The distinct role of boron doping in Sn3O4 microspheres for synergistic removal of phenols and Cr(VI) in simulated wastewater†
Received
7th August 2019
, Accepted 27th November 2019
First published on 29th November 2019
Abstract
A powerful photocatalyst is the key for photocatalytic environmental purification via sunlight harvesting and utilization. In this work, novel boron doped Sn3O4 microspheres constructed from nanoplates were designed and fabricated. Density functional theory (DFT) studies were carried out to investigate the boron doping mechanism, in which DFT predicted that boron doping would enhance the light harvesting capability and the photo-response of Sn3O4, which was proved by diffuse reflectance spectra (DRS) and transient photocurrent responses. Attributed to its unique porous structure, and enhanced light harvesting and high carrier utilization capabilities, the boron doped Sn3O4 microsphere exhibited high efficiency for Cr(IV) removal and azo dyestuff degradation. Moreover, an unexpected synergistic effect upon simultaneous removal of phenols (phenol, bisphenol A and p-chlorophenol) and Cr(VI) was observed.
Environmental significance
Wastewater from industrial production, e.g. printing, dyeing and chemical industries, brings about serious pollution to the environment. The pollutants in wastewater from industries are complex, mainly including organic dyes, phenolic organic substances, antibiotics, and inorganic heavy metal ions, such as Cr6+, Pb2+ and Hg2+. Therefore, the development of green approaches to simultaneously deal with organic pollutants or heavy metal ions in wastewater is an important issue. Here, we designed novel boron doped petal-like Sn3O4 nano/microspheres. These boron doped Sn3O4 microspheres displayed high degradation efficiency for azo dyestuffs (methyl orange, acid orange II, rhodamine B and methylene blue) and an unexpected synergistic effect upon simultaneous removal of phenols (phenol, bisphenol A and p-chlorophenol) and Cr(VI) in simulated wastewater. The work presented a new idea and method to fabricate efficient photocatalysts for wastewater purification in industrial production.
|
1. Introduction
Sn3O4, as an interesting semiconductor, has attracted increasing attention due to its unique structure, appealing electrochemical performance and practical application in catalysis.1,2 It is well known that the electrical, optical, and catalytic properties of semiconductors are strongly related to their crystal morphologies and microstructures.3–5
Among various morphological structures, three-dimensional (3D) nano/microstructures, e.g. nanotube arrays, cuboids and microspheres, are demonstrated to be better in photocatalytic performance than low dimensional planar geometries.6–8 It is reported that self-assembled 3D nanostructures possess unique properties such as porous structure and large specific surface area, which favors the multiple reflection effects such as light enhancing and light harvesting, and provides rich active sites during the photocatalytic reaction.9,10 For example, phosphorus-doped hexagonal tubular g-C3N4 exhibits better hydrogen evolution performance than bulk g-C3N4 because its tubular structure favors the enhancement of light scattering and active sites.11 Also, a 3D flower-like ZnO sphere with a large specific surface area was designed to boost hydrogen evolution and the synthesized 3D flower-like ZnO microstructure could continuously produce hydrogen for 24 h without a noble metal.12
Bare Sn3O4 exhibits obvious photocatalytic performance,13,14 but its performance still did not meet the practical requirement. Although great effort has been achieved on modification of Sn3O4, its lower light harvesting capability as well as its ability to inefficiently separate and transfer photoinduced electron–hole pairs largely restricts its photocatalytic performance and hinders its commercial application.15,16 To overcome the inherent defects of pristine semiconductors, it is believed that constructing heterojunctions is an effective strategy to solve these challenges.17,18 For example, Li et al.19 reported that a novel Z-scheme Sn3O4/g-C3N4 heterostructure synthesized by a simple two-step hydrothermal process exhibited improved separation efficiency of charge carriers and enlarged specific surface area, which largely boosted the removal efficiency of tetracycline hydrochloride in water. In another reported heterojunction system, it is found that Sn3O4 coupled with rGO exhibited excellent photocatalytic performance and stability in hydrogen evolution with respect to pristine Sn3O4.20 Moreover, element doping is another desirable means for photocatalyst modification, e.g. enhancing the light harvesting capability, enlarging the specific surface area, enhancing the redox capability or suppressing the recombination of charge carriers by induced crystal defects.21–23 For instance, a boron-doped TiO2 photocatalyst was fabricated by a solvothermal method by using tetra-butyl titanate and boric acid. Attributed to its enhanced light harvesting capability and more developed specific surface area, the boron doped TiO2 is more effective for removing endocrine disrupting compounds and non-steroidal anti-inflammatory drugs.24 Other boron doped nano-structured photocatalysts, e.g. B-doped TiO2 nanosquares,25 B-doped TiO2 nanotubes,26 B-doped carbon nanodots27 and B-doped g-C3N4/SnS2,28 were also reported, and the enhanced light absorption or photocatalytic performance for environment purification was obtained. Xie et al.29 reported an interesting strategy for designing Sn4+ self-doped hollow SnS microcubes with highly photocatalytic performance by template-assisted synthesis. However, compared with designing heterogeneous systems, there are rare reports about element doping applied to modify the photocatalytic properties of Sn3O4 microspheres.
Hence, we focused on the boron doping modification of Sn3O4. A series of novel boron doped Sn3O4 petal-like microspheres were developed and applied to environmental purification, e.g. efficient removal of Cr(VI) and organic pollutants. Moreover, we used the density functional theory (DFT) to reveal the boron doping mechanism in the Sn3O4 crystal structure. Boron doping could benefit the enhanced light harvesting, the considerable Cr(VI) adsorption, the efficient charge carrier separation and the enhanced redox abilities.
2. Experimental
2.1. Sample fabrication
All chemicals were of analytical grade. Tin(II) chloride and boric acid were purchased from Shanghai Macklin Biochemical CO., Ltd. and Aladdin Industrial Corporation, respectively. Moreover, trisodium citrate dihydrate, urea, dyes (methyl orange, acid orange II, rhodamine B and methylene blue) and 1,5-diphenylcarbazide were obtained from Sinopharm Chemical Reagent Co. Ltd. China.
The synthetic method of B0.3–Sn3O4 was performed by the following process. 3 g tin(II) chloride and 0.246 g boric acid were dissolved in 60 mL deionized water by magnetic stirring to form a transparent solution at room temperature (about 12 °C). After adding a given mass of trisodium citrate dehydrate and urea, the pH value was adjusted to 4 by using 1 M hydrochloric acid solution, which was magnetically stirred overnight. The mixed solution was transferred into a 100 mL Teflon reaction kettle, which was reacted for 12 h at 180 °C. After the reaction kettle was cooled down naturally to room temperature, the samples were washed with deionized water, ethanol and acetone several times, respectively. Then the collected samples were dried at 120 °C for 10 h under vacuum conditions and are denoted as B0.3–Sn3O4. Sn3O4 and other ratios of boron doping samples were synthesized using the same method. Here, x indicates the molar ratio (B
:
Sn).
2.2. Characterization
Powder X-ray diffraction (XRD) patterns were recorded on a Bruker AXS D8 Discover with Cu Kα (λ = 0.15418 nm), a scan rate of 0.05° s−1, a test voltage of 40 kV and an applied current of 40 mA. Transmission electron microscopy (TEM) and high-resolution transmission electron microscopy (HRTEM) were performed on a JEPL JEM-2010FEF field emission electron microscope with an accelerating voltage of 200 kV. Scanning electron microscopy (SEM) was conducted on an FLA650F with an accelerating voltage of 15 kV. The N2 adsorption/desorption isotherms were recorded on an automatic analyzer (ASAP 2020) at 77 K. Fourier transform infrared (FT-IR) spectra were obtained using a Nicolet 5700 FT-IR spectrometer while the laser Raman spectra were measured on a Via-Reflex Raman microprobe. X-ray photoelectron spectroscopy (XPS) was carried out on a PHI Quantum 2000 XPS system with a monochromatic Al Kα source and a charge neutralizer to reveal the chemical states of elements. UV-vis diffuse reflectance spectra (DRS) were analyzed using a UV-vis spectrophotometer (UV-2550, Shimadzu) calibrated with BaSO4 reference standard. Photoluminescence (PL) emission spectra were investigated on a fluorescence spectrometer (Hitachi F-4500, Japan). Electrochemical measurements were performed on an electrochemical workstation (CHI660E A15459), in which the electrolyte solution, reference electrode and counter electrode were 0.1 M Na2SO4, saturated Ag/AgCl and platinum, respectively. Electron spin resonance (ESR) spectra were detected with an electron spin resonance spectrometer (Bruker ER200-SLC, Germany), wherein 5,5-dimethyl-1-pyrroline N-oxide (DMPO: 50 Mm, 0.2 mL) was used as the stabilizer of active species in aqueous solution.
2.3. Photocatalytic performance
Degradation of organic pollutants.
The degradation of organic pollutants (10 ppm methyl orange, 10 ppm acid orange II, 10 ppm rhodamine B and 20 ppm methylene blue) was used to investigate the photocatalytic performance, which was measured on a photochemical reactor with a 400 W metal halide lamp (light intensity: 148.36 mW cm−2) simulating natural light. The suspension mixed with 50 mL organic pollutant solution and 30 mg photocatalyst was magnetically stirred in the dark for 40 min to achieve the dynamic equilibrium of adsorption and desorption. The photocatalytic system was maintained at room temperature by circulating cooled water. During the photocatalytic process, 2 mL mixed suspension was extracted after a given time interval then centrifuged and analyzed using a UV-vis spectrophotometer (UV-6300 Double Beam Spectrophotometer, Mapada Instruments). The synergistic effect between phenol (20 ppm phenol, 20 ppm bisphenol A, 20 ppm p-chlorophenol) degradation and Cr(VI) reduction was also evaluated. The degradation rate of organic pollutants was calculated by the following formula:
C
t and C0 are the instantaneous concentration and the initial concentration, respectively. The concentrations of phenols in the reaction solution were quantified using a high performance liquid chromatograph (HPLC, Sykam, German), as described in our previous literature.30
Reduction of Cr(VI).
To further investigate the photocatalytic performance of synthesized samples, the photocatalytic reduction of 10 ppm and 20 ppm Cr(VI) solutions was performed on a photochemical reactor equipped with a 400 W metal halide lamp and circulating cooled water system. Prior to irradiation, the suspension mixed with 50 mL Cr(VI) solution and 30 mg photocatalyst was magnetically stirred in the dark for 40 min to establish the dynamic equilibrium of adsorption and desorption. At a given time interval of the photocatalytic process, approximately 2 mL suspension was extracted and centrifuged then the supernatant was collected. The 1,5-diphenylcarbazide colorimetric method was applied to determine the Cr(VI) concentration in the supernatant using a UV-vis spectrophotometer.31 Typically, 1 mL reduced Cr(VI) solution and 9 mL 0.2 M H2SO4 solution were injected into a 10 mL volumetric flask, respectively. Then, sufficient 1,5-diphenylcarbazide solution was injected into the flask which was shaken for uniformity. 1,5-Diphenylcarbazide will combine with Cr(VI) to form a purple complex, which could be detected using a UV-vis spectrophotometer at 540 nm.
