A. Neve, Y. Feng, H. Chen and C. Shi, Biomater. Sci., 2017, 5, 2357–2368 RSC.
- Y. Hu, Y. Li and F. J. Xu, Acc. Chem. Res., 2017, 50, 281–292 CrossRef.
- J. Y. Liu, Y. Li, Y. Hu, G. Cheng, E. Ye, C. Shen and F. J. Xu, Mater. Sci. Eng., C, 2018, 83, 160–168 CrossRef PubMed.
- H. Liu, Y. Jiao, Y. Wang, C. Zhou and Z. Zhang, Adv. Drug Delivery Rev., 2008, 60, 1650–1662 CrossRef.
- K. M. Xiu, N. Zhao, W. T. Yang and F. J. Xu, Acta Biomater., 2013, 9, 7439–7448 CrossRef CAS.
- H. Hu, W. Yuan, F. Liu, G. Cheng, F. J. Xu and J. Ma, ACS Appl. Mater. Interfaces, 2015, 7, 8942–8951 CrossRef CAS.
- Y. Yang, X. Wang, F. Yang, L. Wang and D. Wu, Adv. Mater., 2018, 30, 1707071 CrossRef.
- H. S. Du, W. M. Liu, M. L. Zhang, C. L. Si, X. Y. Zhang and B. Li, Carbohydr. Polym., 2019, 209, 130–144 CrossRef CAS.
- Z. H. Wang, Y. Zhu, M. Y. Chai, W. T. Yang and F. J. Xu, Biomaterials, 2011, 33, 1873–1883 CrossRef.
- Y. Hu, Y. Zhu, W. T. Yang and F. J. Xu, ACS Appl. Mater. Interfaces, 2012, 5, 703–712 CrossRef.
- X. Yang, M. Chai, Y. Zhu, W. T. Yang and F. J. Xu, Bioconjugate Chem., 2012, 23, 618–626 CrossRef CAS.
- Y. Hu, Z. Zhang, Y. Li, X. Ding, D. Li, C. Shen and F. J. Xu, Macromol. Rapid Commun., 2018, 39, 1800069 CrossRef.
- L. Xie, M. Shen, Y. Hong, H. Ye, L. Huang and J. Xie, Carbohydr. Polym., 2020, 229, 115436 CrossRef.
- H. Hu, H. Q. Song, B. R. Yu, Q. Cai, Y. Zhu and F. J. Xu, Polym. Chem., 2015, 6, 2466–2477 RSC.
- H. Hu, X. J. Hou, X. C. Wang, J. J. Nie, Q. Cai and F. J. Xu, Polym. Chem., 2016, 7, 3107–3116 RSC.
- T. X. Zhu, J. J. Mao, Y. Cheng, H. R. Liu, L. Lv, M. Z. Ge, S. H. Li, J. Y. Huang, Z. Chen, H. Q. Li, L. Yang and Y. K. Lai, Adv. Mater. Interfaces, 2019, 6, 1900761 CrossRef.
- Y. Habibi, L. A. Lucia and O. J. Rojas, Chem. Rev., 2010, 110, 3479–3500 CrossRef CAS.
- M. N. Collins and C. Birkinshaw, Carbohydr. Polym., 2013, 92, 1262–1279 CrossRef CAS.
- K. Y. Lee and D. J. Mooney, Prog. Polym. Sci., 2012, 37, 106–126 CrossRef CAS.
- R. A. A. Muzzarelli, F. Greco, A. Busilacchi, V. Sollazzo and A. Gigante, Carbohydr. Polym., 2012, 89, 723–739 CrossRef CAS PubMed.
- L. E. Dickinson and S. Gerecht, Front. Physiol., 2016, 7, 341 CrossRef.
- C. Martin, W. L. Low, M. C. I. M. Amin, I. Radecka, P. Raj and K. Kenward, Pharm. Pat. Anal., 2013, 2, 341–359 CrossRef CAS.
- T. Dargaville, B. Farrugia, J. Broadbent, S. Pace, Z. Upton and N. Voelcker, Biosens. Bioelectron., 2013, 41, 30–42 CrossRef CAS.
