DOI:
10.1039/D4TB00846D
(Paper)
J. Mater. Chem. B, 2024,
12, 6128-6136
Fluorinated BPA derivatives enhanced 10B delivery in tumors†
Received
18th April 2024
, Accepted 20th May 2024
First published on 22nd May 2024
Abstract
Boron neutron capture therapy (BNCT) is an emerging approach for treating malignant tumors with binary targeting. However, its clinical application has been hampered by insufficient 10B accumulation in tumors and low 10B concentration ratios of tumor-to-blood (T/B) and tumor-to-normal tissue (T/N). Herein, we developed fluorinated BPA derivatives with different fluorine groups as boron delivery agents for enabling sufficient 10B accumulation in tumors and enhancing T/B and T/N ratios. Our findings demonstrated that fluorinated BPA derivatives had good biological safety. Furthermore, fluorinated BPA derivatives showed improved 10B accumulation in tumors and enhanced T/B and T/N ratios compared to the clinical boron drug fructose-BPA (f-BPA). In particular, in B16-F10 tumor-bearing mice, fluorinated BPA derivatives met the requirements for clinical BNCT even at half of the clinical dose. Thus, fluorinated BPA derivatives are potentially effective boron delivery agents for clinical BNCT in melanoma.
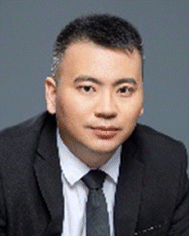
Zhenhua Li
| Dr. Zhenhua Li completed his doctorate in inorganic chemistry at Changchun Institute of Applied Chemistry, Chinese Academy of Sciences in 2015. After postdoc training at the Joint Department of Biomedical Engineering, University of North Carolina (UNC) at Chapel Hill and North Carolina State University, he joined the 10th affiliated Hospital, Southern Medical University. His research focuses on the design of biomimetic materials for cancer therapy and regenerative medicine. |
1. Introduction
Boron neutron capture therapy (BNCT) is an emerging radiotherapy with binary targeting properties, which was clinically approved in Japan in 2020 and exhibited excellent efficacy in inhibiting locally aggressive tumors.1–4 The high-energy alpha particles (4He) and lithium-7 (7Li) nuclei released during BNCT can selectively destroy cancer cells without damaging the surrounding normal tissues due to the killing range (5–9 μm) of these nuclei being approximately the diameter of the cells.5,6 Clinical trials showed that BNCT had been the most promising treatment for infiltrative, diffuse, and metastatic tumors, including glioblastoma, malignant melanoma, and recurrent head and neck cancers.7–11
Successful BNCT relies on the capture of thermal neutrons by 10B in tumor cells.12 Therefore, the key to BNCT drug development is to realize the highly selective 10B accumulation in tumor cells. Ideal boron delivery agents require the following conditions, such as the 10B concentration ratios of tumor-to-blood (T/B) and tumor-to-normal tissue (T/N) being not less than 3 (T/B > 3
:
1 and T/N > 3
:
1), sufficient 10B contents (>20 μg 10B per g tumor) in tumor cells, rapid clearance from blood and normal tissues, and prolonged retention time in the tumor.13–15 Currently, only two boron compounds, 4-boronophenylalanine (BPA) and disodium mercaptoundecahydro-closo-dodecaborate (BSH), are approved to apply in clinical BNCT trials.16–19 Although BSH has a higher boron content, its tumor specificity is relatively poor. A large number of studies have demonstrated that BPA can selectively enter into cancer cells by the overexpressed L-amino acid transporter-1 (LAT-1) in tumor cells due to the structure of phenylalanine.20,21 But BPA has poor water solubility and fructose is utilized to increase the aqueous solubility of BPA by binding to the boric acid of BPA for clinical applications.22–24 Nonetheless, insufficient 10B accumulation in tumors and low T/B ratios are still the main reasons limiting the effectiveness of BNCT.14 Therefore, it is crucial to develop novel boron drugs which can increase the 10B concentration in tumor cells and enhance T/B and T/N ratios for BNCT treatment.
Recent studies have shown that the presence of fluorocarbon bonds can improve the permeability of cell membranes and the pharmacokinetic properties of drugs leading to enhanced drug uptake in tumor cells.25–28 Fluorine substituents have been common ingredients in drugs in recent decades, especially in oncology applications, where approximately 50% of marketed drugs are fluorinated, including a variety of antitumor drugs.29,30 Besides, fluorine can increase the affinity for natural receptors and improve metabolic stability due to its polar hydrophobicity, thereby enhancing drug availability.31 Metelev et al. reported the synthesis of fluorocarbon-based oligodeoxynucleotide duplexes (ODND) and demonstrated a significant increase in cellular uptake after modification with fluorocarbons. Thus, it is believed that the high affinity of modification with fluorocarbons for cell membranes contributes to enhanced intracellular uptake.32
Based on these, in this work, we synthesized fluorinated BPA derivatives with different fluorine groups by a simple method to investigate their 10B delivery efficiency in both glioblastoma and melanoma models commonly used in clinical BNCT (Fig. 1). Our findings indicated that the cellular uptake of fluorinated BPA derivatives was higher than that of f-BPA in both GL261 and B16-F10 tumor cells and was in accordance with the requirement of BNCT (>109 10B per cell, i.e., 16.67 ng 10B per 106 cells). Notably, the 10B uptake in B16-F10 cells was higher than that in GL261 cells. Moreover, fluorinated BPA derivatives demonstrated higher 10B accumulation in tumors and higher T/B and T/N ratios than f-BPA in GL261 and B16-F10 tumor-bearing mice, whereas they showed insufficient 10B uptake in GL261 tumor-bearing mice according to the BNCT requirement (>20 μg 10B per g tumor). In contrast, in B16-F10 tumor-bearing mouse models, the 10B content in tumors reached 39.78 ± 4.52 ng g−1 tumor for 2F-BPA, 40.20 ± 2.83 ng g−1 tumor for 3F-BPA, and 38.42 ± 3.28 ng g−1 tumor for 4F-BPA, significantly surpassing the requirements for 10B content in tumors. Furthermore, the biosafety of F-containing drugs is of great concern, and the results of our safety experiments showed that fluorinated BPA derivatives had better biocompatibility in GL261 and B16-F10 cells and had no significant systemic toxicity in vivo. Therefore, fluorinated BPA derivatives have great potential to become efficient boron delivery agents in malignant melanoma for clinical BNCT.