The removal efficiency of Cr(VI) at a given time interval was confirmed by the following expression:
Reduction rate of Cr(VI) = (1 − Ct/C0) × 100% |
where
Ct and
C0 are the instantaneous concentration at a given irradiating time interval and the initial concentration of Cr(
VI), respectively.
3. Results and discussion
3.1. Crystal properties
Fig. 1 clearly shows that the diffraction peaks at 27.08°, 31.72°, 32.31°, 33.01°, 37.07°, 50.02°, 51.69°, and 55.88° were indexed to the (111), (−210), (−121), (210), (130), (−301), (−1−32) and (2−41) planes of Sn3O4, respectively, which was well consistent with the standard pattern of anorthic-phase Sn3O4 (JCPDS no. 16-0737). Notably, although wide-angle XRD patterns reflected that no crystalline phase change was detected in boron doped samples, with respect to Sn3O4, the peak for Bx–Sn3O4 at 27.08° obviously shifted toward a high 2θ value. It was favored by Bragg's law (nλ = 2d
sin
θ) that the high 2θ value implied the shrinkage of the lattice spacing. With respect to Sn3O4, the peak at 27.08° of Bx–Sn3O4 obviously shifted toward a higher 2θ value. According to Bragg's law (nλ = 2d
sin
θ), the higher 2θ value implied the shrinkage of the lattice spacing. B3+ possesses a smaller ionic radius (ca. 0.027 nm) than that of Sn4+ (ca. 0.069 nm) and approximate Pearson absolute electronegativity (ca. 4.30 eV). Therefore, we could infer that B3+ can be successfully inserted into the crystal lattice of Sn3O4 and Sn4+ can be replaced by B3+. A similar doping mechanism was reported in previous literature.32,33
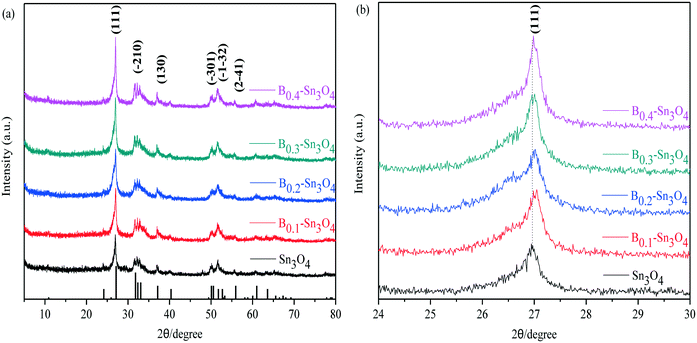 |
| Fig. 1 The XRD patterns of the samples. (a) Wide-angle XRD patterns of Sn3O4 and Bx–Sn3O4; (b) magnified patterns of the (111) crystal plane of Sn3O4 and Bx–Sn3O4. | |
Moreover, the enhanced intensity of the (111) plane was detected, indicating that boron doping improved the crystallinity of Sn3O4. Here, the Scherrer equation: D = Kλ/(β
cos
θ) was used to calculate the average crystallite size based on the strongest diffraction plane (111).34,35 The average crystallite size presented in Table 1 further confirmed the decrease of crystallite size induced by B doping. However, B0.4–Sn3O4 has a bigger average crystal size than B0.2 and B0.3-doped Sn3O4. The possible reason could be that the high B concentration could influence the growth of the Sn3O4 crystal and not benefit the doping of B into Sn3O4. Boron doping would diminish the self-diffusion velocity and interfacial energy and affect the formation of Sn3O4 crystal nuclei, which decreased the crystallite size.
Table 1 Average crystallite size of Sn3O4 and Bx–Sn3O4 calculated by the Scherrer equation
Sample |
Average crystallite size (nm) |
Sample |
Average crystallite size (nm) |
Sn3O4 |
26.48 |
B0.3–Sn3O4 |
15.92 |
B0.1–Sn3O4 |
21.59 |
B0.4–Sn3O4 |
18.26 |
B0.2–Sn3O4 |
16.79 |
|
|
3.2. Density functional theory calculation
To further reveal the boron doping mechanism and its influence on the electronic structure, density functional theory (DFT) calculation was performed to optimize the geometry and calculate the energy. First-principles calculation was performed using Material Studio based on generalized gradient approximation (GGA) in the Perdew–Burke–Ernzerhof (PBE) form. The plane wave cutoff energy was 400 eV and a 2 × 3 × 3 K-point set was adopted. Under conventional energy (10−5 eV), the unit cells were thoroughly relaxed until the total energy converges within 0.001 eV. To achieve relative positions of the highest occupied states, a slab model of four atomic layers with a vacuum layer of 20 Å thickness was carried out. In this work, Sn4+ atoms located at different positions were replaced on the basis of XRD analysis and symmetry principle. The two replaced sites are denoted as Sn3O4–B1 and Sn3O4–B2, respectively. It is shown in Fig. S1(a–c)† that the geometrically optimized crystal structures were remarkably different from the pristine crystal.
It could be seen in Fig. S1{(d1)–(f1)}† that the bottom of the conduction band and the top of the valence band were in different k-space vertical lines, revealing that Sn3O4 and boron doped Sn3O4 were indirect band gap semiconductors. Thus the electron transfer from the valence band to the conduction band was an indirect process, which is beneficial to suppressing the recombination of free charge carriers. Moreover, the calculated band gaps of Sn3O4, Sn3O4–B1 and Sn3O4–B2 were 1.76 eV, 2.16 eV and 2.20 eV. It was the Sn3O4–B1 model that was anticipated to be the optimum boron doping solution complying with the principle of more stable crystal structures and the lowest band gap energy.
As shown in Fig. S1{(d2)–(f2)},† the total density of states (TDOS) of Sn3O4 and boron doped Sn3O4 were mainly attributed to the partial density of states (PDOS) of Sn2+ p orbit, O2− s orbit and O2− p orbit. It could be confirmed that the DOS of Sn3O4 and boron doped Sn3O4 crossed the zero energy point of Fermi levels, implying a half-metallic characteristic.36 It was worth noting that an obviously enhanced density of state was observed at the conduction band edge of Sn3O4–B1 compared to that of Sn3O4, which could imply that Sn3O4–B1 would display improved photo-absorption and photo-response.37
3.3. Morphological structure
The specific morphology exhibited distinctive superior properties, such as porous structure and large specific surface area. To reveal the morphology of the as-synthesized samples, a transmission electron microscopy (TEM) test was performed. Fig. 2(a1) shows that B0.3–Sn3O4 particles are 3D microspheres with a uniform diameter of ∼300 nm. As shown in Fig. 2(a1), three lattice fringe spacing values of 0.342 nm, 0.273 nm and 0.239 nm were clearly distinguished in the high-resolution transmission electron microscopy image, which were indexed to the (−120), (210) and (130) planes of Sn3O4 (JCPDS No. 16-0737), respectively. From Fig. 2(a1), the HRTEM image, we can see that the lattice spacing for the (−120) crystal plane in B doped Sn3O4 is about 0.342 nm which is slightly smaller than that of bare Sn3O4 (0.347 nm). The possible reason could be that B doping induced the shrinkage of the lattice spacing. Moreover, elemental mapping and EDX proved that boron was homogeneously dispersed in the Sn3O4 crystal.
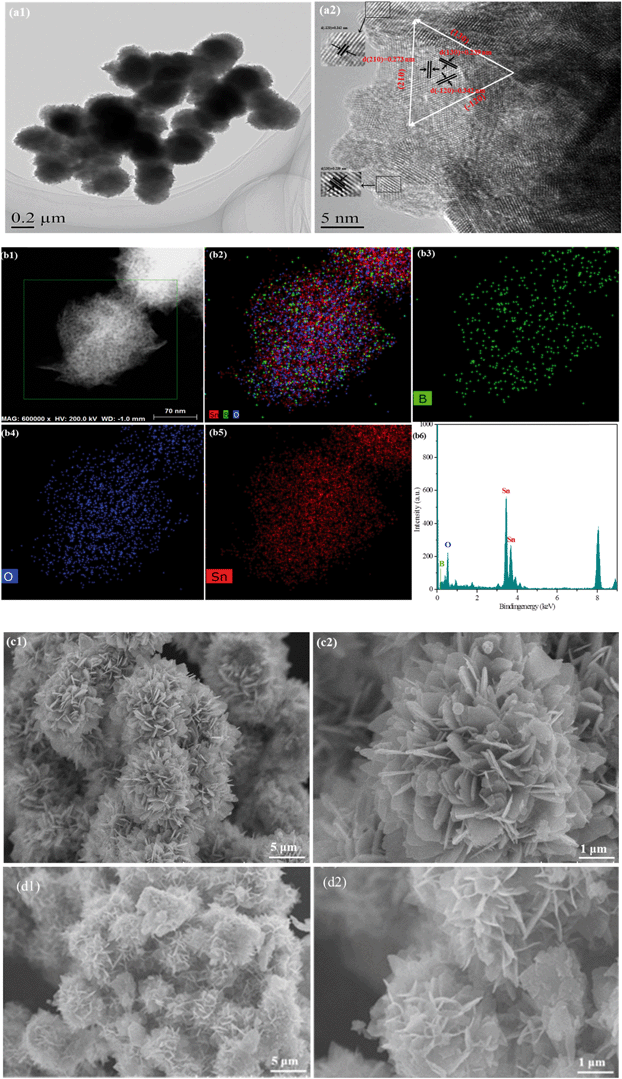 |
| Fig. 2 Morphological structure of the typical samples. (a) TEM images of B0.3–Sn3O4; (b) elemental mapping images and EDX spectra of B0.3–Sn3O4; SEM images of the samples: (c) Sn3O4; (d) B0.3–Sn3O4. | |
The scanning electron microscopy (SEM) images of Sn3O4 and Bx–Sn3O4 are shown in Fig. 2(c and d) and S2(a–c),† indicating that Sn3O4 and Bx–Sn3O4 were petal-like microspheres self-assembled from nanosheets. Although low boron content doping had a little influence on the morphological structure of Sn3O4, high boron content doping makes Sn3O4 sheets become small and the integrity of petal-like microspheres was slightly decreased.