- D. Margolis, O. Hoffstad, J. Nafash, C. Leonard, C. Freeman, S. Hennessy and D. Wiebe, Diabetes Care, 2011, 34, 2363–2367 CrossRef.
- P. H. Wang, B. S. Huang, H. C. Horng, C. C. Yeh and Y. J. Chen, J. Chin. Med. Assoc., 2018, 81, 94–101 CrossRef.
- X. Yang, W. Liu, N. Li, M. Wang, B. Liang, I. Ullah, A. Luis Neve, Y. Feng, H. Chen and C. Shi, Biomater. Sci., 2017, 5, 2357–2368 RSC.
- S. D. Tymen, I. G. Rojas, X. Zhou, Z. J. Fang, Y. Zhao and P. T. Marucha, Brain, Behav., Immun., 2013, 28, 207–217 CrossRef CAS.
- T. Velnar, T. Bailey and V. Smrkolj, J. Int. Med. Res., 2009, 37, 1528–1542 CrossRef CAS.
- S. Singh, A. Young and C. E. McNaught, Surgery, 2017, 35, 473–477 Search PubMed.
-
L. Häkkinen, L. Koivisto, J. Heino and H. Larjava, in Stem Cell Biology and Tissue Engineering in Dental Sciences, ed. A. Vishwakarma, P. Sharpe, S. Shi and M. Ramalingam, Academic Press, Boston, 2015, pp. 669–690 Search PubMed.
- H. Kuivaniemi and G. Tromp, Gene, 2019, 707, 151–171 CrossRef CAS.
- A. Gosain and L. DiPietro, World J. Surg., 2004, 28, 321–326 CrossRef PubMed.
- S. Schreml, R. M. Szeimies, L. Prantl, M. Landthaler and P. Babilas, J. Am. Acad. Dermatol., 2010, 63, 866–881 CrossRef.
- Z. Hussain, H. E. Thu, S. F. Ng, S. Khan and H. Katas, Colloids Surf., B, 2017, 150, 223–241 CrossRef CAS.
- D. Simões, S. Miguel, M. Ribeiro, P. Coutinho, A. Mendonça and I. Correia, Eur. J. Pharm. Biopharm., 2018, 127, 130–141 CrossRef.
- G. Han and R. Ceilley, Adv. Ther., 2017, 34, 599–610 CrossRef.
- X. Ding, S. Duan, X. Ding, R. Liu and F. J. Xu, Adv. Funct. Mater., 2018, 28, 1802140 CrossRef.
- J. Li, X. Li, X. Ni, X. Wang, H. Li and K. W. Leong, Biomaterials, 2006, 27, 4132–4140 CrossRef CAS.
- S. Naahidi, M. Jafari, M. Logan, Y. Wang, Y. Yuan, H. Bae, B. Dixon and P. Chen, Biotechnol. Adv., 2017, 35, 530–544 CrossRef CAS.
- A. Oryan, A. Kamali, A. Moshiri, H. Baharvand and H. Daemi, Int. J. Biol. Macromol., 2017, 107, 678–688 CrossRef.
- S.-S. Kim, S.-H. Lim, S. Cho, S. Gwak, Y. Hong, B.-C. Chang, M. H. Park, K. Song, C. Choi and B. Kim, Exp. Mol. Med., 2006, 38, 273–283 CrossRef CAS PubMed.
- Y. Li, J. Rodrigues and H. Tomás, Chem. Soc. Rev., 2012, 41, 2193–2221 RSC.
- M. Swierczewska, H. S. Han, K. Kim, J. H. Park and S. Lee, Adv. Drug Delivery Rev., 2016, 99, 70–84 CrossRef CAS.
- L. M. Zhang, C. X. Wu, J. Y. Huang, X. H. Peng, P. Chen and S. Q. Tang, Carbohydr. Polym., 2012, 88, 1445–1452 CrossRef CAS.
- S. Sakai, T. Matsuyama, K. Hirose and K. Kawakami, Biomacromolecules, 2010, 11, 1370–1375 CrossRef CAS.