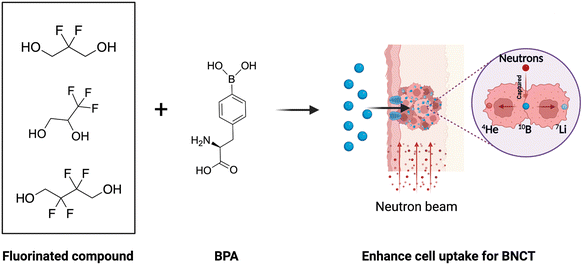 |
| Fig. 1 Schematic illustration of fluorinated BPA derivatives enhancing the 10B accumulation in tumors in vivo. The figure was created with BioRender.com. | |
2. Materials and methods
2.1. Materials and characterization
10BPA was purchased from Sunshine Lake Pharma Co., Ltd (China). Fructose was purchased from Aladdin Chemical Reagent Co., Ltd (China). 2,2-difluoropropane-1,3-diol, 2,2-difluoropropane-1,3-diol, and 2,2,3,3-tetrafluorobutane-1,4-diol were purchased from Bidepharm (China). HNO3 was purchased from Damao. The cell counting kit-8 (CCK-8) was purchased from Bioss Biotechnology Co., Ltd (China). All chemical reagents were used directly without additional purification. 1H nuclear magnetic resonance (NMR) spectra were measured using an Advance Neo 400 MHz spectrometer (Bruker). Electrospray ionization mass spectrometry (ESI-MS) was conducted using an ISQ EM model (Thermo Scientific) mass spectrometer. The 10B contents were analyzed by ICP-MS (Thermo Scientific).
2.2. Synthesis of fluorinated BPA derivatives
1 g of BPA was added into 10 mL of water with stirring, and then 1 M HCl was dropwise added into the BPA solution until the solution turned clear from turbid. Then BPA·HCl as a white powder was obtained after freeze-drying.
Small molecules with different fluorine groups and BPA·HCl (at a molar ratio of 1
:
1.5) were added into dry DMSO with stirring. After 24 h, the solution was mixed with water and filtered using 0.22 μm PES syringe filters. The final compounds were obtained after freeze-drying and then characterized by 1H NMR (D2O) and ESI-MS.
(S)-2-Amino-3-(4-(5,5-difluoro-1,3,2-dioxaborinan-2-yl)phenyl)propanoic acid (2F-BPA).
1H NMR (400 MHz, D2O) δ 7.68–7.63 (m, 2H), 7.28–7.22 (m, 2H), 4.15 (dd, J = 7.8, 5.5 Hz, 1H), 3.72 (t, J = 14.1 Hz, 4H), 3.25 (dd, J = 14.5, 5.6 Hz, 1H), 3.11 (dd, J = 14.5, 7.8 Hz, 1H).
ESI-MS: m/z calculated for C12H1410BF2NO4, [M + H]+ 285.1; found 285.0983.
(2S)-2-Amino-3-(4-(4-(trifluoromethyl)-1,3,2-dioxaborolan-2-yl)phenyl)propanoic acid (3F-BPA).
1H NMR (400 MHz, D2O) δ 7.48–7.42 (m, 2H), 7.12–7.07 (m, 2H), 3.53 (dd, J = 7.8, 5.1 Hz, 1H), 2.97 (dd, J = 13.7, 5.1 Hz, 1H), 2.76 (dd, J = 13.8, 7.8 Hz, 1H).
ESI-MS: m/z calculated for C12H1310BF3NO4, [M + H]+ 303.09; found 303.0888.
(S)-2-Amino-3-(4-(5,5,6,6-tetrafluoro-1,3,2-dioxaborepan-2-yl)phenyl)propanoic acid (4F-BPA).
1H NMR (400 MHz, D2O) δ 7.48–7.42 (m, 2H), 7.12–7.07 (m, 2H), 3.53 (dd, J = 7.8, 5.1 Hz, 1H), 2.97 (dd, J = 13.7, 5.1 Hz, 1H), 2.76 (dd, J = 13.8, 7.8 Hz, 1H).
ESI-MS: m/z calculated for C13H1410BF4NO4, [M + H]+ 335.1; found 335.0947.
2.3. Cell culture
GL261 (mouse glioma cells) cells and B16-F10 (mouse melanoma cells) cells were cultured in RPMI-1640 medium and DMEM containing 10% fetal bovine serum (Gibco) and 1% penicillin–streptomycin in a humidified atmosphere with 5% CO2 at 37 °C, respectively.
2.4.
In vitro cytotoxicity of fluorinated BPA derivatives
The cytotoxicity of fluorinated BPA derivatives was investigated using the CCK-8 kit. The GL261 cells and B16-F10 cells (5 × 103 cells per well) were seeded into 96-well plates and incubated overnight, respectively. The samples (f-BPA, 2F-BPA, 3F-BPA, and 4F-BPA) with different concentrations were added to the cells. After incubating for 24 h, the medium in each well was replaced with 100 μL of fresh medium containing 10 μL of CCK-8 for another 2 h incubation. The absorbance of the samples at 450 nm was detected with a multifunctional enzyme labeler.
