DOI:
10.1039/D1AY01570B
(Minireview)
Anal. Methods, 2022,
14, 7-16
Applications of electrochemical biosensors based on functional antibody-modified screen-printed electrodes: a review
Received
15th September 2021
, Accepted 22nd November 2021
First published on 23rd November 2021
Abstract
The detection of biomolecular analytes is of great importance in clinical, environmental, and argo-food areas, among which the electrochemical methodology is attracting much attention. In particular, screen-printed electrode (SPE)-based sensing applications have exhibited potential possibility for on-site detection, especially for fast clinical biomarker detection, since they provide a miniaturized but robust and portable electrode detection system. In this context, we focused on the modification of SPE with functional antibodies to improve the electrochemical detection performance in versatile sensing applications, particularly for COVID-19 detection. These antibodies were immobilized onto the electrode surface via various methodologies, through which the powerful potential from the modification of SPE was revealed. Finally, more novel and excellent works on the biomolecular modification of SPE and the prospects of this technology from its state-of-art status to commercialization are previewed and future perspectives in this field are mentioned.
1. Introduction
Sensing applications in highly assorted fields, such as clinical and biomedical areas, food analysis and quality control, as well as environmental monitoring, are desired to be equipped with the capability to solve different analytical problems in a fast and reliable way, preferably by returning real-time information that provides immediate feedback. Here, the electrochemical sensing technique with intrinsic simplicity, rapid response, high sensitivity, and low cost, is probably among the most promising of the alternative methodologies reported in the past few years.1–5 Additionally, electrochemical sensors properly hold the potential for on-site analysis by transitioning away from the conventional commonly used relatively cumbersome electrochemical cell systems to miniaturized and portable electrodes, such as screen-printed electrodes (SPEs),6,7 which are the main topical objects of this review.
Compared to traditional disk electrodes that are more stuck to laboratory use, SPEs are additionally characterized by disposable use, flexible design, and a smaller size, which meet the needs for on-site analysis.8,9 In recent years, nanotechnology and the exploitation of new fabrication techniques have helped to enhance the electroanalytical characteristics of SPEs.10 For example, nanomaterials (carbon nanotubes,11,12 graphene,13,14 gold nanoparticles,15,16etc.) used on the working electrode (WE) of SPE can significantly improve the surface activity due to their much higher specific surface area and superior electrocatalytic properties. This procedure can be performed through an automatic dispenser in a mass-producible way on the planar SPE or before mixing the modifier in the ink while printing. This enhancement can, on the one hand, help to detect some conductive analytes directly,17–20 while on the other hand, it is frequently applied to improve the immobilization of the recognition element, which is often a biomolecule, for further analyte identification and signal transduction.
In this article, we would like to narrowly review SPE-based electrochemical biosensors that are modified with functional antibodies for sensing applications in clinical, environmental, and argo-food areas. In addition, diagnosis of the current global outbreak of coronavirus is discussed as one more demand supporting the significance of developing electrochemical detection methodology for more accurate and rapid detection demands.
2. Functional SPEs modified with antibodies
Antibody-based sensors utilize the natural affinity of antibodies toward antigen and date as far back as 1959.21 The most successful application of this immunoassay may be the enzyme-linked immunosorbent assay (ELISA), in which primary antibodies immobilized on the solid surface capture a target antigen present in the sample proteins. Then, a secondary antibody, which is commonly conjugated with an enzyme, is introduced, followed by a washing process for the whole system. Finally, a chemiluminescent substrate is added for enzyme reaction and an optical result is presented to detect or quantify the target antigen. Electrochemical antibody-based sensors usually bear resemblance to the ELISA, while owning some other special characteristics from the SPE surface modification and the electronic measurement itself.
2.1 Immobilization of antibodies by indirect or direct competitive methodologies
Unlike the direct immobilization of analytes in ELISA, conjugated forms of analytes can be immobilized on the electrode in an electrochemical immunosensor to render much-improved sensitivity.22 Such immobilization is more compatible with the competitive immunoassay methodology, and more accurate analyses can be performed for small molecules. As shown in Fig. 1A, Kim et al. constructed an easy and rapid immunosensing platform for the highly sensitive and selective detection of zearalenone (ZEN) based on the direct immobilization of analyte-conjugated bovine-serum albumin (BSA) on the SPCE surface.23 Anti-zearalenone monoclonal antibody (ZEN-MAb) as the primary antibody, alkaline phosphatase (ALP)-labeled secondary antibody, and 1-napthyl phosphate (1-NP) as a substrate were subsequently added. The binding of the primary antibody involved an indirect competitive immunoreaction with ZEN in the solution. The binding of the secondary antibody labeled with ALP followed by the addition of the 1-NP substrate allowed determination of the concentration of ZEN via DPV of the generated enzyme product 1-napthol. This immunosensor had good specificity, selectivity, reproducibility and sensitivity for ZEN and the potential to be easily extended to the detection of other small analyte molecules.
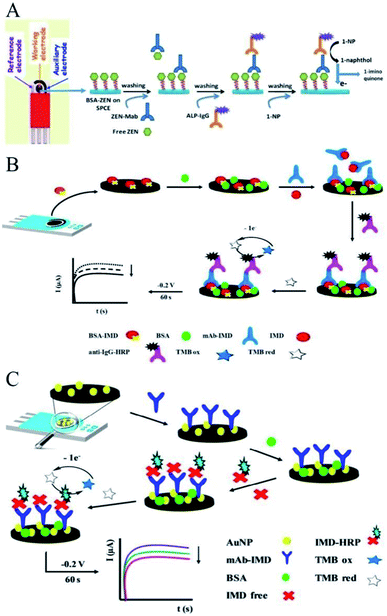 |
| Fig. 1 Immobilization of antibodies by indirect or direct competitive methodologies. (A) Scheme of the construction of an indirect competitive immunosensing platform for ZEN detection. Reprinted from ref. 23, Copyright 2019, with permission from Elsevier. (B) Scheme of the construction of an indirect competitive immunosensing platform for IMD detection. Reprinted from ref. 24, Copyright 2019, with permission from The Royal Society of Chemistry. (C) Direct competitive immunosensor for the determination of IMD. Reprinted from ref. 25, Copyright 2019, with permission from Elsevier. | |
Similar work in the detection of imidacloprid (IMD) was developed by Costa-Garcia and colleagues, with their scheme shown in Fig. 1B.24 BSA-labeled antigen (BSA-IMD) immobilized on the SPCE surface and free IMD analyte competed for the specific mAb-IMD monoclonal antibody. The HRP label was specifically linked to the electrode through anti-IgG-HRP secondary antibodies. After that, the added TMB reagent was enzymatically oxidized by the HRP molecules. The oxidized TMB could be reduced back at the surface of the SPCE by applying a constant potential of −0.2 V for 60 s, producing an associated catalytic current that was proportional to the IMD amount in an indirect detection way.
A direct competitive immunosensor for the electrochemical determination of IMD has also been developed.25 In this biosensor design (Fig. 1C), free IMD in the sample competed with IMD conjugated with HRP for recognition by the antibodies. After that, 3,3′,5,5′-tetramethybenzidine (TMB) was enzymatically oxidized by HRP, followed by the oxidized TMB being reduced back at the surface of the SPCE. This process gave an associated catalytic current that was inversely proportional to the IMD amount. In this work, nanomaterials were also used to help immobilize the biomolecules.