- S. G. Levesque and M. S. Shoichet, Adv. Funct. Mater., 2007, 18, 874–885 CAS.
- J. P. Gong, Science, 2014, 344, 161–162 CrossRef CAS PubMed.
- D. Gan, W. Xing, L. Jiang, J. Fang, C. Zhao, F. Ren, L. Fang, K. Wang and X. Lu, Nat. Commun., 2019, 10, 1487–1496 CrossRef PubMed.
- C. Ghobril and M. W. Grinstaff, Chem. Soc. Rev., 2015, 44, 1820–1835 RSC.
- R. Wang, J. Z. Li, W. Chen, T. T. Xu, S. F. Yun, Z. Xu, Z. Q. Xu, T. Sato, B. Chi and H. Xu, Adv. Funct. Mater., 2017, 27, 1604894 CrossRef.
- Z. Wei, J. H. Yang, Z. Q. Liu, F. Xu, J. X. Zhou, M. Zrinyi, Y. Osada and Y. M. Chen, Adv. Funct. Mater., 2015, 25, 1352–1359 CrossRef CAS.
- Z. X. Deng, Y. Guo, X. Zhao, P. X. Ma and B. L. Guo, Chem. Mater., 2018, 30, 1729–1742 CrossRef CAS.
- P. Del Gaudio, F. De Cicco, R. P. Aquino, P. Picerno, P. Russo, F. Dal Piaz, V. Bizzarro, R. Belvedere, L. Parente and A. PetrellaDepartment, Carbohydr. Polym., 2015, 115, 629–635 CrossRef.
- J. Wu, A. Q. Chen, Y. J. Zhou, S. Zheng, Y. Yang, Y. An, K. Xu, H. C. He, J. M. Kang, J. A. Luckanagul, M. Xian, J. Xiao and Q. Wang, Biomaterials, 2019, 222, 119398 CrossRef.
- Q. Xu, A. Sigen, Y. S. Gao, L. R. Guo, J. Creagh-Flynn, D. Z. Zhou, U. Greiser, Y. X. Dong, F. G. Wang, H. Y. Tai, W. G. Liu, W. Wang and W. X. Wang, Acta Biomater., 2018, 75, 63–74 CrossRef.
- M. Goh, Y. Hwang and G. Tae, Carbohydr. Polym., 2016, 147, 251–260 CrossRef.
- M. Kucharzewski, E. Rojczyk, K. Wilemska-Kucharzewska, R. Wilk, J. Hudecki and M. J. Los, Eur. J. Pharmacol., 2019, 843, 307–315 CrossRef.
- C. G. Wang, M. Wang, T. Z. Xu, X. X. Zhang, C. Lin, W. Y. Gao, H. Z. Xu, B. Lei and C. Mao, Theranostics, 2019, 9, 65–76 CrossRef.
- L. Mi, H. Xue, Y. Li and S. Jiang, Adv. Funct. Mater., 2011, 21, 4028–4034 CrossRef.
- X. Zhao, H. Wu, B. Guo, R. Dong, Y. Qiu and P. Ma, Biomaterials, 2017, 122, 34–47 CrossRef PubMed.
- T. R. Nimal, G. Baranwal, M. C. Bavya, R. Biswas and R. Jayakumar, ACS Appl. Mater. Interfaces, 2016, 8, 22074–22083 CrossRef PubMed.
- F. Fan, P. Zhang, L. Wang, T. Sun, C. Cai and G. Yu, Biomacromolecules, 2019, 20, 3798–3808 CrossRef.
- A. Oryan, A. Kamali, A. Moshiri, H. Baharvand and H. Daemi, Int. J. Biol. Macromol., 2018, 107, 678–688 CrossRef.
- J. Yang, F. Xu and C. R. Han, Biomacromolecules, 2017, 18, 1019–1028 CrossRef.
- E. M. Ahmed, J. Adv. Res., 2015, 6, 105–121 CrossRef PubMed.
- F. Ullah, M. B. H. Othman, F. Javed, Z. Ahmad and H. M. Akil, Mater. Sci. Eng., C, 2015, 57, 414–433 CrossRef PubMed.