2.5. Serum chemistry and hematological analysis
Female C57BL/6J mice aged 5–6 weeks were intravenously injected with f-BPA and fluorinated BPA derivatives of different doses (BPA: 188, 250, and 375 mg kg−1). Blood samples were collected for serum chemistry and hematological analysis at day 3 post-injection.
2.6.
In vitro cellular uptake of fluorinated BPA derivatives
GL261 and B16-F10 cells with a density of 1 × 106 cells per well were seeded into 6-well plates and grown overnight. The fresh medium containing various concentrations of fluorinated BPA derivatives was added into each well. After co-incubation for 3 h and 24 h, the cells were carefully washed three times with PBS solution to remove 10B in the medium. The obtained cells were digested with HNO3 and heated with a microwave-accelerated reaction system. The cellular 10B concentration was measured by ICP-MS after diluting with deionized water.
2.7. Establishment of tumor-bearing mouse models
Female C57BL/6J mice aged 5–6 weeks were purchased from Sipeifu Biotechnology Co., Ltd (Beijing, China), and all animal experiments were approved by the Dongguan People's Hospital Ethics Committee and conducted following the Regulations for the Administration of Affairs Concerning Experimental Animals (P.R. China). GL261 and B16-F10 tumor-bearing mouse models were established by injecting subcutaneously with GL261 cells and B16-F10 cells (2 × 106 cells per mouse) in the right flank, respectively.
2.8.
In vivo biodistribution of fluorinated BPA derivatives
When the tumors reached 50–100 mm3 in volume, the tumor-bearing mice were randomly divided into 4 groups (n = 3): f-BPA, 2F-BPA, 3F-BPA, and 4F-BPA. The tumor-bearing mice were injected intravenously with fluorinated BPA derivatives (BPA: 250 mg kg−1). The tumors, blood, and main organs including the heart, liver, spleen, lungs, and kidneys were collected at 2 h after injection. These organs and tissues were weighed and digested using HNO3, followed by heating with a microwave-accelerated reaction system. The 10B content was detected by ICP-MS after diluting with deionized water.
2.9. Statistical analysis
All experimental data were processed using GraphPad Prism v.8.3. The data are presented as mean ± standard deviation (SD). The significance of group differences was assessed by an ordinary one-way ANOVA test and a two-way ANOVA test with multiple comparisons. A p value of less than 0.05 was considered statistically significant (*p < 0.05, **p < 0.01, ***p < 0.001, and ****p < 0.0001).
3. Results and discussion
3.1. Synthesis and characterization of fluorinated BPA derivatives
Due to the difficulties in the development of 10B delivery agents to fulfill such high requirements for BNCT, BPA is still the primary choice for clinical BNCT and is approved for marketing in Japan. To improve the water solubility and cellular uptake of BPA, we chose diol-containing fluorinated compounds to bind with BPA to form novel fluorinated BPA derivatives. These fluorinated BPA derivatives have the potential to improve the BPA delivery efficiency, including the accumulation in tumors, T/N ratio, and T/B ratio.
The fluorinated BPA derivatives were synthesized as shown in Fig. S1 (ESI†). (S)-2-Amino-3-(4-(5,5-difluoro-1,3,2-dioxaborinan-2-yl)phenyl)propanoic acid (2F-BPA), (2S)-2-amino-3-(4-(4-(trifluoromethyl)-1,3,2-dioxaborolan-2-yl)phenyl)propanoic acid (3F-BPA) and (S)-2-amino-3-(4-(5,5,6,6-tetrafluoro-1,3,2-dioxaborepan-2-yl)phenyl)propanoic acid (4F-BPA) were synthesized using 2,2-difluoropropane-1,3-diol, 2,2-difluoropropane-1,3-diol, and 2,2,3,3-tetrafluorobutane-1,4-diol with BPA·HCl to form boron ester bonds, respectively. In the procedure, the hydrochloride salt of BPA (BPA·HCl) was prepared and DMSO was chosen as the solvent for the reaction due to the poor solubility of BPA in water and organic solvents. After the reaction, the excess BPA was removed by repeated filtration in water. Finally, the pure fluorinated BPA derivatives were obtained after freeze-drying. As shown in Fig. 2 and Fig. S2 (ESI†), we characterized the fluorinated BPA derivatives using 1H NMR and ESI-MS, indicating the successful preparation of fluorinated BPA derivatives. In detail, the integral ratios between the characteristic peaks in the fluorinated BPA derivatives, as depicted in Fig. 2, aligned precisely with the theoretical values. Moreover, compared to BPA (Fig. S3, ESI†), the hydrogen peaks on the benzene ring of the newly synthesized fluorinated BPA derivatives showed significant chemical shifts. In addition, fluorinated BPA derivatives had good water solubility at in vivo injectable doses.