2.2 Immobilization of antibodies with the help of graphene
As mentioned before, the modification of the SPE surface with nanomaterials can vastly help to immobilize the captured antibodies. In particular, graphene, which was described as “more than just a flat crystal” by Novoselov, has been vastly implemented in screen-printed electrochemical biosensors.26 In an earlier work, Kim and co-workers explored a convenient graphene-SPCE platform for the non-enzymatic label-free immunosensing of parathion.27 Unlike other approaches, as illustrated in Fig. 2A, the therein used graphene SPCE did not need any mixing with other materials and the bioconjugation process was also straightforwardly performed without the use of cross-linkers or any other cumbersome chemistry. The SPCE was first modified with dispersed graphene sheets and the electrode surface was then electrochemically treated with 2-aminobenzyl amine so as to generate active –NH2 functional groups. The above-functionalized electrodes were then simply incubated with the anti-parathion antibodies to realize the desired biosensor. In this way, the antibody molecules were bound to the graphene-modified SPCE through the Fc region. This oriented immobilization allowed the biosensor to have free Fab regions on the bio-recognizing probe. As such, an enhanced detection sensitivity of the biosensor was achieved by activating all the possible available antibody probes in the system.
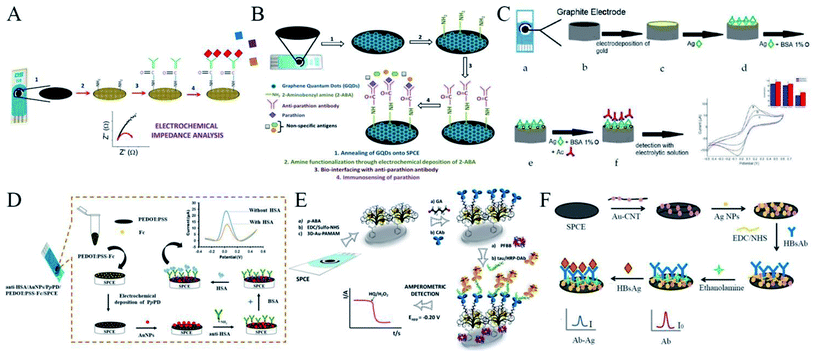 |
| Fig. 2 Immobilization of antibodies with the help of nanomaterials, in terms of graphene, metal nanoparticles, and nanocomposite. (A) Scheme of the construction of a convenient graphene-SPCE platform for the immunosensing of parathion. Reprinted from ref. 27, Copyright 2016, with permission from Elsevier. (B) QODs were used to help immobilize antibodies. Reprinted from ref. 28, Copyright 2017, with permission from Elsevier. (C) Scheme of the construction of an electrochemical immunosensor for the diagnosis of visceral leishmaniasis based on a AuNPs-modified SPCE. Reprinted from ref. 32 (open access). (D) Scheme of the construction of an immunosensing platform with the help of a nanocomposite for HSA detection. Reprinted from ref. 36, Copyright 2020, with permission from Elsevier. (E) Scheme of the construction of an electrochemical immunosensor with the help of a nanocomposite for the detection of tau protein. Reprinted from ref. 38, Copyright 2020, with permission from Elsevier. (F) An electrochemical immunosensor based on the specificity of antigen–antibody interaction for the detection of HBsAg. Reprinted from ref. 39, Copyright 2020, with permission from Elsevier. | |
Furthermore, developed nanostructures of graphene quantum dots (GODs) were used in another similar work.28 GODs have attracted immense research interest for the assembly of electrochemical biosensors and can behave both as excellent electron acceptors and donors as they are structurally similar to graphene. As shown in Fig. 2B, Kim et al. for the first time explored the use of GODs for the development of a label-free EIS-based screen-printed immunosensor for pesticide detection. In this work, SPCE was modified with GODs and then electrochemically modified with 2-aminobenzyl amine to craft –NH2 functionality of the anti-parathion antibodies. The application of GODs, in comparison to graphene sheets, seemed to offer certain advantages as they could be easily decorated with more homogeneity on the screen-printed surface, which led to more efficient control for higher sensor precision and accuracy. The functional biomolecule anti-parathion antibody was decorated on the sensor with the help of the in situ availability of the functional groups on the GOD surface, which allowed robustly assembling the 2-aminobenzylamine moieties to facilitate the amine functionality. These modifications of nanomaterials on the SPCE significantly helped the immobilization of antibodies to facilitate immunosensing of the target analyte.
2.3 Immobilization of antibodies on metal nanoparticles
Similar with graphene, research into metal nanoparticles (NPs)-based electrochemical detection for bioanalysis has also drawn increasing attention.29 These metal NPs have a high surface area, high mechanical strength but ultra-light weight, rich electronic properties, and excellent chemical and thermal stability, which make them highly suitable for the immobilization of antibodies in electrochemical immunoassays. In the construction of an immunosensor that could detect an antigen within a very low concentration range, the heavy loading of antibodies on the electrode is crucial and the choice of an appropriate supporting layer can enhance the immobilization of the antibodies.30 The formation of gold nanoparticles (AuNPs) by electrodeposition on the working electrode of the SPCE for further immobilization represents a well-known platform for gold-modified screen-printed carbon electrodes.31
For example, Alves-Balvedi et al. proposed an electrochemical immunosensor for the diagnosis of visceral leishmaniasis based on a AuNPs-modified SPCE (Fig. 2C).32 This kind of platform guaranteed the effective adsorption of all antigens so that the epitope of the specific recognition for leishmaniasis occurred efficiently and without cross-reaction with the evaluated Chagas disease (CD). The modification with AuNPs provided an increased surface area and hence could potentially improve the sensitivity.
2.4 Immobilization of antibodies on nanocomposites
Though AuNPs are, with undoubtedly one of the most widely used nanomaterials, as well as a key component in the fabrication of nanocomposites on disposable devices, due to their unique properties, including high conductivity and biocompatibility,33 the individual usage of these nanosized particles often suffers from their strong tendency to agglomerate, which diminishes and affects their utility.34 Stabilizers, also called capping agents, including polymers, surfactants, and chelating agents, play an important role in the preparation of these metal NPs, particularly in controlling their size and preventing their aggregation in solution.35
For instance, Numnuam et al. fabricated a novel, label-free immunosensor for human serum albumin (HSA) detection in urine and human serum.36 As shown in Fig. 2D, the SPCE was modified with a poly(3,4-ethylenedioxythiophene):poly(styrenesulfonate) and ferrocene nanocomposite (PEDOT:PSS-Fc), which was first coated with poly(para-phenylenediamine) (PpPD) and then functionalized with AuNPs. Anti-HSA was immobilized on the AuNPs/PpPD layer by direct chemisorption and the HSA sample concentration could be determined from the change in the Fc oxidation peak current caused by the formation of a HAS/anti-HAS immunocomplex. In this way, the PEDOT:PSS effectively entrapped the Fc and the current signal was improved by the high conductivity of the polymer. AuNPs, adsorbed onto the electrodeposited layer of PpPD, significantly provided excellent conductivity and enhanced the population of anti-HAS molecules by enlarging the surface area of the bioreceptor.
