Factors determining the toxicity of engineered nanomaterials to Tetrahymena thermophila in freshwater: the critical role of organic matter†
Received
8th September 2019
, Accepted 5th December 2019
First published on 6th December 2019
Abstract
The environmental behavior and toxicity of nanomaterials can be significantly affected by the ubiquitous natural organic matter (NOM) of aquatic environments. However, general mechanisms governing the effects of different organic matter samples on nanomaterials remain elusive at present. Thus, the environmental behavior and toxicity of organic matter with different components or conformation, such as humic acid (HA), bovine serum albumin (BSA) and denatured BSA (DB), on five nanomaterials (i.e., Ag nanoparticles (NPs), polystyrene nanoparticles (PS NPs), TiO2 NPs, nano-zerovalent iron (NZVI), and multiwall carbon nanotubes (MWCNTs)) to Tetrahymena thermophila (T. thermophila) were studied. BSA and DB were found to significantly increase (up to 99%) the bioaccumulation of nanomaterials (Ag NPs and PS NPs) in T. thermophila by specific recognition. This increase was lower in DB-coated nanomaterials, compared to BSA-coated nanomaterials, revealing the importance of protein conformation in the specific recognition process. The toxicity results revealed that different organic matter samples mostly affected Ag NPs and NZVI (toxicity by dissolved metal ions) by altering their biological uptake. In the case of nanomaterials whose toxicity was dominated by suspended particles, the organic matter could alter the toxicity of these materials by changing their surface properties and their state in environmental media, and by scavenging free radicals, instead of altering the bioaccumulation process. In general, the toxicity of HA-coated nanomaterials was significantly lower than those of DB- or BSA-coated nanomaterials. Our results suggest that it is essential to consider the type and characteristics of the organic matter when evaluating the toxicity of NPs, since this represents a more realistic state of exposure.
Environmental significance
The bioavailability of nanomaterials (NMs) can be significantly affected by ubiquitous organic matter. However, the general mechanisms governing the effects of organic matter on NMs remain elusive. This work reported the influence mechanism of humic acid (HA), bovine serum albumin (BSA) and denatured BSA (DB) on the toxicity of NMs including metal, metal oxide, carbon, and organic NMs to Tetrahymena thermophila. Organic matter can alter the toxicity of NMs by changing their surface properties, suspension state in media, bioaccumulation and scavenging free radicals. Also, the bioaccumulation of NMs could be highly related to the bioavailability of organic matter. After all, this study summarized the general mechanisms of organic matter on various nanomaterials which was helpful to evaluate the environmental risk of NMs.
|
1. Introduction
Nanomaterials have been widely used in numerous fields, such as electronics, computers, medicine, and cosmetics owing to their special characteristics.1,2 However, the production of nanomaterials for consumer or industrial use has increased the risk of their release into aquatic ecosystems.3 A variety of nanomaterials have been detected in surface waters, which include TiO2 nanoparticles (NPs), Ag NPs, Au NPs, and so on.4,5 The environmental behavior and ecological toxicity of nanomaterials to aquatic ecosystems have been widely studied.6–8 Specifically, the significance of natural organic matter (NOM) in the environmental transformation of nanomaterials has been widely covered.6,9 NOM, which is ubiquitous in aquatic environments, typically contains a variety of complex organic compounds like humic acid (HA), proteins, polysaccharides, lipids, and so on.6,10–12 It has been established that NOM could adsorb on the surface of nanomaterials to form a layer, due to its active surface, forming the so-called eco-corona.13,14 The environmental behavior and biological toxicity of nanomaterials largely depend on the amount and type of NOM adsorbed on nano-surfaces.3,14
Many studies have reported the effects of different types of organic matter on the environmental behavior and toxicity of nanomaterials, which helps to reduce uncertainty in the risk assessment of aquatic systems.6,15 For instance, Ren et al. studied the effects of different organic matter samples on the stability of TiO2 NPs.16 Organic matter was found to affect the mobility of TiO2 NPs according to its structure, surface change, molecular size, and hydrophobicity.16 Wang et al. found that the adsorption of bovine serum albumin (BSA) could accelerate the accumulation of Ag NPs in Tetrahymena thermophila (T. thermophila) significantly, whereas HA had no effect on the bioaccumulation of Ag NPs.17 Li et al. found that the bioaccumulation of Ag NPs in rice seedlings depended on the NOM fraction, with the difference being ascribed to the types of functional groups of these fractions.18 Apart from chemical composition, the properties of organic matter also depend on its molecular conformation. For example, the proteins in aquatic environments can degrade and undergo denaturation under natural conditions (e.g., light, temperature, microorganisms, ions, etc.).11,19 However, to date, the molecular conformation of organic matter has not been confirmed to influence its biological interaction with nanomaterials.
Despite these advances, the general mechanisms that govern the effects of organic matter on nanomaterial-induced biological responses remain elusive.6,14,20 For instance, Stojiljkovic et al. reported that BSA enhanced the uptake of Au NPs in microglial cells.21 According to Wilhelm et al., BSA could reduce the uptake of maghemite nanomaterials significantly in human ovarian tumor cells by reducing the interactions between the nanoparticles and cell membrane.22 In addition, reports suggest that HA can alleviate the toxicity of polystyrene nanoparticles (PS NPs), graphene oxide (GO), CuO NPs, ZnO NPs, and Cu NPs towards Daphnia magna.23–27 However, Castro et al. found that HA could increase the toxicity of GO towards Daphnia magna.28 This makes it clear that even if the compositions of corona are the same, the manner in which the corona has an impact on the biological effects of nanomaterials also depends on the properties of nanomaterials, the species of test organisms, environmental conditions, and so on.6,14 There still remain many challenges in explaining the mechanisms of the interaction and toxicological consequences between nanomaterials and NOM. Therefore, it is necessary to study the biological effects of different nanomaterials in the presence of different organic matter samples, which would help us summarize the general rules.
In this study, HA and BSA were selected as the representatives of humic-like NOM and protein-like NOM, respectively.29 Denatured BSA (DB) was also selected to study the effects of conformational changes of organic matter on nanomaterials. Commercial Ag NPs, nano-zerovalent iron (NZVI), TiO2 NPs, and multiwall carbon nanotubes (MWCNTs) were selected, according to the report of the U.S. Environmental Protection Agency (EPA). All of them are considered to have a wide range of applications and may have human and environmental health implications. Polystyrene nanoparticles (PS NPs) were selected, since they have been considered as an emerging pollutant in recent years.30 This study also used T. thermophila, a protozoan, widely distributed in freshwater, which served as a key food chain link, to explore: (i) the environmental behavior of the above three organic matter samples and five nanomaterials in freshwater and (ii) the bioaccumulation and toxicity of the different nanomaterials at their sublethal concentrations in the presence of different organic matter samples. The mechanism of the toxicity influence of organic matter on various nanomaterials was summarized. Finally, the relationship between the bioaccumulation and toxicity of the nanomaterials was also discussed.