As is well known, the smaller particle size and improved dispersion in aqueous solution would promote the photocatalytic performance. As the laser particle size shows in Fig. S3,† boron doping greatly decreased the particle size, which made the nanosheets become thinner. The smaller particle size of the catalyst implied more particle numbers in the same mass, which corresponds to improved dispersion in aqueous solution. 30 mg Sn3O4 or Bx–Sn3O4 was added in 50 mL deionized water and tested for turbidity. As shown in Table S1,† turbidity indicated that boron doping improved the dispersion of Sn3O4 in aqueous solution. Bx–Sn3O4 exhibited the highest turbidity.
3.4. Pore structure and specific surface area
The pore-size distributions (inset figure) and specific surface areas of the as-synthesized samples were studied by nitrogen adsorption–desorption isotherms. As shown in Fig. S4,† all the samples could be classified as typical type IV, indicating the presence of massive micropores induced by the strong interaction between nitrogen and materials.38 Moreover, the hysteresis loops of Sn3O4, B0.1–Sn3O4 and Bx–Sn3O4 (x = 0.2–0.4) were indexed to H2, H2 and H3, respectively.39,40 Remarkably, it was noted that the N2 sorption isotherms of Sn3O4 and B0.1–Sn3O4 show intensive adsorption in the middle and high pressure regions, implying the presence of ordered interstitial mesopores with uniform pore size and the slit-like pores constituted by the aggregated microspheres.41 However, it was clear that the N2 adsorption of Bx–Sn3O4 (x = 0.2–0.4) in the middle and high pressure regions diminished greatly compared with those of Sn3O4 and B0.1–Sn3O4, which illustrated the decrease of interstitial mesopores. It could be concluded by pore size distribution curves (inset figure) that micropores and macropores in Bx–Sn3O4 diminished remarkably, further demonstrating the influence of the doped boron content on the porosity.
The surface area and average particle size are vital to the photocatalytic performance. Table 2 shows that the reduction of grain diameter could contribute to the enlarged surface area, which was related to the doped boron content. Moreover, B0.3–Sn3O4 possessed the largest specific surface area (2.20 times that of Sn3O4). However, we observed that the pore volume and pore size of Bx–Sn3O4 greatly diminished with the increase of B content, demonstrating that boron doping destroyed the micropores and macropores, which was consistent with the regular morphology transformation confirmed by SEM analysis.
Table 2 The surface area, pore volume and average pore size
Sample |
Surface areaa (m2 g−1) |
Pore volumeb (cm3 g−1) |
Pore sizec (nm) |
BET surface area calculated by the linear part of the BET plot of P/P0 = 0.05–0.3.
Total pore volume, confirmed by the volume of N2 adsorbed at P/P0 = 0.995.
Average pore diameter estimated by the adsorption branch of the isotherm and the Barrett–Joyner–Halenda formula.
|
Sn3O4 |
31.35 |
0.126 |
16.13 |
B0.1–Sn3O4 |
36.42 |
0.052 |
15.63 |
B0.2–Sn3O4 |
53.34 |
0.066 |
5.00 |
B0.3–Sn3O4 |
69.14 |
0.078 |
5.77 |
B0.4–Sn3O4 |
55.74 |
0.071 |
6.55 |
3.5. Surface properties and compositions
Surface chemical properties were investigated by infrared spectroscopy (FT-IR). In Fig. S5,† it could be observed that the absorption peaks at 617 and 1384 cm−1 were ascribed to asymmetric and symmetric vibrations of the Sn–O–Sn bond, while the absorption peaks at 1632 cm−1 and the broad absorption peaks at 3436 cm−1 were assigned to the characteristic stretch vibration of the adsorbed water molecules and the surface hydroxyl groups, respectively.42,43 Although the vibration intensity of the typical Sn–O bond of Bx–Sn3O4 at 617 cm−1 obviously enhanced compared with that of Sn3O4, the vibration intensity of Bx–Sn3O4 weakened with the increase of the doped B content, which further confirmed the successful doping of boron.
It is known that Raman spectroscopy is an effective method to investigate the lattice strain of semiconductors.44 As Raman spectra show in Fig. 3, two major bands are located in the range of 50–250 cm−1 and 400–800 cm−1, respectively. The peaks centered at 166.6 and 203.2 cm−1 were indexed to the stretching vibration of Sn–O bonds in Sn3O4.45 Importantly, due to B3+ with a small ionic radius and high electronegativity, the substitution of Sn4+ by B3+ implied that the length of the B–O bond was smaller than that of the Sn–O bond and stronger compressive stress increased the vibrational energy and promoted Sn–O bond shift to low positions {Fig. 3(a)}.
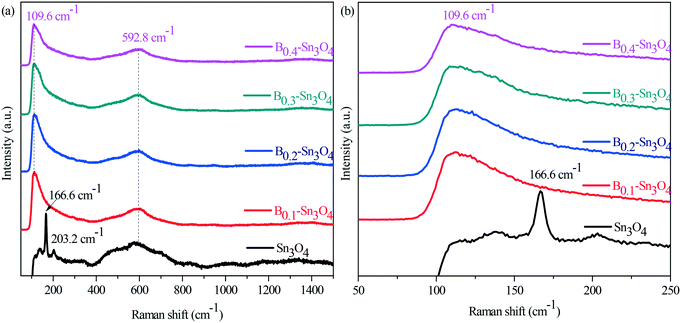 |
| Fig. 3 Raman spectra of Sn3O4 and Bx–Sn3O4. (a) The survey Raman spectra; (b) the enlarged spectra in the range of 50 nm to 250 nm. | |
Moreover, according to the relationship between Raman stretching frequency and bond length, a variety of bond lengths could be calculated by the exponential formula:
ν = 21 349 × exp(−1.9176RM − O) |
where
ν is the Raman shift and
RM–O is the metal–oxygen bond length. The lower Raman shift implied the longer bond length.
46 As shown in Table S2,
† it could be sure that boron doping induced the length growth of the Sn–O bond, producing a loosened local crystal structure. B
0.3–Sn
3O
4 possessed the longest Sn–O bond length, favoring the separation of photoinduced electron–hole pairs and their transfer over the surface of the photocatalyst.
The chemical composition and surface states of typical samples were analyzed by X-ray photoelectron spectroscopy (XPS). As shown in Fig. 4(a–e), it could be confirmed that C1s, B1s, O1s and Sn3d elements were detected using the survey and high-resolution spectra, which was consistent with the elemental mapping images and EDX analysis data. Moreover, the Cr element was the Cr adsorbed on B0.3–Sn3O4 (after five cyclic runs) during photocatalytic reduction.47 The peak of B1s centered at 191.49 eV was confirmed to be typical B–O coordination, indicating that boron replaced Sn4+ in Sn3O4 to form the B–O bond. In the broad O1s spectrum, the fitted peaks centered at 530.11 and 531.05 eV were attributed to crystal lattice oxygen and the hydroxyl group on the surface of the photocatalyst. While the peak at 532.26 eV was related to the crystal defects and oxygen vacancies induced by B3+ substitution of Sn4+.33,48
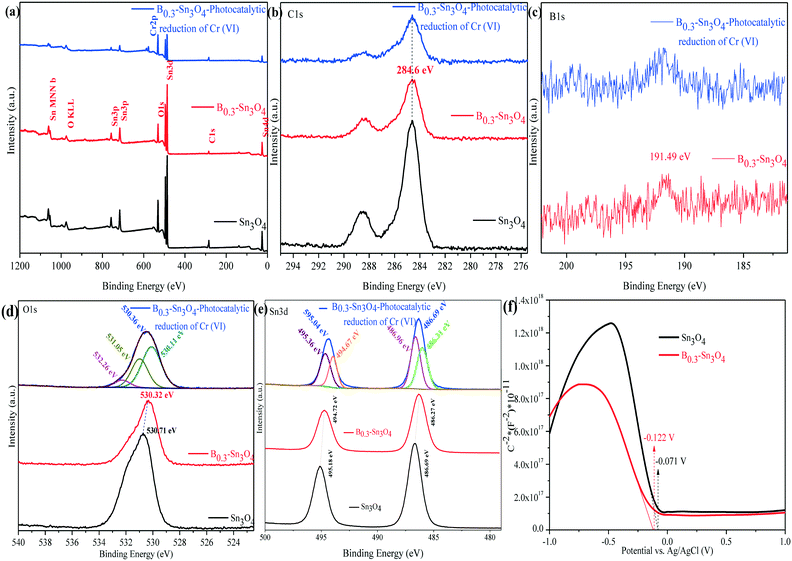 |
| Fig. 4 XPS spectra of Sn3O4, B0.3–Sn3O4 and B0.3–Sn3O4 {after photocatalytic reduction of Cr(VI)}. (a) Survey spectrum; high-resolution spectra: (b) C1s, (c) B1s, (d) O1s and (e) Sn3d; (f) Mott–Schottky curves for Sn3O4 and B0.3–Sn3O4. | |
The XPS spectrum of Sn3d at 486.69 and 595.04 eV was ascribed to Sn3d5/2 and Sn3d3/2, in which the two fitted peaks centered at 486.31 and 486.96 eV of Sn3d5/2 were assigned to Sn2+ and Sn4+, while the fitted peaks located at 494.67 and 495.36 eV correspond to Sn3d3/2 with Sn2+ and Sn4+.49 Importantly, compared with Sn3O4, the negative shifts of O (530.71 eV to 530.32 eV) and Sn {(495.18 eV to 494.72 eV and 486.69 eV to 486.27 eV)} were observed, implying the change of the electron structure of B0.3–Sn3O4 caused by the increase of the Sn–O bond length. It was confirmed by Raman analysis that the increase of the bond length may favor the separation of photoinduced electron–hole pairs, which changed the surface electron density.50 The XPS analysis result of Cr2p over the reacted B0.3–Sn3O4 will be shown later {Fig. 10(d)}.
As is well known, the Mott–Schottky method is an effective method to reveal the relationship between the Fermi level (EF) and semiconductor type as well as the electronic structure transformation in modified semiconductors. Fig. 4(f) shows the Mott–Schottky curves of the Sn3O4 and B0.3–Sn3O4 electrodes, respectively. The negative slopes of the linear region were well in line with the feature of p-type semiconductors. Importantly, in comparison with Sn3O4, the Fermi level of B0.3–Sn3O4 shifted negatively, being consistent with the negative binding energy shifts of O and Sn observed in XPS analysis, which further proved that the extension of the Sn–O bond length (proved by Raman analysis) favored the separation of photoinduced electron–hole pairs and promoted the photoinduced e− to transfer to the surface of Sn3O4. Moreover, the negative shift of the Fermi level ensured the effect of the high reduction capability.
