DOI:
10.1039/D3MA01132A
(Review Article)
Mater. Adv., 2024,
5, 4634-4659
Recent advances in engineering prodrug-based nanomedicines for cancer therapy
Received
16th December 2023
, Accepted 24th April 2024
First published on 8th May 2024
Abstract
Despite substantial progress, nanomedicines still face numerous obstacles in cancer therapy. The combination of prodrugs and nanoparticle drug delivery systems has cooperatively led to unprecedented levels of control over the pharmacological properties of antitumor drugs, which gained significant attention a decade ago and is believed to propose pioneering clinical outcomes. Manipulating the matter within the nanoscale, prodrug-based nanomedicines are unified in classification and categorized by promoiety size and nanoconstruction formulation in the review. In addition, we focus on the updates of a broad spectrum of fabrication advances and applications in the field of prodrug-based nanotechnologies in multiple cancer therapeutic strategies in response to the tumor microenvironment, with special emphasis on chemotherapy and immunotherapy. Finally, the prospects and the potential threats of prodrug-based nanoassemblies toward the market and clinical uses are highlighted.
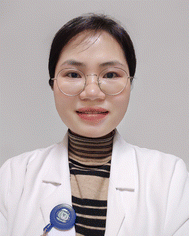 Linlin Shi | Dr. Linlin Shi obtained her PhD from Zhejiang University, China in 2021. She currently is a physician working at Department of Hematology, Sir Run Run Shaw Hospital, China. She has broad research interests in the areas of medical nanotechnology, drug delivery system, and cancer targeted theranostics. She has published papers in well-reputed SCI journals, including Signal Transduction and Targeted Therapy, Journal of Controlled Release, ACS Applied Materials & Interfaces, Theranostics and Nanoscale Advances with a total citation of 400+. |
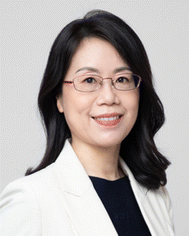 Jin Zhang | Prof. Jin Zhang is the executive director of Department of Hematology, Sir Run Run Shaw Hospital, China. She is the deputy leader and the member of several important professional committees of Hematology in China. She has worked on several academic research projects at the School of Medicine, Zhejiang University. Her research interests include the pathogenesis and advanced therapy of leukemia and lymphoma, the targeted drug delivery of hematologic malignancies, and the CAR-T therapy of lymphoma and myeloma. She has authored/co-authored 10+ research and review papers in well-reputed SCI journals, including Blood, Oncology Reports and Frontiers in Oncology. |
1. Introduction
The field of anticancer drug delivery has seen a growing interest in the use of drug nanocarriers to improve therapeutic efficacy and to reduce the risk of adverse reactions due to the inherent toxicity of these molecules. As concluded by Shen et al., the successful delivery of nanomedicine involves five steps, including blood circulation, tumor accumulation, tumor penetration, cellular internalization, and drug release (also called the CAPIR cascade).1 Each step exhibits distinct biological barriers, and the inability of conventional nanomedicines to efficiently circumvent all of these barriers results in their unsatisfactory therapeutic outcomes. The physicochemical features of nanomedicines including size, shape, surface charge and composition all play essential roles in each step and determine in vivo interaction with biomolecules, especially with proteins.2,3 The proteins adsorbed onto the nanoparticle surface (also called, protein corona) were found in a recent study to perturb proteostasis and disrupt the cellular metabolism pathway, including glycolysis and lipid metabolism in cancer cells.4 As a result, thorough comprehension of the biological effects and the structure–function relationship of nanomaterials is crucial for designing effective and safe nanoplatforms with optimal physicochemical properties and tumor-oriented capabilities.5–7
Ideally, the delivery of drugs loaded into nanodevices should:8–10 (i) protect the drug from degradation/metabolization, (ii) deliver the pharmacologically active molecule to the target tissue or cell, (iii) reach the specific subcellular organelles (e.g., cytoplasm, lysosomes, mitochondria, and nucleus), (iv) control drug release with the help of exogenous (i.e., temperature, magnetic field, and light) or endogenous (i.e., pH, enzymes, and redox) stimuli, and (v) even combine a therapeutic functionality with an imaging capability (the so-called “nanotheranostic” approach). However, the direct physical encapsulation of antitumor drugs into nanocarriers during nanoformation often faces serious challenges such as the burst release of encapsulated drug before reaching tumors upon systemic administration. The overuse of organic co-solvents during the formulation process to stabilize the poorly-soluble drugs leads to low activity and severe side effects. For all these reasons, developing nanoplatforms that accomplish improved physicochemical properties, prolonged systemic circulation, controlled drug release, and lessened drug toxicity is critically required.11,12
As an alternative strategy, the prodrug-based nanomedicine approach has found wide application to alleviate or even suppress the aforementioned limitations of nanomedicine.13 A prodrug is formed by the covalent linkage between a drug and a (macro)molecule that is further metabolized (e.g., hydrolytic degradation) to release the active form in vivo.14,15 The covalent modification of a drug molecule into an inactive precursor from which the drug will be subsequently freed after administration offers several benefits, including:16 (i) sustained and tumor microenvironment responsive drug release, (ii) reduced toxicity before the metabolization occurs, (iii) increased drug chemical stability and solubility, and (iv) facile encapsulation by nanocarriers or self-assembly into nanoparticles (NPs). To date, plenty of prodrug strategies, such as antibody–drug conjugates (ADCs) that target the over-expressed cancer-specific antigens, account for momentous positions in oncotherapy.16,17 Researchers including Satpathy et al. demonstrated that Her-2 targeted ADC self-assembled nanoparticles presented long-time retention in tumor tissues, which induced a strong therapeutic response in breast and ovarian tumor models.18,19 Thus, the combination of a prodrug strategy and nanotechnology is supposed to take advantage of the merits of both strategies and to achieve a potent synergetic effect for cancer therapy.
The prodrug nanocarriers can be roughly divided into organic and inorganic delivery systems.19 However, the classification of prodrug-based nanodrug delivery systems (nano-DDS) hasn’t been further reasonably unified for cancer therapy. Luo et al. first categorized prodrug nano-DDS into polymer–drug conjugates, self-assembling small molecular weight prodrugs and prodrug-encapsulated nanoparticles for cancer chemotherapy in 2014.9 Meng et al. classified that solely by the structure and the constitution of prodrugs in 2019.14 Zhang et al. further introduced prodrug-based nano-DDS using small molecule based, polymer–drug conjugate based and biomacromolecule-drug conjugate based prodrug nanoformulations in 2021.20 In this review, we unified the classification and categorized the prodrug platforms according to the promoiety size and nanoconstruction formulation. Prodrug-based nanomedicines can be divided into small molecular prodrug (SMP)-based or macromolecular prodrug (also described as polymer prodrug, PP)-based Nano-DDS according to the molecular weight of the prodrug.14,21 The SMP-based Nano-DDS with promoieties such as lipid fatty acids and cholesterol derivatives have been extensively used, which are further discussed in this review. Furthermore, according to the formulation and the construction approach, the prodrug-based Nano-DDS could be distinguished as self-assembled prodrug nanosystems and prodrug-encapsulated NPs.22,23
The prodrug-based system has become a notable trend in recent years to facilitate more efficient delivery of anticancer drugs and has been extensively explored in various cancer therapies. Our previous study demonstrated that reconstructing chemotherapeutics cabazitaxel (CTX) and 7-ethyl-10-hydroxy camptothecin (SN38) with a polyunsaturated fatty acid (i.e., docosahexaenoic acid, DHA) and cholesterol via hydrolyzable ester bonds confers the generated prodrugs with sustained drug release profiles and improved pharmacokinetics for systemic chemotherapy in vivo.24,25 The targeted drug delivery systems based on prodrug nanocarriers for cancer chemotherapy were also summarized in several published studies.23,26,27 For example, Sun et al. recently introduced the latest updates of taxane prodrug-based nanomedicines (TPNS) and discussed the new trends of tumor stimuli-responsive TPNS.26 In addition, prodrug-based nanomedicines have also been substantially exploited for cancer immune and combination therapy, which were also discussed in several review papers.14,28,29 For instance, Yang et al. introduced prodrug nanomedicines as a promising strategy to improve cancer immunotherapy.28
In this review, we summarize the current emerging research studies and applications of prodrug nanomedicines as efficient strategies to improve multiple common cancer therapies, including chemotherapy, photodynamic therapy (PDT), photothermal therapy (PTT), molecular-targeting therapy, immunotherapy, and combination therapy. We are the first to summarize the self-assembled nanomedicines based on photosensitizer (PS) or photothermal agent (PTA) prodrugs for PDT and PTT. Specifically, the applications of prodrug nanomedicines in cancer immunotherapy are introduced by the mechanism of action in this review, which can probably inspire the design of prodrug nanoparticles that can specifically target the corresponding tumor immuno-microenvironment. We also discuss the principles, challenges, clinical and commercial perspectives related to the development of nanomedicine-based prodrug approaches toward cancer therapy.
2. Classification of prodrug-based nanomedicines
Prodrug-based Nano-DDS consist of SMP- or PP-based Nano-DDS on the basis of their molecular weights (Fig. 1). Both SMPs or PPs can self-assemble into NPs or be encapsulated in nanocarriers (organic or inorganic carrier) by noncovalent interactions (e.g., hydrophobic forces and electrostatic interactions). The nanosystems based on those two prodrugs are reviewed in the following section.
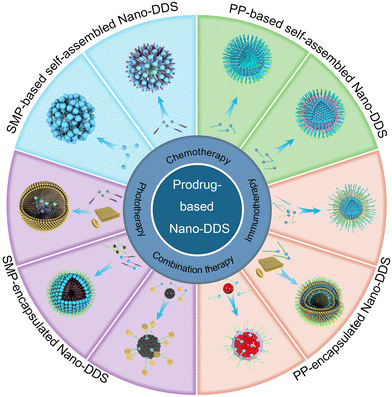 |
| Fig. 1 Schematic of the formulation of prodrug-based nanoparticulate drug delivery systems (Nano-DDS) for cancer therapy. SMP: small molecular prodrug; PP: polymer prodrug. | |
2.1 SMP-based Nano-DDS
SMP-based Nano-DDS are formed by self-assembly of the SMP or loading of nanocarriers (e.g., liposomes or micelles) with SMPs. The SMP-based nanostrategy utilizes properties of the conjugates, such as high solubility and pharmacokinetics properties, that are more suitable for biological applications than those of a single drug molecule.13
The self-assembly of SMPs can be divided into the nanoassembly of homodimer/oligomer and heterodimers/oligomer according to their chemical structure.30 Researches on the self-assembly of homodimeric chemotherapeutics into NPs for cancer therapy are summarized in Table 1. Mostly focusing on the construction of homodimeric taxanes or camptothecin (CPT), those studies reported self-assembled SMP nanoplatforms with high drug loading and tumor responsive drug release for cancer chemotherapy. Small changes in the length or the adjustment of linkages exert great influences on the antitumor activity of homodimeric prodrug nanoassemblies. The generated dimeric anticancer drug ligand via a ROS activatable thioketal linkage is often coassembled with photosensitizers to form colloidal-stable nanoassemblies and to achieve synergistic chemo-photodynamic therapy.31–33
Table 1 Research on the self-assembly of homodimeric chemotherapeutics into NPs for cancer therapy
Chemotherapeutics |
Drug |
Structure |
Findings |
Ref. |
Antimitotic agents |
Paclitaxel (PTX) |
|
Glu-PTX2 NPs could be internalized by cancer cells and exert potent cytotoxicity toward HeLa and MCF-7 cells. |
39
|
|
Among three dimers, the PTX2-Te dimer exhibits preferable cytotoxicity on HeLa cells, which is comparable to Taxol. |
40
|
|
PTX-SS-PTX dimer formed nanoparticles exhibited the most efficient endocytosis and cytotoxicity on HeLa cells and B16F10 cells. |
41
|
|
Nanoparticles coassembled from dimeric PTX prodrugs and porphyrin achieved synergistic chemo-photodynamic therapy. |
31
|
Cabazitaxel (CTX) |
|
Self-assembled dimeric CTX preserves inherent pharmacologic efficacy while reducing in vivo systemic and immune toxicity. |
42 and 43
|
|
Driven by a photosensitizer, PPa, CTX-S-CTX self-assembled into nanoparticles and demonstrated potent antitumor activity in a 4T1 mice model. |
44
|
|
Coassembly with a photosensitizer, chlorin e6 (Ce6), CTX-TK-CTX self-assembled into nanoassemblies and produced durable tumor regression and low systemic toxicity in an orthotopic patient-derived xenograft (PDX) model of malignant melanoma. |
32
|
Docetaxel (DTX) |
|
The longest diselenide bond-containing linkage facilitated the self-delivery of DTX prodrugs and achieved the maximum accumulation of DTX in tumor cells. |
45
|
|
DTX-STeS-DTX NPs resulted in the improved antitumor efficacy of DTX with satisfactory safety on the 4T1 tumor mice model. |
46
|
Topoisomerase inhibitors |
7-Ethyl-10-hydroxy camptothecin (SN38) |
|
SN38 dimer self-assembled nanoparticles possess potent cytotoxic activity. |
47
|
Camptothecin (CPT) |
|
CPT-dimer self-assembled nanoparticles could be internalized by cancer cells and exert potent cytotoxicity on HeLa and HepG2 cells. |
48
|
DNA intercalators |
Doxorubicin (DOX) |
|
Trisulfide bond-bridged prodrug nanoassemblies exhibit high selective cytotoxicity on tumor cells, notably reducing the systemic toxicity of DOX. |
49
|
Cyclin dependent kinase inhibitor |
Palbociclib (Palb) |
|
The carrier-free nanomedicine self-assembled from Palb-TK-Palb and Ce6 showed high cellular uptake and in vitro antitumor activity on MDA-MB-231 cells. |
33
|
NF-κB inhibitor |
Dihydroar-temisinin (DHA) |
|
Ce6&DHA-S-DHA@CMN NPs enabled higher tumor accumulation, inhibited tumor metastasis, and achieved favorable chemo-photodynamic combination therapy. |
50
|
Insulin-like growth factor-1 receptor |
Podophyllo-toxin (POD) |
|
Incorporating VK3 and F127 into POD dimer self-assembled nanoparticles increased the therapeutic efficacy in MCF-7 tumor-bearing mice. |
51
|
For heterodimer/oligomer formulation, the side chain conjugated to the therapeutic drug can be hydrophilic (e.g., lactose, folic acid, or a peptide) or hydrophobic (e.g., a lipid or fatty acid). The self-assembly of SMPs into nanostructures often produces considerably high drug-loading efficiency and avoids issues encountered in nanocarrier binding and excipient-trigged adverse reactions, making SMP-based Nano-DDS a promising platform for anticancer drug delivery.34
In addition, the simple and reproducible one-step nanoprecipitation preparation methods used for SMP-based NPs are beneficial for scaling-up to industrial production and clinical translation.35 However, the short blood circulation time caused by the lack of a dense shield layer (e.g., a long chain polyethylene glycol [PEG]) and poor structural stability due to a relatively higher critical micelle concentration significantly hamper their drug potency, safety, and eventually hinder their clinical application.23 Therefore, nanosystems self-assembled from SMPs are usually stabilized with a small amount of amphiphilic block copolymer containing a long PEG chain to achieve both a higher drug-loading efficiency and structural stability.36,37 For example, a paclitaxel (PTX)-based self-assembled prodrug was developed by conjugating oleic acid to paclitaxel, and then a small amount of amphiphilic block copolymer tocopheryl PEG2k succinate was selected as a surface active agent.38 Impressively, PEGylated nanoassemblies exhibited higher stability compared with that of PTX self-assembled naked NPs. These PEGylated NPs achieved significantly high drug loading (56% of PTX, w/w), and the anticancer activity of this hybrid nanocarrier in a human epidermoid carcinoma murine model was significantly higher than that of the conventional clinical formulation (Taxol).