2. Methods and materials
2.1 Materials
NZVI (35–45 nm) and MWCNTs (length 5 μm, diameter 6–9 nm) were purchased from Sigma-Aldrich, whereas commercial polyvinyl pyrrolidone (PVP)-capped Ag NPs (15 nm) were purchased from Shanghai Huzheng Nanotechnology Co. Ltd. (China). TiO2 NPs (25 nm) were purchased from Acros Organics, whereas polystyrene latex microspheres (PS NPs, 50 nm) were purchased from Alfa Aesar, and fluorescent polystyrene nanospheres (fPS NPs, 50 nm) were purchased from Phosphorex. fPS NPs could not be used for the determination of toxicity, as the fluorescence of PS NPs interfered with the determination of ROS and cell membrane damage. The stock suspensions of all solid nanomaterials were prepared in 1 g L−1 concentrations using artificial freshwater. Artificial, moderately hard freshwater was prepared according to the U.S. Environmental Protection Agency protocol (EPA-821-R-02-013) (the composition of the artificial freshwater has been described in the ESI†).17 Both organic matter samples, viz. BSA (lyophilized powder, molecular weight (MW) of 66 kDa) and HA, were purchased from Sigma-Aldrich (USA). HA (1 g) was dissolved in NaOH solution (0.025 mol L−1, 1 L) and stirred for 2 h. Then the solution was filtered through a 0.45 μm cellulose membrane. The pH of the filtrate was adjusted to 8.0 using HCl and then filtered again. The HA solution was stored at 4 °C in the dark. A stock solution of BSA (2 g L−1) was prepared using ultrapure water. The BSA stock solution (2 g L−1) was denatured with guanidine hydrochloride solution (6 mol L−1) for 1 h in an ice bath. Then, the DB solution was filtered 3 times using a 3 kDa ultrafiltration centrifuge tube to remove guanidine hydrochloride and subsequently stored at 4 °C. Before the tests, the total organic carbon (TOC) concentrations of these stock solutions were determined using a TOC analyzer (Multi N/C 2100, Analytik Jena, Germany).
2.2 Organism culture
T. thermophila SB210 (a ciliate, pear-shaped, ca. 50 μm long and 20 μm wide) was procured from the Institute of Hydrobiology, Chinese Academy of Sciences, Wuhan. T. thermophila was cultured and maintained axenically at 28 °C in a nutrient-enriched medium (pH = 7.0) containing 2% w/w proteose peptone, 0.1% w/w yeast extract, 0.003% w/w Fe–ethylenediaminetetraacetic acid (EDTA), 100 units per mL penicillin G, 0.025 mg L−1 amphotericin B, and 100 mg L−1 streptomycin sulfate.31
2.3 Experimental procedure for the exposure of the biological culture
After 24–48 h of cultivation, cells in the logarithmic phase were centrifuged for 5 min at 2500 rpm and subsequently washed with ultrapure water twice. The precipitate was then re-suspended in artificial freshwater to obtain a concentration of 1 × 106 cells per mL for subsequent experiments using a Z2 Coulter counter (Beckman Coulter, Inc., IN). The exposure medium was prepared in a 24-well plate (Corning) and incubated for 12 h to achieve the adsorption equilibrium of organic matter on the surface of nanomaterials. Each nanomaterial sample was incubated with 10 mg L−1 (TOC) HA, BSA, or DB solution. After 12 h incubation, the cells were mixed (1
:
1 in volume) with the exposure medium to obtain a final concentration of 5 × 105 cells per mL. Cells exposed to nanomaterials separately or to freshwater were regarded as the control groups. The 24-well plate was placed in the dark at 25 °C for 6 h at 80 rpm for all the exposure processes.
2.4 Determination of the exposure concentrations of the nanomaterials
6 h-toxicity tests were designed and conducted to determine the sublethal concentrations of the different nanomaterials. The exposure concentrations of Ag NPs were 5, 10, 20, 30, 40, and 50 mg L−1. The exposure concentrations of MWCNTs were 20, 50, 100, 150, and 200 mg L−1, whereas the exposure concentrations of PS NPs, TiO2 NPs and NZVI were 20 and 200 mg L−1. After 6 h exposure, the agglomerated nanomaterials in the exposure medium were removed by centrifugation at 1000 rpm for 1 min. The cells were collected by centrifugation at 2500 rpm for 5 min and re-suspended in 1 mL of phosphate buffered saline (PBS). Then, the living cell numbers of the different exposure groups were measured using a Z2 Coulter counter. The toxicity data are shown in Fig. S1,† and the final exposure concentrations of the five nanomaterials in the following tests were 5 mg L−1 for Ag NPs, 20 mg L−1 for MWCNTs, and 200 mg L−1 for PS NPs, TiO2 NPs, and NZVI, which were the sublethal concentrations of these nanomaterials.
2.5 Physicochemical properties of the nanomaterials in freshwater
After 12 h incubation, exposure suspensions of 1 mL each were collected and centrifuged at 200
000g for 20 min. Then, 2% HNO3 was added to the supernatant of each suspension and the dissolved ions were determined by inductively coupled plasma mass spectrometry (ICP-MS, NexION 350D, PerkinElmer, America). The hydrodynamic diameter and zeta potential of the nanomaterials in the presence or absence of the three organic matter samples were determined using a Zetasizer Nano ZS instrument (Malvern, UK). The dispersity of the nanomaterials in freshwater was observed by transmission electron microscopy (TEM, JEM-2100F, Japan).
2.6 Adsorption of organic matter
The five nanomaterials at selected concentrations were incubated with 10 mg L−1 BSA, HA, or DB solutions for 12 h. After that, the nanomaterials in freshwater were separated by centrifugation (100
000g, 20 min for Ag NPs and PS NPs; 20
000g, 20 min for MWCNTs, TiO2 NPs, and NZVI) and the precipitates were gently washed once with ultrapure water and then freeze dried. Subsequently, their Fourier transform infrared (FTIR) spectra (Frontier, PerkinElmer) were recorded in the spectral range of 500–4000 cm−1. The supernatants were collected to determine the amount of organic matter adsorbed on the nanomaterials. Then, the fluorescence intensity of the supernatants at Ex/Em = 280/340 nm was measured on a fluorescence spectrometer (F-7000, HITACHI, Japan) to determine the content of BSA/DB. Meanwhile, the absorbances of the supernatants at 254 nm were measured by ultra-violet (UV) spectroscopy (U-3900H, HATACHI, Japan) to determine the content of HA.32 The ratio of adsorbed organic matter to nanomaterials was determined by dividing the adsorbed organic matter on the nanomaterials by the total concentration of organic matter present in the exposure medium (10 mg L−1).