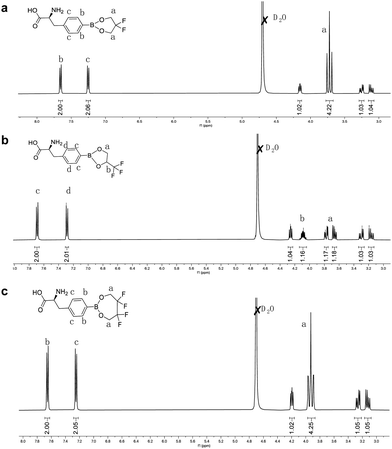 |
| Fig. 2
1H NMR spectra of fluorinated BPA derivatives. The corresponding protons are marked in the structures of (a) 2F-BPA, (b) 3F-BPA, and (c) 4F-BPA. | |
3.2. Biological safety of fluorinated BPA derivatives
Cytotoxicity is an essential indicator for assessing drug biocompatibility.33 We evaluated the cytotoxicity of fluorinated BPA derivatives in GL261 and B16-F10 tumor cells using the cell counting kit-8 (CCK-8) assay. Various concentrations of fluorinated BPA derivatives and f-BPA were added into GL261 and B16-F10 cells and incubated for 24 h. As shown in Fig. 3a–d, the cell viability of GL261 tumor cells with fluorinated BPA derivatives and f-BPA remained over 90%, even up to 50 ppm of the 10B concentration. Similarly, the cell viability of B16-F10 tumor cells also showed a comparable level (Fig. 3e–h). In sum, the CCK-8 assay results suggested that fluorinated BPA derivatives, like f-BPA, exhibited excellent biocompatibility in both B16-F10 and GL261 tumor cells. We also evaluated the biosafety of fluorinated BPA derivatives by cell proliferation. After 72 h incubation of GL261 and B16-F10 cells with f-BPA and fluorinated BPA derivatives, there was no significant change in the cell number in various groups compared to the control group, indicating that fluorinated BPA derivatives are relatively safe (Fig. S4, ESI†).
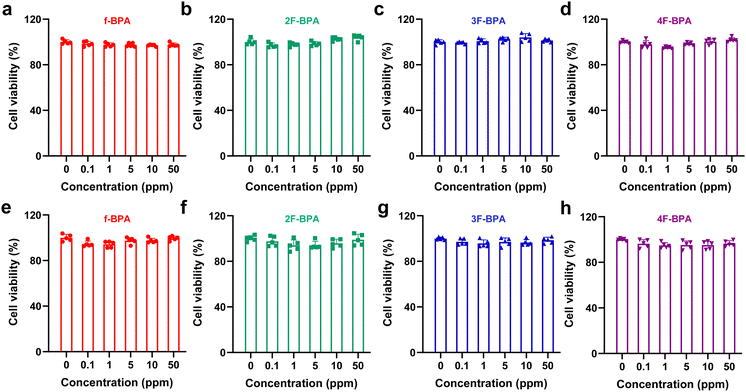 |
| Fig. 3 The cytotoxicity of f-BPA and fluorinated BPA derivatives at different concentrations measured using the CCK-8 assay: (a) f-BPA in GL261 cells, (b) 2F-BPA in GL261 cells, (c) 3F-BPA in GL261 cells, (d) 4F-BPA in GL261 cells, (e) f-BPA in B16-F10 cells, (f) 2F-BPA in B16-F10 cells, (g) 3F-BPA in B16-F10 cells and (h) 4F-BPA in B16-F10 cells. | |
In addition, serum chemistry and hematological analysis are important indicators of biosafety.34 We evaluated serum chemistry and blood indexes at day 3 post-intravenous injection with f-BPA and fluorinated BPA derivatives. As shown in Table 1 and Fig. S5 (ESI†), there were no significant changes in hepatic function, kidney function, myocardial enzymes, and blood indexes between the groups (including the control, f-BPA, 2F-BPA, 3F-BPA, and 4F-BPA) and they were all in the normal ranges. Similarly, there were no obvious changes in the lower and higher dose fluorinated BPA derivative groups (Tables S1, S2 and Fig. S7, S7, ESI†). Furthermore, there was no significant decrease in the body weights of the mice after intravenous injection of fluorinated BPA derivatives (Fig. S8, ESI†). These results indicated that fluorinated BPA derivatives had no significant systemic toxicity with excellent biological safety.
Table 1 Serum chemistry of mice intravenously injected with f-BPA and fluorinated BPA derivatives (BPA: 250 mg kg−1) at day 3 (n = 3)
|
ALT (U L−1) |
AST (U L−1) |
BUN (mg dL−1) |
CREA (μmol L−1) |
UA (μmol L−1) |
CK (U L−1) |
LDH (U L−1) |
Control |
56.00 ± 10.19 |
185.87 ± 18.01 |
23.98 ± 3.60 |
20.68 ± 4.91 |
73.20 ± 1.40 |
1541.47 ± 96.75 |
602.38 ± 32.02 |
f-BPA |
63.47 ± 5.10 |
213.37 ± 28.58 |
21.12 ± 5.18 |
22.50 ± 1.18 |
90.07 ± 22.25 |
1999.33 ± 201.95 |
885.56 ± 139.13 |
2F-BPA |
56.06 ± 17.92 |
173.27 ± 32.98 |
29.20 ± 3.04 |
18.67 ± 1.74 |
73.28 ± 16.11 |
1470.12 ± 394.20 |
720.95 ± 74.08 |
3F-BPA |
46.52 ± 3.92 |
100.11 ± 17.92 |
26.63 ± 5.98 |
18.73 ± 7.57 |
99.65 ± 38.44 |
1318.10 ± 149.96 |
690.32 ± 133.10 |
4F-BPA |
48.94 ± 13.94 |
172.50 ± 41.65 |
21.00 ± 3.63 |
20.30 ± 2.50 |
63.69 ± 10.24 |
1580.17 ± 195.08 |
645.73 ± 63.95 |
We further evaluated the biosafety of fluorinated BPA derivatives by the hematoxylin and eosin (H&E) staining analysis. After the intravenous injection for 3 days, the major organs including the heart, liver, spleen, lungs, and kidneys were collected and sectioned. All groups showed no significant systemic toxicity to the major organs, implying that fluorinated BPA derivatives had good biosafety (Fig. S9, ESI†). Moreover, we also estimated the change of boron concentration in the blood of mice over time. As shown in Fig. S10 (ESI†), the boron concentration in the blood gradually decreased with time, indicating that the fluorinated BPA derivatives could be gradually metabolized.
3.3.