SMP entrapment into nanocarriers (e.g., liposomes, solid lipids, polymeric NPs, and cell membranes) for tumor delivery is another strategy to achieve valid therapy with increased SMP stability, improved bioavailability, enhanced targeting to tumor cells, and increased capacity for encapsulation efficiency of both lipophilic and hydrophilic prodrugs.52–54 The NPs used for SMP loading can be divided into organic and inorganic delivery systems. Organic nanocarriers, including polymer NPs, micelles, liposomes, dendrimers, and nanoemulsions have been studied for drug delivery,55,56 while inorganic NPs, including silica, gold, silver, iron, and quantum dots, have favorable properties for extensive diagnostic and therapeutic use.57–59 However, the main disadvantage of this entrapment strategy is related to the relatively low drug loading caused by using a high proportion of carrier materials during preparation.
2.2 PP-based Nano-DDS
Unlike SMPs, PPs refer to the conjugates where the carrier materials usually have a macromolecular weight and occupy a large portion of the nanostructure, and the polymers themselves play an important role in adjusting the hydrophilic–lipophilic balance of the conjugates.60 The most widely used polymers in formulating PPs include PEG block copolymers (BCPs), polypeptides, polyaminoacids, and polysaccharides.61–64 Each type of polymer offers distinct benefits for drug delivery. For example, the stretched PEG in the outer shell plays an important role in avoiding the elimination of Nano-DDS by the mononuclear phagocytes in the reticuloendothelial system, and polymers including polypeptides and polysaccharides usually have a large number of active groups (e.g., hydroxyl, amino, and carboxyl) for easier chemical modification and higher drug-conjugating efficiency.65,66
PP-based Nano-DDS can also be distinguished by the self-assembly of PPs or PP-encapsulated nanoscaffolds. As elaborate nanoformulations, self-assembly of drug-conjugated polymers (usually amphiphilic copolymer) to form polymeric micelles possess several potential advantages,67–69 including: (i) increased chemical stability due to the protection by the nanostructures, (ii) facilitated codelivery of different therapeutic agents for combinational therapy, and (iii) easier subsequent functionalizations on the surface of NPs for enhanced drug delivery efficiency. Conversely, although the loading of PPs in various nanocarriers can compromise the drug-loading efficiency to a degree, it often ensures higher stability and longer blood circulation time of the nanosystem.70
As depicted in Fig. 2, according to the construction of macromolecular prodrugs, the PP-based platform can be divided into a pure prodrug platform (PPP) or a combinative prodrug platform (CPP).14 PPP refers to the direct use of drugs as a building unit in the polymer backbone, which is usually designed to ensure that drug molecules have longer persistence during in vivo circulation, as well as to increase the drug loading and drug type to achieve a combination therapy. CPP mostly consists of polymer–anticancer drug conjugates for improved drug solubility and bioavailability. As shown in Fig. 3, the synthesis of drug–polymer conjugate can consist of three strategies:60,65 (i) conjugating a drug to a presynthesized polymer, (ii) conjugating a drug to a monomer prior to polymerization, and (iii) growing a polymer chain initiated from a functionalized drug. The “conjugation to” approach is the most widely employed approach to achieve drug–polymer nanoparticulate systems.
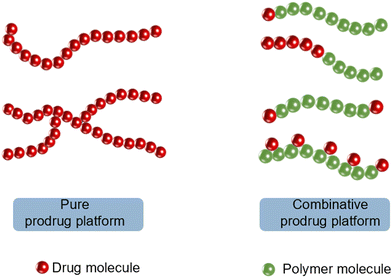 |
| Fig. 2 Classification of polymer prodrug platforms. PP based on a pure prodrug platform and combinative prodrug platform. | |
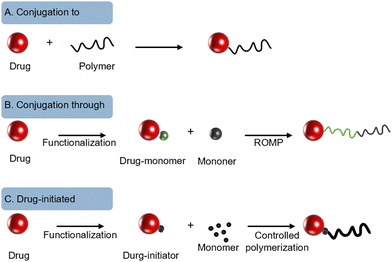 |
| Fig. 3 Three main strategies for the synthesis of drug–polymer conjugates. (A) “Conjugation to” refers to conjugating a drug to a presynthesized polymer. (B) “Conjugation through” refers to conjugating a drug to a monomer prior to polymerization. ROMP: ring-opening metathesis polymerization. (C) “Drug-initiated” refers to growing a polymer chain initiated from a functionalized drug. | |
3. Prodrug-based Nano-DDS approaches for anticancer therapies
Prodrug-based Nano-DDS, which integrate the advantages of both prodrug and nanomedicines into a single nanoplatform, have been developed for effective drug delivery and have multiple advantages for high drug loading, enhanced stability, tumor targeting ability, and safety. This strategy has been widely studied for cancer therapy. In this section, the latest updates in prodrug-based Nano-DDS for various anticancer approaches are reviewed.
3.1 Prodrug-based Nano-DDS in chemotherapy
Chemotherapy remains an essential tool in cancer therapy. Although considerable efforts have been made toward the high-efficiency delivery of chemotherapeutic agents (CAs), several obstacles still limit the curative effect of chemotherapy, including:71,72 (i) the inferior physiochemical properties of anticancer drugs, (ii) their poor metabolic stability and the quick clearance from the body of the CAs, (iii) undesirable distribution and tumor accumulation of the CAs, and (iv) inefficient cellular uptake of the CAs. These disadvantages contribute to the limited therapeutic efficiency and severe toxicity of chemotherapy. In response, the formulation of prodrug (SMP or PP)-based nanomedicines has been widely designed and studied to overcome the delivery barriers of CAs that include the inferior chemical stability, limited tumor targeting, poor tumor accumulation, and low cellular uptake.23,73
3.1.1 SMP-based nanomedicines for chemotherapy.
Among the rational design of SMP-based nanomedicines, the direct self-assembly of homodimeric chemotherapeutics into NPs produces high drug loading, high self-assembly stability, high tumor selectivity, and low excipient-related toxicity. The linkers in the formulation of homodimeric CAs for efficient self-assembly into nanosystems are shown in Table 1. For example, Yang et al. separately introduced disulfide, thioether bonds, and trisulfide bonds for the construction of a doxorubicin (DOX) dimer (Fig. 4).49 Only the trisulfide bond could effectively convert DOX homodimeric prodrugs into self-assembled NPs, and the trisulfide bond insertion showed higher glutathione (GSH) sensitivity compared with that of the disulfide bond. Therefore, the trisulfide bond-bridged prodrug nanoassemblies exhibited high drug-loading efficiency (67.24%, w/w) and tumor selective cytotoxicity, notably reducing the systemic toxicity of DOX.
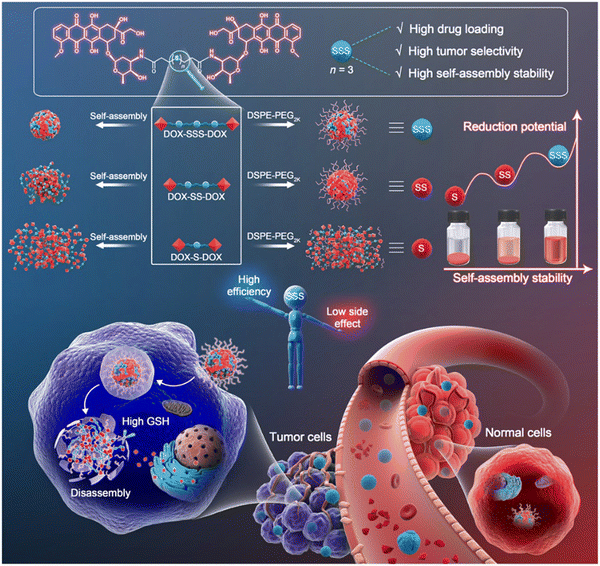 |
| Fig. 4 Construction of nanoassemblies based on trisulfide bond-bridged doxorubicin (DOX) for cancer therapy. Reproduced from reference 49 with permission from Science, copyright 2020. | |
Nano-DDS based on a lipid–CA conjugate has also attracted attention for cancer chemotherapy. Lipids such as fatty acids, cholesterol derivatives, phospholipids, and triglycerides, have been extensively used as promoieties covalently conjugated to CAs.24,74 The lipid conjugation and subsequent nanosystem formulation helped to:75,76 (i) resolve the poor solubility of several CAs, (ii) increase their biocompatibility with biomaterials approved by the Food and Drug Administration (FDA), and (iii) be readily internalized by cancer cells because of their lipophilic nature. Motivated by this concept, Wang and colleagues exploited a combinational strategy that involved a structure-guided prodrug formulation and a sequential prodrug-induced self-assembly process by conjugating the chemotherapeutic CTX with several lipids including DHA, linolenic acid, linoleic acid, oleic acid, stearic acid, and heptanoic acid. The resulting prodrug amphiphiles spontaneously formed nanocrystals in aqueous solutions.12 The DHA modification on CTX produced enhanced in vivo stability, pharmacologic efficacy, therapeutic efficacy, and safety in comparison with conjugation with other lipids.
In addition to the self-assembled systems, structural similarities between lipid prodrugs and lipid-based nanocarriers may facilitate prodrug loading or insertion, for instance via simple nanocarrier membrane anchoring.77 As shown in Fig. 5(A), our work demonstrated that the conjugation of cholesterol with therapeutic SN38 substantially increased the miscibility with a liposomal scaffold.25 Our esterase-activatable prodrug (Chol-SN38)-based nanoassemblies exhibited elevated therapeutic efficacy in a human lung carcinoma murine model and improved drug tolerability in mice in comparison to the SN38 clinical counterpart irinotecan (Fig. 5(B) and (C)).
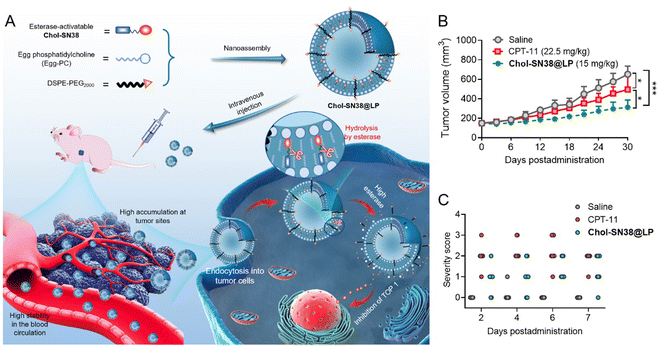 |
| Fig. 5
In vivo delivery of esterase-activatable SN38 prodrug-based NPs Chol-SN38@LP. (A) Schematic illustration of the preparation and intravenous administration of Chol-SN38@LP for cancer therapy. Tumor growth curves of mice (B) and the bloody diarrhea severity score in mice (C) after treatment with Chol-SN38@LP and irinotecan (CPT-11). Reproduced from reference 25 with permission from The Royal Society of Chemistry, copyright 2022. | |
Lipid–CA based nanosystems have also been substantially applied to deliver inorganic therapeutics including the platinum (Pt) family.78 For instance, a lipid-based Pt prodrug was formulated to grow alkyl chains at the axial positions and to ensure the stability in the hydrophobic core of the NPs for increased cancer therapy in the serial studies of Farokhzad et al.79–81 Their recent research showed that both the Pt prodrugs and cysteine-based poly-(disulfide amide) (Cys-PDSA) polymers that readily react with GSH were formulated together with lipid–PEG to generate Pt(IV) prodrug-loaded Cys-PDSA NPs (CP5 NPs) for efficient Pt delivery and reversal of drug resistance (Fig. 6(A)). Prodrug-based NP formulation and tumor microenvironment responsive release of Pt showed prolonged persistence of Pt in systemic blood circulation and achieved tumor specific drug release of the payload in the redox tumor microenvironment (Fig. 6(B)). The resulting CP5 NPs displayed substantial growth inhibition of cisplatin-resistant A2780cis xenograft tumors and negligible systemic toxicities compared with those of the conventional form (Fig. 6(C)).81
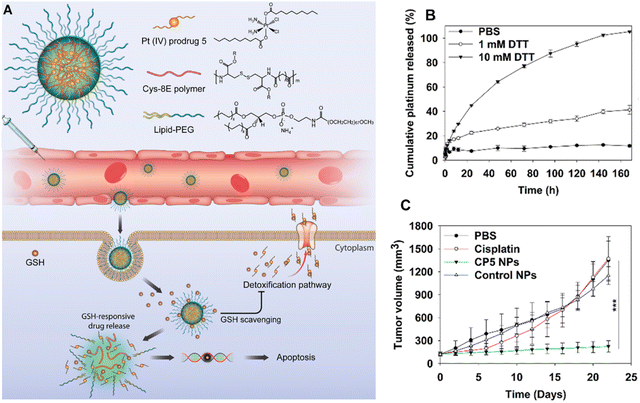 |
| Fig. 6 Study of Cys-PDSA NPs (CP5 NPs). (A) Illustration of the Pt(IV) prodrug-based nanoplatform for treating cisplatin-resistant tumors. (B) Drug release profile of CP5 NPs upon redox stimuli with dithiothreitol (DTT). (C) Tumor volumes of A2780cis tumor-bearing mice after treatment with CP5 NPs. Reproduced from reference 81 with permission from American Chemical Society, copyright 2018. | |
3.1.2 PP-based nanomedicines for chemotherapy.
Polymeric nanosystems are the most popular and widely used nanoplatforms for treating malignancies. The excellent properties of polymeric nanocarriers enable tailored control to a suitable size for tissue penetration and specific cellular intake via sophisticated material modification.82 Various polymers have been successfully used to synthesize polymer–drug conjugates for cancer therapy. Nanosystems self-assembled from amphiphilic polymer–CA conjugates or formulated from the encapsulation of polymer–CA conjugates with nanocarriers each have specific advantages. BCPs have been the most commonly used polymers to synthesize self-assembling NPs based on polymer–drug conjugates via “conjugation to”.83,84 The hydrophobicity of the drug is of crucial importance as this will govern the self-assembly of the conjugate into nanocarriers. Various CAs have been conjugated with BCPs to form self-assembled micelles and successfully achieved higher water solubility and drug payloads with sustained drug release in the circulation than seen with the traditional system containing physically entrapped CAs.85–87 For example, block copolymer poly(ethylene glycol)-block-poly(pyridyldisulfide ethylmethacrylate) (PEG-PDSEMA) was conjugated to thiolactone functionalized drug (PTX, CPT) to produce imidazole and disulfide bonds-incorporated BCPs, which subsequently self-assembled into stable micellar NPs (Fig. 7(A) and (B)).85 The resulting CA-BCP polymer-based self-assembled NPs achieved extremely high drug loading (>50%), pH-sensitive charge transition for cellular internalization, and GSH-responsive drug cleavage for precise drug release inside cancer cells. Finally, those two NPs exhibited complete tumor completion in tumor murine models.85
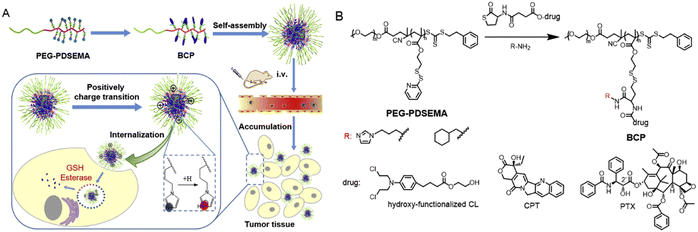 |
| Fig. 7 Preparation and study of block copolymer prodrug (BCP) NPs. (A) Schematic illustration of the construction of BCPs, self-assembly into micellar NPs, and intratumoral delivery process. (B) Chemical synthesis of BCPs via thiolactone chemistry reaction. Reproduced from reference 85 with permission from Elsevier Ltd, copyright 2017. | |
In comparison with self-assembled nanomedicines based on polymer–CA conjugates, the encapsulation of the PP with carriers (i.e., biphilic polymer carrier or liposomes) can enhance chemical stability because of the miscibility with the scaffold and the protective effect of the nanostructures. This facilitated the codelivery of different therapeutic agents in the same vehicle for combinational therapy and facile functionalization on the surface of Nano-DDS for enhanced drug delivery.70,88 Polymer materials, such as poly(lactic acid) (PLA), poly(lactic-co-glycolic acid) (PLGA), polystyrene, polyvinylalcohol, polyglycolic acid, polyethyleneimine, poly(acrylic acid), poly(glutamic acid), and cellulose, are employed for the polymer–drug conjugation and subsequent polymeric NP fabrication for drug delivery.89 Wang et al., recently reported a simple and rational design for the conjugation of SN38 to oligo-ε-caprolactone (oligoCL) via an esterase-activatable linkage to form oligoCLn-SN38 conjugates that were subsequently encapsulated by structurally similar matrices (i.e., PEG-block-poly(ε-caprolactone) copolymers (PEG-b-PCL)) to form injectable NPs.90 Compared with treatment using the clinical counterpart CPT-11, oligoCLn-SN38 conjugate-based Nano-DDS administration achieved 62.8% (p = 0.001, versus CPT-11) reduction in terms of tumor number in orthotopic colonic cancer.