3.6. Optical properties
Light harvesting properties largely influence the photocatalytic performance. Normally, it was believed that the hierarchical mesoporosity could make contribution to efficient path length within the spheres via multiple reflecting and scattering effects of light, which enhanced light harvesting.51 However, as proved by pore size results, the pore size of the samples decreased with the increase of doped boron content. Thus the porosity could play a secondary role in promoting the light harvesting capability. Indeed, as illustrated by the UV-vis diffuse reflectance spectra in Fig. 5(a), the obtained data revealed that the introduction of boron remarkably enhanced light harvesting across the investigated ultraviolet wavelength region and resulted in the blue shift of the light absorption edge of Bx–Sn3O4 with the increase of boron doping amount, which was also confirmed by DFT calculation.
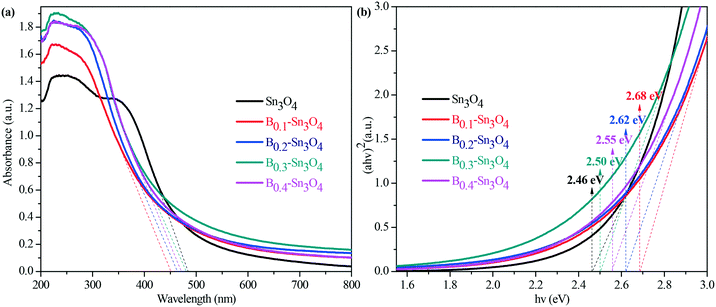 |
| Fig. 5 (a) UV-vis diffuse reflectance spectrum; (b) the plots of (ahν)2versus hν. | |
The indirect optical band gap (Eg) values of Sn3O4 and Bx–Sn3O4 shown in Fig. 5(b) were estimated according to the Tauc curve. The Tauc curve was converted by (ahν)2versus hν from the UV-vis spectra, in which a, h and ν are the absorption coefficient, Planck constant and light frequency, respectively.52 It could be considered that the Eg value of B0.3–Sn3O4 (2.50 eV) was larger than that of Sn3O4 (2.46 eV).
3.7. Photoluminescence spectra
Photoluminescence (PL) spectroscopy can be used to investigate the separation and transfer of charge carriers of semiconductors, in which the low PL emission intensity reflected the small recombination rate of charge carriers.53Fig. 6 clearly shows that the PL intensity of Bx–Sn3O4 decreased remarkably compared with Sn3O4, further proving that boron could suppress the recombination of photoinduced electron–hole pairs based on the DFT analysis, which was related to more effective crystal defects as well as small uniform particle size caused by boron doping. Furthermore, a suitable increase in doped boron content is effective to weaken the PL intensity, in which B0.3–Sn3O4 performed the lowest PL intensity.
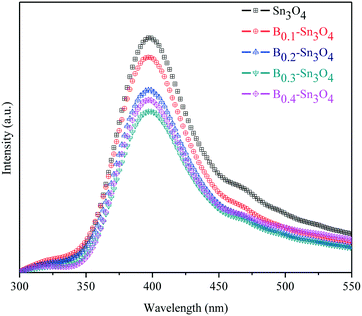 |
| Fig. 6 Photoluminescence (PL) spectra of Sn3O4 and Bx–Sn3O4. | |
3.8. Photoelectrochemical experiments
In the analysis of transient photocurrent responses, the stronger photocurrent could imply the more excellent light harvesting capability and higher separation efficiency of photogenerated charge carriers.54 It could be clearly observed in Fig. 7(a) that the rapid and invariable photocurrent responses were detected in both Sn3O4 and Bx–Sn3O4 under light illumination. The photocurrent density of Bx–Sn3O4 is remarkably stronger than that of Sn3O4, attributed to the boron doping enhancing the light harvesting capability and the generation and the separation of photoinduced free charge carriers. B0.3–Sn3O4 showed the strongest transient photocurrent (4.95 mA) which was 2.33 times that of Sn3O4 (2.12 mA). Thus the high photocatalytic performance could be anticipated over the B doped samples.
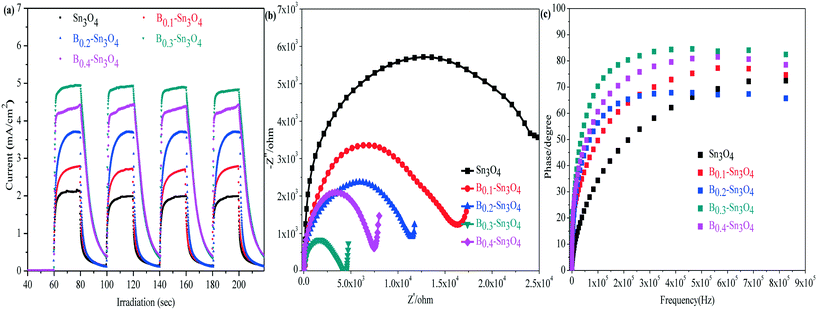 |
| Fig. 7 Photoelectrochemical measurements of Sn3O4 and Bx–Sn3O4. (a) Transient photocurrent responses of the prepared samples; (b) EIS Nyquist curves of the as-synthesized samples; (c) Bode-phase plot of the obtained samples. | |
Electrochemical impedance spectroscopy (EIS) is another valid electrochemical method to reveal the electron transfer and separation efficiency on the electrode.55 It is believed that the small EIS arc radius indicated the small charge transfer resistance between the working electrode and electrolyte solution, implying a more efficient photoinduced e− transfer capability. As illustrated in Fig. 7(b), compared with Sn3O4, the EIS arc radius of Bx–Sn3O4 shrank orderly with increasing boron content, further implying that boron doping enhanced the separation and transfer of photoinduced electron–hole pairs.
The electronic lifetime of the photocatalyst could be evaluated using the Bode-phase plot which was calculated by the following the formula: τ = 1/(2πfmax), where τ is the photoinduced electronic lifetime and fmax is the characteristic peak frequency of the intermediate frequency semicircle.56 In general, the lower characteristic peak frequency corresponded to the longer photoinduced electronic lifetime and faster electronic transfer process. It could be found in Fig. 7(c) that the fmax of Bx–Sn3O4 shifted negatively to a low peak frequency position, implying the longevity of photoinduced electrons. The calculated electronic lifetime results are shown in Table 3. The electronic lifetime of Bx–Sn3O4 remarkably increased, in which B0.3–Sn3O4 displayed the longest electronic lifetime (0.89 μs) which was 2.17 times that of Sn3O4 (0.41 μs), which further proved that the increase of Sn–O bond length favored the separation of photoinduced electron–hole pairs.
Table 3 The electronic lifetime (τ) of Sn3O4 and Bx–Sn3O4
Samples |
f
max [Hz] |
τ [μs] |
Samples |
f
max [Hz] |
τ [μs] |
Sn3O4 |
387 161 |
0.41 |
B0.3–Sn3O4 |
178 940 |
0.89 |
B0.1–Sn3O4 |
318 396 |
0.49 |
B0.4–Sn3O4 |
216 857 |
0.73 |
B0.2–Sn3O4 |
263 771 |
0.60 |
|
|
|
3.9. Photocatalytic performance and stability
To investigate the photocatalytic performance of the synthesized samples, the degradation of dyes (MO, orange II, RhB and MB) were first performed. As shown in Fig. 8(a), it was worth noting that although Sn3O4 and Bx–Sn3O4 showed high photocatalytic degradation performance for anionic dyes (MO and orange II), the degradation over RhB was inferior to that over commercial TiO2. It could be concluded that boron doping remarkably enhanced the photocatalytic performance, which followed the order B0.3–Sn3O4 > B0.4–Sn3O4 > B0.2–Sn3O4 > B0.1–Sn3O4 > Sn3O4. On the one hand, it was reported that the hierarchical/porous structure with high specific surface area and small particle size may play an important role in the photocatalytic process, providing more active sites and favoring the molecular transport of reactants, which greatly improve the photocatalytic performance.57 On the other hand, boron doping loosened the local crystal structure of Sn3O4 by increasing the length of the Sn–O bond, which facilitated the separation of photoinduced electron–hole pairs (as proved by Raman spectroscopy, XPS analysis and photoelectrochemical experiments) and promoted the free charge carriers to fleetly react with O2 and H2O. Light harvesting was also vital for the photocatalytic performance. Boron doping remarkably optimized the light harvesting properties as predicted in DFT studies and proved by DRS/photoelectrochemical measurements, which greatly increased ˙O2− and ˙OH radicals. Therefore, the improved photocatalytic activity of Bx–Sn3O4 microspheres could be attributed to their distinctive porous structure, high utilization of carriers and enhanced light harvesting capability.