In vitro cellular uptake of fluorinated BPA derivatives to enhance 10B content
Sufficient 10B contents (>16.67 ng 10B per 106 cells) in tumor cells are vital for successful BNCT. To assess whether fluorinated BPA derivatives were effectively taken up into tumor cells, different concentrations of fluorinated BPA derivatives (1 ppm, 5 ppm, and 10 ppm) were co-incubated with GL261 and B16-F10 tumor cells, with f-BPA as the control. The 10B concentrations in tumor cells were detected by inductively coupled plasma mass spectroscopy (ICP-MS). As shown in Fig. 4a–d, the 10B contents in GL261 tumor cells gradually increased with concentration escalation of f-BPA and fluorinated BPA derivatives. The cellular uptake of f-BPA and fluorinated BPA derivatives also showed similar increasing trends in B16-F10 tumor cells (Fig. 4e–h). This result indicated a concentration-dependent uptake of 10B in GL261 and B16-F10 tumor cells. In addition, the detection of 10B by ICP-MS showed that f-BPA and fluorinated BPA derivatives could be rapidly taken up into tumor cells at 3 h and there was a significant increase at 24 h post-incubation, both in GL261 tumor cells (Fig. S11, ESI†) and in B16-F10 tumor cells (Fig. S12, ESI†).
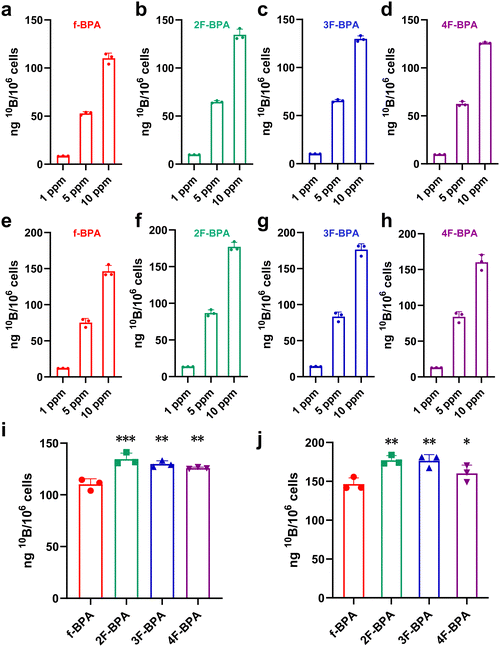 |
| Fig. 4
In vitro cellular uptake of f-BPA and fluorinated BPA derivatives. The 10B concentration in GL261 cells after 24 h incubation with various concentrations of (a) f-BPA, (b) 2F-BPA, (c) 3F-BPA and (d) 4F-BPA. The 10B concentration in B16-F10 cells after 24 h incubation with various concentrations of (e) f-BPA, (f) 2F-BPA, (g) 3F-BPA and (h) 4F-BPA. (i) The 10B concentration in GL261 cells after 24 h incubation with f-BPA, 2F-BPA, 3F-BPA and 4F-BPA at the same 10B dose (10 ppm). (j) The 10B concentration in B16-F10 cells after 24 h incubation with f-BPA, 2F-BPA, 3F-BPA and 4F-BPA at the same 10B dose (10 ppm) (*p < 0.05, **p < 0.01, ***p < 0.001 compared with f-BPA). | |
In addition, we compared the cellular uptake of f-BPA and fluorinated BPA derivatives in GL261 tumor cells for 24 h at a 10B concentration of 10 ppm. As shown in Fig. 4i, the average values of 10B per 106 cells reached 110.13 ± 5.46 ng for f-BPA, 134.51 ± 5.81 ng for 2F-BPA, 129.83 ± 3.05 ng for 3F-BPA and 125.87 ± 0.89 ng for 4F-BPA, and the value exceeded the required 10B content (16.67 ng per 106 cells) for BNCT. The concentration of 10B in tumor cells with fluorinated BPA derivatives (including 2F-BPA, 3F-BPA, and 4F-BPA) was higher than that in tumor cells with f-BPA. In B16-F10 tumor cells, the mean 10B content per 106 cells of f-BPA (146.44 ± 8.02 ng), 2F-BPA (177.06 ± 6.04 ng), 3F-BPA (176.43 ± 7.99 ng), and 4F-BPA (160.19 ± 10.80 ng) was also more than the required amount of 10B for BNCT, and the cellular uptake of 10B for fluorinated BPA derivatives was higher than that for f-BPA (Fig. 4j). Moreover, the cellular uptake of 10B was higher in B16-F10 tumor cells than that in GL261 tumor cells. These results suggested that fluorinated BPA derivatives have a more pronounced advantage over f-BPA for 10B delivery in GL261 and B16-F10 tumor cells. Therefore, fluorinated BPA derivatives may be better candidates to deliver sufficient 10B into tumor cells.
3.4.
In vivo biodistribution of fluorinated BPA derivatives to enhance the 10B accumulation in tumors
The key to efficient BNCT is the accumulation of sufficient 10B content (>20 μg 10B per g tumor) in the tumor and high T/B and T/N ratios during neutron irradiation. To verify fluorinated BPA derivatives as potential boron delivery agents, we investigated whether fluorinated BPA derivatives could deliver sufficient 10B to the tumor site and enhance the T/B and T/N ratios by intravenous injection of fluorinated BPA derivatives, with f-BPA as the control. The injection dose of 10B was 250 mg kg−1 of BPA (equivalent to half of the clinical dose). The accumulation of 10B in the tumor, blood, and various organs was measured by ICP-MS.