- M. R. Kummara, A. Kumar and S. S. Han, Int. J. Biol. Macromol., 2017, 101, 165–171 CrossRef.
- S. Liu, M. Kang, K. Li, F. Yao, O. Oderinde, G. Fu and L. Xu, Chem. Eng. J., 2017, 334, 2222–2230 CrossRef.
- M. R. Park, B. B. Seo and S. C. Song, Biomaterials, 2013, 34, 1327–1336 CrossRef.
- P. Sacco, M. Borgogna, A. Travan, E. Marsich, S. Paoletti, F. Asaro, M. Grassi and I. Donati, Biomacromolecules, 2014, 15, 3396–3405 CrossRef PubMed.
- H. Cui, N. Pan, W. Fan, C. Liu, Y. Li, Y. Xia and K. Sui, Adv. Funct. Mater., 2019, 29, 1807692 CrossRef.
- S. Akay, R. Heils, H. K. Trieu, I. Smirnova and O. Yesil-Celiktas, Carbohydr. Polym., 2017, 161, 228–234 CrossRef PubMed.
- M. Shoichet, R. Li, M. White and S. Winn, Biotechnol. Bioeng., 1996, 50, 374–381 CrossRef PubMed.
- V. I. Lozinsky, I. Y. Galaev, F. M. Plieva, I. N. Savina, H. Jungvid and B. Mattiasson, Trends Biotechnol., 2003, 21, 445–451 CrossRef PubMed.
- H. Zhang, F. Zhang and J. Wu, React. Funct. Polym., 2013, 73, 923–928 CrossRef.
- C. Xiao and Y. Gao, J. Appl. Polym. Sci., 2008, 107, 1568–1572 CrossRef.
- S. Yan, J. Ren, Y. Jian, W. Wang, W. Yun and J. Yin, Biomacromolecules, 2018, 19, 4554–4564 CrossRef.
- R. Fredrick, A. Podder, A. Viswanathan and S. Bhuniya, J. Appl. Polym. Sci., 2019, 136, 47665 CrossRef.
- M. F. Akhtar, M. Hanif and N. M. Ranjha, Saudi Pharm. J., 2016, 24, 554–559 CrossRef.
- M. C. G. Pellá, M. K. Lima-Tenório, E. T. Tenório-Neto, M. R. Guilherme, E. C. Muniz and A. F. Rubira, Carbohydr. Polym., 2018, 196, 233–245 CrossRef PubMed.
- N. Bhattarai, J. Gunn and M. Q. Zhang, Adv. Drug Delivery Rev., 2010, 62, 83–99 CrossRef PubMed.
- M. N. Collins and C. Birkinshaw, Carbohydr. Polym., 2013, 92, 1262–1279 CrossRef PubMed.
- Y. P. Liang, X. Zhao, T. L. Hu, B. J. Chen, Z. H. Yin, P. X. Ma and B. L. Guo, Small, 2019, 15, 1900046 CrossRef PubMed.
- C. Lee, J. Shin, J. S. Lee, E. Byun, J. H. Ryu, S. H. Um, D. I. Kim, H. Lee and S. W. Cho, Biomacromolecules, 2013, 14, 2004–2013 CrossRef.
- Z. Y. Li, B. M. Yuan, X. M. Dong, L. J. Duan, H. Y. Tian, C. L. He and X. S. Chen, RSC Adv., 2015, 5, 94248–94256 RSC.
- K. Obara, M. Ishihara, T. Ishizuka, M. Fujita, Y. Ozeki, T. Maehara, Y. Saito, H. Yura, T. Matsui, H. Hattori, M. Kikuchi and A. Kurita, Biomaterials, 2003, 24, 3437–3444 CrossRef.
- Y. Hong, F. F. Zhou, Y. J. Hua, X. Z. Zhang, C. Y. Ni, D. H. Pan, Y. Q. Zhang, D. M. Jiang, L. Yang, Q. N. Lin, Y. W. Zou, D. S. Yu, D. E. Arnot, X. H. Zou, L. Y. Zhu, S. F. Zhang and H. W. Ouyang, Nat. Commun., 2019, 10, 2060 CrossRef.