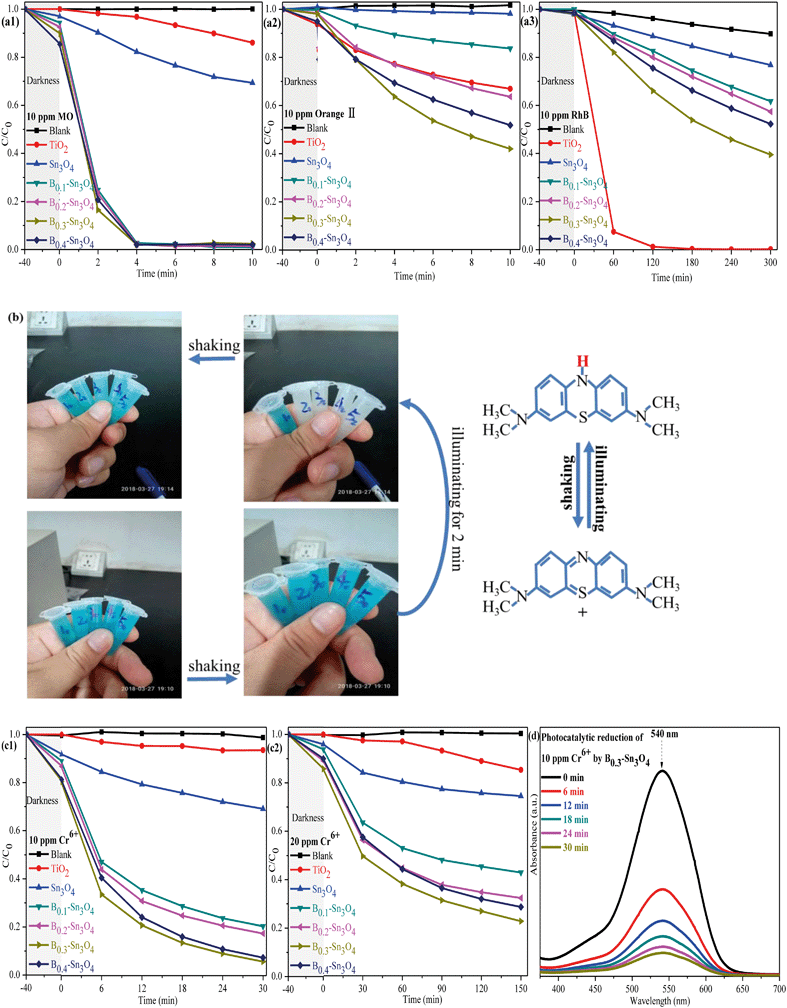 |
| Fig. 8 Comparison of the photocatalytic performance of the synthesized samples. (a) Removal of dyes (10 ppm MO, 10 ppm orange II and 10 ppm RhB); (b) reduction of 20 ppm MB; (c) removal of 10 ppm and 20 ppm Cr(VI) solution; (d) concentration change of 10 ppm Cr6+ caused by B0.3–Sn3O4 (recorded using a UV-vis spectrophotometer). | |
The 20 ppm MB solution was also applied to investigate the photocatalytic performance of the synthesized samples. The sample tubes are denoted as Xy, in which X (equal to 1, 2, 3, 4, and 5) was indexed to Sn3O4 and Bx–Sn3O4 in sequence while y represents the time of light irradiation. As indicated in Fig. 8(b), the color of the MB solution without light irradiation for 40 min (X0 = 10, 20, 30, 40, and 50) remained unchanged after shaking. After 2 min of light irradiation, although Bx–Sn3O4 (X2 = 22, 32, 42, 52) decolorized the MB solution into a colorless solution and the blue color did recover after simply shaking multiple times. Significantly, this could be defined as hydrogenation reduction caused by the irradiation process rather than photocatalytic degradation.58,59 Why could Bx–Sn3O4 except Sn3O4 reduce MB? The DFT studies and the following band structure calculation revealed that boron doping enhanced the redox capability of Sn3O4. The following formula was carried out to calculate the values of the valence band (VB) and conduction band (CB):
where
EVB,
ECB and
X are the valence band, the conduction band and the absolute electronegativity of the semiconductor, while
Ee is a constant (∼4.5 eV) defined as the free electrons on a hydrogen scale.
60,61 As shown in Table S3,
† the conduction band values of B
x–Sn
3O
4 decreased compared with Sn
3O
4, implying the enhancement of the reduction capacity, which may benefit the reduction of highly toxic Cr
6+.
Then, the removal of Cr(VI) to Cr(III) was carried out to evaluate the photocatalytic performance of the samples. As is well known, heterogeneous catalysis generally goes through seven steps: external diffusion, internal diffusion, adsorption process, catalytic reaction, desorption of reaction products, internal diffusion, and external diffusion, in which excellent adsorption performance will greatly promote the catalytic performance. Table S4† indicates that Bx–Sn3O4 showed an excellent adsorption capacity to Cr(VI) compared with bare Sn3O4 and TiO2, which was attributed to the increase of specific surface area promoting adsorption of Cr(VI). It could be found in Fig. 8(c) that the obvious adsorption of Cr(VI) was observed in the boron doped samples compared with bare Sn3O4, which was attributed to that the increase of specific surface area promoted the adsorption of Cr(VI). The synergistic effect between the adsorption and photocatalytic reaction of Bx–Sn3O4 remarkably boosted the removal of Cr(VI), in which B0.3–Sn3O4 almost completely removed 10 ppm Cr(VI) (pH 7) {including the adsorbed Cr(VI) and reduced 10 ppm Cr(VI) to Cr(III)} within 30 min. However, the removal of 20 ppm Cr6+ (pH 8) became significantly inefficient due to its high pH value. It was reported that the redox potential of Cr(VI)/Cr(III) and the conduction band of Sn3O4 would change under high pH conditions, resulting in a decrease of thermodynamic driving force of 79 mV with one pH unit increase.62 Besides, the reduced Cr(III) would easily precipitate to form Cr(OH)3 on the surface of the catalyst at high pH, covering the active sites and blocking the channel of molecular transport, which remarkably decreased the photocatalytic efficiency.63 It was worth noting that Bx–Sn3O4 showed more efficient photocatalytic performance in comparison with Sn3O4 and commercial TiO2, implying that Bx–Sn3O4 could be a promising photocatalyst for Cr(VI) removal.
As is well known, Cr(VI) species are greatly affected by the pH value of the solution. H2CrO4 and CrO42− existed under strong acid conditions and conditions above pH 7, respectively. While HCrO4− was the predominant species in the pH range of 2–7.64,65 A comparative experiment was performed to investigate the photocatalytic removal of Cr(VI) under different pH conditions adjusted with H2SO4 (1 M) and NaOH (1 M). As shown in Fig. 9(a), it could be found that the pH conditions remarkably affected the removal of Cr(VI) species. Under pH 5, the adsorption and the photocatalytic reduction of HCrO4− were enormously enhanced, in which B0.3–Sn3O4 could efficiently reduce 98.68% of HCrO4− in 18 min. However, the poor absorption and photocatalytic reduction of CrO42− were found under the conditions of pH 9, which confirmed that alkaline conditions did not benefit the removal of Cr(VI) over B0.3–Sn3O4.
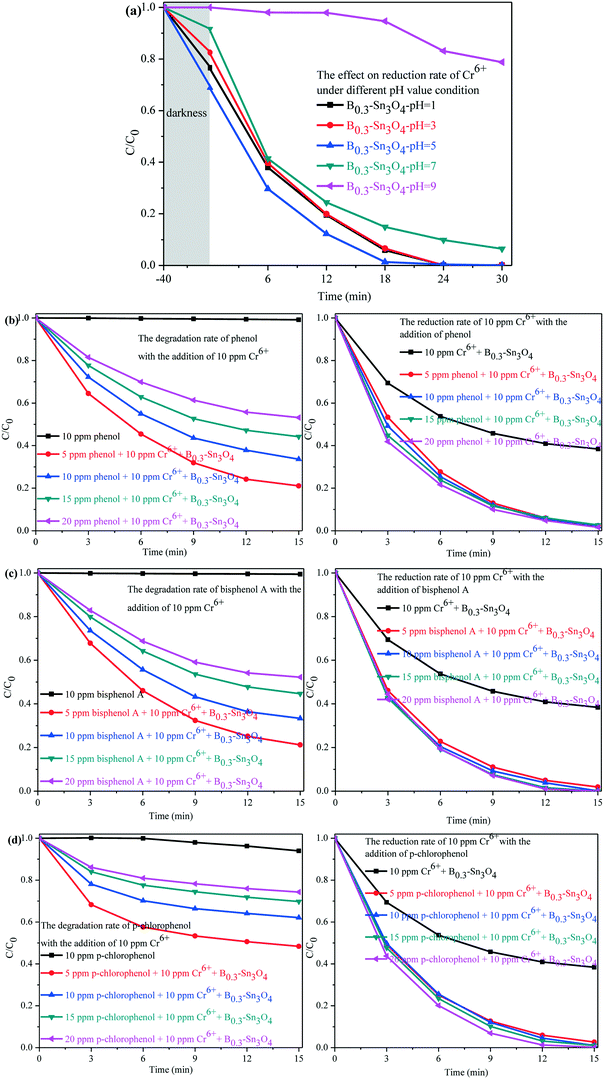 |
| Fig. 9 Comparative experiments for the reduction of Cr(VI) over B0.3–Sn3O4. (a) Photocatalytic reduction of Cr(VI) under different pH conditions; the synergistic effect between phenol degradation and Cr(VI) reduction {(b) phenol; (c) bisphenol A; (d) p-chlorophenol}. | |
Since organic pollutants and toxic heavy metals always coexist in industrial wastewater, this brings big difficulty in water purification.66,67 As shown in Fig. 9(b–d), a series of toxic phenols (phenol, bisphenol A and p-chlorophenol) with different concentrations and 10 ppm Cr(VI) solution were simulated as model industrial wastewater systems to further explore the synergistic effect between phenol degradation and Cr(VI) removal. For example, the model industrial wastewater (5 ppm phenols + 10 ppm Cr(VI)) was prepared by mixing 25 mL phenols (10 ppm) with 25 mL Cr(VI) (20 ppm). Other concentration models were used with the same method. It could be confirmed that B0.3–Sn3O4 showed little effect on phenol degradation with the absence of 10 ppm Cr(VI), which indicated that B0.3–Sn3O4 exhibits negligible degradation over phenols due to the selective-photocatalytic effect. Fortunately, both the degradation of phenols and the removal of 10 ppm Cr(VI) were greatly enhanced instantaneously due to the synergistic effect, implying that the phenols and Cr(VI) acted as sacrificial agents of h+/˙OH radicals and e−, respectively, which remarkably suppressed the recombination of photoinduced electron–hole pairs. But the removal efficiency of 10 ppm Cr(VI) increased insignificantly with the increase of phenol concentration, which indicated that the high concentration of phenols would not enhance the synergistic effect on the removal of 10 ppm Cr(VI).
Recycling tests for the degradation of MO and reduction of Cr(VI) were performed to evaluate the stability of B0.3–Sn3O4. It was observed in Fig. 10(a) that the degradation rate of MO declined slightly while the removal rate of Cr(VI) declined obviously, which was mainly attributed to the mass loss of B0.3–Sn3O4 due to the removal of the precipitated Cr(OH)3 on the catalyst surface during the washing process with HCl (1 M) and water.