3.2 Prodrug-based nanomedicines in phototherapy
PDT and PTT are light-regulated, noninvasive medical technologies that have been widely studied in cancer treatment. They use irradiation with PSs to generate reactive oxygen species (ROS) or PTAs to generate local hyperthermia (>50 °C) for destroying cancer cells.91–93 In recent years, PSs and PTAs have been conjugated with multiple components including lipids, drugs or polymers to formulate PS/PTA-based prodrugs that can self-assembled into micelles or be delivered by nanoscaffolds.94,95 The reports on self-assembled nanoparticles based on PS or PTA prodrugs for cancer PDT and PTT are summarized in Table 2. Chlorin e6 (Ce6) is the most studied photosensitizer in the prodrug formulation and subsequent self-assembly into nanoparticles for cancer PDT therapy.97,99,108
Table 2 Self-assembled nanomedicines based on photosensitizer or photothermal agents conjugate for photodynamic therapy (PDT) and photodermal therapy (PTT) based antitumor therapy
Photosensitizer |
Prodrug conjugate |
Therapy |
Findings |
Ref. |
Pyropheophorbide-a (PPa) |
PR104A-S-PPa |
PDT/chemotherapy |
Nanoplatforms self-assembled from PR104A-TK-PPa exhibited higher tumor accumulation and better combination therapy efficiency in 4T1 tumor-bearing mice. |
99
|
PR104A-TK-PPa |
Chlorin e6 (Ce6) |
Ce6-linolenic acid |
PDT/chemotherapy |
Coassembly with photoactivatable LTK-cabazitaxel prodrug, Ce6-LA achieved a dramatic shrinkage of tumors in a melanoma PDX mouse model. |
95
|
Ce6-R9-125I-RGD |
PDT/radiotherapy |
Self-assembled with miR-139-5p, Ce6-R9-125I-RGD-MNPs formed nanoparticles inhibited growth of HeLa cells and tumors. |
106
|
PEG-Poly(Ser-Ce6) |
PDT/chemotherapy |
Coassembly of PTX2-Azo and PEG-Poly(Ser-Ce6) induced synergistic antitumor effect in HeLa tumor bearing mice. |
97
|
Methylene blue (MB) |
MB-SS-biotin-azide (MBP) |
PDT |
MBNPs, self-assembled from MBP, showed remarkable PDT efficacy and negligible toxicity in HeLa tumor model. |
107
|
Methylene violet (RAX) |
RAX-perylene diimide (PRX) |
PDT/PTT |
Nanoparticles self-assembled from PRX exhibited high phototoxicity against A549 cells in vitro. |
108
|
Diketopyrrolopyrrole (DPP) |
Diketopyrrolopyrrole-benzothiazole-triphenylamine (DPP-BT-TPA) |
PTT/chemotherapy/antiangiogenic therapy |
Coassembly of DPP-BT-TPA with CPT-SS-CA4 exhibited improved combinative antitumor efficacy in 4T1 tumor-bearing mice. |
94
|
Cypate |
Cypate-Phe-Phe-Lys(SA-HCQ)-Tyr(H2PO3)-OH (Cyp-HCQ-Yp) |
PTT/autophagy inhibition |
Under sequential catalysis of ALP and CES, Cyp-HCQ-Yp is converted to Cyp-Y, which self-assembles into its nanoparticle Cyp-NP and showed enhanced cancer therapy in HepG2 tumor-bearing mice. |
105
|
PDT therapy often results in the exhaustion of oxygen and the over production of ROS, and most studies therefore combine PDT therapy with hypoxia- or ROS-activated therapy.32,96,97 For example, Feng et al., prepared a multifunctional liposome by simultaneously loading hydrophobic hexadecylamine-conjugated chlorin e6 (hCe6) and hydrophilic hypoxia-activated prodrug AQ4N, and a 64Cu isotope was also chelated to Ce6 for in vivo PET imaging.98 Post-iv injection of the AQ4N-hCe6-liposome after irradiation could induce severe tumor hypoxia, which was favorable for the activation of AQ4N and producing effective tumor regression in 4T1 tumor-bearing mice. Another study adopted a similar concept for combinative therapy by formulating a PS prodrug by the ligation of hypoxia-activated prodrug PR104A to PS using a ROS sensitive thioether or thioketal linkage. Both two heterodimeric prodrugs self-assembled into high drug coloading combinative NPs, which efficiently generated ROS under light irradiation for PDT (Fig. 8(A)). Meanwhile, the ROS sensitive linkers rapidly underwent cleavage to release PR104A, which was then further activated by the hypoxia in the tumor for synergistic antitumor efficacy (Fig. 8(B)). Such PS prodrug-based NPs successfully facilitate specific drug release, showing high-efficiency synergy in combination with PDT and hypoxia-activated therapy (Fig. 8(C)).99
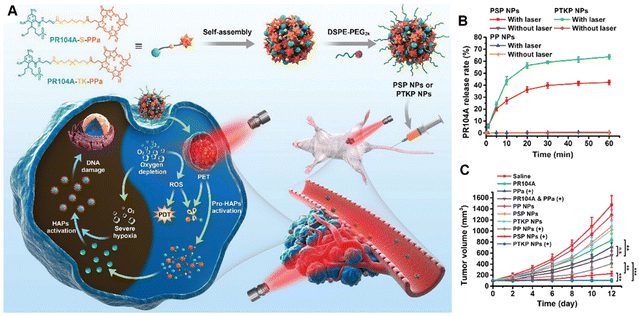 |
| Fig. 8 Use of self-assembled heterodimeric prodrug-based NPs. (A) Schematic of the preparation of self-assembled heterodimeric prodrug-NPs (PSP NPs or PTKP NPs). (B) In vitro PR104A release of prodrug-NPs under laser irradiation. (C) Tumor growth curves. Reproduced from reference 99 with permission from The Royal Society of Chemistry, copyright 2020. | |
PTT, which relies on local hyperthermia generated by PTAs under near-infrared (NIR) laser irradiation is an attractive alternative approach to PDT.100,101 The heat induces cell death by apoptosis or necrosis for efficient tumor eradication, conferring noninvasiveness and reduced side effects. Unfortunately, it may cause severe collateral heating damage to neighboring healthy tissues, which can induce serious side effects and unendurable heat pain to patients. Hence, to perform PTT at a mild local temperature (<45 °C) is of clinical significance.102 However, mild-temperature PTT alone exhibits a limited anticancer ability in most cases, thereby necessitating adjuvant strategies (e.g., chemotherapy, gene therapy, or immunotherapy) to enhance its therapeutic efficacy.103,104
Inspired by these studies, a study proposed an alkaline phosphatase (ALP) and carboxylesterase (CES) dual-responsive prodrug to enhance the therapeutic efficacy of mild-temperature PTT through autophagy inhibition. Under sequential enzymatic catalysis by ALP and CES, this prodrug (Cypate-Phe-Phe-Lys(SA-hydroxychloroquine)-Tyr(H2PO3)-OH) (Cyp-HCQ-Yp, Fig. 9(A)–(C)) is converted into Cyp-Y which self-assembles into Cyp-Y-NPs in tumor cells, accompanied with release of the autophagy inhibitor HCQ.105 The exceptional therapeutic antitumor effect of Cyp-HCQ-Yp in vivo was achieved by the dual-enzyme-controlled intracellular NP formation and autophagy inhibition in tumors.
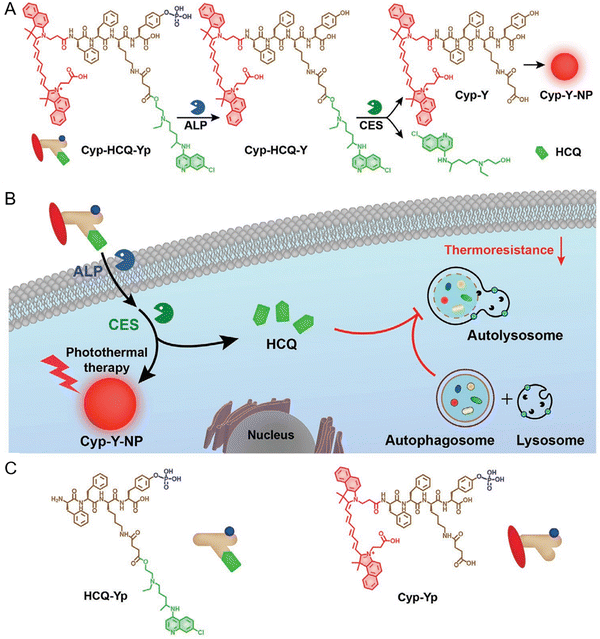 |
| Fig. 9 A “smart” prodrug Cyp-HCQ-Yp for autophagy-inhibited mild-temperature photodermal therapy. (A) The enzymatic transformation of Cyp-HCQ-Yp into Cyp-Y which self-assembles into Cyp-Y-NPs. (B) Schematic of the working mechanism of Cyp-HCQ-Yp. (C) Chemical structures of two control agents HCQ-Yp and Cyp-Yp. Reproduced from reference 105 with permission from Wiley, copyright 2021. | |
3.3 Prodrug-based nanomedicines in molecular-targeting therapy
Distinct from traditional cytotoxic chemotherapy, targeted therapy efficiently inhibits cancer proliferation by interfering with the specific signaling pathways and relevant kinases involved in tumor development and metastasis.109 Small molecular inhibitors (SMIs), including tyrosine kinases inhibitor (TKI), represent a major portion of targeting agents used for targeted therapy.110 However, many existing SMIs have extremely low water solubility and require oral medication in large doses that may produce poor biodistribution and severe systemic toxicities. The chemical modification of such inhibitors is a viable way to increase self-assembly or loading by nanocarriers in water. TKIs are widely studied for prodrug formulation.111 Li et al. synthesized a redox-sensitive prodrug polymers poly(oligo(ethylene glycol) methacrylate) (POEG)-b-PSSDas and a redox-insensitive POEG-b-PCCDas that comprise POEG hydrophilic blocks and oncogenic TKI dasatinib (DAS, the inhibitor of Src/Bcr-Abl)-conjugated hydrophobic blocks to form mixed micelles with DOX. The system substantially enhanced the solubility of DAS and facilitated the intravenous delivery of DAS.112 The incorporation of disulfide linkages into the codelivery system facilitated efficient cleavage of DAS from prodrug micelles in tumor tissues, generating increased tumor inhibition and survival rate in an aggressive 4T1.2 tumor mice model.
Another study designed a TKI-based prodrug that covalently linked epidermal growth factor receptor (EGFR)-TKI gefitinib with a NIR chromophore via a cleavable disulfide bond. This prodrug (G-SS-NIR) could readily self-assemble into NPs driven by the insertion of disulfide bonds and intermolecular π–π interaction in aqueous media (Fig. 10(A)).113 The Akt inhibitor celastrol (CEL) is encapsulated into the NPs to form a nanodrug (CEL@G-SS-NIR). Upon the overexpression of GSH in the tumor, the nanodrug is activated and releases two inhibitors to exert a tumoricidal effect and enable NIR for both fluorescence and multispectral optoacoustic tomography (Fig. 10(B) and (C)). Furthermore, the strong “turn-on” fluorescence caused by aggregation-induced emission and multispectral optoacoustic tomography imaging inside the tumor allows for precise monitoring of the drug release. The study validated that the nanodrug containing two inhibitors exhibits high inhibition efficacy against orthotopic nonsmall cell lung cancer in murine models (Fig. 10(D)).
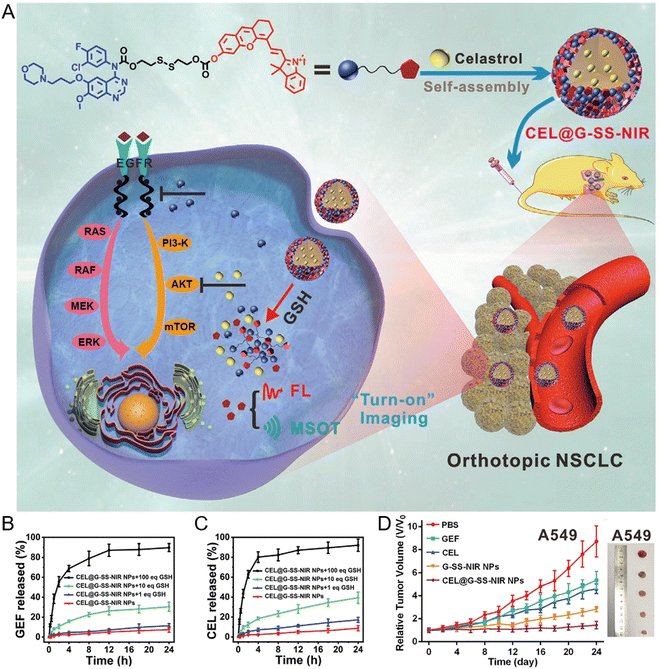 |
| Fig. 10 Formation and in vivo delivery of a gefitinib prodrug-based nanodrug (CEL@G-SS-NIR NPs). (A) Schematic illustration for the formation of CEL@G-SS-NIR NPs and the therapeutic action on non-small cell lung cancer (NSCLC). Drug release profile of gefitinib (B) and celastrol (CEL) (C) from nanodrugs treated with various equivalents of GSH. (D) Tumor volume of NSCLC-bearing mice treated with different drug formulations. Reproduced from reference 113 with permission from Wiley, copyright 2020. | |
3.4 Prodrug-based nanomedicines in immunotherapy
Immunotherapy has shifted the clinical paradigm of cancer management. Recently, prodrug-based nanoplatforms have been widely used for efficient delivery of immune modulators and to integrate multiple immune regulators with variable chemophysical properties and pharmacokinetic profiles into a single nanoplatform to provide synergistic immunotherapy.28,114,115 In this section, we summarize the major applications of prodrug nanomedicines as strategies to improve cancer immunotherapy.
3.4.1 Immunogenic cell death (ICD)-based prodrug Nano-DDS for cancer immunotherapy.
Both chemotherapy (e.g., DOX, oxaliplatin, PTX) and PDT can enhance antitumor immunity by inducing ICD.116,117 Tumor cells undergoing ICD will release immune-stimulatory damage-associated molecular patterns (e.g., calreticulin, ATP, and high mobility group protein B1) that are recognized by antigen-presenting cells (APCs; e.g., dendritic cells) and thus initiate their activation.118 Activated APC cells encounter tumor-associated antigens (TAAs) and present these to T lymphocytes, eliciting a protective T-cell immune response. The ICD inducer-based NPs can considerably improve solubility and stability for systemic injection, which ultimately induces potent ICD effects for anticancer therapy.119 Chen et al. designed a core–shell prodrug-based magnetic NP (ETP-PtFeNP) that contained polymeric shells of an oxaliplatin prodrug modified with an α-enolase-targeting peptide and a core made of oleic acid–Fe3O4120 (Fig. 11(A)). They showed that peptide-modified shell caused substantial tumor accumulation and subsequent endocytosis, and the Pt(IV) prodrugs were activated to specifically release oxaliplatin in the highly reductive conditions inside tumor cells. In addition, the Fe3O4 in the NP core can be activated to release ferric ions to generate cytotoxic ROS in the acidic tumor microenvironment (Fig. 11(B) and (C)). Thus, the released oxaliplatin and ferric ions synergistically induced strong ICD-associated immunogenicity and antitumor immune responses via both endoplasmic reticulum (ER) and non-ER associated pathways (Fig. 11(D)).