- X. Huang, Y. Q. Zhang, X. M. Zhang, L. Xu, X. Chen and S. C. Wei, Mater. Sci. Eng., C, 2013, 33, 4816–4824 CrossRef PubMed.
- B. Singh and Rajneesh, J. Drug Delivery Sci. Technol., 2018, 47, 200–208 CrossRef.
- C. Yang, L. Xu, Y. Zhou, X. M. Zhang, X. Huang, M. Wang, Y. Han, M. L. Zhai, S. C. Wei and J. Q. Li, Carbohydr. Polym., 2010, 82, 1297–1305 CrossRef CAS.
- W. Mozalewska, R. Czechowska-Biskup, A. K. Olejnik, R. A. Wach, P. Ulański and J. M. Rosiak, Radiat. Phys. Chem., 2017, 134, 1–7 CrossRef CAS.
- J. Guo, Y. Zhang and X. Q. Yang, Food Hydrocolloids, 2012, 26, 277–285 CrossRef CAS.
- F. Chen, S. Yu, B. Liu, Y. Ni, C. Yu, Y. Su, X. Zhu, X. Yu, Y. Zhou and D. Yan, Sci. Rep., 2016, 6, 20014 CrossRef CAS.
- J. Y. Sun, X. H. Zhao, W. R. K. Illeperuma, O. Chaudhuri, K. H. Oh, D. J. Mooney, J. J. Vlassak and Z. G. Suo, Nature, 2012, 489, 133–136 CrossRef CAS.
- Q. Chen, L. Zhu, H. Chen, H. L. Yan, L. N. Huang, J. Yang and J. Zheng, Adv. Funct. Mater., 2015, 25, 1598–1607 CrossRef CAS.
- J. Malda, J. Visser, F. P. Melchels, T. Jungst, W. E. Hennink, W. J. A. Dhert, J. Groll and D. W. Hutmacher, Adv. Mater., 2013, 25, 5011–5028 CrossRef CAS PubMed.
- J. P. Gong, Soft Matter, 2010, 6, 2583–2590 RSC.
- Y. Ping, C. D. Liu, G. P. Tang, J. S. Li, J. Li, W. T. Yang and F. J. Xu, Adv. Funct. Mater., 2010, 20, 3106–3116 CrossRef CAS.
- F. Tao, Y. Cheng, X. Shi, H. Zheng, Y. Du, W. Xiang and H. Deng, Carbohydr. Polym., 2020, 230, 115658 CrossRef PubMed.
- X. Zhao, B. L. Guo, H. Wu, Y. P. Liang and P. X. Ma, Nat. Commun., 2018, 9, 2784 CrossRef PubMed.
- Y. Li, X. Wang, Y. N. Fu, Y. Wei, L. Zhao and L. Tao, ACS Appl. Mater. Interfaces, 2018, 10, 26046–26055 CrossRef CAS.
- Q. Tang, C. Luo, B. Lu, Q. Fu, H. Yin, Z. Qin, D. Lyu, L. Zhang, Z. Fang, Y. Zhu and K. Yao, Acta Biomater., 2017, 61, 101–113 CrossRef CAS.
- K. Lee and D. Mooney, Prog. Polym. Sci., 2012, 37, 106–126 CrossRef CAS PubMed.
- C. Lee, J. Shin, J. S. Lee, E. Byun, J. H. Ryu, S. H. Um, D. I. Kim, H. Lee and S. W. Cho, Biomacromolecules, 2013, 14, 2004–2013 CrossRef CAS PubMed.
- L. Gao, Y. Zhou, J. Peng, C. Xu, Q. Xu, M. Xing and J. Chang, NPG Asia Mater., 2019, 11, 66 CrossRef.
- J. Leppiniemi, P. Lahtinen, A. Paajanen, R. Mahlberg, S. Metsä-Kortelainen, T. Pinomaa, H. Pajari, I. Vikholm-Lundin, P. Pursula and V. P. Hytönen, ACS Appl. Mater. Interfaces, 2017, 9, 21959–21970 CrossRef CAS PubMed.