Another method involves forming dendrimers, which has been claimed are useful soft nanocontainers for the supramolecular encapsulation of NPs through electrostatic or hydrophobic interactions.37 In this way, Razzino and colleagues utilized poly(amidoamine) (PAMAM) dendrimers, which are particularly useful in the synthesis of different types of metal NPs, to encapsulate AuNPs in their new integrated amperometric biosensor for the determination of tau protein, a hallmark of Alzheimer's disease (AD).38 As shown in Fig. 2E, their approach involved modifying an SPCE with a three-dimensional network PAMAM–AuNPs nanocomposite (3D-Au–PAMAM) as a biocompatible material and template due to the many surface functional –NH2 groups. This was then covalently immobilized through carbodiimide/hydroxysulfosuccinimide chemistry onto the SPCE modified with electrografted p-aminobenzoic acid (p-ABA) and then further used as a scaffold to develop a sandwich immunosensor upon anti-tau capture antibody (CAb) anchoring using glutaraldehyde (GA) as a cross-linking agent. Thus, antibodies were covalently immobilized and a sandwich immunoassay was then implemented using a detector antibody labeled with horseradish peroxidase (HRP-DAb), and amperometric detection was finally carried out using the H2O2/HQ system.
These nanomaterials could also be used in complexes. Jakmunee et al. developed an electrochemical immunosensor based on the specificity of the antigen–antibody interaction for the detection of hepatitis B surface antigen (HBsAg).39 As shown in Fig. 2F, the SPCE was modified with carbon nanotubes decorated with AuNPs (AuNPs–CNT) and silver nanoparticles (AgNPs). The AuNPs–CNT provided good biocompatibility and a large surface area for densely immobilizing the hepatitis B surface antibody (HBsAb) on the electrode, which helped to improve the signal. AgNPs were then deposited on the modified electrode for use as a redox probe in the detection system, providing a high current response and low oxidation potential. The detection method was based on the decrease in the oxidation peak currents with the increase in HBsAg concentration, because the formation of the antibody–antigen complex on the electrode surface acted as an insulator and hindered electron transfer, which allowed the label-free direct detection of the HBsAg. In another work, Bagheri et al. reported a new dual-modality immunosensor based on a molecularly imprinted polymer (MIP) and a nanostructured biosensing layer.40 The MIP was prepared with methyl acrylate as a monomer and ethylene glycol dimethacrylate as a crosslinker in the presence of prostate specific antigen and myoglobin as templates. The modified electrode and synthesized nanoparticles were fully characterized and the ability of the biosensor to detect two targets simultaneously with sensitivity and specificity was demonstrated to offer a powerful opportunity for the generation of new biosensors.
2.5 Immobilization of antibodies on alternative substrates
Besides the modification of nanomaterials, the use of an alternative substrate in SPE-based biosensors can also help to fabricate an ideal immunodevice. For example, Oliveira et al. focused on the preparation of homemade Ag screen-printed interdigitated electrodes (SPIDEs) to detect the pancreatic cancer biomarker, carbohydrate antigen 19-9 (CA19-9).41 As shown in Fig. 3A, these electrodes were prepared with low-cost, screen-printing protocols for large-scale production, and were based on a Ag layer separated by a width of 180 μm on a polyethylene terephthalate (PET) substrate. The electrode surface had a heterogeneous morphology according to scanning electron microscopy (SEM). The electrodes were further modified by carbon nano-onions (CNOs) and graphene oxide (GO) films, upon which a layer of anti-CA19-9 antibodies was immobilized. The high surface area and mesoporous nature of the CNOs led to porous films with a large roughness for better immobilization of the antibodies, which revealed a 16-fold increase in the SPIDE/CNO–GO surface roughness compared to electrodes without the incorporation of CNOs. They were able to detect CA19-9 in a wide dynamic range (from 0.3 to 100 U ml−1), with a limit of detection of 0.12 U ml−1, at a cost of only ∼US$0.05 per device unit for the novel SPIDEs. The sensor device was generic, and thus could be explored for other biosensors and could even be applied as a non-invasive sensor biointegrated onto the skin for testing sweat, saliva or urine as samples, meaning it would be amenable for point-of-care sensing applications.
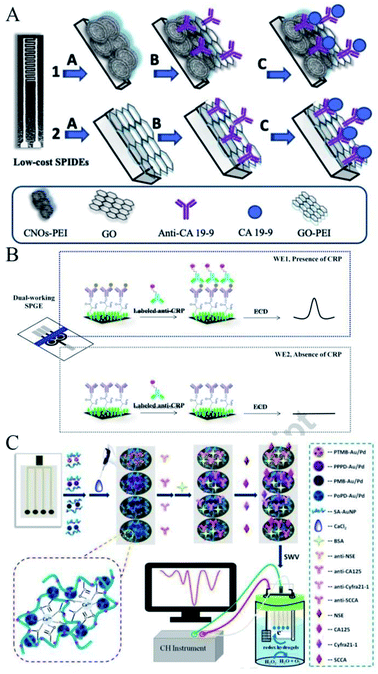 |
| Fig. 3 Immobilization of antibodies on an alternative substrate. (A) Scheme of the preparation of homemade Ag screen-printed interdigitated electrodes. Reprinted from ref. 41, Copyright 2019, with permission from Elsevier. (B) Construction of the multiple-working electrodes. Reprinted from ref. 42, Copyright 2018, with permission from Elsevier. (C) Construction of a label-free amperometric immunosensor with multiple working electrodes and one signal output channel. Reprinted from ref. 43, Copyright 2018, with permission from Elsevier. | |
In addition, SPEs can be custom-designed to realize multiple working electrodes. As shown in Fig. 3B, Chailapakul et al. fabricated a portable, low-cost, and sensitive electrochemical immunosensor with dual-working electrodes to simultaneously compare the presence and absence of the target analyte C-reactive protein (CRP) with a reduced analysis time.42 Also, Ma et al. designed a label-free amperometric immunosensor with multiple working electrodes and one signal output channel without a counter and reference electrode to simultaneously detect four tumor markers (Fig. 3C).43 The multiple working electrodes could be individually modified for each target of interest and the developed SPCE could be used in the construction of multiplexed label-free immunosensors. More creative electrodes have also been reported by others. For example, Zucolotto et al. fabricated a disposable electrode by thermal evaporation on polyethylene terephthalate substrates covered with a nanometric gold layer manufactured in three-contact configurations for the label-free detection of zika virus.44 Also, Janegitz et al. fabricated screen-printed platinum electrodes on bio-based poly(ethylene terephthalate) substrates for the development of flexible electrochemical sensors for the detection of Parkinson's disease biomarkers.45
2.6 Immobilization of antibodies on screen-printed gold electrodes
These SPEs introduced above were mainly carbon electrodes; however, there are also electrochemical immunosensors based on the SPGE. Different from the sensors based on the SPCE, antibodies can be immobilized onto a gold surface via physical or chemical adsorption involving electrostatic or ionic bonds, hydrophobic interactions, and van der Waals forces,46via covalent attachment,47,48 by using the biotin-avidin approach49 or by immobilizing intermediate binding proteins50,51 and through entrapment into the polymer matrix.52 Among all the possible strategies, the self-assembled monolayer (SAM) is currently one of the most widespread methods for the electrode functionalization for antibody immobilization.53,54 SAMs on gold surfaces are usually represented as a perfect monolayer with molecules in a close-packed configuration. Compton et al. reported a fully functional chemicodetection principle for a successful biosensing device.55 As shown in Fig. 4A, an SPGE was modified by thiol chemistry, following the method of Maalouf,56 forming a biotin-thiol and spacer-thiol SAM on the gold surface. Following the blockage of unspecific binding sites with BSA, incubation with neutravidin resulted in the replacement of BSA at the biotinylated thiols. Polyclonal anti-E. coli antibodies were added to capture the target bacteria on the substrate during incubation with a cell suspension. The rapid and sensitive electrochemical detection of pathogens once immobilized on the surface was thus realized. In another work, a label-free impedimetric immunosensor based on the use of SPGE for the detection of Salmonella typhimurium was reported by Arduini's group.57 With the aim to enhance the immunosensor electrochemical performances, different protocols have been accomplished to pre-treat the working electrode surface used as a platform to immobilize the immunological chain in a self-assembled monolayer configuration.