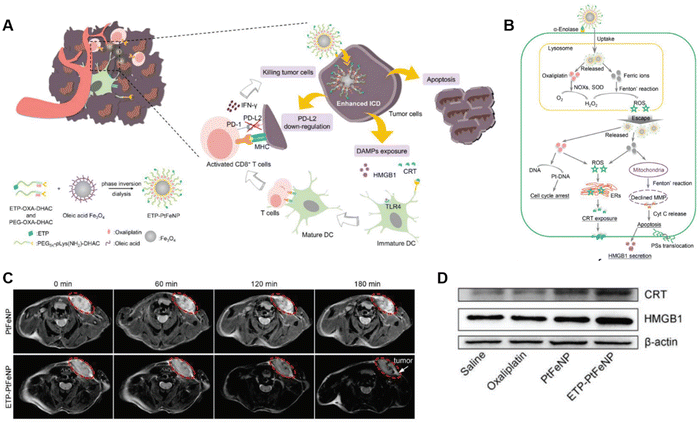 |
| Fig. 11 Study of ETP-PtFeNP formulations. (A) The preparation of ETP-PtFeNP and representation of elevated antitumor immune responses with ETP-PtFeNP treatment. (B) Illustration of ETP-PtFeNP modulation via three pathways for ICD induction. Magnetic resonance images (C) and western blotting analysis of CRT and HMGB1 expression (D) for mice bearing 4T1 breast tumors following treatment with ETP-PtFeNP. Reproduced from reference 120 with permission from WILEY, copyright 2019. | |
3.4.2 Immunosuppression inhibition-based prodrug nanomedicines for attenuating immune tolerance.
Immune checkpoints play essential roles in immunotolerance in cancer immunotherapy.121,122 In recent years, immune checkpoint blockade (ICB) has reached a milestone in cancer therapy. However, those therapeutic inhibitors, including targeting peptides, antibodies, and SMIs, block immune cell checkpoints that are both important and irrelevant to cancer therapy.123 Such an indiscriminate blockade on immune cells limits their efficacy and readily causes the autoimmune toxicity of the therapy.124 Thus, constructing prodrugs by chemical modification and delivering them via nanocarriers to target immune checkpoints of cancer-reactive immune cells may be beneficial. To illustrate this concept, substantial efforts have been devoted to developing immune checkpoint inhibitors based prodrugs and nonosystems for efficient targeting and delivery.125,126
Recently, monoclonal antibody-based ICB therapy has significantly advanced cancer immunotherapies in the clinic. For example, antibodies to PD-L1, PD-1, or CTLA-4 have been conjugated to lipid components to form antibody functionalized lipid NPs or have been conjugated to biodegradable polymer (PLGA, poly(amidoamine) dendrimers) (PMLA/LLL) to form polymeric NPs for ICB therapy.127–129 As evidence of this, Xiao et al. developed a dual pH-sensitive prodrug-based NP (CUR@PPC-αPD-1), formed by the conjugation of the antibodies to PD-1 (αPD-1) with di-block copolymer (CDM-PEG-PDPA) via an ammonolysis reaction and loaded curcumin in their core130 (Fig. 12(A)–(C)). The antibody-modified NPs specifically bound the circulating PD-1+ T cells, producing effective tumor accumulation. Afterward, the pH-sensitive linker in the surface of αPD-1-modified NPs was cleaved in the acidic tumor microenvironment to release αPD-1 at the tumor site. After being internalized by tumor cells, the pH-responsive nanomedicine disassembled under the acidic condition of lysosomes, causing a rapid release of the encapsulated curcumin, which inhibited the nuclear factor kappa B (NF-κB) pathway and enhanced the effect of anti-PD-1/PD-L1 immunotherapy. This combination enabled the simultaneous blockade of the PD-1/PD-L1 pathway and tumor infiltration by antitumor T cells enabled by curcumin delivery, causing impressive antitumor efficiency.130
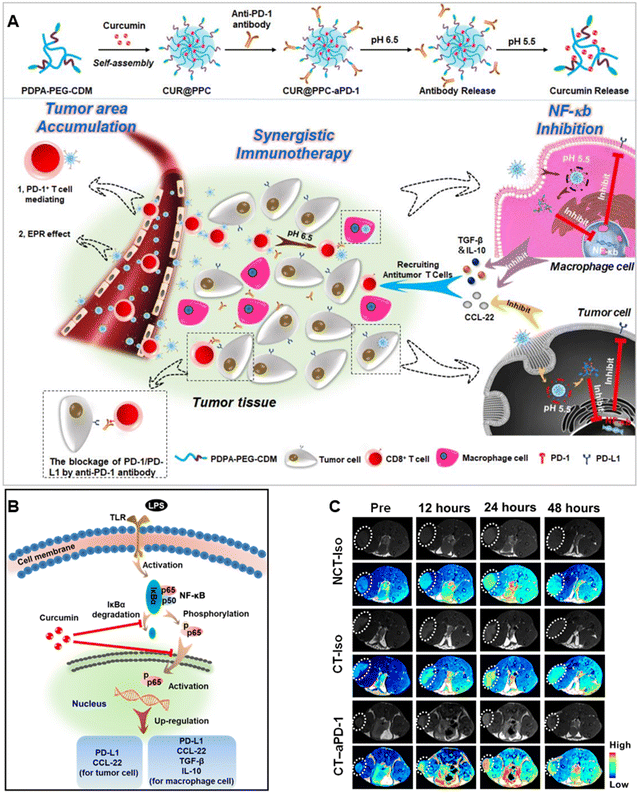 |
| Fig. 12 Study of nanodrug PDPA-PEG-CDM. (A) Preparation and tumor-targeted delivery of a dual pH-sensitive nanodrug. (B) Schematic of curcumin to suppress cytokine secretion and PD-L1 expression by inhibiting the NF-κB pathway. (C) In vivo T1-weighted magnetic resonance imaging (T1WI) of mice bearing B16F10 tumors after iv injection of MnO2@PPC-αPD-1 or MnO2@PPC-Iso. Reproduced from reference 130 with permission from Science, copyright 2020. | |
Except for immune checkpoints, several other factors also cause strong local immunosuppression, including indoleamine 2,3-dioxygenase (IDO),131 interleukin 10 (IL-10),132 TGF-β,133 NF-κB,134 and CD47.135 Thus, strategies are also needed to block these immunosuppressive factors. IDO is a key negative feedback protein that acts by suppressing the activity of the effector T cells and recruiting regulatory T cells, thus inhibits the activity of infiltrating cytotoxic T lymphocytes (CTLs) and produces an immunosuppressive tumor microenvironment (ITM). The IDO pathway inhibitors (e.g., NLG919) have shown encouraging clinical outcomes.136 However, the commonly used small-molecule drugs have shown poor pharmacokinetic effects when administered in free form. Therefore, IDO inhibitors formulated prodrug-based nanomedicines have recently been investigated for combating IDO-induced immune tolerance. Han et al., recently constructed a peptide–drug conjugate for IDO-1-induced immune resistance inhibition.137 First, a peptide–drug conjugate was synthesized by conjugating the hydrophilic targeting motif arginylglycyl-aspartic acid (RGD) with a potent IDO-1 inhibitor NLG919, via two protonatable histidines, and then self-assembled into NPs. The NLG919 prodrug has several significant advantages, including specific tumor targeting via modification of RGD, pH-responsive disassembly by protonatable histidines, and controllable esterase-catalyzed drug release of NLG919 (Fig. 13(A)). The NLG919 prodrug had enhanced inhibition of intratumoral IDO to considerably improve the therapeutic efficacy in the combination of PD-L1 blockade therapy and reduce the systemic toxicity (Fig. 13(B)).
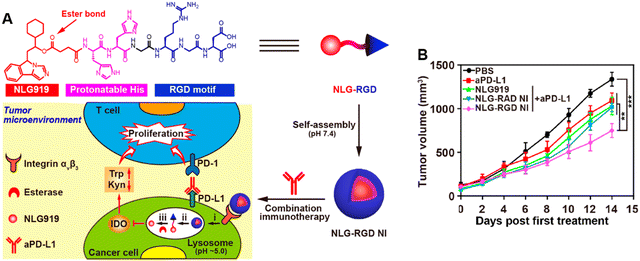 |
| Fig. 13 Preparation and antitumor study of a prodrug-based nanomedicine NLG-RGD. (A) Schematic of the self-assembly of NLG-RGD and the mechanism of combination therapy using NLG-RGD NI and aPD-L1. (B) Tumor growth curves of Pan02 tumors in mice using NLG-RGD NI and aPD-L1. Reproduced from reference 137 with permission from American Chemical Society, copyright 2020. | |
3.4.3 Cancer vaccine-based prodrug formulated nanomedicines for cancer immunotherapy.
Vaccination therapy is an attractive strategy for tumor immunotherapy.138 The vaccination therapy starts with the release of TAAs and costimulation signals (adjuvants), which are internalized and processed by APCs in secondary lymphoid organs. The antitumor immunotherapy is thereby enhanced by cross-presentation of tumor epitopes by APCs to naive or memory T cells.139 The ICD therapy can be categorized as an endogenous vaccination therapy as this generated endogenous TAAs by the tumor to activate APCs and initiate antitumor immunotherapy. However, the majority of cancer vaccination is realized by the exogenous administration of such TAAs (i.e., peptides, cell membrane.) together with adjuvants, which are generally defined as “exogenous vaccination”.115
Despite considerable advances having been made in cancer exogenous vaccines, several disappointing studies have hindered their widespread use.140 These obstacles may be ascribed to unsynchronized delivery of the neoantigens and adjuvants to the tumor draining lymph nodes (dLNs) in a traditional drug delivery platform, causing immune tolerance and decreased proliferation of tumor-cytotoxic CTLs. Nanoplatforms are the best tools to address these challenges. They can encapsulate antigens and adjuvants to prevent degradation and achieve stable codelivery.141 For example, Kuai et al. designed novel vaccine high-density lipoprotein-mimicking nanodiscs that coupled with redox responsive antigen and CpG oligonucleotide adjuvant (TLR-9 agonist) for antigen/adjuvant codelivery (Fig. 14(A) and (B)).142 Strikingly, the authors revealed that the vaccine nanodiscs produced up to 47- and 31-fold greater frequencies of neoantigen-specific CTLs than those produced by soluble vaccines and the strongest adjuvant in clinical trials (CpG in Montanide), respectively. More importantly, when combined with ICB therapy, the nanovaccine eliminated the growth of both MC-38 and B16F10 tumors (Fig. 14(C)–(E)).
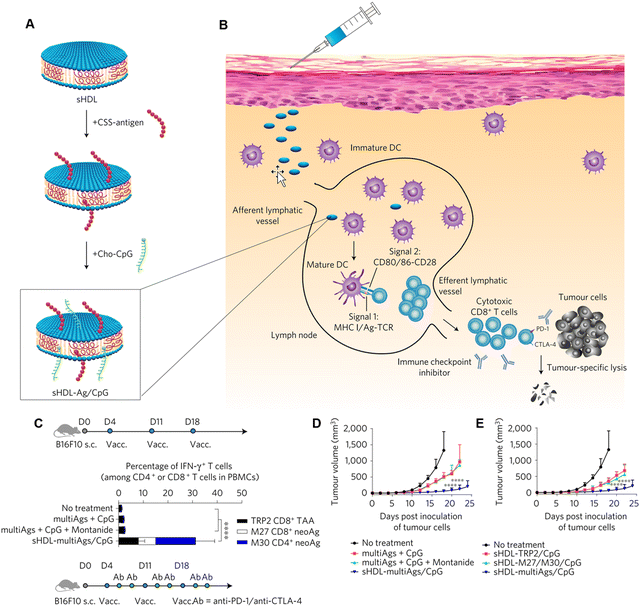 |
| Fig. 14 Design of high-density lipoprotein (sHDL)-mimicking nanodiscs that coupled with redox responsive antigen peptide (Ag) and adjuvant CpG for codelivery. (A) sHDL nanodisc platform engineered for codelivery of Ag peptides and adjuvants. (B) Ag and CpG were efficiently codelivered to draining lymph nodes by nanodiscs, promoting strong Ag presentation by dendritic cells (DCs) (Signal 1), inducing DC maturation (Signal 2) and causing the activation of Ag-specific CD8+ cytotoxic T-lymphocyte (CTL) responses. Activated CTLs recognize and kill their target cancer cells. The combination with ICB further amplifies the potency of nanodisc vaccination. (C) The schedule of tumor eradication by combination immunotherapy with multiepitope vaccine and ICB. (D) and (E) The tumor growth curves of B16F10 tumor-bearing mice after various drug formulations. Reproduced from reference 142 with permission from Springer Nature, copyright 2016. | |
Another major challenge for vaccination therapy is to prime a strong and effective immune response of effective CTLs through cross-presentation of the antigens.143 Ideally, the antigens should successfully escape from the lysosomes of APCs and be loaded onto MHC-I molecules for presentation to CD8+ T cells instead of being loaded onto MHC-II for presentation to CD4+ T cells. Wei et al.144 addressed this challenge by preparing a redox-sensitive polycondensate neoepitope (PNE) to deliver neoantigens and adjuvants for tumor vaccination immunotherapy. The PNEs were constructed by crosslinking neoantigen peptides (monomer A) and amphiphilic adjuvants (monomer B) with a redox-liable linker (Fig. 15). The PNEs showed an efficient LN accumulation and DC uptake. More importantly, the intracellular reduction in APCs could trigger rapid release of neoantigens from PNEs to facilitate endosomal escape and cross-presentation of neoantigens by MHC-I, thereby considerably improving the immune response in a prophylactic mouse model.
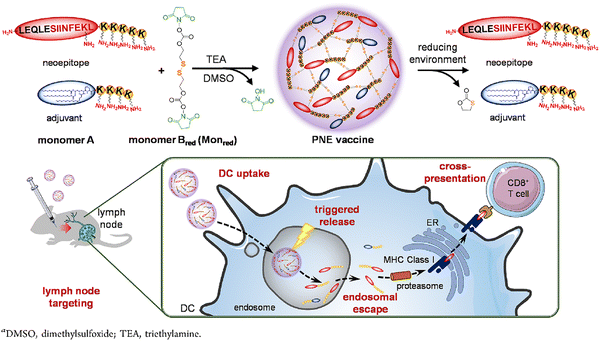 |
| Fig. 15 Schematic of the synthesis, responsive release, and in vivo efficacy of redox-sensitive polycondensate neoepitope (PNE) vaccines. Reproduced from reference 144 with permission from American Chemical Society, copyright 2020. | |
3.4.4 Immune adjuvant-based prodrug nanomedicines for adoptive cell therapy of tumors.
Various costimulating pathways have been identified, and corresponding agonists that function as vaccine adjuvants have been developed.145 The adjuvants are critical components of many vaccines and can enhance the magnitude, breadth, quality, and longevity of the immune response to the antigens. One widely used immune adjuvant, Toll-like receptor agonists (TLRa) have been shown to be the most potent adjuvants discovered to effectively stimulate APCs for antigen presentation and T-cell immune responses.146 A TLR-9a based on a CpG-motif oligonucleotide has been widely investigated in preclinical trials.147 However, because of their working mechanism and high toxicity when administrated in a free form and subsequent spread into the systemic circulation, the novel drug formulations need to be exploited. Although polymeric carriers have a potential for improved TLR delivery by integrating both TLRa and antigens into one vector, noncovalent encapsulation of TLRa often causes burst release of payloads after administration.