2.7 Suspension stability of the nanomaterials in freshwater
The influence of the three organic matter samples on the suspension stability of the five nanomaterials was investigated. A TiO2 NP suspension (0.1 mL) was taken from the exposure medium from the same depth (1 cm) at 0, 0.5, 1, 2, 4, and 12 h intervals. They were then digested with 1 mL of a 68% HNO3 solution and 0.5 mL of concentrated sulfuric acid–ammonium sulfate solution at 120 °C for 2 h. Similarly, a NZVI suspension (1 mL) and an Ag NP suspension (0.1 mL) were taken and digested with 1 mL of 68% HNO3 at 120 °C for 2 h. Then, the Ti and Fe concentrations were determined by ICP-MS. A suspension of MWCNTs (2 mL) was taken and the absorbance at 800 nm was determined by UV-spectroscopy.33 The suspension of fPS NPs was analyzed by fluorescence spectrometry (F-7000, HITACHI, Japan) at Ex/Em = 550/610 nm.
2.8 Biological uptake of the nanomaterials
After 6 h exposure, the agglomerated nanomaterials in the exposure medium were separated by centrifugation at 1000 rpm for 1 min and then added to a 1% v/v formaldehyde solution to a 24-well plate. The cells were collected by centrifugation at 1000 rpm for 5 min and washed gently with 1 mL PBS (containing 10 mM EDTA) twice. Next, the cells after exposure to Ag NPs or NZVI NPs were digested with 1 mL 68% HNO3 at 120 °C for 2 h. Cells after exposure to TiO2 NPs were digested with 1 mL 68% HNO3 and 0.5 mL of a concentrated sulfuric acid–ammonium sulfate solution at 120 °C for 2 h. The bioaccumulation of Ag NPs, NZVI, and TiO2 NPs in T. thermophila was determined by ICP-MS. Cells on exposure to fPS NPs were re-suspended in 1 mL freshwater, and the bioaccumulation of fPS NPs was measured by fluorescence spectrometry at Ex/Em = 550/610 nm. The accumulation of MWCNTs was determined by gel electrophoresis (the detailed procedure is described in the ESI†).
The pathways for the uptake of the nanomaterials alone in the absence of the organic matter in T. thermophila were investigated by inhibition experiments and TEM imaging of cells. For the inhibition experiments, T. thermophila cells were incubated with 5 mg L−1 cytochalasin D (Solarbio, China) for 3 h. After removing the inhibitor-containing solution by centrifugation at 2500 rpm for 5 min, the T. thermophila cells were re-suspended in 5 mg L−1 cytochalasin D and mixed with the exposure medium. The uninhibited T. thermophila cells were also mixed with the exposure medium without cytochalasin D, following the same method used for the control group. After 6 h exposure, the bioaccumulation of the five nanomaterials in T. thermophila cells was determined following the method described above. TEM imaging of cells was conducted as described in the ESI.†
2.9 Toxicity test of the nanomaterials
After 6 h exposure, reactive oxygen species (ROS) and malondialdehyde (MDA) contents and the cell membrane damage and lysosomal membrane damage of the T. thermophila cells exposed to the five nanomaterials in the presence or absence of the organic matter were assessed. The ROS generated in T. thermophila cells was determined using 2′,7′-dichlorodihydrofluorescein diacetate (H2DCF-DA, Sigma-Aldrich, St. Louis, USA).34 The MDA content was measured using the thiobarbituric acid (TBA) method. The damage to the cell membranes of T. thermophila cells was determined using ethidium bromide (EB, Solarbio, China). The damage to the lysosomal membranes of T. thermophila cells was determined using neutral red (NR, Solabio, China).35 The procedures are described in the ESI.†
2.10 Data analysis
All data were reported as mean values ± standard deviation. One-way ANOVA tests were used to assess the significant difference between the control and treatment groups. For p < 0.05, the difference was considered to be significant and this was indicated by either asterisks or number signs, separately. Linear regression was used to determine the regression coefficient (r2) and the linear relationships were considered to be significant if p < 0.05. SPSS® Statistics 20.0 software was used to analyze all the data.
3. Results
3.1 Influence of the organic matter on the environmental behaviors of the different nanomaterials
The physico-chemical properties of the nanomaterials in the absence or presence of the organic matter are shown in Table 1 and S2.† All the nanomaterials were negatively charged in freshwater, with charges in the range of −9.55 to −24.27 mV. The three organic matter samples studied herein improved the absolute surface potentials of TiO2 NPs, NZVI, and MWCNTs. The hydrodynamic diameters (RH) demonstrated that Ag NPs, PS NPs, and fPS NPs were all well dispersed after 12 h of incubation in freshwater. As for TiO2 NPs, NZVI, and MWCNTs, they aggregated to form particles in the micrometric size range. The dispersion/aggregation status of the nanomaterials in freshwater is also shown in Fig. S2.† In the presence of the organic matter, only the RH of PS NPs increased slightly, whereas the RH of the other four nanomaterials decreased significantly in the presence of the organic matter (BSA, HA, or DB). This was particularly true for TiO2 NPs, NZVI, and MWCNTs. The coating of the organic matter on the nanomaterials could have been responsible for this behavior. The FTIR spectra of the nanomaterials after incubation with the organic matter (Fig. S3†) indicated the presence of the organic matter on the surface of the nanomaterials. The adsorption ratios of the three organic matter samples on the nanomaterials are summarized in Table 1. Ag NPs, TiO2 NPs, and NZVI exhibited a higher adsorption capacity towards BSA and DB than towards HA (p < 0.05). The adsorption capacity of Ag NPs towards DB was slightly higher than that towards BSA, which was an increase of 29.2% compared with the other nanomaterials (p = 0.06).