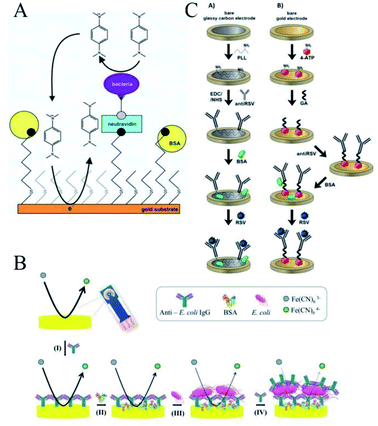 |
| Fig. 4 Immobilization of antibodies on the screen-printed gold electrode. (A) Fully functional chemicodetection principle for a successful biosensing device. Reprinted from ref. 55, Copyright 2019, with permission from American Chemical Society. (B) Using PIT to immobilize the anti-E. coli antibodies on the gold electrode surface. Reprinted from ref. 59 (open access). (C) Comparison of the performance of screen-printed gold and the glassy carbon electrodes. Reprinted from ref. 62, (open access). | |
However, in spite of the many advantages SAM offers in many applications, there are also several issues that should be considered in order to develop some alternative methods to covalently bind antibodies on gold electrode surfaces in an easier, more rapid, and reliable way.58 Ventura et al. proposed a simple and low-cost EIS immunosensor based on an SPGE for the detection of Escherichia coli (E. coli) in drinking water.59 As shown in Fig. 4B, anti-E. coli antibodies were immobilized onto the gold electrode surface using the photochemical immobilization technique (PIT), a simple procedure able to steer antibodies in a convenient orientation of the Fab region once immobilized onto the gold surface.60,61 In this work, PIT was used for the first time in the functionalization of commercial gold electrodes in order to develop an “on-off” electrochemical immunosensor based on EIS. Their results demonstrated that PIT could effectively achieve antibody immobilization even on commercial cheap electrodes.
There are also comparisons of the performances of screen-printed gold and glassy carbon electrodes. As shown in Fig. 4C, Nidzworski et al. presented the development and comparison of label-free electrochemical immunosensors based on screen-printed gold and glassy carbon disc electrodes (GCDEs) for the efficient and rapid detection of respiratory syncytial virus (RSV).62 In this work, the antibody specific to the F protein of RSV was successfully immobilized on the modified electrodes. Antibody coupling on the Au surface was conducted via 4-aminothiophenol (4-ATP) and glutaraldehyde (GA). The GCDE surface was modified with poly-L-lysine (PLL) for direct anti-RSV conjugation after EDC/NHS (1-ethy1-3-(3-dimethylaminopropyl) carbodiimide/N-hydroxysuccinimide) activation. Electrochemical characterizations of these immunosensors were carried out by CV and EIS to compare the performance between these two immunosensors in terms of their sensitivity, limit of detection (LOD), dynamic range, and specificity. In terms of the results, the GCDE-based sensor showed a better dynamic range of antigen detection than the SPGE-based sensor. However, the GCDE platform was less sensitive and showed a higher LOD for RSV, which was probably due to the higher density of anti-RSV antibody coverage on the Au surface. Thus, the SPGE-based immunosensor had better analytical performance for virus detection than the GCDE platform due to its high sensitivity and very low RSV detection, with the results obtained with good reproducibility in this work.
2.7 Pre-isolation of the target in antibody-modified SPEs
In some other SPE-based electrochemical immunoassay works, pre-isolation of the target analytes, which are usually of low buoyant density and small size in biological samples, is often performed before capturing and detection on the electrode surface. Shiddiky et al. introduced a simple method for the direct isolation and subsequent detection of a specific population of exosomes using an engineered superparamagnetic material with multifunctional properties, namely, gold-loaded ferric oxide nanocubes (Au–NPFe2O3NC).63 In this method (Fig. 5A), the Au–NPFe2O3NC were initially functionalized with a generic tetraspanin antibody and dispersed in sample fluids, where they worked as “dispersible nanocarriers” to capture the bulk population of exosomes. After magnetic collection and purification, the Au–NPFe2O3NC-bound exosomes were transferred to the tissue-specific, antibody-modified SPCE. As a proof of principle, they used a specific placental marker, placenta alkaline phosphatase (PLAP), to detect the exosomes secreted from placental cells. The peroxidase-like activity of Au–NPFe2O3NC was then used to accomplish an ELISA-based sensing protocol for naked-eye observation along with the UV-visible and electrochemical detection of PALP-specific exosomes present in placental cell-conditioned media. Similar work (Fig. 5B) was performed by Omidfar and colleagues for the analysis of gene-specific methylation using Fe3O4/N-trimethyl chitosan/gold (Fe3O4/TMC/Au) nanocomposites as a tracing tag to label a DNA probe and with highly specific anti-5-methylcytosin monoclonal antibody-entrapped polythiophene (PT) as an immobilization platform for capturing the target element.64 Pingarron and co-workers also reported the first bioplatform able to determine electrochemically 5-hydroxymethylcytosine (5-hmC) methylation events at localized sites and single-base sensitivity.65 As shown in Fig. 5C, the described bioplatform relied on a specific antibody (anti-5-hmC) immobilized on the SPCE surface and further conjugated with commercial bioreagents loaded with multiple HRP molecules that allowed recognize the epimark in target DNA, which was captured through hybridization onto streptavidin-magnetic microbeads modified with a complementary DNA capture probe. In these methods, the SPCE was modified with biomolecule antibodies to capture the compound, whereas some other similar works for detecting miRNA,66–68 cocaine,69 DNA methylation,70,71 and even COVID-19 (ref. 72) involved only the use of screen-printed disposable electrodes as electrochemical transducers. These SPEs were not modified with biomolecules and thus are not introduced in detail in this review. In addition to this, there are some other excellent works related to SPE modification with biomolecular antibodies.73–76
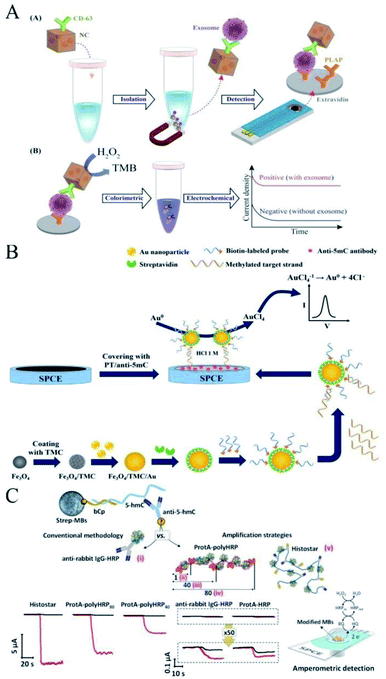 |
| Fig. 5 Pre-isolation of the target in antibody-modified SPEs. Immobilization of antibodies on a screen-printed gold electrode. (A) Simple method for the direct isolation and subsequent detection of a specific population of exosomes. Reprinted from ref. 63, Copyright 2019, with permission from American Chemical Society. (B) Similar work for the analysis of gene-specific methylation. Reprinted from ref. 64, Copyright 2015, with permission from Elsevier. (C) Scheme of the construction of a bioplatform that relies on a specific antibody (anti-5-hmC) immobilized on the SPCE surface. Reprinted from ref. 65, Copyright 2020, with permission from American Chemical Society. | |
3. SPE-based immunosensors for the detection of COVID-19
Coronavirus disease (COVID-19), caused by the novel severe acute respiratory syndrome coronavirus 2 (SARS-CoV-2), has spread worldwide since the end of 2019 and has resulted in an ongoing pandemic that is threatening the whole of humankind's health.77 As the virus holds the characteristic of relatively high transmissibility, increasing research on rapid identification of the virus is currently being implemented to prevent further outbreaks, among which electrochemical detection-based point-of-care testing has advantages in terms of its ease of use, greater approachability on the user end, more timely detection, and comparable accuracy and sensitivity.78 Many freshly released reviews have summarized the electrochemical investigations for COVID-19 detection from different perspectives.79–84 Also, here below we would like to discuss these specially-made SPE-based electrochemical immunosensors for the detection of COVID-19.