- X. He, Y. Ding, W. Xie, R. Sun, N. Hunt, J. Song, X. Sun, C. Peng, Q. Zeng, Y. Tan and Y. Liu, ACS Biomater. Sci. Eng., 2019, 5, 4726–4738 CrossRef CAS.
- Q. Zhou, H. Kang, M. Bielec, X. Wu, Q. Cheng, W. Wei and H. Dai, Carbohydr. Polym., 2018, 197, 292–304 CrossRef CAS PubMed.
- J. Shin, S. Choi, J. H. Kim, J. H. Cho, Y. Jin, S. Kim, S. Min, S. K. Kim, D. Choi and S. W. Cho, Adv. Funct. Mater., 2019, 29, 1903863 CrossRef CAS.
- Z. Li, F. Zhou, Z. Li, S. Lin, L. Chen, L. Liu and Y. Chen, ACS Appl. Mater. Interfaces, 2018, 10, 25194–25202 CrossRef CAS.
- L. Shi, Y. Zhao, Q. Xie, C. Fan, J. Hilborn, J. Dai and D. Ossipov, Adv. Healthcare Mater., 2017, 7, 1700973 CrossRef.
- J. Kang, Z. Li, C. L. Organ, C. M. Park, C. T. Yang, A. Pacheco, D. Wang, D. J. Lefer and M. Xian, J. Am. Chem. Soc., 2016, 138, 6336–6339 CrossRef CAS PubMed.
- J. Wu, A. Chen, Y. Zhou, S. Zheng, Y. Yang, Y. An, K. Xu, H. He, J. Kang, J. Luckanagul, M. Xian, J. Xiao and Q. Wang, Biomaterials, 2019, 222, 119398 CrossRef CAS PubMed.
- Q. Xu, S. Aa, Y. Gao, L. Guo, J. Creagh-Flynn, D. Zhou, U. Greiser, Y. Dong, F. Wang, H. Tai, W. Liu, W. Wang and W. Wang, Acta Biomater., 2018, 75, 63–74 CrossRef CAS.
- Z. Gao, B. Gotland, G. Tronci and P. D. Thornton, J. Mater. Chem. B, 2019, 7, 7494–7501 RSC.
- Y. Hu, C. Wen, L. Song, N. Zhao and F. J. Xu, J. Controlled Release, 2017, 255, 154–163 CrossRef CAS PubMed.
- X. Shen, J. Shamshina, P. Berton, G. Gurau and R. Rogers, Green Chem., 2016, 18, 53–75 RSC.
- N. Capanema, H. Mansur, A. Caires, S. Carvalho, L. Oliveira and H. Mansur, Int. J. Biol. Macromol., 2017, 106, 1218–1234 CrossRef.
- S. Du, X. Chen, X. Chen, S. Li, G. Yuan, T. Zhou, J. Li, Y. Jia, D. Xiong and H. Tan, Macromol. Chem. Phys., 2019, 220, 1900399 CrossRef CAS.
- C. W. Wang, H. Y. Niu, X. Y. Ma, H. Hong, Y. Yuan and C. S. Liu, ACS Appl. Mater. Interfaces, 2019, 11, 34595–34608 CrossRef CAS PubMed.
- N. Mohamad, E. Y. X. Loh, M. B. Fauzi, M. H. Ng and M. Amin, Drug Delivery Transl. Res., 2019, 9, 444–452 CrossRef CAS PubMed.
- H. Du, W. Liu, M. Zhang, C. Si, X. Zhang and B. Li, Drug Delivery Transl. Res., 2019, 209, 130–144 CAS.
- W. J. Huang, Y. X. Wang, Z. Q. Huang, X. L. Wang, L. Y. Chen, Y. Zhang and L. N. Zhang, ACS Appl. Mater. Interfaces, 2018, 10, 41076–41088 CrossRef CAS.
- R. Mehvar, J. Controlled Release, 2000, 69, 1–25 CrossRef CAS.