Table 1 Physico-chemical properties of the nanomaterials in the absence or presence of the organic matter. Values are means ± standard deviation (SD) (n = 2 or 3)
Samples |
Particle size (nm) |
Surface coating |
Organic matter |
Hydrodynamic diameters (nm) |
Zeta potential (mV) |
Adsorption ratio (%) |
Represents p < 0.05 compared to the control group (freshwater without the organic matter).
|
Ag NPs (5 mg L−1) |
15 |
PVP |
None |
60.35 ± 5.39 |
−13.73 ± 0.83 |
— |
HA |
52.84 ± 1.50a |
−13.83 ± 1.81 |
11.6 ± 0.4 |
BSA |
48.90 ± 1.13a |
−13.77 ± 1.32 |
25.7 ± 0.8 |
DB |
54.76 ± 1.92 |
−13.87 ± 1.91 |
33.2 ± 4.4 |
PS NPs (200 mg L−1) |
50 |
None |
None |
48.27 ± 0.99 |
−21.33 ± 1.50 |
— |
HA |
51.12 ± 0.89a |
−18.33 ± 0.86a |
52.0 ± 11.7 |
BSA |
49.23 ± 0.71 |
−18.63 ± 1.20a |
54.0 ± 1.8 |
DB |
50.34 ± 0.76a |
−18.70 ± 0.36a |
58.0 ± 2.9 |
TiO2 NPs (200 mg L−1) |
25 |
None |
None |
2693.67 ± 131.23 |
−9.55 ± 0.40 |
— |
HA |
1516.00 ± 110.73a |
−18.80 ± 0.60a |
62.8 ± 0.0 |
BSA |
1554.00 ± 43.49a |
−17.87 ± 0.15a |
90.9 ± 2.7 |
DB |
1884.67 ± 66.25a |
−16.97 ± 0.57a |
88.7 ± 1.3 |
NZVI (200 mg L−1) |
35–45 |
None |
None |
4275.33 ± 811.17 |
−14.03 ± 0.31 |
— |
HA |
1734.67 ± 111.20a |
−21.10 ± 0.10a |
18.6 ± 0.2 |
BSA |
2138.33 ± 189.37a |
−19.57 ± 1.23 |
34.1 ± 2.6 |
DB |
2146.33 ± 392.93a |
−17.77 ± 0.38a |
34.9 ± 0.6 |
MWCNTs (20 mg L−1) |
Diameter 6–9 nm, length 5 μm |
None |
None |
7188.00 ± 321.03 |
−12.13 ± 3.53 |
— |
HA |
2090.00 ± 245.51a |
−19.00 ± 0.85a |
33.8 ± 3.7 |
BSA |
3867.50 ± 1177.33 |
−16.60 ± 1.40a |
35.3 ± 0.6 |
DB |
4384.00 ± 1079.95 |
−17.57 ± 2.50a |
34.6 ± 1.8 |
The suspension stability of Ag NPs, fPS NPs, TiO2 NPs, NZVI, and MWCNTs in the presence or absence of the organic matter is shown in Fig. 1. The results showed that the three nanomaterials settled rapidly in freshwater (within 0.5 h). After 12 h of incubation, the fractions of the nanomaterials remaining in the water column followed the trend: MWCNTs (19.34 ± 1.56%) > TiO2 NPs (2.63 ± 0.27%) > NZVI (0.13 ± 0.03%). The presence of the three organic matter samples significantly increased the suspension stability of TiO2 NPs and MWCNTs (p < 0.05), but not that of NZVI (probably because of the weaker interaction between NZVI and the organic matter). BSA and HA improved the suspension stability of TiO2 NPs to a larger extent compared to DB (p < 0.05). As for MWCNTs, BSA, HA, and DB exhibited similar promotion properties. The results of dissolution are listed in Table S3.† Only 2% Ag+ could be found in environmental media in the case of 5 mg L−1 Ag NP exposure suspension, whereas the three organic matter samples did not affect the dissolution of Ag NPs.
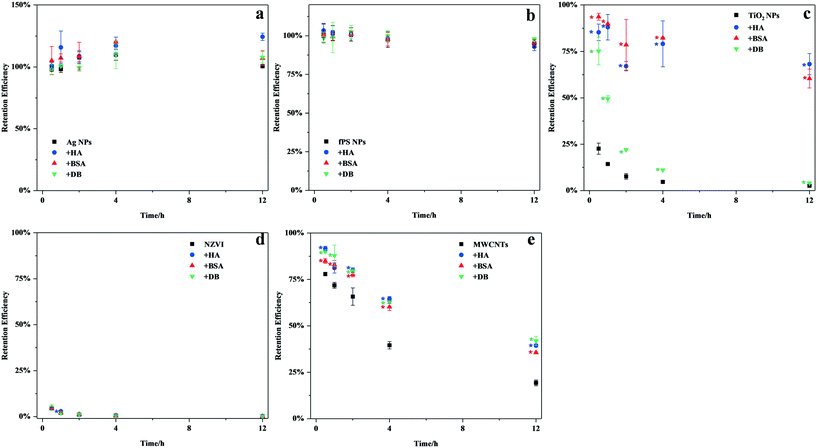 |
| Fig. 1 The suspension stability of a) Ag NPs, b) fPS NPs, c) TiO2 NPs, d) NZVI, and e) MWCNTs in the absence or presence of the organic matter (HA, BSA, or DB). Values are means ± SD (n = 2 or 3). | |
3.2 Influence of the organic matter on the biological uptake of the different nanomaterials
Next, the influence of the organic matter on the bioaccumulation of the different nanomaterials was investigated. As revealed by TEM (Fig. 2), Ag NPs, TiO2 NPs and NZVI were clearly seen distributed in the food vacuoles after 6 h of exposure. However, no bioaccumulation of MWCNTs was observed in the microscopy or TEM images (Fig. 2 and S4†). Gel-electrophoresis confirmed that there was no bioaccumulation in the case of MWCNTs (data not shown). fPS NPs were used to study the bioaccumulation of PS NPs. As shown in Table S2,† fPS NPs possessed hydrodynamic diameters and surface potential similar to PS NPs. Moreover, as revealed by FTIR, both NPs showed similar chemical compositions (Fig. S5†). Therefore, it was suggested that fPS NPs would show biological uptake behaviors similar to PS NPs. The fluorescence images in Fig. 3a and b showed bioaccumulation of fPS NPs. Then, the accumulation of the nanomaterials in T. thermophila in the presence of the organic matter was quantitatively investigated.