SARS-CoV-2 is a novel Coronaviridae virus possessing a single-strand and positive RNA genome ∼3 kb in length, which encodes for 10 genes, including the 5′ untranslated region, replicase complex (orf1ab), spike (S), envelope (E), membrane (M), and nucleocapsid (N) structural proteins, 3′-untranslated region, and several unidentified non-structural open reading frames.85 Among these, the S and N proteins have the potential to be used as biomarkers because they can distinguish different types of corona virus and most detection methods are based on the diagnostics of these gene regions or antigens. As shown in Fig. 6A, Zourob et al. reported for the first time the combination of cotton fibers and electrochemical assays in a carbon nanofiber-modified SPCE for the detection of the SARS-CoV-2 antigen.86 The cotton-tipped electrochemical immunosensor played dual function roles as the sample collector and detector, allowing the rapid, simple, and low-cost detection of the virus without prior sample preparation. Also in this method, the electrode was typically used for the immunosensor fabrication on which the N antigen was immobilized after functionalization of the sensor surface by electrografting. A competitive assay was used for the detection of the N protein showing excellent sensitivity and selectivity. Besides, the electrochemical detection could be performed using a handheld potentiostat connected to a smartphone for the signal reading, which, significantly satisfied the needs for the direct, low-cost, and rapid detection of the COVID-19 virus.
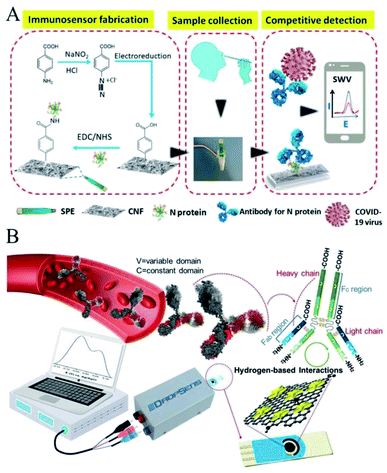 |
| Fig. 6 SPE-based immunosensors for the detection of COVID-19. (A) Schematic of the cotton-tipped electrochemical immunosensor for COVID-19. Reprinted from ref. 86, Copyright 2020, with permission from American Chemical Society. (B) Interaction of G–Au NS complex with IgG antibodies against the S1 glycoprotein of SARS-CoV-2. Reprinted from ref. 87, Copyright 2021, with permission from Elsevier. | |
Similarly, Hashemi and co-workers developed an improved label-free SPCE-based nanosensor composed of activated graphene oxide in conjunction with Au nanostars toward the direct detection of monoclonal IgM antibodies against the S1 glycoprotein of SARS-CoV-2 within blood specimens of infected or suspected people with infectious COVID-19 disease (Fig. 6B).87 The sensor exhibited a strong correlation with the obtained data from the gold-standard ELISA assay, showing the fantastic performance of the developed electrochemical method for the precise, rapid, and confident detection of COVID-19.
Besides, some other excellent electrochemical works that have adopted other detection strategies, like a catalytic hairpin circuit,88 aptamer-based imaging,89 supersandwich-type recognition for targeting RNA,90 RCA amplification with sandwich hybridization,91 and works utilizing other kinds of electrodes, like the glassy carbon electrode,92 3D-printed graphene-based nanocomposite electrode,93 Au laser-scribed graphene electrode,94 and mass-producible laser-engraved graphene electrode,95 have been reported to jointly fight for the accurate and rapid detection of COVID-19. All these methodologies show the potential of the electrochemical detection strategy in the diagnostics of global diseases, like the ongoing COVID-19. Notably, some works have attempted to integrate the electrochemical detection system into a POC system with a smartphone attachment, enabling the diagnostic system to evolve into a more portable tool for SARS-CoV-2 virus detection.90,94–96
4. Conclusions and perspectives
Screen-printed electrodes (SPEs) are emerging platforms with outstanding potential for their use as transducers in electrochemical biosensors. Their superior advantages, including disposability, portability, low-volume loading, making them ideal for the on-site detection in clinical, environmental, and argo-food areas. Additionally, their further modification with functional biomolecules broadens the wide range of electrochemical biosensor designs. In this review, we summarized the functional antibodies modified on the working electrode of the SPE.
The strategy of immobilizing antibodies on the electrode surface is seemingly derived from the ELISA method, followed by specific electrochemical detection methodologies. Antibodies are stable, together with possessing superior selectivity and specificity. In spite of this, the relatively high cost and challenging availability of some special monoclonal antibodies are the main obstructive factors in the determination of selecting this biosensing approach.
As for the detection medium itself, SPE seems to be one of the favorite choices of researchers for the fast, accurate, but cost-effective detection of disease or other emerging analytes. Also, efforts toward attaching a portable detection meter, like a smartphone via USB or Bluetooth connection, significantly meets the further requirement of fast and point-of-care detection, which, from another way to think about it, would improve the potentiality of SPE biosensors to be commercialized, like a well commercialized glucose meter that was thus previewed.
In conclusion, the high potential of SPE-based biosensors is worthy of much attention. Also, the combination of such miniaturized electrochemical transducers, cheap and portable electrochemical detection instruments, and the modification with functional biomolecules make for an ideal system for sensing applications in various areas, even in the current fight for the detection of COVID-19. More excellent works will be previewed in the coming years.
Conflicts of interest
The authors declare that they have no known competing financial interests or personal relationships that could have appeared to influence the work reported in this paper.