The in vivo distribution of f-BPA and fluorinated BPA derivatives in GL261 tumor-bearing mice was assayed at 2 h after intravenous injection (Fig. 5a), and the 10B content of individual organs and blood is demonstrated in Fig. S13 (ESI†). As shown in Fig. 5b, the 10B uptake in tumors reached 9.56 ± 0.30 ng per g tumor for 2F-BPA, 9.36 ± 0.43 ng per g tumor for 3F-BPA, and 9.27 ± 1.06 ng per g tumor for 4F-BPA. Although the 10B content in the tumor did not reach the BNCT-requirements, fluorinated BPA derivatives still improved the 10B accumulation in the tumor compared with f-BPA (5.12 ± 1.13 ng per g tumor). Surprisingly, the T/B ratio of fluorinated BPA derivatives (2F-BPA: 1.66 ± 0.35, 3F-BPA: 1.72 ± 0.21, 4F-BPA: 1.54 ± 0.10) was significantly higher than that of f-BPA (0.97 ± 0.20) (Fig. 5c). T/N is also a key to the success of BNCT. As shown in Fig. 5d–g, the T/N ratios of fluorinated BPA derivatives were significantly higher than those of f-BPA, indicating that fluorinated BPA derivatives selectively targeted tumor cells and reduced damage to surrounding normal tissues relative to f-BPA. Therefore, fluorinated BPA derivatives may become more advantageous boron delivery agents than f-BPA.
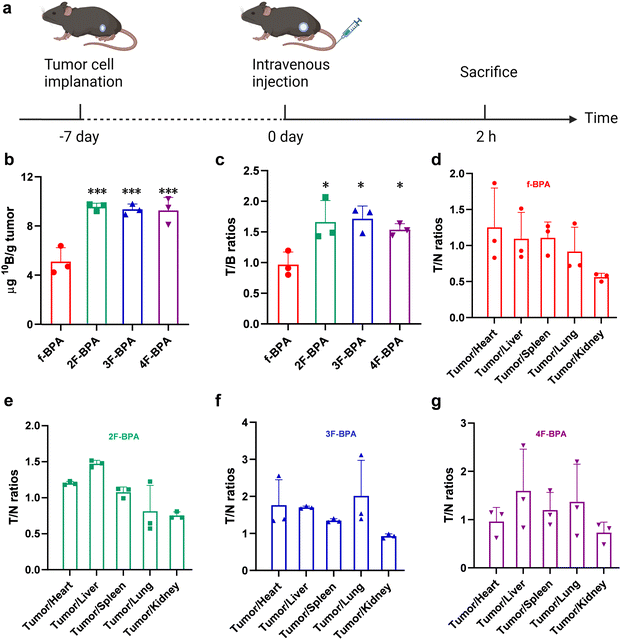 |
| Fig. 5
In vivo biodistribution of f-BPA and fluorinated BPA derivatives at 2 h after intravenous injection for GL261 tumor-bearing mice. (a) Scheme of the experimental timeline. (b) The 10B contents in tumors measured by ICP-MS for f-BPA, 2F-BPA, 3F-BPA and 4F-BPA. (c) Tumor-to-blood (T/B) ratios of f-BPA, 2F-BPA, 3F-BPA and 4F-BPA. Tumor-to-normal tissue (T/N) ratios (including the heart, liver, spleen, lungs, and kidneys) of (d) f-BPA, (e) 2F-BPA, (f) 3F-BPA and (g) 4F-BPA (ns > 0.05, *p < 0.05, **p< 0.01, ***p < 0.001 compared with f-BPA). | |
In addition, the B16-F10 tumor-bearing mouse model was also used to study the in vivo distribution of fluorinated BPA derivatives. As shown in Fig. 6a, the 10B content in tumors reached 39.78 ± 4.52 ng per g tumor for 2F-BPA, 40.20 ± 2.83 ng per g tumor for 3F-BPA, and 38.42 ± 3.28 ng per g tumor for 4F-BPA. In contrast, the 10B uptake in the surrounding normal tissues (including the heart, liver, spleen, lungs, and kidneys) and blood was relatively low (Fig. S14, ESI†). Moreover, the 10B content in the tumors of fluorinated BPA derivatives was greater than that of f-BPA (28.48 ± 2.66 ng per g tumor), and there was a statistically significant difference. As shown in Fig. 6b, the T/B ratios of 3F-BPA and 4F-BPA reached 4.72 ± 0.11 and 4.76 ± 0.05, respectively, which were significantly higher than that of f-BPA (3.95 ± 0.45), suggesting that 3F-BPA and 4F-BPA are more responsive to the needs of BNCT. In addition, the T/N ratios of fluorinated BPA derivatives were also almost always greater than 3, except for the tumor-to-kidney ratios (approximately 2), which also met the requirements of clinical BNCT (Fig. 6c–f). And the T/N ratios of fluorinated BPA derivatives were higher than those of f-BPA, indicating that fluorinated BPA could better target tumor cells and decrease the harm to surrounding normal tissues.