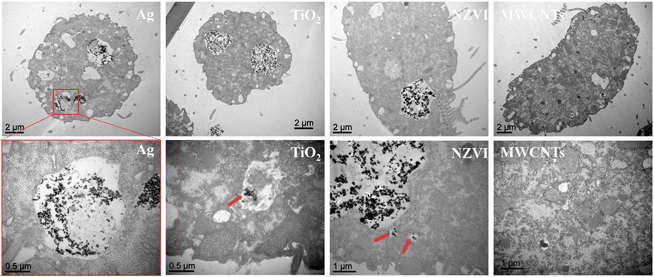 |
| Fig. 2 TEM images of T. thermophila exposed to Ag NPs, TiO2 NPs, NZVI or MWCNTs for 6 h. | |
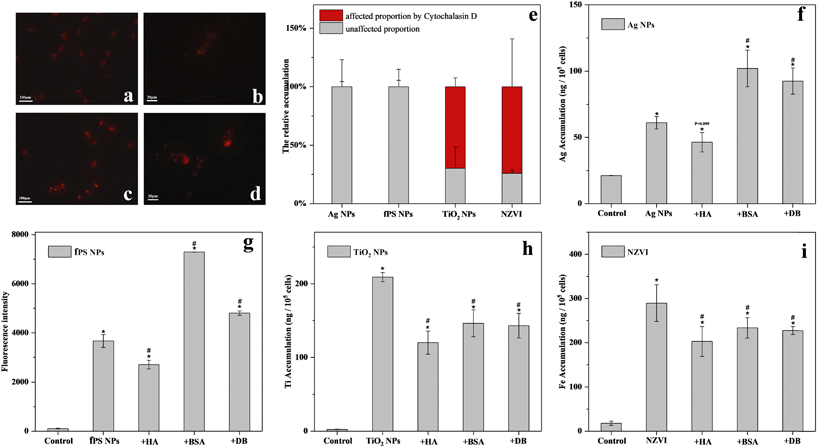 |
| Fig. 3 a and b) Fluorescence images of T. thermophila exposed to fPS NPs for 6 h, c and d) fluorescence images of T. thermophila exposed to fPS NPs and BSA for 6 h, and e) inhibition proportion of the nanomaterials bioaccumulated by T. thermophila after suppressing the actin-related phagocytosis. Bioaccumulation of nanomaterials: f) Ag NPs, g) fPS NPs, h) TiO2 NPs, and i) NZVI by T. thermophila in the presence/absence of the organic matter. Values are means ± SD (n = 3). * represents p < 0.05 (compared to the control group in fresh water). # represents p < 0.05 (compared to the control with the nanomaterials alone). | |
It can be seen in Fig. 3 that HA hindered the bioaccumulation of fPS NPs, TiO2 NPs, and NZVI. As for BSA, it promoted the bioaccumulation of Ag NPs and fPS NPs significantly (by 66.7 and 99%, respectively). In contrast, BSA reduced the bioaccumulation of TiO2 NPs and NZVI. The fluorescence images (Fig. 3a–d) revealed that BSA improved the internalization of fPS NPs. The effects of DB were similar to those of BSA (i.e., it hindered the internalization of TiO2 NPs and NZVI). As for Ag NPs and fPS NPs, DB improved the internalization by 51.1 and 31%, respectively. The promotion effect of DB on fPS NPs was significantly lower than that of BSA (p < 0.05). The promotion effect of DB on Ag NPs was nearly similar to that of BSA (p > 0.05). After suppressing the actin-related phagocytosis, the uptake of TiO2 NPs and NZVI was significantly inhibited (Fig. 3e, by 69 and 76%, respectively, p < 0.05), while the biological uptake of the other NPs remained unchanged.
3.3 Influence of the organic matter on the toxicity of the different nanomaterials
As shown in Fig. 4, Ag NPs affected the lysosomal membrane integrity of T. thermophila exclusively, leaving the ROS level and MDA content constant and the cell membrane integrity intact. The presence of HA mitigated the lysosomal membrane damage of T. thermophila induced by Ag NPs, while DB aggravated this effect. In the case of PS NPs, it induced the upregulation of the ROS level, while damaging the integrity of the cell and lysosome membranes. Similarly, HA mitigated the toxicity of PS NPs. BSA also mitigated the increase of the ROS level induced by PS NPs, but strongly increased the damage to the integrity of the cell membrane. The effect of DB resembled that of BSA, but to a lesser extent. In the case of TiO2 NPs, it increased the ROS level and interrupted the integrity of the cell membrane. HA had no effect on the toxicity of TiO2 NPs, while both BSA and DB aggravated the toxicity of this nanomaterial. As for NZVI, it increased the ROS level and MDA content and also strongly affected the lysosomal membrane integrity. HA strongly mitigated the increase of the ROS level while alleviating the lysosomal membrane damage. BSA and DB slightly reduced the ROS level and alleviated the lysosomal membrane damage. The presence of BSA and DB also reduced the MDA content significantly. The presence of MWCNTs induced excessive ROS production in T. thermophila. HA strongly mitigated the increase of the ROS level, while BSA and DB increased the ROS level in T. thermophila to a greater extent compared to MWCNTs alone.
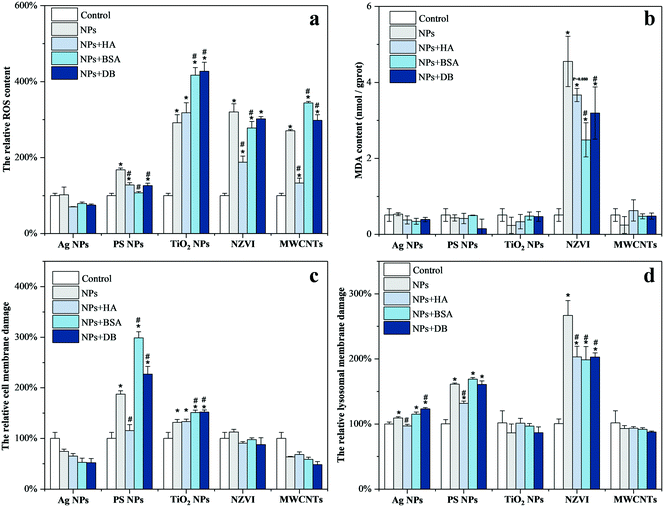 |
| Fig. 4 a) Relative ROS content, b) MDA content, c) relative cell membrane damage, and d) relative lysosomal membrane damage of T. thermophila after 6 h of exposure to the five nanomaterials in the absence or presence of the organic matter (HA, BSA, or DB). Values are means ± SD (n = 3). * represents p < 0.05 (compared to the control group in fresh water). # represents p < 0.05 (compared to the control with the the nanomaterials alone). | |
HA was found to have no mitigative effect or mitigate on the toxicity of the five nanomaterials from the toxicity results. However, as for BSA and DB, they aggravated the toxicity of Ag NPs, PS NPs, TiO2 NPs, and MWCNTs. Only in the case of NZVI, the presence of BSA and DB mitigated the toxicity of NZVI.