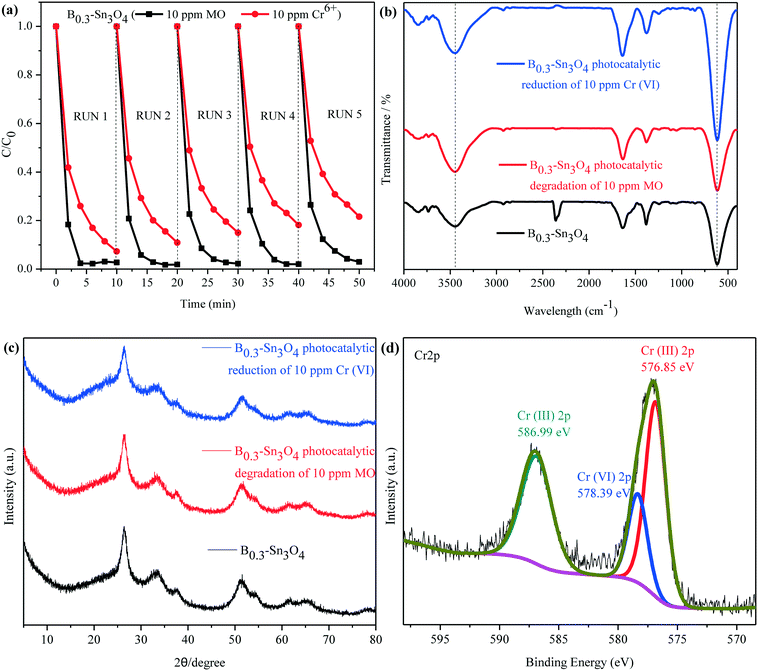 |
| Fig. 10 Photocatalytic recycling experiment of B0.3–Sn3O4. (a) Recycling runs for the degradation of MO and the removal of Cr(VI); (b) and (c) FT-IR spectra and XRD patterns of fresh and reused B0.3–Sn3O4 after five cyclic runs; (d) XPS of Cr 2p after five cyclic runs. | |
No obvious shift or new characteristic vibration peaks were observed in FT-IR for the recycled samples {Fig. 10(b)}. As for the XRD pattern presented in Fig. 10(c), no evident crystalline structure change was observed over the recycled samples. As illustrated in Fig. 10(d), the Cr 2p of B0.3–Sn3O4 after the fifth cyclic run could be curve-fitted into three peaks, where the binding energy located at 576.85 eV and 586.99 eV corresponded to typical Cr(III) 2p while 578.39 eV was ascribed to adsorbed Cr(VI) 2p caused by the large surface area, pore structure and electrostatic interaction, revealing that B0.3–Sn3O4 could effectively adsorb Cr(VI) then reduce the adsorbed Cr(VI) to Cr(III) by photoinduced electrons produced in the photocatalyst. Therefore, after the photocatalytic reduction reaction, there are both the adsorbed Cr(VI) and reduced Cr(III). Moreover, from the Gaussian fitting analysis, we can see that the ratio of Cr3+
:
Cr6+ was 4.6
:
1, revealing that most of the adsorbed Cr(VI) was reduced to Cr(III). The recycling experiment and stability characterization proved that B0.3–Sn3O4 had excellent photocatalytic stability and chemical stability, which favored the practical applications.
3.10. Photocatalytic mechanism
With the help of the DMPO (5,5-dimethyl-1-pyrroline N-oxide) trapping agent in H2O and methanol dispersion, the ESR technique was performed to detect the generation of ˙OH and ˙O2− radicals in Sn3O4 and B0.3–Sn3O4 under light illumination. In Fig. 11(a), four characteristic peaks of the DMPO–˙OH adducts were observed. The valence band of Sn3O4 (+2.46 eV) was more positive than the standard oxidation potential E0(OH−/˙OH) (+2.27 V vs. NHE).68,69 Thus, h+ could oxidize H2O to produce ˙OH. It was worth noting that the DMPO–˙OH signal of B0.3–Sn3O4 was enhanced greatly compared with bare Sn3O4, which was due to the enhanced reduction ability, the enhanced light harvesting capability in the ultraviolet region and the efficient separation of photoinduced electron–hole pairs. In Fig. 11(b), although the DMPO–˙O2− adduct signals of B0.3–Sn3O4 were detected, their intensity did not enhance greatly. Furthermore, the calculation values for the conduction band of Sn3O4 (0.19 eV) or B0.3–Sn3O4 (0.17 eV) were more positive than the standard reduction potential E0(O2/*O2−) (−0.28 V vs. NHE), implying that the intense ˙O2− signal was produced by local crystal structure defects (oxygen vacancies and tin vacancies) and donor defect sites instead of the photoinduced e−, which is similar to the phenomenon in other reports.30,70,71
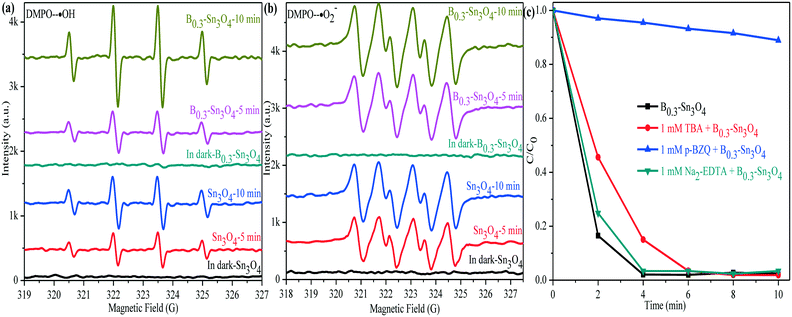 |
| Fig. 11 DMPO spin-trapping ESR spectra of Sn3O4 and B0.3–Sn3O4. (a) DMPO–˙OH; (b) DMPO–˙O2−; (c) species trapping experiments for degradation of MO. | |
To investigate the role of active species involved in the photocatalytic process, the trapped experiments for the B0.3–Sn3O4 samples in MO degradation were further performed to explore the photocatalytic mechanism. It is well known that tert-butyl alcohol (TBA, 1 mM), p-benzoquinone (p-BZQ, 1 mM) and disodium ethylenediamine-tetraacetate (Na2-EDTA) could act as the radical scavengers of hydroxyl radicals (˙OH), superoxide radicals (˙O2−) and photoinduced holes (h+), respectively.72 As the results show in Fig. 11(c), it could be clearly observed that the introduction of TBA and Na2-EDTA only slightly weakened the photocatalytic performance of B0.3–Sn3O4. However, the addition of p-BZQ remarkably restrained the MO degradation, which revealed that the ˙O2− played the main role in removing MO. It was reported that the reduction potential of Cr(VI)/Cr(III) was +0.52 V vs. NHE (pH 7) and was more positive than the conduction band of Sn3O4 and Bx–Sn3O4.73 Therefore, Cr(VI) could be reduced to Cr(III).
Based on the experiment of the synergistic effect between phenol degradation and Cr(VI) reduction, a possible mechanism was proposed. Firstly, HCrO4− was adsorbed on the Sn3O4 nanospheres. Then, HCrO4− was reduced by photoinduced electrons produced in the photocatalyst while the phenols were degraded by h+/˙OH.
phenols + h+/˙OH/+ HCrO4− + 4H+ + 3e− → Cr(OH)3 + CO2 + H2O(pH = 7). |
To prove that phenols were successfully degraded during synergistic removal between phenol degradation and Cr(VI) reduction, we investigated the total organic carbon (TOC). As is well known, the lower TOC content reveals a lower content of the organic compound (phenols in this work), which indicates that the organic pollutant was successfully degraded into inorganic carbon, such as CO32−, HCO3− or H2CO3. As confirmed in Table S5,† the TOC proved that phenols were successfully degraded during the synergistic experiments between 20 ppm phenol degradation and 10 ppm Cr(VI) reduction.
4. Conclusions
In summary, the novel and stable boron doped Sn3O4 microspheres were synthesized by a facile and low-cost hydrothermal method. XRD and DFT were applied to reveal the boron doping mechanism, in which DFT predicted the enhanced light harvesting properties and photo-response, which was further proved by DRS and transient photocurrent responses. Moreover, the excellent structural features, such as optimized specific surface area, uniform particle size and porous structure, provided more active sites and favored the transport of reactants, which played an important role in improving the photocatalytic performance. Confirmed by Raman, XPS and PL analyses, boron doping could loosen the local crystal structure of Sn3O4 by increasing the length of the Sn–O bond, which facilitates the separation of photoinduced electron–hole pairs. Finally, the synergistic effect between phenol degradation and Cr(VI) removal over boron doped Sn3O4 microspheres could provide a promising route to efficiently purify industrial wastewater.
Conflicts of interest
There are no conflicts to declare.
Acknowledgements
This research was financially supported by the National Natural Science Foundation of China (21567008 and 21707055), the Project Supported by Guangdong Province Universities and Colleges Pearl River Scholar Funded Scheme (2019), the Guangdong Basic and Applied Basic Research Foundation (2019A1515011249 and 2019A1515012130), the Key-Area Research and Development Program of Guangdong Province (2019B110206002), the program for Innovative Research Team of Guangdong University of Petrochemical Technology, the National Science Fund for Distinguished Young Scholars (21425627), the National Natural Science Foundation of China-SINOPEC Joint fund (U1663220), the Local Innovative and Research Teams Project of Guangdong Pearl River Talents Program (2017BT01C102), the Program of 5511 Talents in Scientific and Technological Innovation of Jiangxi Province (20165BCB18014), the Academic and Technical Leaders of the Main Disciplines in Jiangxi Province (20172BCB22018), the Yangfan Talents Project of Guangdong province and the Natural Science Foundation for Distinguished Young Scholars of Hunan Province, China (2017JJ1026).
References
- F. Lawson, Tin oxide-Sn3O4, Nature, 1967, 215, 955–956 CrossRef CAS.
- H. Song, S. Y. Son, S. K. Kim and G. Y. Jung, A facile synthesis of hierarchical Sn3O4 nanostructures in an acidic aqueous solution and their strong visible-light-driven photocatalytic activity, Nano Res., 2015, 8, 3553–3561 CrossRef CAS.
- N. C. Zheng, T. Ouyang, Y. B. Chen, Z. Wang, D. Y. Chen and Z. Q. Liu, Ultrathin CdS shell sensitized hollow S-doped CeO2 spheres for efficient visible-light photocatalysis, Catal. Sci. Technol., 2019, 9, 1357–1364 RSC.
- C. L. Yu, H. B. He, X. Q. Liu, J. L. Zeng and Z. Liu, Novel SiO2 nanoparticle- decorated BiOCl nanosheets exhibiting high photocatalytic performances for the removal of organic pollutants, Chin. J. Catal., 2019, 40, 1212–1221 CrossRef CAS.
- R. B. Wei, Z. L. Huang, G. H. Gu, Z. Wang, L. X. Zeng, Y. B. Chen and Z. Q. Liu, Dual-cocatalysts decorated rimous CdS spheres advancing highly-efficient visible-light photocatalytic hydrogen production, Appl. Catal., B, 2018, 231, 101–107 CrossRef CAS.
- L. J. Ji, Y. H. Zhang, S. Y. Miao, M. D. Gong and X. Liu, In situ synthesis of carbon doped TiO2 nanotubes with an enhanced photocatalytic performance under UV and visible light, Carbon, 2017, 125, 544–550 CrossRef CAS.
- Y. Guo, P. F. Wang, J. Qian, Y. H. Ao, C. Wang and J. Hou, Phosphate group grafted twinned BiPO4 with significantly enhanced photocatalytic activity: Synergistic effect of improved charge separation efficiency and redox ability, Appl. Catal., B, 2018, 234, 90–99 CrossRef CAS.
- Y. Zhang, J. R. Chen, L. Hua, S. J. Li, X. X. Zhang, W. C. Sheng and S. S. Cao, High photocatalytic activity of hierarchical SiO2 @ C-doped TiO2 hollow spheres in UV and visible light towards degradation of rhodamine B, J. Hazard. Mater., 2017, 340, 309–318 CrossRef CAS PubMed.