To facilitate efficient dLN-targeted delivery of immune adjuvants, Irvine and colleagues designed an “albumin hitchhiking” approach.148 Dyes with certain hydrophobic moieties can efficiently bind to endogenous albumin, and the albumin/dye compounds can then be transferred to LNs in patients with cancer. Inspired by this phenomenon and the role of albumin as a fatty acid transporter, the TLR9 agonist CpG was modified with selected hydrophobic chemical groups (cholesterol, monoacyl lipid, and diacyl lipid) that bind to albumin, which facilitates transportation of CpG to LNs (Fig. 16(A)). These amphiphiles can self-assemble into three-dimensional spherical micelles with a CpG corona and a lipid core in aqueous solutions (Fig. 16(B)). With the optimized lipophilic moiety-diacyl lipid tail, the CpG trafficking to LNs was significantly enhanced compared with that of native CpG or CpG with suboptimal modifications. The optimized amphiphilic CpG self-assembled micelles had a 30-fold increase in T-cell priming and tumoricidal efficacy. Furthermore, this “albumin hitchhiking” approach greatly reduced the toxicities of CpG.
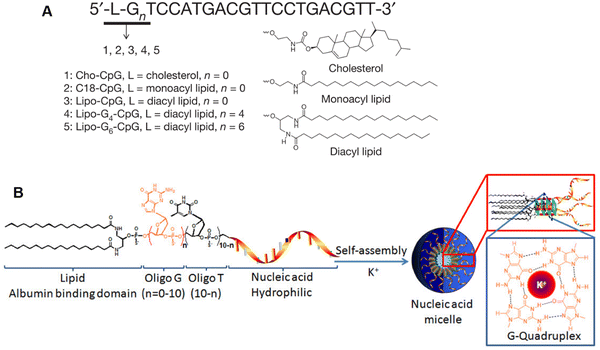 |
| Fig. 16 Construction and characterization of lymph node-targeted G-quadruplex-stabilized CpG adjuvants. (A) Structure of Amph-CpGs. (B) G-quadruplex-stabilized CpG micelles self-assembled from amphiphiles. Reproduced from reference 148 with permission from Springer Nature, copyright 2014. | |
In comparison with TLR-9a, TLR-7/8a (such as imiquimod, R848) have better potential for clinical translation since all APCs in humans express both TLR-7 and TLR-8.149,150 To facilitate the LN drainage and intracellular release of TLR7/8 agonists, De et al. synthesized an IMDQ prodrug based on the conjugation with acid-activatable amphiphilic block copolymer.151 The IMDQ-conjugated amphiphilic block polymer could successfully self-assemble into micellar NPs (AmphIMDQ) to reduce what was described as “wasted inflammation” during systemic circulation (Fig. 17(A)). AmphIMDQ provoked a significantly stronger LN-localized response compared with that of soluble IMDQ and hydrophilic block copolymer-assembled IMDQ micelles (Hydro), causing strong activation of DCs (Fig. 17(B)). AmphIMDQ also showed excellent acid sensitivity in the endosomal compartment, causing hydrolysis into the unimer fraction by cleaved ketal bonds but remained highly stable under physiological conditions representing systemic circulation. Localized treatment with a NP-conjugated IMDQ was confirmed to potently activate DCs in the sentinel LN and promote proliferation of tumor antigen-specific CD8+ T cells, which considerably improve the therapeutic effect upon combination with anti-PD-L1 checkpoint inhibition and Flt3L, a growth factor that expands and mobilizes DCs from the bone marrow (Fig. 17(C)).152
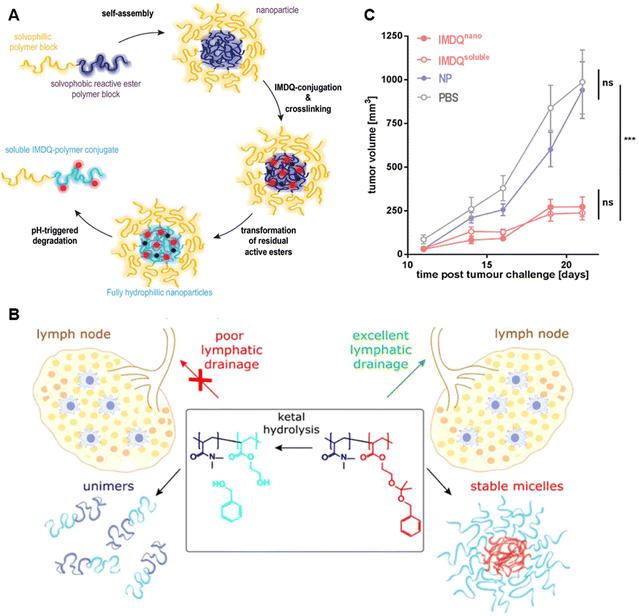 |
| Fig. 17 Assembly, fabrication, and efficacy evaluation of IMDQnano. (A) Schematic of the fabrication of IMDQnano. Reproduced from reference 152 with permission from WILEY, copyright 2018. (B) Concept of localized tumor treatment with IMDQnano in draining lymph nodes. Reproduced from reference 151 with permission from American Chemical Society, copyright 2018. (C) Tumor growth of B16F10 tumors in mice, in response to treatment with soluble IMDQ and nanoformulated IMDQ. Reproduced from reference 152 with permission from WILEY, copyright 2018. | |
4. Prodrug-based combination nanotherapy
The heterogeneity and complexity of tumors often results in the emergence of drug resistance and tumor relapse following cancer treatment via a single agent strategy. Therefore, using a single chemotherapy agent is less common in actual clinical applications. Combination therapies help to overcome the limitations encountered with each therapy alone and has shown enhanced tumor responses in patients.153 However, the therapeutic challenges of combination chemotherapeutic therapy include unsatisfactory therapeutic effects and unfavorable toxicities caused by incorrect choice of dosages, incorrect ratio of drugs, and inaccurate drug release. Prodrug-based Nano-DDS has shown considerable benefits in combination therapy in comparison with conventional nanocarriers (e.g., micelles, liposomes, and polymeric NPs).
Combination drug therapy based on a prodrug nanoplatform can be categorized into three types:154 (i) all drugs are bound together to construct a prodrug for nanoformulation; (ii) some of the free therapeutic drug is encapsulated in the anticancer prodrug assembled nanosystem; and (iii) some of the free therapeutic drug is used as additive therapy but not included in the prodrug-based antitumor nanosystem. The combinative prodrug nanosystem also could be divided into a self-assembled system from prodrugs or a prodrug-encapsulated system by nanocarriers. These hybrid nanocarriers based on anticancer prodrugs could:155 (i) enhance the assembly stability of nanostructures and prolong blood circulation time and (ii) achieve targeting therapy (improving the uptake efficiency by tumor cells or release the functional drug in the specific site inside tumor cells). Since drugs are distinctive in physiochemical characteristics and mode of action, evaluating the specific contribution of each component in multifunctional nanoformulations in cancer therapy is of importance in order to optimize drug selection, drug ratio and to control the drug release of multiple drugs according to their molecular and pharmacodynamic mechanisms.14,154,156
Recently, according to the logical design and the specific function of each component, the rational combination of chemotherapeutics, PS and ICB drugs has shown a high-efficiency synergy in prodrug-based Nano-DDS.157,158 For example, as shown in Fig. 18, Choi et al. designed light-triggered chemodrug–photosensitizer conjugate–based NPs (LT-NPs) for reversing ITM into high immunogenic tumors to potentiate the antitumor efficacy of ICB. The conjugate of photosensitizer (verteporfin; VPF), DOX, and cathepsin B-specific cleavable peptide (FRRG) are prepared via a one-step amide bond reaction and can self-assemble into LT-NPs. Upon visible light irradiation, the LT-NPs specifically release VPF and DOX in cathepsin B-overexpressing cancer cells and induce effective ICD and maturation of DCs to stimulate cross presentation of cancer specific antigens to T cells in tumor models. Furthermore, the combination of iLT-NPs with PD-L1 blockade has shown strong immune responses and has considerably inhibited tumor growth, tumor recurrence, and lung metastasis.159
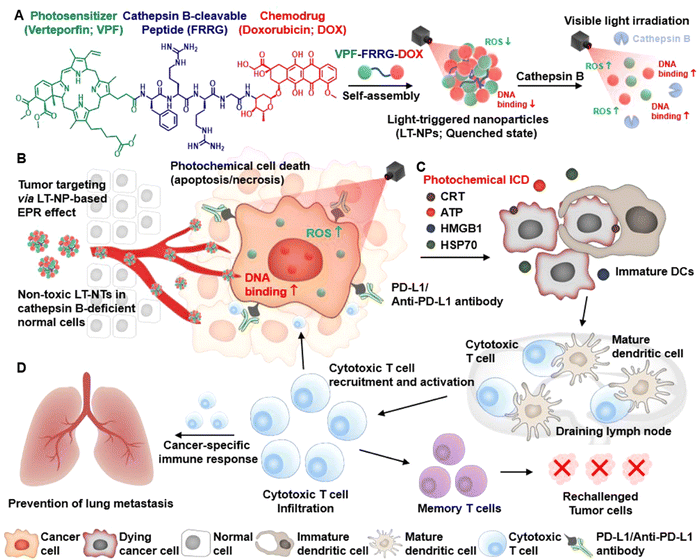 |
| Fig. 18 Schematic of light-triggered chemodrug–photosensitizer conjugate–based NPs (LT-NPs) to potentiate cancer ICB. (A) LT-NPs are prepared by self-assembly of cathepsin B-specific cleavable, light-trigged prodrug VPF-FRRG-DOX. (B) LT-NPs accumulated in tumor tissues specifically release VPF and DOX in cathepsin B-overexpressing cancer cells, generating ROS upon visible light irradiation. (C) Synergy of photochemotherapy by LT-NPs causes a strong ICD effect for dendritic cells (DCs) and cytotoxic T-cell activation. (D) Combinatorial LT-NP treatment with anti-PD-L1 antibody induces complete tumor regression of both primary tumors and distant pulmonary metastatic tumors by effective antitumor immune responses. Reproduced from reference 159 with permission from American Chemical Society, copyright 2021. | |
5. Summary and outlook
The past several years have witnessed the rapid development of nanomedicines. Approved by the FDA in 2005, the albumin bound PTX self-assembled nanoparticle nab-Paclitaxel (Abraxane®) is the most successful nanomedicine in the market utilized for cancer therapy at the current stage. The billion-dollar market achieved by Abraxane® has spurred researchers to further focus their efforts in this area.160,161 Considering the tumor is heterogeneous and evolutionary in divergent tumor types and distinct tumor development stages, the existing strategy is far from perfect. More rational and efficient Nano-DDS have to be exploited to facilitate the highly efficient delivery of anticancer agents in multiple cancer therapies. Prodrug-based nanomedicines can be decorated with any logical design and can be integrated with combinative therapeutic regimens for targeting multiple singling pathways.162
Despite the significant number of promising prodrug-based nanoformulations undergoing clinical development, a few formulations are expected to quickly enter the market for cancer therapy. The patents and clinical trials available related to prodrug-based nano-drugs for cancer therapy are discussed and reviewed in several papers.27,163 Nanoformulations self-assembled from both DHA–paclitaxel conjugate and poliglumex paclitaxel conjugate have been evaluated in phase III clinical studies, which has achieved minimal hypersensitivity reactions and improved therapeutic effect for cancer therapy.164,165 The accomplishments in clinical translation of those paclitaxel prodrug-based nanoformulations are supposed to threaten the position of Abraxane® in the marketing of cancer chemotherapy in the future. However, compared to the rapid FDA approval and the great achievement made by Abraxane®, those formulations struggle in marketing and clinical translation. Among them, insufficient improvements in pharmacokinetics while adding the preparation complexity might explain the unsatisfactory therapeutic outcomes and delayed industrialization of those prodrug-based nanomedicines. The more functionalities conferred to the nanocarriers, the greater difficulty that pharmaceutical development will face and the greater costs the patients will bear.
The great achievements of prodrug-based nanoparticulate drug delivery strategies in various cancer therapies have been presented in this review. The marketing potential and current barriers to clinical translation of this platform are also introduced. The clinical translation of prodrug nanomedicine will benefit from the effective communication between the medical care, academia, industry, and drug administration of government. Green and cost-effective chemistry is key to scale up the prodrug-NPs. Furthermore, developing preclinical mice models that recapitulate human cancers and conducting studies under the guideline of drug administrations will be helpful for generating reliable data sets for clinical translation of prodrug nanomedicine for cancer therapy.
Abbreviations
NPs | Nanoparticles |
ADCs | Antibody–drug conjugates |
nano-DDS | Nanodrug delivery system |
SMP | Small molecular prodrug |
PP | Polymer prodrug |
DHA | Docosahexaenoic acid |
CTX | Cabazitaxel |
SN38 | 7-Ethyl-10-hydroxy camptothecin |
TPNS | Taxanes prodrugs-based nanomedicines |
PDT | Photodynamic therapy |
PTT | Photothermal therapy |
PSs | Photosensitizers |
PTAs | Photothermal agents |
PEG | Polyethylene glycol |
PTX | Paclitaxel |
DTX | Docetaxel |
CPT | Camptothecin |
DOX | Doxorubicin |
Palb | Palbociclib |
POD | Podophyllotoxin |
BCPs | Block copolymers |
PPP | Pure prodrug platform |
CPP | Combinative prodrug platform |
CAs | Chemotherapeutic agents |
GSH | Glutathione |
FDA | The food and drug administration |
DHA | Docosahexaenoic acid |
Pt | Platinum |
Cys-PDSA | Cysteine-based poly-(disulfide amide) |
PLA | Poly(lactic acid) |
PLGA | Poly(lactic-co-glycolic acid) |
PEG-b-PCL | PEG-block-poly(ε-caprolactone) |
hCe6 | Hexadecylamine-conjugated chlorin e6 |
NIR | Near-infrared |
ALP | Alkaline phosphatase |
CES | Carboxylesterase |
Cyp-HCQ-Yp | (Cypate-Phe-Phe-Lys(SA-hydroxychloroquine)-Tyr(H2PO3)-OH) |
SMIs | Small molecular inhibitors |
TKI | Tyrosine kinases inhibitor |
DAS | Dasatinib |
EGFR | Epidermal growth factor receptor |
CEL | Celastrol |
NSCLC | Non-small cell lung cancer |
ICD | Immunogenic cell death |
APCs | Antigen-presenting cells |
TAAs | Tumor-associated antigens |
ER | Endoplasmic reticulum |
ICB | Immune checkpoint blockade |
NF-κB | Nuclear factor kappa B |
IDO | Indoleamine 2,3-dioxygenase |
IL-10 | Interleukin 10 |
CTLs | Cytotoxic T lymphocytes |
ITM | Immunosuppressive tumor microenvironment |
dLNs | Draining lymph nodes |
DCs | Dendritic cells |
PNE | Polycondensate neoepitope |
TLRa | Toll-like receptor agonists |
VPF | Verteporfin |
Author contributions
L. Shi and J. Zhang are responsible for the concept development, writing, revision, and review of the manuscript. S. Lin is responsible for collecting the reference for the manuscript. F. Zhou and H. Jiang are responsible for the collation of the manuscript. All authors contributed to the article and approved the submitted version.
Conflicts of interest
The authors declare no conflicts of interest.
Acknowledgements
This work is financially supported by the Zhejiang University Education Foundation (519400-11103), China.
References
- Q. Sun, Z. Zhou, N. Qiu and Y. Shen, Rational design of cancer nanomedicine: nanoproperty integration and synchronization, Adv. Mater., 2017, 29, 1606628 CrossRef PubMed
.
- Y. Kumar, A. Sinha, K. Nigam, D. Dwivedi and J. Sangwai, Functionalized nanoparticles: Tailoring properties through surface energetics and coordination chemistry for advanced biomedical applications, Nanoscale, 2023, 15, 6075–6104 RSC
.
- M. Lundqvist, J. Stigler, G. Elia, I. Lynch, T. Cedervall and A. Dawson, Nanoparticle size and surface properties determine the protein corona with possible implications for biological impacts, Proc. Natl. Acad. Sci. U. S. A., 2008, 105, 14265–14270 CrossRef CAS PubMed
.