4. Discussion
4.1 Influence of the organic matter on the cellular uptake of the nanomaterials
The cellular uptake of Ag NPs, PS NPs, TiO2 NPs, and NZVI, except MWCNTs, could be observed. However, previous research studies have reported microscopy details and subcellular locations of MWCNTs (less than 200 nm) in the intracellular region.36 The selected MWCNTs probably had poor dispersity and large hydrodynamic diameters, since large MWCNTs were difficult to internalize.37 Considering the other four nanomaterials, HA showed reduced bioaccumulation in all cases. The biological uptake of NPs in the presence of NOM was found to depend on the adsorption ability of NPs and the cell membrane, which could be affected by electrostatic repulsion, steric hindrance, or suspension stabilization.6 Specifically, HA coating had been found to hinder the contact of the nanomaterials with organisms through steric or electrostatic repulsion.38,39 In contrast, proteins exhibited a dual characteristic in our tests, in line with previous reports.21,22 The phagocytosis process, a nonspecific intake pattern, was the prevailing uptake pathway of TiO2 NPs and NZVI. However, Ag NPs and PS NPs did not enter the cell through phagocytosis as shown in Fig. 3e, but probably entered the cell via the caveolae-mediated pathway and clathrin-mediated endocytosis pathway, which could be ascribed to smaller RH.17 Wang et al. also reported that BSA increased the uptake of NPs entering the cells through the caveolae-mediated pathway, due to the albumin receptor localized in the caveolae.17,40 Therefore, it could be speculated that BSA significantly increased the accumulation of the nanomaterials (e.g., Ag NPs and fPS NPs) entering the cells through specific recognition. In the case of the nanomaterials that entered the cells through the non-specific intake pattern (e.g., TiO2 NPs and NZVI), the adsorption of BSA hindered the adhesion onto the cell membrane,41 thereby reducing the intake of the nanomaterials.
As shown in Fig. S6,† DB showed significant peaks at 1613 and 1683 cm−1, and the intensity proportion of the peak was 50.78% at 1648 cm−1 for DB, which was higher than that of BSA at 1640 cm−1 as shown in Table S4.† These results suggested the unfolding of the α-helical structure, along with the formation of β-sheeted and more disordered structures.42,43 The conformational changes could have accounted for the lower promotion effect of DB for the bioaccumulation of fPS NPs compared with BSA. This suggested that the identification of the coating protein also depended on the protein conformation. This change in the protein conformation reduced the specificity of the biological recognition process. As for Ag NPs, DB and BSA showed similar effects on the bioaccumulation. Sulfur-bearing residues have been reported to form covalent bonds with Au or Ag.44,45 Previously, we had reported that BSA mainly attaches to Ag NPs through Ag–S bonds between the thiol group and Ag.46 Although BSA contains 35 cysteine residues, only a maximum of eight disulfide bonds can be attached to the nanosurface.44 DB contains a linear flexible molecular chain, compared to a folded structure in BSA,47,48 which could consequently result in more sulfur-bearing residues being exposed. The unraveling of the DB helix was likely to expose more sulfur residues and increase the binding ability of Ag NPs to DB (Table 1), which offsets poorer specific recognition caused by conformational changes.
4.2 Influence of the organic matter on the cytotoxicity of the nanomaterials
To date, it was considered that the toxicity of the nanomaterials could originate from: (i) a biochemical reaction induced by the dissolved ions in the environmental medium, (ii) bioaccumulated nanomaterials (e.g., oxidation stress and metal dissolution),2,49 (iii) physical50 or chemical damage by direct contact,51 or (iv) indirect disturbance by environmental factors (e.g., sunlight50,52 and nutrients53), which depend on the properties of the nanomaterials and the species of test organisms.
The exposure to Ag NPs or NZVI did not cause cellular membrane damage in T. thermophila, but resulted in significant lysosomal membrane damage. Although NZVI generated excess MDA, which is commonly considered as a marker of lipid peroxidation, no adverse effects on the cell membrane of T. thermophila were found. This suggested that the biochemical reactions caused by the accumulation of the nanomaterials in the cells accounted for the toxicity of Ag NPs and NZVI. Many studies confirmed the toxicity of metal containing NPs (e.g., metals, metal oxides, and semiconductor NPs) to be involved in the release of the corresponding toxic metal ions into the lysosomes.54,55 Considering the metal NPs (Ag NPs and NZVI) in our study, the dissolution of toxic ions (Ag+/Fe2+) in lysosomes is the main toxicity mechanism in the case of T. thermophila. Many studies have reported that Ag+ can bind to the sulfhydryl group of proteins,56 and destroy the normal physiological activities of the cells. Moreover, dissolved Fe2+ can generate hydroxyl radicals by the Fenton reaction, then attack the lipid membrane, and produce excess ROS and MDA.57,58 Oxidative stress and membrane damage were recognized to be the biotoxicity mechanisms of MWCNTs and TiO2 NPs.59–61 It clearly showed membrane damage and excessive ROS production for the TiO2 NP exposure group. However, no membrane damage was observed in the MWCNT exposure suspension. It could be explained on the basis that MWCNTs were not accessible to T. thermophila and this needs further research. The hydrophobicity of PS NPs can destroy the cell membrane structure or lead to the dysfunction of the cell membrane.24,62,63 This causes significant membrane damage and slight ROS production (the process is shown in Fig. 5). Liu et al. also reported that PS NPs were internalized by endothelial cells through caveolae/raft-mediated endocytosis and upregulated intracellular calcium, which increased the cell permeability and ultimately the generation of oxidative stress.62
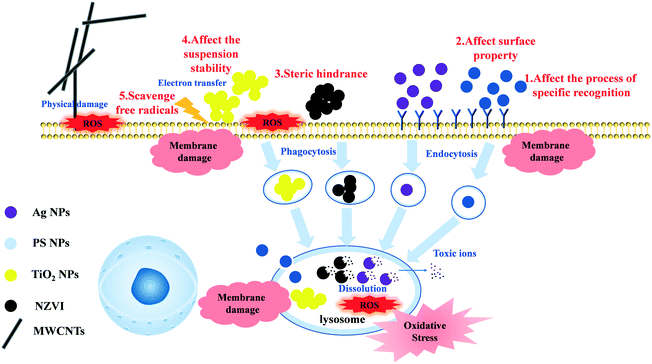 |
| Fig. 5 The schematic diagram about the toxicity mechanism of the different nanomaterials to T. thermophila and the influence mechanism of the organic matter on the nanomaterials. | |
The correlations between the biological accumulation of the nanomaterials and the positive physiological responses are described in Fig. 6. As for Ag NPs and NZVI, there were good correlations between the physiological responses and bioaccumulation in the presence or absence of different organic matter samples, suggesting that the nanomaterials coated with the organic matter showed cytotoxicity similar to their bare nanomaterials. The main effects of the organic matter on the toxicity of the nanomaterials mostly resulted from changes in the biological uptake of the nanomaterials. This viewpoint was supported by some studies.64,65 In fact, the main toxicity mechanism of these kinds of nanomaterials (e.g., Ag NPs, ZnO NPs, CuO NPs) was the dissolution of toxic ions, which occurred in the environmental media under intracellular/acidic conditions.2,66 The number of dissolved toxic ions in the environmental media could be reduced by the complexation with organic matter, which results in lower toxicity.66 However, the effect of the adsorbed organic matter on nanomaterial dissolution was not included after nanomaterials enter the cells.