- Z. W. Tong, D. Yang, Z. Li, Y. H. Nan, F. Ding, Y. C. Shen and Z. Y. Jiang, Thylakoid-inspired multishell g-C3N4 nanocapsules with enhanced visible-light harvesting and electron transfer properties for high-efficiency photocatalysis, ACS Nano, 2017, 11, 1103–1112 CrossRef CAS PubMed.
- N. Tian, Y. H. Zhang, X. W. Li, K. Xiao, X. Du, F. Dong, G. I. N. Waterhouse, T. R. Zhang and H. W. Huang, Precursor-reforming protocol to 3D mesoporous g-C3N4 established by ultrathin self-doped nanosheets for superior hydrogen evolution, Nano Energy, 2017, 38, 72–81 CrossRef CAS.
- S. E. Guo, Z. P. Deng, M. X. Li, B. J. Jiang, C. G. Tian, Q. J. Pan and H. G. Fu, Phosphorus-doped carbon nitride tubes with a layered micro-nanostructure for enhanced visible-light photocatalytic hydrogen evolution, Angew. Chem., 2016, 128, 1862–1866 CrossRef.
- S. Y. Guo, T. J. Zhao, Z. Q. Jin, X. M. Wan, P. G. Wang, J. Shang and S. Han, Self-assembly synthesis of precious-metal-free 3D ZnO nano/micro spheres with excellent photocatalytic hydrogen production from solar water splitting, J. Power Sources, 2015, 293, 17–22 CrossRef CAS.
- W. W. Xia, H. Y. Qian, X. H. Zeng, J. Dong, J. Wang and Q. Xu, Visible-light self-powered photodetector and recoverable photocatalyst fabricated from vertically aligned Sn3O4 nanoflakes on carbon paper, J. Phys. Chem. C, 2017, 121, 19036–19043 CrossRef CAS.
- X. F. Chen, Y. Huang, K. C. Zhang, X. S. Feng and M. Y. Wang, Porous TiO2 nanobelts coated with mixed transition-metal oxides Sn3O4 nanosheets core-shell composites as high-performance anode materials of lithium ion batteries, Electrochim. Acta, 2018, 259, 131–142 CrossRef CAS.
- P. Niu, M. Qiao, Y. F. Li, L. Huang and T. Y. Zhai, Distinctive defects engineering in graphitic carbon nitride for greatly extended visible light photocatalytic hydrogen evolution, Nano Energy, 2018, 44, 73–81 CrossRef CAS.
- Y. X. Zeng, X. Liu, C. B. Liu, L. L. Wang, Y. C. Xia, S. Q. Zhang, S. L. Luo and Y. Pei, Scalable one-step production of porous oxygen-doped g-C3N4 nanorods with effective electron separation for excellent visible-light photocatalytic activity, Appl. Catal., B, 2018, 224, 1–9 CrossRef CAS.
- J. J. Li, B. Weng, S. C. Cai, J. Chen, H. P. Jia and Y. J. Xu, Efficient promotion of charge transfer and separation in hydrogenated TiO2/WO3 with rich surface-oxygen-vacancies for photodecomposition of gaseous toluene, J. Hazard. Mater., 2018, 342, 661–669 CrossRef CAS PubMed.
- Y. J. Zou, J. W. Shi, D. D. Ma, Z. Y. Fan, L. H. Cheng, D. K. Sun, Z. Y. Wang and C. M. Niu, WS2/Graphitic Carbon Nitride Heterojunction Nanosheets Decorated with CdS Quantum Dots for Photocatalytic Hydrogen Production, ChemSusChem, 2018, 11, 1187–1197 CrossRef CAS PubMed.
- C. M. Li, S. Y. Yu, H. J. Dong, C. B. Liu, H. J. Wu, H. N. Che and G. Chen, Z-scheme mesoporous photocatalyst constructed by modification of Sn3O4 nanoclusters on g-C3N4 nanosheets with improved photocatalytic performance and mechanism insight, Appl. Catal., B, 2018, 238, 284–293 CrossRef CAS.
- X. Yu, Z. H. Zhao, D. H. Sun, N. Ren, J. H. Yu, R. Q. Yang and H. Liu, Microwave-assisted hydrothermal synthesis of Sn3O4 nanosheet/rGO planar heterostructure for efficient photocatalytic hydrogen generation, Appl. Catal., B, 2018, 227, 470–476 CrossRef CAS.
- W. Wang, M. O. Tadé and Z. P. Shao, Nitrogen-doped simple and complex oxides for photocatalysis: a review, Prog. Mater. Sci., 2018, 92, 33–63 CrossRef CAS.
- Y. C. Huang, B. Long, M. N. Tang, Z. B. Rui, M. S. Balogun, Y. X. Tong and H. B. Ji, Bifunctional catalytic material: an ultrastable and high-performance surface defect CeO2 nanosheets for formaldehyde thermal oxidation and photocatalytic oxidation, Appl. Catal., B, 2016, 181, 779–787 CrossRef CAS.
- J. C. Yu, J. G. Yu, W. K. Ho, Z. T. Jiang and L. Z. Zhang, Effects of F- doping on the photocatalytic activity and microstructures of nanocrystalline TiO2 powders, Chem. Mater., 2002, 14, 3808–3816 CrossRef CAS.
- E. B. Simsek, Solvothermal synthesized boron doped TiO2 catalysts: photocatalytic degradation of endocrine disrupting compounds and pharmaceuticals under visible light irradiation, Appl. Catal., B, 2017, 200, 309–322 CrossRef.
- C. L. Yu, L. F. Wei, X. Li, J. C. Chen, Q. Z. Fan and J. C. Yu, Synthesis and characterization of Ag/TiO2-B nanosquares with highphotocatalytic activity under visible light irradiation, Mater. Sci. Eng., B, 2013, 178, 344–348 CrossRef CAS.
- Y. L. Su, S. Han, X. W. Zhang, X. Q. Chen and L. C. Lei, A novel boron-doped TiO2 nanotubes electrode and its visible-light-driven photoelectrocatalytic properties, Mater. Chem. Phys., 2008, 110, 239–246 CrossRef CAS.
- B. Song, Q. Wang, L. Wang, J. Lin, X. Wei, V. Murugadoss, S. D. Wu, Z. H. Guo, T. Ding and S. Y. Wei, Carbon nitride nanoplatelet photocatalysts heterostructured with B-doped carbon nanodots for enhanced photodegradation of organic Pollutants, J. Colloid Interface Sci., 2020, 559, 124–133 CrossRef CAS PubMed.
- Y. L. Wang, Y. Tian, Z. L. Lang, W. Guan and L. K. Yan, A highly efficient Z-scheme B-doped g-C3N4/SnS2 photocatalyst for CO2 reduction reaction: a computational study, J. Mater. Chem. A, 2018, 6, 21056–21063 RSC.
- Q. Y. Xie, H. Zhou, Z. L. Lv, H. Liu and H. Guo, Sn4+ self-doped hollow cubic SnS as an efficient visible-light photocatalyst for Cr (vi) reduction and detoxification of cyanide, J. Mater. Chem. A, 2017, 5, 6299–6309 RSC.
- C. L. Yu, Z. Wu, R. Y. Liu, D. D. Dionysiou, K. Yang, C. Y. Wang and H. Liu, Novel fluorinated Bi2MoO6 nanocrystals for efficient photocatalytic removal of water organic pollutants under different light source illumination, Appl. Catal., B, 2017, 209, 1–11 CrossRef CAS.
- L. Tangab, G. D. Yang, G. M. Zeng, Y. Cai, S. S. Li, Y. Y. Zhou, Y. Pang, Y. Y. Liu, Y. Zhang and B. Luna, Synergistic effect of iron doped ordered mesoporous carbon on adsorption-coupled reduction of hexavalent chromium and the relative mechanism study, Chem. Eng. J., 2014, 239, 114–122 CrossRef.
- C. M. Li, G. Chen, J. X. Sun, J. C. Rao, Z. H. Han, Y. D. Hu, W. N. Xing and C. M. Zhang, Doping effect of phosphate in Bi2WO6 and universal improved photocatalytic activity for removing various pollutants in water, Appl. Catal., B, 2016, 188, 39–47 CrossRef CAS.
- W. Wang, T. T. Ai and Q. Yu, Electrical and photocatalytic properties of boron-doped ZnO nanostructure grown on PET–ITO flexible substrates by hydrothermal method, Sci. Rep., 2017, 7, 42615 CrossRef CAS.
- J. C. Yu, J. G. Yu, W. K. Ho and L. Z. Zhang, Preparation of highly photocatalytic active nano-sized TiO2 particles via ultrasonic irradiation, Chem. Commun., 2001, 1942–1943 RSC.
- M. S. Lee, S. S. Hong and M. Mohseni, Synthesis of photocatalytic nanosized TiO2-Ag particles with sol–gel method using reduction agent, J. Mol. Catal. A: Chem., 2005, 242, 135–140 CrossRef CAS.
- J. Li, X. Y. Wu, W. F. Pan, G. K. Zhang and H. Chen, Vacancy-Rich Monolayer BiO2-x as a Highly Efficient UV, Visible, and Near-Infrared Responsive Photocatalyst, Angew. Chem., Int. Ed., 2018, 57, 491–495 CrossRef CAS PubMed.
- F. C. Lei, L. Zhang, Y. F. Sun, L. Liang, K. T. Liu, J. Q. Xu, Q. Zhang, B. C. Pan, Y. Luo and Y. Xie, Atomic-Layer-Confined Doping for Atomic-Level Insights into Visible-Light Water Splitting, Angew. Chem., 2015, 127, 9398–9402 CrossRef.
- R. Gomes, P. Bhanja and A. Bhaumik, A triazine-based covalent organic polymer for efficient CO2 adsorption, Chem. Commun., 2015, 51, 10050–10053 RSC.
- L. J. Liu, C. Y. Zhao, D. Pitts, H. L. Zhao and Y. Li, CO2 photoreduction with H2O vapor by porous MgO–TiO2 microspheres: effects of surface MgO dispersion and CO2 adsorption–desorption dynamics, Catal. Sci. Technol., 2014, 4, 1539–1546 RSC.
- M. D. Liu, J. Xu, B. Cheng, W. K. Ho and J. G. Yu, Synthesis and adsorption performance of Mg(OH)2 hexagonal nanosheet–graphene oxide composites, Appl. Surf. Sci., 2015, 332, 121–129 CrossRef CAS.