Acknowledgements
This work was supported by the Zhejiang Provincial Natural Science Foundation of China under Grant No. LGF19H200001 and LGC19H200004.
Notes and references
- E. Palecek and M. Bartosik, Chem. Rev., 2012, 112, 3427–3481 CrossRef CAS PubMed.
- O. Simoska and K. J. Stevenson, Analyst, 2019, 144, 6461–6478 RSC.
- S. Campuzano, M. Pedrero, P. Yánez-Sedeño and J. M. Pingarrón, Electroanalysis, 2019, 31, 1816–1832 CrossRef.
- S. Campuzano, V. Serafin, M. Gamella, M. Pedrero, P. Yanez- Sedeno and J. M. Pingarron, Sensors, 2019, 19, 3762–3781 CrossRef CAS PubMed.
- E. O. Blair and D. K. Corrigan, Biosens. Bioelectron., 2019, 134, 57–67 CrossRef CAS PubMed.
- R. Torre, E. Costa-Rama, H. P. A. Nouws and C. Delerue-Matos, Biosensors, 2020, 10, 139–164 CrossRef CAS PubMed.
- N. B. Mincu, V. Lazar, D. Stan, C. M. Mihailescu, R. Iosub and A. L. Mateescu, Diagnostics, 2020, 10, 517–537 CrossRef CAS PubMed.
- K. Yamanaka, M. C. Vestergaard and E. Tamiya, Sensors, 2016, 16, 1761–1776 CrossRef PubMed.
- B. Perez-Fernandez, A. Costa-Garcia and A. E. Muniz, Biosensors, 2020, 10, 32–57 CrossRef CAS.
- E. Costa-Rama and M. T. Fernandez-Abedul, Biosensors, 2021, 11, 51–73 CrossRef CAS PubMed.
- M. Rizwan and M. U. Ahmed, Inorg. Chem. Commun., 2019, 106, 54–60 CrossRef CAS.
- E. Nagles, O. García-Beltrán and J. A. Calderón, Electrochim. Acta, 2017, 258, 512–523 CrossRef CAS.
- J. Kampeera, P. Pasakon, C. Karuwan, N. Arunrut, A. Sappat, S. Sirithammajak, N. Dechokiattawan, T. Sumranwanich, P. Chaivisuthangkura, P. Ounjai, S. Chankhamhaengdecha, A. Wisitsoraat, A. Tuantranont and W. Kiatpathomchai, Biosens. Bioelectron., 2019, 132, 271–278 CrossRef CAS.
- K. F. Chan, H. N. Lim, N. Shams, S. Jayabal, A. Pandikumar and N. M. Huang, Mater. Sci. Eng., C, 2016, 58, 666–674 CrossRef CAS.
- A. Yousefi, A. Babaei and M. Delavar, J. Electroanal. Chem., 2018, 822, 1–9 CrossRef CAS.
- D. Antuna-Jimenez, M. B. Gonzalez-Garcia, D. Hernandez-Santos and P. Fanjul-Bolado, Biosensors, 2020, 10, 9–30 CrossRef CAS PubMed.
- G. Ibanez-Redin, D. Wilson, D. Goncalves and O. N. Oliveira Jr, J. Colloid Interface Sci., 2018, 515, 101–108 CrossRef CAS PubMed.
- M. Baccarin, S. J. Rowley-Neale, E. T. G. Cavalheiro, G. C. Smith and C. E. Banks, Mikrochim. Acta, 2019, 186, 200 CrossRef PubMed.
- D. A. G. Araújo, J. R. Camargo, L. A. Pradela-Filho, A. P. Lima, R. A. A. Muñoz, R. M. Takeuchi, B. C. Janegitz and A. L. Santos, Microchem. J., 2020, 158, 105297 CrossRef.
- A. M. Santos, A. Wong, T. M. Prado, E. L. Fava, O. Fatibello-Filho, M. Sotomayor and F. C. Moraes, Talanta, 2021, 224, 121804 CrossRef CAS.
- R. S. Yalow and S. A. Berson, Nature, 1959, 184, 1648–1649 CrossRef CAS.
- W. Feng and P. Ji, Biotechnol. Adv., 2011, 29, 889–895 CrossRef CAS PubMed.
- K. Yugender Goud, V. Sunil Kumar, A. Hayat, K. Vengatajalabathy Gobi, H. Song, K.-H. Kim and J. L. Marty, J. Electroanal. Chem., 2019, 832, 336–342 CrossRef CAS.
- B. Perez-Fernandez, J. V. Mercader, B. I. Checa-Orrego, A. Escosura-Muniz and A. Costa-Garcia, Analyst, 2019, 144, 2936–2941 RSC.
- B. Perez-Fernandez, J. V. Mercader, A. Abad-Fuentes, B. I. Checa- Orrego, A. Costa-Garcia and A. Escosura-Muniz, Talanta, 2020, 209, 120465 CrossRef CAS PubMed.
- S. Cinti and F. Arduini, Biosens. Bioelectron., 2017, 89, 107–122 CrossRef CAS PubMed.
- J. Mehta, P. Vinayak, S. K. Tuteja, V. A. Chhabra, N. Bhardwaj, A. K. Paul, K. H. Kim and A. Deep, Biosens. Bioelectron., 2016, 83, 339–346 CrossRef CAS PubMed.
- J. Mehta, N. Bhardwaj, S. K. Bhardwaj, S. K. Tuteja, P. Vinayak, A. K. Paul, K. H. Kim and A. Deep, Anal. Biochem., 2017, 523, 1–9 CrossRef CAS PubMed.
- W. Siangproh, W. Dungchai, P. Rattanarat and O. Chailapakul, Anal. Chim. Acta, 2011, 690, 10–25 CrossRef CAS PubMed.
- J. D. Qiu, R. P. Liang, R. Wang, L. X. Fan, Y. W. Chen and X. H. Xia, Biosens. Bioelectron., 2009, 25, 852–857 CrossRef CAS.
- X. Dai, O. Nekraseova, M. E. Hyde and R. G. Compton, Anal. Chem., 2004, 76, 5924–5929 CrossRef CAS PubMed.
- B. R. Martins, Y. O. Barbosa, C. M. R. Andrade, L. Q. Pereira, G. F. Simao, C. J. de Oliveira, D. Correia, R. T. S. Oliveira Jr, M. V. daSilva, A. C. A. Silva, N. O. Dantas, V. Rodrigues Jr, R. A. A. Munoz and R. P. Alves-Balvedi, Biosensors, 2020, 10, 81–95 CrossRef CAS.
- E. Roduner, Chem. Soc. Rev., 2006, 35, 583–592 RSC.
- C. Sauter, M. A. Emin, H. P. Schuchmann and S. Tavman, Ultrason. Sonochem., 2008, 15, 517–523 CrossRef CAS PubMed.
- R. Grillo, A. H. Rosa and L. F. Fraceto, Chemosphere, 2015, 119, 608–619 CrossRef CAS PubMed.
- J. Choosang, P. Thavarungkul, P. Kanatharana and A. Numnuam, Microchem. J., 2020, 155, 104709 CrossRef CAS.
- A. Sanchez, A. Villalonga, G. Martinez-Garcia, C. Parrado and R. Villalonga, Nanomaterials, 2019, 9, 1745–1762 CrossRef CAS PubMed.