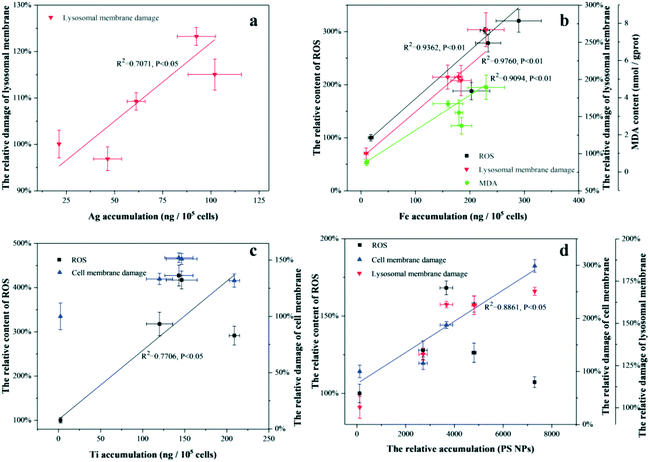 |
| Fig. 6 Correlation relationships between: a) Ag NP accumulation and the relative damage of the lysosomal membrane, b) NZVI accumulation, the relative ROS content, the relative damage of the lysosomal membrane, and the MDA content, c) TiO2 NP accumulation, the relative ROS content, and the relative damage of the cell membrane, and d) PS NP accumulation, the relative ROS content, the relative damage of the cell membrane, and the relative damage of the lysosomal membrane of T. thermophila after 6 h exposure in the absence or presence of the organic matter (HA, BSA, or DB). Values are means ± SD (n = 3). | |
TiO2 NPs and PS NPs did not show good correlations between the physiological responses and the bioaccumulation in the presence or absence of different organic matter samples as shown in Fig. 6, suggesting that the nanomaterials coated with the organic matter exhibited cytotoxicity different to the bare nanomaterials. The toxicity of TiO2 NPs and MWCNTs coated with BSA or DB increased significantly, compared to those of the bare nanomaterials. Goodwin et al. found that the dispersion state of carbon nanotubes could be an important factor in the determination of antibacterial properties.60 Ye et al. reported that natural organic matter (NOM) improved the dispersion of Al2O3 nanoparticles, thereby increasing its biological toxicity.67 Moreover, Liu et al. found that excessive generation of extracellular polymers, especially proteins, could bind the NPs to form aggregates, reducing their nanotoxicity.1 It could be inferred that the organic matter enhanced the toxicity of the nanoparticles by improving the dispersion stability of the nanomaterials. Although HA also improved the suspension stability of TiO2 NPs and MWCNTs by coating, HA is known to act as a ROS scavenger or a physical barrier, reducing the toxicity of the nanomaterials.68 This dual effect of HA resulted in lower or unchanged toxicity. In particular, the biotoxicity of DB coated TiO2 NPs was similar to that of BSA coated TiO2 NPs, although their suspension stability in exposure media was significantly weaker than that of BSA. Moreover, changes in protein conformation could have affected the toxicity of TiO2 NPs in other ways, in comparison with BSA. As for PS NPs, even though the specific biological recognition of organic matter significantly increased the bioaccumulation of BSA/DB-coated nanoparticles, the PS NPs coated with the three organic matter samples significantly reduced the generation of ROS in all cases. Wu et al. found that PS NPs coated with HA were more hydrophilic, compared to bare PS NPs, hence reduced the damage to the cell membrane.13,24 In general, for nanomaterials whose toxicity was dominated by suspended particles, organic matter could change the toxicity of the nanoparticles by altering the surface properties of the nanomaterials and the existing state of the nanomaterials in environmental media, by scavenging free radicals and steric hindrance, among other mechanisms.
The toxicity mechanisms of the nanomaterials were quite complicated (shown in Fig. 5), wherein several factors were always involved. For example, Ag NPs and CuO NPs were toxic to organisms owing to both dissolved Ag+/Cu2+ and Ag NPs/CuO NPs.2,69 Graphene affected the toxicity of algae by a masking effect and by damaging cell membranes through direct contact.50 The toxic effect of the organic matter on the nanomaterials was more complex. It was evident that the biological toxicity of the organic matter on the nanomaterials depended on the type of organic matter and the toxicity mechanisms of the nanomaterials. For nanomaterials whose main mechanism of toxicity was intracellular ion dissolution, organic matter could affect the uptake of the nanomaterials due to steric hindrance and specific recognition. The changes due to accumulation appeared to be the direct reason for changes in toxicity. For nanomaterials with particles being the main toxicity source, organic matter could change the toxicity by altering the surface properties and suspension state of the nanomaterials, acting as antioxidants.
5. Conclusions
In general, BSA and DB increased the toxicity of most of the nanomaterials by increasing their accumulation and dispersion stability. HA reduced the toxicity of most of the nanomaterials by scavenging free radicals and reducing the accumulation of the nanoparticles, except for TiO2 NPs. Our results highlighted the significant influence of the type and conformation of the organic matter on the toxicity of nanomaterials in aquatic environments. The results indicated that organic matter, which could be utilized by organisms, could result in nanomaterials exhibiting more severe effects on the organisms. More attention should be paid to the effects of bioavailable organic matter (such as proteins) on the toxicity of nanomaterials. In other words, the environmental risk of nanomaterials in domestic sewage, eutrophic rivers, and lakes (rich in active organic matter) could be severely underestimated, when compared to laboratory simulations. Furthermore, we recommend carrying out more detailed studies on the coexistence of the different environmental factors and coronas as well as contaminants with nanomaterials, since this will provide a real risk assessment of environmental nanomaterials.
Conflicts of interest
There are no conflicts to declare.
Acknowledgements
This work was supported by the National Natural Science Foundation of China (Grant No. 41977352 and 51778031).
References
- J. Z. Liu, J. Tang, J. J. Wan, C. X. Wu, B. Graham, P. G. Kerr and Y. H. Wu, Functional sustainability of periphytic biofilms in organic matter and Cu2+ removal during prolonged exposure to TiO2 nanoparticles, J. Hazard. Mater., 2019, 370, 4–12 CrossRef CAS PubMed.
- A. Ivask, K. Juganson, O. Bondarenko, M. Mortimer, V. Aruoja, K. Kasemets, I. Blinova, M. Heinlaan, V. Slaveykova and A. Kahru, Mechanisms of toxic action of Ag, ZnO and CuO nanoparticles to selected ecotoxicological test organisms and mammalian cells in vitro: A comparative review, Nanotoxicology, 2014, 8, 57–71 CrossRef CAS PubMed.