- J. Y. Liu, Y. Li, Y. Hu, G. Cheng, E. Y. Ye, C. A. Shen and F. J. Xu, Mater. Sci. Eng., C, 2018, 83, 160–168 CrossRef CAS PubMed.
- H. Fang, J. Chen, L. Lin, F. Liu, H. Tian and X. Chen, ACS Appl. Mater. Interfaces, 2019, 11, 47785–47797 CrossRef CAS PubMed.
- Y. L. Li, L. Zhu, Z. Liu, R. Cheng, F. Meng, J. H. Cui, S. J. Ji and Z. Zhong, Angew. Chem., Int. Ed., 2009, 48, 9914–9918 CrossRef CAS PubMed.
- A. R. Unnithan, N. A. M. Barakat, P. B. T. Pichiah, G. Gnanasekaran, R. Nirmala, Y. S. Cha, C. H. Jung, M. El-Newehy and H. Y. Kim, Carbohydr. Polym., 2012, 90, 1786–1793 CrossRef CAS PubMed.
- G. Sun, X. Zhang, Y. I. Shen, R. Sebastian, L. E. Dickinson, K. Fox-Talbot, M. Reinblatt, C. Steenbergen, J. W. Harmon and S. Gerecht, Proc. Natl. Acad. Sci. U. S. A., 2011, 108, 20976–20981 CrossRef CAS PubMed.
- G. Sun, Y. I. Shen, S. Kusuma, K. Fox-Talbot, C. J. Steenbergen and S. Gerecht, Biomaterials, 2011, 32, 95–106 CrossRef CAS PubMed.
- P. Wang, S. B. Huang, Z. C. Hu, W. Yang, Y. Lan, J. Y. Zhu, A. Hancharou, R. Guo and B. Tang, Acta Biomater., 2019, 100, 191–201 CrossRef CAS PubMed.
- G. F. Shi, W. T. Chen, Y. Zhang, X. M. Dai, X. G. Zhang and Z. M. Wu, Langmuir, 2019, 35, 1837–1845 CrossRef CAS PubMed.
- Q. Y. Zhu, M. Jiang, Q. Liu, S. N. Yan, L. B. Feng, Y. Lan, G. Q. Shan, W. Xue and R. Guo, Biomater. Sci., 2018, 6, 2472–2486 RSC.
- N. A. O'Connor, A. Abugharbieh, F. Yasmeen, E. Buabeng, S. Mathew, D. Samaroo and H. P. Cheng, Int. J. Biol. Macromol., 2015, 72, 88–93 CrossRef PubMed.
- R. Wang, H. Hu, Q. Cai, N. Zhao, Y. Zhu and F. J. Xu, Sci. China: Chem., 2015, 58, 1461–1470 CrossRef CAS.
- A. Rodrigues and M. Emeje, Carbohydr. Polym., 2012, 87, 987–994 CrossRef CAS.
- A. Hassan, M. B. K. Niazi, A. Hussain, S. Farrukh and T. Ahmad, J. Polym. Environ., 2018, 26, 235–243 CrossRef CAS.
- S. Baghaie, M. T. Khorasani, A. Zarrabi and J. Moshtaghian, J. Biomater. Sci., Polym. Ed., 2017, 28, 2220–2241 CrossRef CAS PubMed.
- S. Batool, Z. Hussain, M. B. K. Niazi, U. Liaqat and M. Afzal, J. Drug Delivery Sci. Technol., 2019, 52, 403–414 CrossRef CAS.
- M. E. Villanueva, A. M. D. Diez, J. A. Gonzalez, C. J. Perez, M. Orrego, L. Piehl, S. Teves and G. J. Copello, ACS Appl. Mater. Interfaces, 2016, 8, 16280–16288 CrossRef CAS PubMed.
- H. Salehi, M. Mehrasa, B. Nasri-Nasrabadi, M. Doostmohammadi, R. Seyedebrahimi, N. Davari, M. Rafienia, M. E. Hosseinabadi, M. Agheb and M. Siavash, J. Res. Med. Sci., 2017, 22, 110–118 CrossRef PubMed.
|
This journal is © The Royal Society of Chemistry 2020 |