- R. Cai, J. Ren, M. Guo, T. Wei, Y. Liu, C. Xie, P. Zhang, Z. Guo, A. Chetwynd, P. C. Ke, I. Lynch and C. Chen, Dynamic intracellular exchange of nanomaterials’ protein corona perturbs proteostasis and remodels cell metabolism, Proc. Natl. Acad. Sci. U. S. A., 2022, 119, e2200363119 CrossRef CAS PubMed
.
- Q. Zhou, J. Xiang, N. Qiu, Y. Wang, Y. Piao, S. Shao, J. Tang, Z. Zhou and Y. Shen, Tumor abnormality-oriented nanomedicine design, Chem. Rev., 2023, 123, 10920–10989 CrossRef CAS PubMed
.
- C. Jugo, E. Wojnilowicz, A. Tan and F. Caruso, A focus on “Bio” in bio-nanoscience: the impact of biological factors on nanomaterial interactions, Adv. Healthcare Mater., 2021, 10, e2100574 CrossRef PubMed
.
- J. Wang, Y. Li and G. Nie, Multifunctional biomolecule nanostructures for cancer therapy, Nat. Rev. Mater., 2021, 6, 766–783 CrossRef CAS PubMed
.
- D. Fan, Y. Cao, M. Cao, Y. Wang, Y. Cao and T. Gong, Nanomedicine in cancer therapy, Signal Transduction Targeted Ther., 2023, 8, 293 CrossRef PubMed
.
- C. Luo, J. Sun, B. Sun and Z. He, Prodrug-based nanoparticulate drug delivery strategies for cancer therapy, Trends Pharmacol. Sci., 2014, 35, 12–22 CrossRef PubMed
.
- M. Mitchell, M. Billingsley, R. Haley, M. Wechsler, N. Peppas and R. Langer, Engineering precision nanoparticles for drug delivery, Nat. Rev. Drug Discovery, 2021, 20, 101–124 CrossRef CAS PubMed
.
- T. Chien, T. Suydam and A. Woodrow, Prodrug approaches for the development of a long-acting drug delivery systems, Adv. Drug Delivery Rev., 2023, 198, 114860 CrossRef PubMed
.
- H. Wang, Z. Lu, L. Wang, T. Guo and J. Wu, New Generation nanomedicines constructed from self-assembling small-molecule prodrugs alleviate cancer drug toxicity, Cancer Res., 2017, 77, 6963–6974 CrossRef CAS PubMed
.
- G. Li, B. Sun, Y. Li, C. Luo, Z. He and J. Sun, Small-molecule prodrug nanoassemblies: an emerging nanoplatform for anticancer drug delivery, Small, 2021, 17, e2101460 CrossRef PubMed
.
- Q. Meng, H. Hu, L. Zhou, Y. Zhang, B. Yu, Y. Shen and H. Cong, Logical design and application of prodrug platforms, Polym. Chem., 2019, 10, 306–324 RSC
.
- R. Walther, J. Rautio and A. Zelikin, Prodrugs in medicinal chemistry and enzyme prodrug therapies, Adv. Drug Delivery Rev., 2017, 118, 65–77 CrossRef CAS PubMed
.
- X. Zhang, X. Li, Q. You and X. Zhang, Prodrug strategy for cancer cell-specific targeting: A recent overview, Eur. J. Med. Chem., 2017, 139, 542–563 CrossRef CAS PubMed
.
- C. Dumontet, J. Reichert, P. Senter, J. Lambert and A. Beck, Antibody-drug conjugates come of age in oncology, Nat. Rev. Drug Discovery, 2023, 22, 641–661 CrossRef CAS PubMed
.
- M. Satpathy, L. Wang, R. Zielinski, W. Qian, Y. Wang, A. Mohs, B. Kairdolf, X. Ji, J. Capala, M. Lipowska, S. Nie, H. Mao and L. Yang, Targeted drug delivery and image-guided therapy of heterogeneous ovarian cancer using HER2-targeted theranostic nanoparticles, Theranostics, 2019, 9, 778–795 CrossRef CAS PubMed
.
- X. Xia, X. Yang, W. Huang, X. Xia and D. Yan, Self-assembled nanomicelles of affibody-drug conjugate with excellent therapeutic property to cure ovary and breast cancers, Nano-Micro Lett., 2021, 14, 33 CrossRef PubMed
.
- Y. Zhang, H. Cui, R. Zhang, H. Zhang and W. Huang, Nanoparticulation of prodrug into medicines for cancer therapy, Adv. Sci., 2021, 8, e2101454 CrossRef PubMed
.
- Z. Deng and S. Liu, Controlled drug delivery with nanoassemblies of redox-responsive prodrug and polyprodrug amphiphiles, J. Controlled Release, 2020, 326, 276–296 CrossRef CAS PubMed
.
- Z. Wang, J. Chen, N. Little and J. Lu, Self-assembling prodrug nanotherapeutics for synergistic tumor targeted drug delivery, Acta Biomater., 2020, 111, 20–28 CrossRef CAS PubMed
.
- M. Lin, C. Hung, C. Hsu, Z. Lin and J. Fang, Prodrugs in combination with nanocarriers as a strategy for promoting antitumoral efficiency, Future Med. Chem., 2019, 11, 2131–2150 CrossRef CAS PubMed
.
- L. Shi, Y. Wang, Q. Wang, Z. Jiang, L. Ren, Y. Yan, Z. Liu, J. Wan, L. Huang, B. Cen, W. Han and H. Wang, Transforming a toxic drug into an efficacious nanomedicine using a lipoprodrug strategy for the treatment of patient-derived melanoma xenografts, J. Controlled Release, 2020, 324, 289–302 CrossRef CAS PubMed
.
- L. Shi, X. Wu, T. Li, Y. Wu, L. Song, W. Zhang, L. Yin, Y. Wu, W. Han and Y. Yang, An esterase-activatable prodrug formulated liposome strategy: potentiating the anticancer therapeutic efficacy and drug safety, Nanoscale Adv., 2022, 952–966 RSC
.
- L. Sun, P. Zhao, M. Chen, J. Leng, Y. Luan, B. Du, J. Yang, Y. Yang and R. Rong, Taxanes prodrug-based nanomedicines for cancer therapy, J. Controlled Release, 2022, 348, 672–691 CrossRef CAS PubMed
.
- K. Chen, A. Plaunt, F. Leifer, J. Kang and D. Cipolla, Recent advances in prodrug-based nanoparticle therapeutics, Eur. J. Pharm. Biopharm., 2021, 165, 219–243 CrossRef CAS PubMed
.
- B. Yang, J. Gao, Q. Pei, H. Xu and H. Yu, Engineering prodrug nanomedicine for cancer immunotherapy, Adv. Sci., 2020, 7, 2002365 CrossRef CAS PubMed
.
- Z. Liu, W. Jiang, J. Nam, J. Moon and B. Kim, Immunomodulating nanomedicine for cancer therapy, Nano Lett., 2018, 18, 6655–6659 CrossRef CAS PubMed
.
- S. Li, X. Shan, Y. Wang, Q. Chen, J. Sun, Z. He, B. Sun and C. Luo, Dimeric prodrug-based nanomedicines for cancer therapy, J. Controlled Release, 2020, 326, 510–522 CrossRef CAS PubMed
.
- Y. Zhang, Q. Pei, Y. Yue and Z. Xie, Binary dimeric prodrug nanoparticles for self-boosted drug release and synergistic chemo-photodynamic therapy, J. Mater. Chem. B, 2022, 10, 880–886 RSC
.
- L. Huang, X. Chen, Q. Bian, F. Zhang, H. Wu, H. Wang and J. Gao, Photosensitizer-stabilized self-assembling nanoparticles potentiate chemo/photodynamic efficacy of patient-derived melanoma, J. Controlled Release, 2020, 328, 325–338 CrossRef CAS PubMed
.
- Z. Huang, H. Hu, T. Xian, Z. Xu, D. Tang, B. Wang and Y. Zhang, Carrier-free nanomedicines self-assembled from palbociclib dimers and Ce6 for enhanced combined chemo-photodynamic therapy of breast cancer, RSC Adv., 2023, 13, 1617–1626 RSC
.
- A. Cheetham, R. Chakroun, W. Ma and H. Cui, Self-assembling prodrugs, Chem. Soc. Rev., 2017, 46, 6638–6663 RSC
.
- H. Li, W. Zang, Z. Mi, J. Li, L. Wang, D. Xie, L. Zhao and D. Wang, Tailoring carrier-free nanocombo of small-molecule prodrug for combinational cancer therapy, J. Controlled Release, 2022, 352, 256–275 CrossRef CAS PubMed
.
- H. Wang, J. Chen, C. Xu, L. Shi, M. Tayier, J. Zhou, J. Zhang, J. Wu, Z. Ye, T. Fang and W. Han, Cancer nanomedicines stabilized by π–π stacking between heterodimeric prodrugs enable exceptionally high drug loading capacity and safer delivery of drug combinations, Theranostics, 2017, 7, 3638–3652 CrossRef CAS PubMed
.
- B. Sun, C. Luo, X. Zhang, M. Guo, M. Sun, H. Yu, Q. Chen, W. Yang, M. Wang, S. Zuo, P. Chen, Q. Kan, H. Zhang, Y. Wang, Z. He and J. Sun, Probing the impact of sulfur/selenium/carbon linkages on prodrug nanoassemblies for cancer therapy, Nat. Commun., 2019, 10, 3211 CrossRef PubMed
.
- C. Luo, J. Sun, B. Sun, D. Liu, L. Miao, T. Goodwin, L. Huang and Z. He, Facile fabrication of tumor redox-sensitive nanoassemblies of small-molecule oleate prodrug as potent chemotherapeutic nanomedicine, Small, 2016, 6353–6362 CrossRef CAS PubMed
.
- Z. Wang, M. Zhuang, T. Sun, X. Wang and Z. Xie, Self-assembly of glutamic acid linked paclitaxel dimers into nanoparticles for chemotherapy, Bioorg. Med. Chem. Lett., 2017, 2493–2496 CrossRef CAS PubMed
.
- R. Xia, Q. Pei, J. Wang, Z. Wang, X. Hu and Z. Xie, Redox responsive paclitaxel dimer for programmed drug release and selectively killing cancer cells, J. Colloid Interface Sci., 2020, 580, 785–793 CrossRef CAS PubMed
.
- Q. Pei, X. Hu, S. Liu, Y. Li, Z. Xie and X. Jing, Paclitaxel dimers assembling nanomedicines for treatment of cervix carcinoma, J. Controlled Release, 2017, 254, 23–33 CrossRef CAS PubMed
.
- L. Zhou, H. Xie, X. Chen, J. Wan, S. Xu, Y. Han, D. Chen, Y. Qiao, L. Zhou, S. Zheng and H. Wang, Dimerization-induced self-assembly of a redox-responsive prodrug into nanoparticles for improved therapeutic index, Acta Biomater., 2020, 113, 464–477 CrossRef CAS PubMed
.
- L. Ren, S. Ren, L. Shu, Z. Wang, K. Shi, W. Han and H. Wang, Nanodelivery of a self-assembling prodrug with exceptionally high drug loading potentiates chemotherapy efficacy, Int. J. Pharm., 2021, 605, 120805 CrossRef CAS PubMed
.
- S. Zhang, Z. Wang, Z. Kong, Y. Wang, X. Zhang, B. Sun, H. Zhang, Q. Kan, Z. He, C. Luo and J. Sun, Photosensitizer-driven nanoassemblies of homodimeric prodrug for self-enhancing activation and synergistic chemo-photodynamic therapy, Theranostics, 2021, 11, 6019–6032 CrossRef CAS PubMed
.
- L. Li, S. Zuo, F. Dong, T. Liu, Y. Gao, Y. Yang, X. Wang, J. Sun, B. Sun and Z. He, Small changes in the length of diselenide bond-containing linkages exert great influences on the antitumor activity of docetaxel homodimeric prodrug nanoassemblies, Asian J. Pharm. Sci., 2021, 16, 337–349 CrossRef PubMed
.
- T. Liu, L. Li, S. Wang, F. Dong, S. Zuo, J. Song, X. Wang, Q. Lu, H. Wang, H. Zhang, M. Cheng, X. Liu, Z. He, B. Sun and J. Sun, Hybrid chalcogen bonds in prodrug nanoassemblies provides dual redox-responsivity in the tumor microenvironment, Nat. Commun., 2022, 13, 7228 CrossRef CAS PubMed
.
- H. Kasai, T. Murakami, Y. Ikuta, Y. Koseki, K. Baba, H. Oikawa, H. Nakanishi, M. Okada, M. Shoji, M. Ueda, H. Imahori and M. Hashida, Creation of pure nanodrugs and their anticancer properties, Angew. Chem., Int. Ed., 2012, 51, 10315–10318 CrossRef CAS PubMed
.
- Q. Pei, X. Hu, Z. Li, Z. Xie and X. Jing, Small molecular nanomedicines made from a camptothecin dimer containing a disulfide bond, RSC Adv., 2015, 5, 81499–81501 RSC
.
- Y. Yang, B. Sun, S. Zuo, X. Li, S. Zhou, L. Li, C. Luo, H. Liu, M. Cheng, Y. Wang, S. Wang, Z. He and J. Sun, Trisulfide bond-mediated doxorubicin dimeric prodrug nanoassemblies with high drug loading, high self-assembly stability, and high tumor selectivity, Sci. Adv., 2020, 6, eabc1725 CrossRef CAS PubMed
.
- R. Luo, Z. Zhang, L. Han, Z. Xue, K. Zhang, F. Liu, F. Feng, J. Xue, W. Liu and W. Qu, An albumin-binding dimeric prodrug nanoparticle with long blood circulation and light-triggered drug release for chemo-photodynamic combination therapy against hypoxia-induced metastasis of lung cancer, Biomater. Sci, 2021, 9, 3718–3736 RSC
.
- B. Liang and D. Zhou, ROS-activated homodimeric podophyllotoxin nanomedicine with self-accelerating drug release for efficient cancer eradication, Drug Delivery, 2021, 28, 2361–2372 CrossRef CAS PubMed
.
- D. Mei, L. Gong, Y. Zou, D. Yang, H. Liu, Y. Liang, N. Sun, L. Zhao, Q. Zhang and Z. Lin, Platelet membrane-cloaked paclitaxel-nanocrystals augment postoperative chemotherapeutical efficacy, J. Controlled Release, 2020, 324, 341–353 CrossRef CAS PubMed
.
- Z. Edis, J. Wang, M. Waqas, M. Ijaz and M. Ijaz, Nanocarriers-mediated drug delivery systems for anticancer agents: an overview and perspectives, Int. J. Nanomed., 2021, 16, 1313–1330 CrossRef PubMed
.
- S. Khizar, N. Alrushaid, F. Khan, N. Zine, N. Renault, A. Errachid and A. Elaissari, Nanocarriers based novel and effective drug delivery system, Int. J. Pharm., 2023, 632, 122570 CrossRef CAS PubMed
.
- J. Majumder, O. Taratula and T. Minko, Nanocarrier-based systems for targeted and site specific therapeutic delivery, Adv. Drug Delivery Rev., 2019, 144, 57–77 CrossRef CAS PubMed
.
- N. Kaushik, S. Borkar, S. Nandanwar, P. Panda, E. Choi and N. Kaushik, Nanocarrier cancer therapeutics with functional stimuli-responsive mechanisms, J. Nanobiotechnol., 2022, 20, 152 CrossRef CAS PubMed
.
- M. Amaldoss, J. Yang, P. Koshy, A. Unnikrishnan and C. Sorrell, Inorganic nanoparticle-based advanced cancer therapies: promising combination strategies, Drug Discovery Today, 2022, 27, 103386 CrossRef CAS PubMed
.
- C. Núñez, S. Estévez and M. Chantada, Inorganic nanoparticles in diagnosis and treatment of breast cancer, J. Biol. Inorg. Chem., 2018, 23, 331–345 CrossRef PubMed
.
- B. Sekhon and S. Kamboj, Inorganic nanomedicine-part 2, Nanomedicine, 2010, 6, 612–618 CrossRef CAS PubMed
.