- C. A. Razzino, V. Serafin, M. Gamella, M. Pedrero, A. Montero- Calle, R. Barderas, M. Calero, A. O. Lobo, P. Yanez-Sedeno, S. Campuzano and J. M. Pingarron, Biosens. Bioelectron., 2020, 163, 112238 CrossRef CAS.
- J. Upan, P. Banet, P.-H. Aubert, K. Ounnunkad and J. Jakmunee, Electrochim. Acta, 2020, 349, 136335 CrossRef CAS.
- P. Karami, H. Bagheri, M. Johari-Ahar, H. Khoshsafar, F. Arduini and A. Afkhami, Talanta, 2019, 202, 111–122 CrossRef CAS PubMed.
- G. Ibanez-Redin, R. H. M. Furuta, D. Wilson, F.
M. Shimizu, E. M. Materon, L. Arantes, M. E. Melendez, A. L. Carvalho, R. M. Reis, M. N. Chaur, D. Goncalves and O. N. Oliveira Jr, Mater. Sci. Eng., C, 2019, 99, 1502–1508 CrossRef CAS PubMed.
- S. Jampasa, W. Siangproh, R. Laocharoensuk, T. Vilaivan and O. Chailapakul, Talanta, 2018, 183, 311–319 CrossRef CAS PubMed.
- L. Zhao, H. Han and Z. Ma, Biosens. Bioelectron., 2018, 101, 304–310 CrossRef CAS PubMed.
- H. A. M. Faria and V. Zucolotto, Biosens. Bioelectron., 2019, 131, 149–155 CrossRef CAS PubMed.
- G. C. M. Oliveira, J. H. S. Carvalho, L. C. Brazaca, N. C. S. Vieira and B. C. Janegitz, Biosens. Bioelectron., 2020, 152, 112016 CrossRef PubMed.
- S. Sharma, H. Byrne and R. J. O'Kennedy, Essays Biochem., 2016, 60, 9–18 CrossRef PubMed.
- J. A. Ho, W. L. Hsu, W. C. Liao, J. K. Chiu, M. L. Chen, H. C. Chang and C. C. Li, Biosens. Bioelectron., 2010, 26, 1021–1027 CrossRef CAS PubMed.
- S. K. Vashist, C. K. Dixit, B. D. MacCraith and R. O'Kennedy, Analyst, 2011, 136, 4431–4436 RSC.
- A. C. Barton, S. D. Collyer, F. Davis, G. Z. Garifallou, G. Tsekenis, E. Tully, R. O'Kennedy, T. Gibson, P. A. Millner and S. P. Higson, Biosens. Bioelectron., 2009, 24, 1090–1095 CrossRef CAS PubMed.
- M. S. Inkpen, Z. F. Liu, H. Li, L. M. Campos, J. B. Neaton and L. Venkataraman, Nat. Chem., 2019, 11, 351–358 CrossRef CAS PubMed.
- M. Sharafeldin, K. McCaffrey and J. F. Rusling, Analyst, 2019, 144, 5108–5116 RSC.
- H. Yamazoe, Mater. Sci. Eng., C, 2019, 100, 209–214 CrossRef CAS PubMed.
- M. Barreiros dos Santos, J. P. Agusil, B. Prieto-Simon, C. Sporer, V. Teixeira and J. Samitier, Biosens. Bioelectron., 2013, 45, 174–180 CrossRef CAS PubMed.
- F. Malvano, R. Pilloton and D. Albanese, Sensors, 2018, 18, 2168–2178 CrossRef PubMed.
- S. Kuss, R. A. S. Couto, R. M. Evans, H. Lavender, C. C. Tang and R. G. Compton, Anal. Chem., 2019, 91, 4317–4322 CrossRef CAS PubMed.
- R. Maalouf, C. Fournier-Wirth, J. Coste, H. Chebib, Y. Saikali, O. Vittori, A. Errachid, J.-P. Cloarec, C. Martelet and N. Jaffrezic- Renault, Anal. Chem., 2007, 79, 4879–4886 CrossRef CAS PubMed.
- V. Pagliarini, D. Neagu, V. Scognamiglio, S. Pascale, G. Scordo, G. Volpe, E. Delibato, E. Pucci, A. Notargiacomo, M. Pea, D. Moscone and F. Arduini, Electrocatalysis, 2018, 10, 288–294 CrossRef.
- C. Vericat, M. E. Vela, G. Benitez, P. Carro and R. C. Salvarezza, Chem. Soc. Rev., 2010, 39, 1805–1834 RSC.
- M. Cimafonte, A. Fulgione, R. Gaglione, M. Papaianni, R. Capparelli, A. Arciello, S. Bolletti Censi, G. Borriello, R. Velotta and B. Della Ventura, Sensors, 2020, 20, 274–290 CrossRef CAS PubMed.
- B. Della Ventura, L. Schiavo, C. Altucci, R. Esposito and R. Velotta, Biomed. Opt. Express, 2011, 2, 3223–3231 CrossRef CAS PubMed.
- B. Della Ventura, M. Banchelli, R. Funari, A. Illiano, M. De Angelis, P. Taroni, A. Amoresano, P. Matteini and R. Velotta, Analyst, 2019, 144, 6871–6880 RSC.
- W. Bialobrzeska, D. Firganek, M. Czerkies, T. Lipniacki, M. Skwarecka, K. Dziabowska, Z. Cebula, N. Malinowska, D. Bigus, E. Biega, K. Pyrc, K. Pala, S. Zoledowska and D. Nidzworski, Biosensors, 2020, 10, 175–187 CrossRef CAS PubMed.
- K. Boriachek, M. K. Masud, C. Palma, H. P. Phan, Y. Yamauchi, M. S. A. Hossain, N. T. Nguyen, C. Salomon and M. J. A. Shiddiky, Anal. Chem., 2019, 91, 3827–3834 CrossRef CAS PubMed.
- M. Daneshpour, L. S. Moradi, P. Izadi and K. Omidfar, Biosens. Bioelectron., 2016, 77, 1095–1103 CrossRef CAS PubMed.
- E. Povedano, V. Ruiz-Valdepenas Montiel, M. Gamella, M. Pedrero, R. Barderas, A. Pelaez-Garcia, M. Mendiola, D. Hardisson, J. Feliu, P. Yanez-Sedeno, S. Campuzano and J. M. Pingarron, Anal. Chem., 2020, 92, 5604–5612 CrossRef CAS PubMed.
- R. M. Torrente-Rodríguez, V. Ruiz-Valdepeñas Montiel, S. Campuzano, M. Farchado-Dinia, R. Barderas, P. San Segundo-Acosta, J. J. Montoya and J. M. Pingarron, ACS Sens., 2016, 1, 896–903 CrossRef.
- E. Vargas, R. M. Torrente-Rodriguez, V. Ruiz-Valdepenas Montiel, E. Povedano, M. Pedrero, J. J. Montoya, S. Campuzano and J. M. Pingarron, Int. J. Mol. Sci., 2017, 18, 2151–2164 CrossRef PubMed.
- L. Jirakova, R. Hrstka, S. Campuzano, J. M. Pingarrón and M. Bartosik, Electroanalysis, 2019, 31, 293–302 CrossRef CAS.