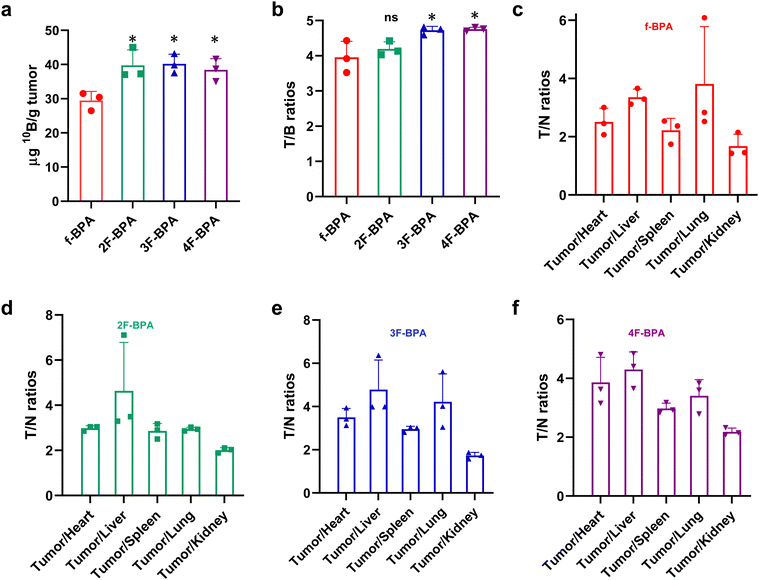 |
| Fig. 6
In vivo biodistribution of f-BPA and fluorinated BPA derivatives at 2 h after intravenous injection for B16-F10 tumor-bearing mice. (a) The 10B contents in tumors measured by ICP-MS for f-BPA, 2F-BPA, 3F-BPA and 4F-BPA. (b) Tumor-to-blood (T/B) ratios of f-BPA, 2F-BPA, 3F-BPA and 4F-BPA. Tumor-to-normal tissue (T/N) ratios (including the heart, liver, spleen, lungs, and kidneys) of (c) f-BPA, (d) 2F-BPA, (e) 3F-BPA and (f) 4F-BPA (ns > 0.05, *p < 0.05, **p < 0.01, ***p < 0.001 compared with f-BPA). | |
The above experimental results showed that fluorinated BPA derivatives had higher 10B accumulation in tumors and enhanced T/B and T/N ratios compared with f-BPA, which could reduce the accumulation of 10B in other organs and blood, minimizing the damage to the surrounding normal tissues. Notably, the 10B uptake in the tumor of fluorinated BPA derivatives in the B16-10 tumor-bearing mouse model was greater than 20 μg g−1 tumor, and the T/B and T/N ratios were greater than 3, showing a better delivery profile compared with the GL261 tumor-bearing mouse model, consistent with the cellular uptake results. Therefore, fluorinated BPA derivatives were more potential boron delivery agents for BNCT of B16-F10 tumors.
4. Conclusions
In summary, we developed fluorinated BPA derivatives with different fluorine groups as boron delivery agents, and the successful preparation was confirmed by 1H NMR and ESI-MS results. Besides, the CCK-8 assay results suggested that fluorinated BPA derivatives had no significant cytotoxicity even at a high 10B concentration of 50 ppm. Serum chemistry and hematological analysis indicated that fluorinated BPA derivatives had no significant systemic toxicity with excellent biological safety. In vitro, fluorinated BPA derivatives showed significantly enhanced uptake in GL261 and B16-F10 tumor cells relative to f-BPA. Furthermore, in GL261 tumor-bearing mice, although the 10B accumulation in tumors for fluorinated BPA derivatives did not meet the requirements of BNCT, the 10B accumulation in tumors, T/B ratio, and T/N ratio were significantly improved compared with f-BPA. Remarkably, the 10B uptake in B16-F10 tumors for fluorinated BPA derivatives was higher than that for f-BPA and was much higher than the 10B content required for BNCT. Compared with f-BPA, fluorinated BPA derivatives also significantly enhanced the T/B and T/N ratios, thus better targeting the tumor tissues and causing less damage to surrounding normal tissues. Therefore, fluorinated BPA derivatives would be promising boron delivery agents for clinical BNCT of tumors, especially melanoma.
Author contributions
Dandan Ding, Xueyi Wang, and Zhenhua Li designed and discussed the experiments; Dandan Ding carried out most experiments, with analytical/experimental contributions from Shushan Mo, Qishan Li, Fei Wang, Xueyi Wang, Caiwen Ou and Zhenhua Li. The manuscript draft was written by Dandan Ding with the review and editing done by Caiwen Ou and Zhenhua Li. All authors have given approval to the final version of the manuscript.
Conflicts of interest
There are no conflicts to declare.
Acknowledgements
This work was supported by the National Natural Science Foundation of China (82202307, 82202339 and 32301162), the Guangdong Basic and Applied Basic Research Foundation (2021B1515120065), and the China Postdoctoral Science Foundation (2021M701640 and 2022M711527).
References
- R. F. Barth, J. A. Coderre, M. G. H. Vicente and T. E. Blue, Clin. Cancer Res., 2005, 11, 3987–4002 CrossRef CAS PubMed
.
- R. F. Barth, A. H. Soloway and R. G. Fairchild, Sci. Am., 1990, 263, 100–107 CrossRef CAS PubMed
.
- M. A. Dymova, S. Y. Taskaev, V. A. Richter and E. V. Kuligina, Cancer Commun., 2020, 40, 406–421 CrossRef PubMed
.
- Y. Shi, Z. Guo, Q. Fu, X. Shen, Z. Zhang, W. Sun, J. Wang, J. Sun, Z. Zhang, T. Liu, Z. Gu and Z. Liu, Nat. Commun., 2023, 14, 1884 CrossRef CAS PubMed
.
- R. L. Moss, Appl. Radiat. Isot., 2014, 88, 2–11 CrossRef CAS PubMed
.
- A. H. Soloway, W. Tjarks, B. A. Barnum, F.-G. Rong, R. F. Barth, I. M. Codogni and J. G. Wilson, Chem. Rev., 1998, 98, 1515–1562 CrossRef CAS PubMed
.
- H. Fukuda, Cells, 2021, 10, 2881 CrossRef CAS PubMed
.
- P. Chang, D. Chow, L. Poisson, R. Jain and C. Filippi, J. Neurol. Sci., 2017, 381, 172–173 CrossRef
.
- R. F. Barth, M. G. H. Vicente, O. K. Harling, W. S. Kiger III, K. J. Riley, P. J. Binns, F. M. Wagner, M. Suzuki, T. Aihara, I. Kato and S. Kawabata, Radiat. Oncol., 2012, 7, 3–21 CrossRef PubMed
.