- V. Delplace, P. Couvreur and J. Nicolas, Recent trends in the design of anticancer polymer prodrug nanocarriers, Polym. Chem., 2014, 5, 1529–1544 RSC
.
- L. Repp, M. Rasoulianboroujeni, H. Lee and G. Kwon, Acyl and oligo(lactic acid) prodrugs for PEG-b-PLA and PEG-b-PCL nano-assemblies for injection, J. Controlled Release, 2021, 330, 1004–1015 CrossRef CAS PubMed
.
- Y. Zhang, P. He, P. Zhang, X. Yi, C. Xiao and X. Chen, Polypeptides-drug conjugates for anticancer therapy, Adv. Healthcare Mater., 2021, 10, e2001974 CrossRef PubMed
.
- S. Robla, M. Alonso and N. Csaba, Polyaminoacid-based nanocarriers: a review of the latest candidates for oral drug delivery, Expert Opin. Drug Delivery, 2020, 17, 1081–1092 CrossRef CAS PubMed
.
- A. Plucinski, Z. Lyu and B. Schmidt, Polysaccharide nanoparticles: from fabrication to applications, J. Mater. Chem. B, 2021, 9, 7030–7062 RSC
.
- S. Senevirathne, K. Washington, M. Biewer and M. Stefan, PEG based anti-cancer drug conjugated prodrug micelles for the delivery of anti-cancer agents, J. Mater. Chem. B, 2016, 4, 360–370 RSC
.
- A. Souza and R. Shegokar, Polyethylene glycol (PEG): a versatile polymer for pharmaceutical applications, Expert Opin. Drug Delivery, 2016, 13, 1257–1275 CrossRef PubMed
.
- X. Dong, R. Brahma, C. Fang and S. Yao, Stimulus-responsive self-assembled prodrugs in cancer therapy, Chem. Sci., 2022, 13, 4239–4269 RSC
.
- A. Nguyen, R. Böttger and S. Li, Recent trends in bioresponsive linker technologies of prodrug-based Self-Assembling nanomaterials, Biomaterials, 2021, 275, 120955 CrossRef CAS PubMed
.
- D. Dutta, W. Ke, L. Xi, W. Yin, M. Zhou and Z. Ge, Block copolymer prodrugs: synthesis, self-assembly, and applications for cancer therapy, Wiley Interdiscip. Rev.: Nanomed. Nanobiotechnol., 2020, 12, e1585 CAS
.
- W. Han, L. Shi, B. Xie, J. Wan, L. Ren, Y. Wang, X. Chen and H. Wang, Supramolecular engineering of molecular inhibitors in an adaptive cytotoxic nanoparticle for synergistic cancer therapy, ACS Appl. Mater. Interfaces, 2020, 12, 1707–1720 CrossRef CAS PubMed
.
- C. Tilsed, S. Fisher, A. Nowak, R. Lake and W. Lesterhuis, Cancer chemotherapy: insights into cellular and tumor microenvironmental mechanisms of action, Front. Oncol., 2022, 12, 960317 CrossRef CAS PubMed
.
- G. Wei, Y. Wang, G. Yang, Y. Wang and R. Ju, Recent progress in nanomedicine for enhanced cancer chemotherapy, Theranostics, 2021, 11, 6370–6392 CrossRef CAS PubMed
.
- F. Kratz, I. Muller, C. Ryppa and A. Warnecke, Prodrug strategies in anticancer chemotherapy, ChemMedChem, 2008, 3, 20–53 CrossRef CAS PubMed
.
- S. Mura, D. Bui, P. Couvreur and J. Nicolas, Lipid prodrug nanocarriers in cancer therapy, J. Controlled Release, 2015, 208, 25–41 CrossRef CAS PubMed
.
- D. Irby, C. Du and F. Li, Lipid-drug conjugate for enhancing drug delivery, Mol. Pharmaceutics, 2017, 14, 1325–1338 CrossRef CAS PubMed
.
- B. Sun, C. Luo, W. Cui, J. Sun and Z. He, Chemotherapy agent-unsaturated fatty acid prodrugs and prodrug-nanoplatforms for cancer chemotherapy, J. Controlled Release, 2017, 264, 145–159 CrossRef CAS PubMed
.
- X. Chen, L. Cui, J. Xu, S. Xian, F. Meng, C. Zhan and H. Wang, De novo engineering of both an omega-3 fatty acid-derived nanocarrier host and a prodrug guest to potentiate drug efficacy against colorectal malignancies, Biomaterials, 2022, 290, 121814 CrossRef CAS PubMed
.
- T. Boztepe, G. Castro and I. León, Lipid, polymeric, inorganic-based drug delivery applications for platinum-based anticancer drugs, Int. J. Pharm., 2021, 605, 120788 CrossRef CAS PubMed
.
- S. Dhar, F. Gu, R. Langer, O. Farokhzad and S. Lippard, Targeted delivery of cisplatin to prostate cancer cells by aptamer functionalized Pt(IV) prodrug-PLGA-PEG nanoparticles, Proc. Natl. Acad. Sci. U. S. A., 2008, 105, 17356–17361 CrossRef CAS PubMed
.
- S. Dhar, N. Kolishetti, S. Lippard and O. Farokhzad, Targeted delivery of a cisplatin prodrug for safer and more effective prostate cancer therapy in vivo, Proc. Natl. Acad. Sci. U. S. A., 2011, 108, 1850–1855 CrossRef CAS PubMed
.
- X. Ling, X. Chen, I. Riddell, W. Tao, J. Wang, G. Hollett, S. Lippard, O. Farokhzad, J. Shi and J. Wu, Glutathione-scavenging poly(disulfide amide) nanoparticles for the effective delivery of PT(IV) prodrugs and reversal of cisplatin resistance, Nano Lett., 2018, 18, 4618–4625 CrossRef CAS PubMed
.
- J. Nicolas and P. Couvreur, Polymer nanoparticles for the delivery of anticancer drug, Med. Sci., 2017, 33, 11–17 Search PubMed
.
- Y. Tam, D. Shin, K. Chen and G. Kwon, Poly(ethylene glycol)-block-poly(d,l-lactic acid) micelles containing oligo(lactic acid)(8)-paclitaxel prodrug: In vivo conversion and antitumor efficacy, J. Controlled Release, 2019, 298, 186–193 CrossRef CAS PubMed
.
- Y. Dong, P. Du, M. Pei and P. Liu, Design, postpolymerization conjugation and self-assembly of a di-block copolymer-based prodrug for tumor intracellular acid-triggered DOX release, J. Mater. Chem. B, 2019, 7, 5640–5647 RSC
.
- W. Ke, W. Yin, Z. Zha, J. Mukerabigwi, W. Chen, Y. Wang, C. He and Z. Ge, A robust strategy for preparation of sequential stimuli-responsive block copolymer prodrugs via thiolactone chemistry to overcome multiple anticancer drug delivery barriers, Biomaterials, 2018, 154, 261–274 CrossRef CAS PubMed
.
- X. Hu, J. Hu, T. Jie, Z. Ge, G. Zhang, K. Luo and S. Liu, Polyprodrug amphiphiles: hierarchical assemblies for shape-regulated cellular internalization, trafficking, and drug delivery, J. Am. Chem. Soc., 2013, 135, 17617–17629 CrossRef CAS PubMed
.
- K. Xie, S. Song, L. Zhou, J. Wan, Y. Qiao, M. Wang, H. Xie, L. Zhou, S. Zheng and H. Wang, Revival of a potent therapeutic maytansinoid agent using a strategy that combines covalent drug conjugation with sequential nanoparticle assembly, Int. J. Pharm., 2019, 556, 159–171 CrossRef CAS PubMed
.
- J. Wan, L. Huang, J. Cheng, H. Qi, J. Jin and H. Wang, Balancing the stability and drug activation in adaptive nanoparticles potentiates chemotherapy in multidrug-resistant cancer, Theranostics, 2021, 11, 4137–4154 CrossRef CAS PubMed
.
- H. Wang, L. Zhou, K. Xie, J. Wu, P. Song, H. Xie, L. Zhou, J. Liu, X. Xu, Y. Shen and S. Zheng, Polylactide-tethered prodrugs in polymeric nanoparticles as reliable nanomedicines for the efficient eradication of patient-derived hepatocellular carcinoma, Theranostics, 2018, 8, 3949–3963 CrossRef CAS PubMed
.
- Y. Wang, H. Xie, K. Ying, B. Xie and H. Wang, Tuning the efficacy of esterase-activatable prodrug nanoparticles for the treatment of colorectal malignancies, Biomaterials, 2021, 120705 CrossRef CAS PubMed
.
- X. Li, J. Lovell, J. Yoon and X. Chen, Clinical development and potential of photothermal and photodynamic therapies for cancer, Nat. Rev. Clin. Oncol., 2020, 17, 657–674 CrossRef PubMed
.
- M. Kolarikova, B. Hosikova, H. Dilenko, K. Barton, L. Valkova, R. Bajgar, L. Malina and H. Kolarova, Photodynamic therapy: innovative approaches for antibacterial and anticancer treatments, Med. Res. Rev., 2023, 43, 717–774 CrossRef CAS PubMed
.
- D. Zhi, T. Yang, J. Hagan, S. Zhang and R. Donnelly, Photothermal therapy, J. Controlled Release, 2020, 325, 52–71 CrossRef CAS PubMed
.
- F. Lu, R. Sang, Y. Tang, H. Xia, J. Liu, W. Huang, Q. Fan and Q. Wang, Fabrication of a phototheranostic nanoplatform for single laser-triggered NIR-II fluorescence imaging-guided photothermal/chemo/antiangiogenic combination therapy, Acta Biomater., 2022, 151, 528–536 CrossRef CAS PubMed
.
- L. Huang, J. Wan, H. Wu, X. Chen, Q. Bian, L. Shi, X. Jiang, A. Yuan, J. Gao and H. Wang, Quantitative self-assembly of photoactivatable small molecular prodrug cocktails for safe and potent cancer chemo-photodynamic therapy, Nano Today, 2021, 36, 101030 CrossRef CAS
.
- Y. Pan, L. Liu, Y. He, L. Ye, X. Zhao, Z. Hu, X. Mou and Y. Cai, NIR diagnostic imaging of triple-negative breast cancer and its lymph node metastasis for high-efficiency hypoxia-activated multimodal therapy, J. Nanobiotechnol., 2023, 21, 312 CrossRef CAS PubMed
.
- S. Zhou, X. Hu, R. Xia, S. Liu, Q. Pei, G. Chen, Z. Xie, X. Jing and A. Paclitaxel, prodrug activatable by irradiation in a hypoxic microenvironment, Angew. Chem., Int. Ed., 2020, 59, 23198–23205 CrossRef CAS PubMed
.
- L. Feng, L. Cheng, Z. Dong, D. Tao, T. Barnhart, W. Cai, M. Chen and Z. Liu, Theranostic liposomes with hypoxia-activated prodrug to effectively destruct hypoxic tumors post-photodynamic therapy, ACS Nano, 2017, 11, 927–937 CrossRef CAS PubMed
.
- D. Zhao, W. Tao, S. Li, L. Li, Y. Sun, G. Li, G. Wang, Y. Wang, B. Lin and C. Luo, Light-triggered dual-modality drug release of self-assembled prodrug-nanoparticles for synergistic photodynamic and hypoxia-activated therapy, Nanoscale Horiz., 2020, 5, 886–894 RSC
.
- M. Zhang, W. Wang, M. Mohammadniaei, T. Zheng, Q. Zhang, J. Ashley, S. Liu, Y. Sun and B. Tang, Upregulating aggregation-induced-emission nanoparticles with blood-tumor-barrier permeability for precise photothermal eradication of brain tumors and induction of local immune responses, Adv. Mater., 2021, 33, e2008802 CrossRef PubMed
.
- S. Alamdari, M. Amini, N. Jalilzadeh, B. Baradaran, R. Mohammadzadeh, A. Mokhtarzadeh and F. Oroojalian, Recent advances in nanoparticle-based photothermal therapy for breast cancer, J. Controlled Release, 2022, 349, 269–303 CrossRef CAS PubMed
.
- J. Xia, X. Qing, J. Shen, M. Ding, Y. Wang, N. Yu, J. Li and X. Wang, Enzyme-loaded ph-sensitive photothermal hydrogels for mild-temperature-mediated combinational cancer therapy, Front. Chem., 2021, 9, 736468 CrossRef CAS PubMed
.
- X. Li, T. Yong, Z. Wei, N. Bie, X. Zhang, G. Zhan, J. Li, J. Qin, J. Yu, B. Zhang, L. Gan and X. Yang, Reversing insufficient photothermal therapy-induced tumor relapse and metastasis by regulating cancer-associated fibroblasts, Nat. Commun., 2022, 13, 2794 CrossRef CAS PubMed
.
- P. Wang, B. Chen, Y. Zhan, L. Wang, J. Luo, J. Xu, L. Zhan, Z. Li, Y. Liu and J. Wei, Enhancing the efficiency of mild-temperature photothermal therapy for cancer assisting with various strategies, Pharmaceutics, 2022, 14, 2279 CrossRef CAS PubMed
.
- G. Gao, X. Sun, X. Liu, Y. Jiang, R. Tang, Y. Guo, F. Wu and G. Liang, Intracellular nanoparticle formation and hydroxychloroquine release for autophagy-inhibited mild-temperature photothermal therapy for tumors, Adv. Funct. Mater., 2021, 31, 2102832 CrossRef CAS
.
- H. Wang, Z. Wang, W. Chen, W. Wang, W. Shi, J. Chen, Y. Hang, J. Song, X. Xiao and Z. Dai, Self-assembly of photosensitive and radiotherapeutic peptide for combined photodynamic-radio cancer therapy with intracellular delivery of miRNA-139-5p, Bioorg. Med. Chem., 2021, 44, 116305 CrossRef CAS PubMed
.
- K. Cheng, J. Qi, J. Zhang, H. Li, X. Ren, W. Wei, L. Meng, J. Li, Q. Li, H. Zhang, W. Deng, H. Sun and L. Mei, Self-assembled nano-photosensitizer for targeted, activatable, and biosafe cancer phototheranostics, Biomaterials, 2022, 291, 121916 CrossRef CAS PubMed
.
- H. Li, L. Yue, M. Wu and F. Wu, Self-assembly of methylene violet-conjugated perylene diimide with photodynamic/photothermal properties for DNA photocleavage and cancer treatment, Colloids Surf., B, 2020, 196, 111351 CrossRef CAS PubMed
.
- P. Bedard, D. Hyman, M. Davids and L. Siu, Small molecules, big impact: 20 years of targeted therapy in oncology, Lancet, 2020, 395, 1078–1088 CrossRef CAS PubMed
.
- Y. Yang, S. Li, Y. Wang, Y. Zhao and Q. Li, Protein tyrosine kinase inhibitor resistance in malignant tumors: molecular mechanisms and future perspective, Signal Transduction Targeted Ther., 2022, 7, 329 CrossRef CAS PubMed
.
- S. Salmaso, F. Mastrotto, M. Roverso, V. Gandin, S. De Martin, D. Gabbia, M. Franco, C. Vaccarin, M. Verona, A. Chilin, P. Caliceti, S. Bogialli and G. Marzaro, Tyrosine kinase inhibitor prodrug-loaded liposomes for controlled release at tumor microenvironment, J. Controlled Release, 2021, 340, 318–330 CrossRef CAS PubMed
.
- J. Sun, Y. Liu, Y. Chen, W. Zhao, Q. Zhai, S. Rathod, Y. Huang, S. Tang, Y. Kwon, C. Fernandez, R. Venkataramanan and S. Li, Doxorubicin delivered by a redox-responsive dasatinib-containing polymeric prodrug carrier for combination therapy, J. Controlled Release, 2017, 258, 43–55 CrossRef CAS PubMed
.
- X. Xie, C. Zhan, J. Wang, F. Zeng and S. Wu, An Activatable Nano-Prodrug for treating tyrosine-kinase-inhibitor-resistant non-small cell lung cancer and for optoacoustic and fluorescent imaging, Small, 2020, 16, 2003451 CrossRef CAS PubMed
.