- S. Sanli, H. Moulahoum, O. Ugurlu, F. Ghorbanizamani, Z. P. Gumus, S. Evran, H. Coskunol and S. Timur, Talanta, 2020, 217, 121111 CrossRef CAS PubMed.
- E. Povedano, A. Valverde, V. R. Montiel, M. Pedrero, S. Yadav, R. Barderas, P. S. Segundo-Acosta, A. Pelaez-Garcia, M. Mendiola, D. Hardisson, S. Campuzano and J. M. Pingarron, Angew. Chem., Int. Ed., 2018, 57, 8194–8198 CrossRef CAS PubMed.
- E. Povedano, V. R. Montiel, A. Valverde, F. Navarro-Villoslada, P. Yanez-Sedeno, M. Pedrero, A. Montero-Calle, R. Barderas, A. Pelaez-Garcia, M. Mendiola, D. Hardisson, J. Feliu, J. Camps, E. Rodriguez-Tomas, J. Joven, M. Arenas, S. Campuzano and J. M. Pingarron, ACS Sens., 2019, 4, 227–234 CrossRef CAS PubMed.
- L. Fabiani, M. Saroglia, G. Galata, R. De Santis, S. Fillo, V. Luca, G. Faggioni, N. D'Amore, E. Regalbuto, P. Salvatori, G. Terova, D. Moscone, F. Lista and F. Arduini, Biosens. Bioelectron., 2021, 171, 112686 CrossRef CAS PubMed.
- X. Doldan, P. Fagundez, A. Cayota, J. Laiz and J. P. Tosar, Anal. Chem., 2016, 88, 10466–10473 CrossRef CAS PubMed.
- S. D. Tallapragada, K. Layek, R. Mukherjee, K. K. Mistry and M. Ghosh, Bioelectrochemistry, 2017, 118, 25–30 CrossRef CAS PubMed.
- A. Valipour and M. Roushani, Microchim. Acta, 2017, 184, 2015–2022 CrossRef CAS.
- A. Baradoke, B. Jose, R. Pauliukaite and R. J. Forster, Electrochim. Acta, 2019, 306, 299–306 CrossRef CAS.
- P. Zhou, X. L. Yang, X. G. Wang, B. Hu, L. Zhang, W. Zhang, H. R. Si, Y. Zhu, B. Li, C. L. Huang, H. D. Chen, J. Chen, Y. Luo, H. Guo, R. D. Jiang, M. Q. Liu, Y. Chen, X. R. Shen, X. Wang, X. S. Zheng, K. Zhao, Q. J. Chen, F. Deng, L. L. Liu, B. Yan, F. X. Zhan, Y. Y. Wang, G. F. Xiao and Z. L. Shi, Nature, 2020, 579, 270–273 CrossRef CAS PubMed.
- Q. Song, X. Sun, Z. Dai, Y. Gao, X. Gong, B. Zhou, J. Wu and W. Wen, Lab Chip, 2021, 21, 1634–1660 RSC.
- J. Kudr, P. Michalek, L. Ilieva, V. Adam and O. Zitka, TrAC, Trends Anal. Chem., 2021, 136, 116192 CrossRef CAS PubMed.
- V. V. Tran, N. H. T. Tran, H. S. Hwang and M. Chang, Biosens. Bioelectron., 2021, 182, 113192 CrossRef CAS PubMed.
- A. K. Kaushik, J. S. Dhau, H. Gohel, Y. K. Mishra, B. Kateb, N.-Y. Kim and D. Y. Goswami, ACS Appl. Bio Mater., 2020, 3, 7306–7325 CrossRef CAS.
- S. Kotru, M. Klimuntowski, H. Ridha, Z. Uddin, A. A. Askhar, G. Singh and M. M. R. Howlader, TrAC, Trends Anal. Chem., 2021, 136, 116198 CrossRef CAS PubMed.
- S. S. Mahshid, S. E. Flynn and S. Mahshid, Biosens. Bioelectron., 2021, 176, 112905 CrossRef CAS PubMed.
- M. Z. H. Khan, M. R. Hasan, S. I. Hossain, M. S. Ahommed and M. Daizy, Biosens. Bioelectron., 2020, 166, 112431 CrossRef CAS PubMed.
- F. Wu, S. Zhao, B. Yu, Y. M. Chen, W. Wang, Z. G. Song, Y. Hu, Z. W. Tao, J. H. Tian, Y. Y. Pei, M. L. Yuan, Y. L. Zhang, F. H. Dai, Y. Liu, Q. M. Wang, J. J. Zheng, L. Xu, E. C. Holmes and Y. Z. Zhang, Nature, 2020, 579, 265–269 CrossRef CAS PubMed.
- S. Eissa and M. Zourob, Anal. Chem., 2021, 93, 1826–1833 CrossRef CAS PubMed.
- S. Alireza Hashemi, S. Bahrani, S. Mojtaba Mousavi, N. Omidifar, N. Ghaleh Golab Behbahan, M. Arjmand, S. Ramakrishna, K. Bagheri Lankarani, M. Moghadami, M. Shokripour, M. Firoozsani and W. H. Chiang, J. Electroanal. Chem., 2021, 894, 115341 CrossRef CAS PubMed.
- Y. Peng, Y. Pan, Z. Sun, J. Li, Y. Yi, J. Yang and G. Li, Biosens. Bioelectron., 2021, 186, 113309 CrossRef CAS PubMed.
- J. C. Abrego-Martinez, M. Jafari, S. Chergui, C. Pavel, D. Che and M. Siaj, Biosens. Bioelectron., 2022, 195, 113595 CrossRef CAS PubMed.
- H. Zhao, F. Liu, W. Xie, T. C. Zhou, J. OuYang, L. Jin, H. Li, C. Y. Zhao, L. Zhang, J. Wei, Y. P. Zhang and C. P. Li, Sens. Actuators, B, 2021, 327, 128899 CrossRef CAS PubMed.
- T. Chaibun, J. Puenpa, T. Ngamdee, N. Boonapatcharoen, P. Athamanolap, A. P. O'Mullane, S. Vongpunsawad, Y. Poovorawan, S. Y. Lee and B. Lertanantawong, Nat. Commun., 2021, 12, 802 CrossRef CAS PubMed.
- M. Alafeef, K. Dighe, P. Moitra and D. Pan, ACS Nano, 2020, 14, 17028–17045 CrossRef CAS PubMed.
- J. Munoz and M. Pumera, Chem. Eng. J., 2021, 425, 131433 CrossRef CAS PubMed.
- T. Beduk, D. Beduk, J. I. de Oliveira Filho, F. Zihnioglu, C. Cicek, R. Sertoz, B. Arda, T. Goksel, K. Turhan, K. N. Salama and S. Timur, Anal. Chem., 2021, 93, 8585–8594 CrossRef CAS PubMed.
- R. M. Torrente-Rodriguez, H. Lukas, J. Tu, J. Min, Y. Yang, C. Xu, H. B. Rossiter and W. Gao, Matter, 2020, 3, 1981–1998 CrossRef PubMed.
- H. Liu, A. Yang, J. Song, N. Wang, P. Lam, Y. Li, H. K. Law and F. Yan, Sci. Adv., 2021, 7, eabg8387 CrossRef CAS PubMed.
|
This journal is © The Royal Society of Chemistry 2022 |