- Y. Mishima, C. Honda, M. Ichihashi, H. Obara, J. Hiratsuka, H. Fukuda, H. Karashima, T. Kobayashi, K. Kanda and K. Yoshino, Lancet, 1989, 334, 388–389 CrossRef PubMed
.
- M. Suzuki, I. Kato, T. Aihara, J. Hiratsuka, K. Yoshimura, M. Niimi, Y. Kimura, Y. Ariyoshi, S. i Haginomori, Y. Sakurai, Y. Kinashi, S. i Masunaga, M. Fukushima, K. Ono and A. Maruhashi, J. Radiat. Res., 2013, 55, 146–153 CrossRef PubMed
.
- J. Li, Q. Sun, C. Lu, H. Xiao, Z. Guo, D. Duan, Z. Zhang, T. Liu and Z. Liu, Nat. Commun., 2022, 13, 2143 CrossRef CAS PubMed
.
- M. Lamba, A. Goswami and A. Bandyopadhyay, Chem. Commun., 2021, 57, 827–839 RSC
.
- R. F. Barth, Z. Zhang and T. Liu, Cancer Commun., 2018, 38, 1–7 Search PubMed
.
- J. Li, X. Wang, Z. Wang, Y. Zhao, Z. Zhang, L. Li, D. Ding, J. Guo, J. Zhang, H. Liu and Z. Li, Biomater. Sci., 2023, 11, 7568–7578 RSC
.
- L. Li, J. Li, Y. Shi, P. Du, Z. Zhang, T. Liu, R. Zhang and Z. Liu, ACS Nano, 2019, 13, 13843–13852 CrossRef CAS PubMed
.
- J. Li, J. Kong, S. Ma, J. Li, M. Mao, K. Chen, Z. Chen, J. Zhang, Y. Chang, H. Yuan, T. Liu, Z. Zhang and G. Xing, Adv. Funct. Mater., 2021, 31, 2100969 CrossRef CAS
.
- N. Kuthala, R. Vankayala, Y. N. Li, C. S. Chiang and K. C. Hwang, Adv. Mater., 2017, 29, 1700850 CrossRef PubMed
.
- I. V. J. Feiner, K. R. Pulagam, K. B. Uribe, R. Passannante, C. Simó, K. Zamacola, V. Gómez-Vallejo, N. Herrero-Álvarez, U. Cossío, Z. Baz, M. M. Caffarel, C. H. Lawrie, D. J. Vugts, L. Rejc and J. Llop, J. Mater. Chem. B, 2021, 9, 410–420 RSC
.
- A. Wittig, W. A. Sauerwein and J. A. Coderre, Radiat. Res., 2000, 153, 173–180 CrossRef CAS PubMed
.
- P. Wongthai, K. Hagiwara, Y. Miyoshi, P. Wiriyasermkul, L. Wei, R. Ohgaki, I. Kato, K. Hamase, S. Nagamori and Y. Kanai, Cancer Sci., 2015, 106, 279–286 CrossRef CAS PubMed
.
- Y. Mori, A. Suzuki, K. Yoshino and H. Kakihana, Pigm. Cell Res., 1989, 2, 273–277 CrossRef CAS PubMed
.
- H. Fukuda, C. Honda, N. Wadabayashi, T. Kobayashi, K. Yoshino, J. Hiratsuka, J. Takahashi, T. Akaizawa, Y. Abe, M. Ichihashi and Y. Mishima, Melanoma Res., 1999, 9, 75–84 CrossRef CAS PubMed
.
- T. Nomoto, Y. Inoue, Y. Yao, M. Suzuki, K. Kanamori, H. Takemoto, M. Matsui, K. Tomoda and N. Nishiyama, Sci. Adv., 2020, 6, eaaz1722 CrossRef CAS PubMed
.
- C. Zhang, K. Yan, C. Fu, H. Peng, C. J. Hawker and A. K. Whittaker, Chem. Rev., 2021, 122, 167–208 CrossRef PubMed
.
- S. Ellipilli and K. N. Ganesh, J. Org. Chem., 2015, 80, 9185–9191 CrossRef CAS PubMed
.
- C. Zhang, T. Liu, W. Wang, C. A. Bell, Y. Han, C. Fu, H. Peng, X. Tan, P. Král, K. Gaus, J. J. Gooding and A. K. Whittaker, ACS Nano, 2020, 14, 7425–7434 CrossRef CAS PubMed
.
- G. Rong, C. Wang, J. Hu, Y. Li and Y. Cheng, J. Controlled Release, 2022, 351, 703–712 CrossRef CAS PubMed
.
- F. Menaa, B. Menaa and O. N. Sharts, J. Mol. Pharm. Org. Process Res., 2013, 1, 2 Search PubMed
.
- M. Wang, H. Liu, L. Li and Y. Cheng, Nat. Commun., 2014, 5, 3053 CrossRef PubMed
.
- L. Dafik, V. Kalsani, A. K. L. Leung and K. Kumar, J. Am. Chem. Soc., 2009, 131, 12091–12093 CrossRef CAS PubMed
.
- V. Metelev, S. Zhang, S. Zheng, A. T. N. Kumar and A. Bogdanov, Theranostics, 2017, 7, 3354–3368 CrossRef CAS PubMed
.
- H. Huang, C. Dong, M. Chang, L. Ding, L. Chen, W. Feng and Y. Chen, Exploration, 2021, 1, 50–60 CrossRef PubMed
.
- Y. Liu, W. Wang, D. Zhang, Y. Sun, F. Li, M. Zheng, D. B. Lovejoy, Y. Zou and B. Shi, Exploration, 2022, 2, 20210274 CrossRef CAS PubMed
.
|
This journal is © The Royal Society of Chemistry 2024 |