- H. Li, Y. Sun, Z. Y. Yuan, Y. P. Zhu and T. Y. Ma, Titanium Phosphonate Based Metal–Organic Frameworks with Hierarchical Porosity for Enhanced Photocatalytic Hydrogen Evolution, Angew. Chem., Int. Ed., 2018, 57, 3222–3227 CrossRef CAS PubMed.
- S. Yang, W. B. Yue, J. Zhu, Y. Ren and X. J. Yang, Graphene-based mesoporous SnO2 with enhanced electrochemical performance for lithium-ion batteries, Adv. Funct. Mater., 2013, 23, 3570–3576 CrossRef CAS.
- W. N. Xing, G. Chen, C. M. Li, Z. H. Han, Y. D. Hu and Q. Q. Meng, Doping effect of non-metal group in porous ultrathin g-C3N4 nanosheets towards synergistically improved photocatalytic hydrogen evolution, Nanoscale, 2018, 10, 5239–5245 RSC.
- X. Y. Xiao, J. Nogan, T. Beechem, G. A. Montaño, C. M. Washburn, J. Wang, S. M. Brozik, D. R. Wheeler, D. B. Burckel and R. Polsky, Lithographically-defined 3D porous networks as active substrates for surface enhanced Raman scattering, Chem. Commun., 2011, 47, 9858–9860 RSC.
- X. F. Chen, Y. Huang, T. P. Li, C. Wei, J. Yan and X. S. Feng, Self-assembly of novel hierarchical flowers-like Sn3O4 decorated on 2D graphene nanosheets hybrid as high-performance anode materials for LIBs, Appl. Surf. Sci., 2017, 405, 13–19 CrossRef CAS.
- H. Liu, J. Yuan, Z. Jiang, W. F. Shangguan, H. Einaga and Y. Teraoka, Novel photocatalyst of V-based solid solutions for overall water splitting, J. Mater. Chem., 2011, 21, 16535–16543 RSC.
- X. D. Meng, G. K. Zhang and N. Li, Bi24Ga2O39 for visible light photocatalytic reduction of Cr (VI): Controlled synthesis, facet-dependent activity and DFT study, Chem. Eng. J., 2017, 314, 249–256 CrossRef CAS.
- E. S. Junior, F. A. L. Porta, M. S. Liu, J. Andrés, J. A. Varela and E. Longo, A relationship between structural and electronic order–disorder effects and optical properties in crystalline TiO2 nanomaterials, Dalton Trans., 2015, 44, 3159–3175 RSC.
- L. P. Zhu, H. Lu, D. Hao, L. L. Wang, Z. H. Wu, L. J. Wang, P. Li and J. H. Ye, Three-dimensional lupinus-like TiO2 nanorod @ Sn3O4 nanosheet hierarchical heterostructured arrays as photoanode for enhanced photoelectrochemical performance, ACS Appl. Mater. Interfaces, 2017, 9, 38537–38544 CrossRef CAS PubMed.
- Z. C. Zhuang, Y. Li, Z. L. Li, F. Lv, Z. Q. Lang, K. N. Zhao, L. Zhou, L. Moskaleva, S. J. Guo and L. Q. Mai, MoB/g-C3N4 Interface Materials as a Schottky Catalyst to Boost Hydrogen Evolution, Angew. Chem., 2018, 130, 505–509 CrossRef.
- J. W. Fu, B. C. Zhua, W. You, M. Jaroniec and J. G. Yu, A flexible bio-inspired H2-production photocatalyst, Appl. Catal., B, 2018, 220, 148–160 CrossRef CAS.
- S. Cho, C. Ahn, J. Y. Park and S. Jeon, 3D nanostructured N-doped TiO2 photocatalysts with enhanced visible absorption, Nanoscale, 2018, 10, 9747–9751 RSC.
- Y. L. Li, P. P. Li, J. S. Wang, Y. L. Yang, W. Q. Yao, Z. Wei, J. S. Wu, X. X. Yan, X. F. Xu, Y. H. Liu and Y. F. Zhu, Water soluble graphitic carbon nitride with tunable fluorescence for boosting broad-response photocatalysis, Appl. Catal., B, 2018, 225, 519–529 CrossRef CAS.
- C. L. Yu, D. B. Zeng, F. Y. Chen, H. B. Ji, J. L. Zeng, D. H. Li and K. Yang, Construction of efficient solar-light-driven quaternary Ag3VO4/Zn3(VO4)2/Zn2V2O7/ZnO heterostructures for removing organic pollutants via phase transformation and in-situ precipitation route, Appl. Catal., A, 2019, 578, 70–82 CrossRef CAS.
- D. D. Zhou, S. S. Dong, D. W. Ki and B. E. Rittmann, Photocatalytic-induced electron transfer via anode-respiring bacteria (ARB) at an anode that intimately couples ARB and a TiO2 photocatalyst, Chem. Eng. J., 2018, 338, 745–751 CrossRef CAS.
- J. L. Yang, Q. J. Shi, R. Zhang, M. Z. Xie, X. Jiang, F. C. Wang, X. W. Chenga and W. H. Han, BiVO4 quantum tubes loaded on reduced graphene oxide aerogel as efficient photocatalyst for gaseous formaldehyde degradation, Carbon, 2018, 138, 118–124 CrossRef CAS.
- J. Jin, J. Yu, D. Guo, C. Cui and W. K. Ho, A hierarchical Z-Scheme CdS-WO3 photocatalyst with enhanced CO2 reduction activity, Small, 2015, 11, 5262–5271 CrossRef CAS PubMed.
- Q. W. Zhao, Z. Li, Q. Deng, L. C. Zhu, S. L. Luo and H. Li, Paired photoelectrocatalytic reactions of glucose driven by a photoelectrochemical fuel cell with assistance of methylene blue, Electrochim. Acta, 2016, 210, 38–44 CrossRef CAS.
- T. Limpanuparb, O. Areekul, P. Montriwat and U. Rajchakit, Blue bottle experiment: learning chemistry without knowing the chemicals, J. Chem. Educ., 2017, 94, 730–737 CrossRef CAS.
- F. Guo, W. L. Shi, H. B. Wang, M. M. Han, W. S. Guan, H. Huang, Y. Liu and Z. H. Kang, Study on highly enhanced photocatalytic tetracycline degradation of type II AgI/CuBi2O4 and Z-scheme AgBr/CuBi2O4 heterojunction photocatalysts, J. Hazard. Mater., 2018, 349, 111–118 CrossRef CAS PubMed.
- C. X. Zheng, G. P. He, X. Xiao, M. L. Lu, H. Zhong, X. X. Zuo and J. M. Nan, Selective photocatalytic oxidation of benzyl alcohol into benzaldehyde with high selectivity and conversion ratio over Bi4O5Br2 nanoflakes under blue LED irradiation, Appl. Catal., B, 2017, 205, 201–210 CrossRef CAS.
- Q. Wang, X. S. Chen, K. E. Yu, Y. Zhang and Y. Q. Cong, Synergistic photosensitized removal of Cr (VI) and Rhodamine B dye on amorphous TiO2 under visible light irradiation, J. Hazard. Mater., 2013, 246, 135–144 CrossRef PubMed.
- Q. Wang, X. D. Shi, J. J. Xu, J. C. Crittenden, E. Q. Liu, Y. Zhang and Y. Q. Cong, Highly enhanced photocatalytic reduction of Cr (VI) on AgI/TiO2 under visible light irradiation: Influence of calcination temperature, J. Hazard. Mater., 2016, 307, 213–220 CrossRef CAS PubMed.
- J. Kotaś and Z. Stasicka, Chromium occurrence in the environment and methods of its speciation, Environ. Pollut., 2000, 107, 263–283 CrossRef.
- Q. P. Wu, J. Zhao, G. H. Qin, C. Y. Wang, X. L. Tong and S. Xue, Photocatalytic reduction of Cr (VI) with TiO2 film under visible light, Appl. Catal., B, 2013, 142, 142–148 CrossRef.
- J. F. Liu, Z. S. Zhao and G. B. Jiang, Coating Fe3O4 magnetic nanoparticles with humic acid for high efficient removal of heavy metals in water, Environ. Sci. Technol., 2008, 42, 6949–6954 CrossRef CAS PubMed.
- Q. Zhang, K. Amor, S. J. G. Galer, I. Thompson and D. Porcelli, Using stable isotope fractionation factors to identify Cr (VI) reduction pathways: Metal-mineral-microbe interactions, Water Res., 2019, 151, 98–109 CrossRef CAS PubMed.
- A. Fujishima, T. N. Rao and D. A. Tryk, Titanium dioxide photocatalysis, J. Photochem. Photobiol., C, 2000, 1, 1–21 CrossRef CAS.
- J. H. Kou, C. H. Lu, J. Wang, Y. K. Chen, Z. Z. Xu and R. S. Varma, Selectivity enhancement in heterogeneous photocatalytic transformations, Chem. Rev., 2017, 117, 1445–1514 CrossRef CAS PubMed.
- F. L. Liao, Z. Y. Zeng, C. Eley, Q. Lu, X. L. Hong and S. C. E. Tsang, Electronic modulation of a copper/zinc oxide catalyst by a heterojunction for selective hydrogenation of carbon dioxide to methanol, Angew. Chem., Int. Ed., 2012, 51, 5832–5836 CrossRef CAS PubMed.
- F. Chen, Q. Yang, S. N. Wang, F. B. Yao, J. Sun, Y. L. Wang, C. Zhang, X. M. Lia, C. G. Niu, D. B. Wang and G. M. Zeng, Graphene oxide and carbon nitride nanosheets co-modified silver chromate nanoparticles with enhanced visible-light photoactivity and anti-photocorrosion properties towards multiple refractory pollutants degradation, Appl. Catal., B, 2017, 209, 493–505 CrossRef CAS.
- D. B. Zeng, K. Yang, C. L. Yu, F. Y. Chen, X. X. Li, Z. Wu and H. Liu, Phase transformation and microwave hydrothermal guided a novel double Z-scheme ternary vanadate heterojunction with highly efficient photocatalytic performance, Appl. Catal., B, 2018, 237, 449–463 CrossRef CAS.
- R. W. Liang, F. F. Jing, L. J. Shen, N. Qin and L. Wu, MIL-53 (Fe) as a highly efficient bifunctional photocatalyst for the simultaneous reduction of Cr (VI) and oxidation of dyes, J. Hazard. Mater., 2015, 287, 364–372 CrossRef CAS PubMed.
Footnotes |
† Electronic supplementary information (ESI) available. See DOI: 10.1039/c9en00899c |
‡ These authors contributed equally to this work. |
|
This journal is © The Royal Society of Chemistry 2020 |
Click here to see how this site uses Cookies. View our privacy policy here.