- J. Martin, H. Cabral, T. Stylianopoulos and R. Jain, Improving cancer immunotherapy using nanomedicines: progress, opportunities and challenges, Nat. Rev. Clin. Oncol., 2020, 17, 251–266 CrossRef PubMed
.
- Q. Sun, M. Barz, B. Geest, M. Diken, W. Hennink, F. Kiessling, T. Lammers and Y. Shi, Nanomedicine and macroscale materials in immuno-oncology, Chem. Soc. Rev., 2019, 48, 351–381 RSC
.
- X. Duan, C. Chan and W. Lin, Nanoparticle-Mediated Immunogenic cell death enables and potentiates cancer immunotherapy, Angew. Chem., Int. Ed., 2019, 58, 670–680 CrossRef CAS PubMed
.
- Z. Li, X. Lai, S. Fu, L. Ren, H. Cai, H. Zhang, Z. Gu, X. Ma and K. Luo, Immunogenic cell death activates the tumor immune microenvironment to boost the immunotherapy efficiency, Adv. Sci., 2022, 9, e2201734 CrossRef PubMed
.
- A. Ahmed and S. Tait, Targeting immunogenic cell death in cancer, Mol. Oncol., 2020, 14, 2994–3006 CrossRef CAS PubMed
.
- L. Galluzzi, I. Vitale, S. Warren, S. Adjemian, P. Agostinis, A. Martinez, T. Chan, G. Coukos, S. Demaria, E. Deutsch, D. Draganov, R. Edelson, S. Formenti, J. Fucikova, L. Gabriele, U. S. Gaipl, S. Gameiro, A. Garg, E. Golden, J. Han, K. Harrington, A. Hemminki, J. Hodge, D. Hossain, T. Illidge, M. Karin, H. Kaufman, O. Kepp, G. Kroemer, J. Lasarte, S. Loi, M. Lotze, G. Manic, T. Merghoub, A. Melcher, K. Mossman, F. Prosper, Ø. Rekdal, M. Rescigno, C. Riganti, A. Sistigu, M. Smyth, R. Spisek, J. Stagg, B. Strauss, D. Tang, K. Tatsuno, S. van Gool, P. Vandenabeele, T. Yamazaki, D. Zamarin, L. Zitvogel, A. Cesano and F. Marincola, Consensus guidelines for the definition, detection and interpretation of immunogenic cell death, J. Immunother. Cancer, 2020, 8, e00033 Search PubMed
.
- Q. Chen, L. Liu, Y. Lu, X. Chen, Y. Zhang, W. Zhou, Q. Guo, C. Li, Y. Zhang, Y. Zhang, D. Liang, T. Sun and C. Jiang, Tumor Microenvironment-triggered aggregated magnetic nanoparticles for reinforced image-guided immunogenic chemotherapy, Adv. Sci., 2019, 6, 1802134 CrossRef PubMed
.
- B. Li, H. Chan and P. Chen, Immune checkpoint inhibitors: basics and challenges, Curr. Med. Chem., 2019, 26, 3009–3025 CrossRef CAS PubMed
.
- J. Wang, T. Yang and J. Xu, Therapeutic development of immune checkpoint inhibitors, Adv. Exp. Med. Biol., 2020, 1248, 619–649 CrossRef CAS PubMed
.
- D. Palmieri and M. Carlino, Immune checkpoint inhibitor toxicity, Curr. Oncol. Rep., 2018, 20, 72 CrossRef PubMed
.
- M. Postow, R. Sidlow and M. Hellmann, Immune-related adverse events associated with immune checkpoint blockade, N. Engl. J. Med., 2018, 378, 158–168 CrossRef CAS PubMed
.
- K. Autio, V. Boni, R. Humphrey and A. Naing, Probody therapeutics: an emerging class of therapies designed to enhance on-target effects with reduced off-tumor toxicity for use in immuno-oncology, Clin. Cancer Res., 2020, 26, 984–989 CrossRef CAS PubMed
.
- S. Picardo, J. Doi and A. Hansen, Structure and optimization of checkpoint inhibitors, Cancers, 2019, 12, 38 CrossRef PubMed
.
- J. Bu, A. Nair, M. Iida, W. Jeong, M. Poellmann, K. Mudd, L. Kubiatowicz, E. Liu, D. Wheeler and S. Hong, An avidity-based PD-L1 antagonist using nanoparticle-antibody conjugates for enhanced immunotherapy, Nano Lett., 2020, 20, 4901–4909 CrossRef CAS PubMed
.
- P. Zhang, J. Miska, C. Lee-Chang, A. Rashidi, W. Panek, S. An, M. Zannikou, A. Lopez-Rosas, Y. Han, T. Xiao, K. Pituch, D. Kanojia, I. Balyasnikova and M. Lesniak, Therapeutic targeting of tumor-associated myeloid cells synergizes with radiation therapy for glioblastoma, Proc. Natl. Acad. Sci. U. S. A., 2019, 116, 23714 CrossRef CAS PubMed
.
- D. Schmid, C. Park, C. Hartl, N. Subedi, A. Cartwright, R. Puerto, Y. Zheng, J. Maiarana, G. Freeman, K. Wucherpfennig, D. Irvine and M. Goldberg, T cell-targeting nanoparticles focus delivery of immunotherapy to improve antitumor immunity, Nat. Commun., 2017, 8, 1747 CrossRef PubMed
.
- Z. Xiao, Z. Su, S. Han, J. Huang, L. Lin and X. Shuai, Dual pH-sensitive nanodrug blocks PD-1 immune checkpoint and uses T cells to deliver NF-κB inhibitor for antitumor immunotherapy, Sci. Adv., 2020, 6, eaay7785 CrossRef CAS PubMed
.
- Y. Guo, Y. Liu, W. Wu, D. Ling, Q. Zhang, P. Zhao and X. Hu, Indoleamine 2,3-dioxygenase (Ido) inhibitors and their nanomedicines for cancer immunotherapy, Biomaterials, 2021, 276, 121018 CrossRef CAS PubMed
.
- M. Saraiva and A. Garra, The regulation of IL-10 production by immune cells, Nat. Rev. Immunol., 2010, 10, 170–181 CrossRef CAS PubMed
.
- H. Lee, Recent advances in the development of TGF-β signaling inhibitors for anticancer therapy, J. Cancer Prev., 2020, 25, 213–222 CrossRef PubMed
.
- L. Liu, H. Guo, A. Song, J. Huang, Y. Zhang, S. Jin, S. Li, L. Zhang, C. Yang and P. Yang, Progranulin inhibits LPS-induced macrophage M1 polarization via NF-κB and MAPK pathways, BMC Immunol., 2020, 21, 32 CrossRef CAS PubMed
.
- M. Logtenberg, F. Scheeren and T. Schumacher, The CD47-SIRPα immune checkpoint, Immunity, 2020, 52, 742–752 CrossRef CAS PubMed
.
- B. Feng, F. Zhou, B. Hou, D. Wang, T. Wang, Y. Fu, Y. Ma, H. Yu and Y. Li, Binary cooperative prodrug nanoparticles improve immunotherapy by synergistically modulating immune tumor microenvironment, Adv. Mater., 2018, 30, e1803001 CrossRef PubMed
.
- X. Han, K. Cheng, Y. Xu, Y. Wang, H. Min, Y. Zhang, X. Zhao, R. Zhao, G. Anderson, L. Ren, G. Nie and Y. Li, Modularly designed peptide nanoprodrug augments antitumor immunity of PD-L1 checkpoint blockade by targeting indoleamine 2,3-Dioxygenase, J. Am. Chem. Soc., 2020, 142, 2490–2496 CrossRef CAS PubMed
.
- M. Peng, Y. Mo, Y. Wang, P. Wu, Y. Zhang, F. Xiong, C. Guo, X. Wu, Y. Li, X. Li, G. Li, W. Xiong and Z. Zeng, Neoantigen vaccine: an emerging tumor immunotherapy, Mol. Cancer, 2019, 18, 128 CrossRef PubMed
.
- H. Wang and D. J. Mooney, Biomaterial-assisted targeted modulation of immune cells in cancer treatment, Nat. Mater., 2018, 17, 761–772 CrossRef CAS PubMed
.
- M. Morse, W. Gwin and D. Mitchell, Vaccine therapies for cancer: then and now, Target. Oncol., 2021, 16, 121–152 CrossRef PubMed
.
- H. Wu, X. Fu, Y. Zhai, S. Gao, X. Yang and G. Zhai, Development of effective tumor vaccine strategies based on immune response cascade reactions, Adv. Healthcare Mater., 2021, 10, e2100299 CrossRef PubMed
.
- R. Kuai, L. Ochyl, K. Bahjat, A. Schwendeman and J. Moon, Designer vaccine nanodiscs for personalized cancer immunotherapy, Nat. Mater., 2017, 16, 489–496 CrossRef CAS PubMed
.
- M. Saxena, S. van der Burg, C. Melief and N. Bhardwaj, Therapeutic cancer vaccines, Nat. Rev. Cancer, 2021, 21, 360–378 CrossRef CAS PubMed
.
- L. Wei, Y. Zhao, X. Hu and L. Tang, Redox-responsive polycondensate neoepitope for enhanced personalized cancer vaccine, ACS Cent. Sci., 2020, 6, 404–412 CrossRef CAS PubMed
.
- W. Lee and M. Suresh, Vaccine adjuvants to engage the cross-presentation pathway, Front. Immunol., 2022, 13, 940047 CrossRef CAS PubMed
.
- F. Steinhagen, T. Kinjo, C. Bode and D. Klinman, TLR-based immune adjuvants, Vaccine, 2011, 29, 3341–3355 CrossRef CAS PubMed
.
- C. Bode, G. Zhao, F. Steinhagen, T. Kinjo and D. Klinman, CpG DNA as a vaccine adjuvant, Expert Rev. Vaccines, 2011, 10, 499–511 CrossRef CAS PubMed
.
- H. Liu, K. Moynihan, Y. Zheng, G. Szeto, A. Li, B. Huang, D. Egeren, C. Park and D. Irvine, Structure-based programming of lymph-node targeting in molecular vaccines, Nature, 2014, 507, 519–522 CrossRef CAS PubMed
.
- G. Lynn, C. Sedlik, F. Baharom, Y. Zhu, R. Ramirez-Valdez, V. Coble, K. Tobin, S. Nichols, Y. Itzkowitz, N. Zaidi, J. Gammon, N. Blobel, J. Denizeau, P. Rochere, B. Francica, B. Decker, M. Maciejewski, J. Cheung, H. Yamane, M. Smelkinson, J. Francica, R. Laga, J. Bernstock, L. Seymour, C. Drake, C. Jewell, O. Lantz, E. Piaggio, A. Ishizuka and R. Seder, Peptide-TLR-7/8a conjugate vaccines chemically programmed for nanoparticle self-assembly enhance CD8 T-cell immunity to tumor antigens, Nat. Biotechnol., 2020, 38, 320–332 CrossRef CAS PubMed
.
- M. Pei, H. Li, Y. Zhu, J. Lu and C. Zhang, In vitro evidence of oncofetal antigen and TLR-9 agonist co-delivery by alginate nanovaccines for liver cancer immunotherapy, Biomater. Sci., 2022, 10, 2865–2876 RSC
.
- S. Van Herck, K. Deswarte, L. Nuhn, Z. Zhong, J. Catani, Y. Li, N. Sanders, S. Lienenklaus, S. De Koker, B. Lambrecht, S. David and B. Geest, Lymph-node-targeted immune activation by engineered block copolymer amphiphiles-TLR7/8 agonist conjugates, J. Am. Chem. Soc., 2018, 140, 14300–14307 CrossRef CAS PubMed
.
- L. Nuhn, S. De Koker, S. Van Lint, Z. Zhong, J. Catani, F. Combes, K. Deswarte, Y. Li, B. Lambrecht, S. Lienenklaus, N. Sanders, S. David, J. Tavernier and B. De Geest, Nanoparticle-conjugate TLR7/8 Agonist localized immunotherapy provokes safe antitumoral responses, Adv. Mater., 2018, 30, e1803397 CrossRef PubMed
.
- A. M. Tsimberidou, E. Fountzilas, M. Nikanjam and R. Kurzrock, Review of precision cancer medicine: Evolution of the treatment paradigm, Cancer Treat. Rev., 2020, 86, 102019 CrossRef CAS PubMed
.
- Y. Ge, Y. Ma and L. Li, The application of prodrug-based nano-drug delivery strategy in cancer combination therapy, Colloids Surf., B, 2016, 146, 482–489 CrossRef CAS PubMed
.
- X. Xu, W. Ho, X. Zhang, N. Bertrand and O. Farokhzad, Cancer nanomedicine: from targeted delivery to combination therapy, Trends Mol. Med., 2015, 21, 223–232 CrossRef CAS PubMed
.
- R. Zhang, H. Wong, H. Xue, J. Eoh and X. Wu, Nanomedicine of synergistic drug combinations for cancer therapy-strategies and perspectives, J. Controlled Release, 2016, 240, 489–503 CrossRef CAS PubMed
.
- B. Ji, M. Wei and B. Yang, Recent advances in nanomedicines for photodynamic therapy
(PDT)-driven cancer immunotherapy, Theranostics, 2022, 12, 434–458 CrossRef CAS PubMed
.
- L. Chen, L. Zhou, C. Wang, Y. Han, Y. Lu, J. Liu, X. Hu, T. Yao, Y. Lin, S. Liang, S. Shi and C. Dong, Tumor-targeted drug and CpG delivery system for phototherapy and docetaxel-enhanced immunotherapy with polarization toward M1-type macrophages on triple negative breast cancers, Adv. Mater., 2019, 31, 1904997 CrossRef CAS PubMed
.
- J. Choi, M. Shim, S. Yang, H. Hwang, H. Cho, J. Kim, W. Yun, Y. Moon, J. Kim, H. Yoon and K. Kim, Visible-light-triggered prodrug nanoparticles combine chemotherapy and photodynamic therapy to potentiate checkpoint blockade cancer immunotherapy, ACS Nano, 2021, 15, 12086–12098 CrossRef CAS PubMed
.
- A. Sofias, M. Dunne, G. Storm and C. Allen, The battle of “nano” paclitaxel, Adv. Drug Delivery Rev., 2017, 122, 20–30 CrossRef CAS PubMed
.
- M. Barkat, S. Beg, F. Pottoo and F. Ahmad, Nanopaclitaxel therapy: an evidence based review on the battle for next-generation formulation challenges, Nanomedicine, 2019, 14, 1323–1341 CrossRef PubMed
.
- B. Mendes, D. Sousa, J. Conniot and J. Conde, Nanomedicine-based strategies to target and modulate the tumor microenvironment, Trends Cancer, 2021, 7, 847–862 CrossRef CAS PubMed
.
- L. Sun, P. Zhao, M. Chen, J. Leng, Y. Luan, B. Du, J. Yang, Y. Yang and R. Rong, Taxanes prodrug-based nanomedicines for cancer therapy, J. Controlled Release, 2022, 348, 672–691 CrossRef CAS PubMed
.
- A. Bedikian, R. DeConti, R. Conry, S. Agarwala, N. Papadopoulos, K. Kim and M. Ernstoff, Phase 3 study of docosahexaenoic acid-paclitaxel versus dacarbazine in patients with metastatic malignant melanoma, Ann. Oncol., 2011, 22, 787–793 CrossRef CAS PubMed
.
- L. Ares, H. Ross, M. Brien, A. Riviere, U. Gatzemeier, J. Von Pawel, E. Kaukel, L. Freitag, W. Digel, H. Bischoff, R. Campelo, N. Iannotti, P. Reiterer, I. Bover, J. Prendiville, A. Eisenfeld, F. Oldham, B. Bandstra, J. Singer and P. Bonomi, Phase III trial comparing paclitaxel poliglumex vs. docetaxel in the second-line treatment of non-small-cell lung cancer, Br. J. Cancer, 2008, 98, 1608–1613 CrossRef PubMed
.
|
This journal is © The Royal Society of Chemistry 2024 |
Click here to see how this site uses Cookies. View our privacy policy here.