- G. V. Lowry, K. B. Gregory, S. C. Apte and J. R. Lead, Transformations of Nanomaterials in the Environment, Environ. Sci. Technol., 2012, 46, 6893–6899 CrossRef CAS.
- A. A. Markus, P. Krystek, P. C. Tromp, J. R. Parsons, E. W. M. Roex, P. de Voogt and R. W. P. M. Laane, Determination of metal-based nanoparticles in the river Dommel in the Netherlands via ultrafiltration, HR-ICP-MS and SEM, Sci. Total Environ., 2018, 631–632, 485–495 CrossRef CAS.
- R. J. B. Peters, G. van Bemmel, N. B. L. Milani, G. C. T. den Hertog, A. K. Undas, M. van der Lee and H. Bouwmeester, Detection of nanoparticles in Dutch surface waters, Sci. Total Environ., 2018, 621, 210–218 CrossRef CAS PubMed.
- Z. Y. Wang, L. Zhang, J. Zhao and B. S. Xing, Environmental processes and toxicity of metallic nanoparticles in aquatic systems as affected by natural organic matter, Environ. Sci.: Nano, 2016, 3, 240–255 RSC.
- A. S. Adeleye, L. M. Stevenson, Y. M. Su, R. M. Nisbet, Y. L. Zhang and A. A. Keller, Influence of Phytoplankton on Fate and Effects of Modified Zerovalent Iron Nanoparticles, Environ. Sci. Technol., 2016, 50, 5597–5605 CrossRef CAS PubMed.
- Y. J. Jung, G. Metreveli, C. B. Park, S. Baik and G. E. Schaumann, Implications of Pony Lake Fulvic Acid for the Aggregation and Dissolution of Oppositely Charged Surface-Coated Silver Nanoparticles and Their Ecotoxicological Effects on Daphnia magna, Environ. Sci. Technol., 2018, 52, 436–445 CrossRef CAS PubMed.
- A. Philippe and G. E. Schaumann, Interactions of Dissolved Organic Matter with Natural and Engineered Inorganic Colloids: A Review, Environ. Sci. Technol., 2014, 48, 8946–8962 CrossRef CAS PubMed.
- R. Grillo, A. H. Rosa and L. F. Fraceto, Engineered nanoparticles and organic matter: A review of the state-of-the-art, Chemosphere, 2015, 119, 608–619 CrossRef CAS PubMed.
- F. G. Meng, G. C. Huang, X. Yang, Z. Q. Li, J. Li, J. Cao, Z. G. Wang and L. Sun, Identifying the sources and fate of anthropogenically impacted dissolved organic matter (DOM) in urbanized rivers, Water Res., 2013, 47, 5027–5039 CrossRef CAS PubMed.
- H. Lin, X. H. Xia, S. Q. Bi, X. M. Jiang, H. T. Wang, Y. W. Zhai and W. Wen, Quantifying Bioavailability of Pyrene Associated with Dissolved Organic Matter of Various Molecular Weights to Daphnia magna, Environ. Sci. Technol., 2018, 52, 644–653 CrossRef CAS PubMed.
- O. O. Fadare, B. Wan, L. H. Guo, Y. Xin, W. P. Qin and Y. Yang, Humic acid alleviates the toxicity of polystyrene nanoplastic particles to Daphnia magna, Environ. Sci.: Nano, 2019, 6, 1466–1477 RSC.
- G. Pulido-Reyes, F. Leganes, F. Fernandez-Pinas and R. Rosal, Bio-nano interface and environment: A critical review, Environ. Toxicol. Chem., 2017, 36, 3181–3193 CrossRef CAS PubMed.
- Z. M. Dong, Y. J. Liu, L. C. Duan, D. Bekele and R. Naidu, Uncertainties in human health risk assessment of environmental contaminants: A review and perspective, Environ. Int., 2015, 85, 120–132 CrossRef CAS PubMed.
- M. J. Ren, H. Horn and F. H. Frimmel, Aggregation behavior of TiO2 nanoparticles in municipal effluent: Influence of ionic strengthen and organic compounds, Water Res., 2017, 123, 678–686 CrossRef CAS PubMed.
- X. R. Wang, D. Y. Liang, Y. Wang, Q. Q. Ma, B. S. Xing and W. H. Fan, Effects of organic matter on uptake and intracellular trafficking of nanoparticles in Tetrahymena thermophila, Environ. Sci.: Nano, 2019, 6, 2116–2128 RSC.
- M. Li, F. Dang, Q. L. Fu, D. M. Zhou and B. Yin, Effects of molecular weight-fractionated natural organic matter on the phytoavailability of silver nanoparticles, Environ. Sci.: Nano, 2018, 5, 969–979 RSC.
- K. Sazawa, H. Yoshida, K. Okusu, N. Hata and H. Kuramitz, Effects of forest fire on the properties of soil and humic substances extracted from forest soil in Gunma, Japan, Environ. Sci. Pollut. Res., 2018, 25, 30325–30338 CrossRef CAS PubMed.
- J. Hedberg, E. Blomberg and I. O. Wallinder, In the Search for Nanospecific Effects of Dissolution of Metallic Nanoparticles at Freshwater-Like Conditions: A Critical Review, Environ. Sci. Technol., 2019, 53, 4030–4044 CrossRef CAS PubMed.
- A. Stojiljkovic, K. Kuehni-Boghenbor, V. Gaschen, G. Schupbach, M. Mevissen, C. Kinnear, A. M. Moller and M. H. Stoffel, High-content analysis of factors affecting gold nanoparticle uptake by neuronal and microglial cells in culture, Nanoscale, 2016, 8, 16650–16661 RSC.
- C. Wilhelm, C. Billotey, J. Roger, J. N. Pons, J. C. Bacri and F. Gazeau, Intracellular uptake of anionic superparamagnetic nanoparticles as a function of their surface coating, Biomaterials, 2003, 24, 1001–1011 CrossRef CAS PubMed.
- J. Saavedra, S. Stoll and V. I. Slaveykova, Influence of nanoplastic surface charge on eco-corona formation, aggregation and toxicity to freshwater zooplankton, Environ. Pollut., 2019, 252, 715–722 CrossRef CAS PubMed.
- J. Y. Wu, R. F. Jiang, W. Lin and G. F. Ouyang, Effect of salinity and humic acid on the aggregation and toxicity of polystyrene nanoplastics with different functional groups and charges, Environ. Pollut., 2019, 245, 836–843 CrossRef CAS PubMed.
- Y. L. Xiao, M. G. Vijver and W. J. G. M. Peijnenburg, Impact of water chemistry on the behavior and fate of copper nanoparticles, Environ. Pollut., 2018, 234, 684–691 CrossRef CAS PubMed.
- A. Thit, K. Huggins, H. Selck and A. Baun, Acute toxicity of copper oxide nanoparticles to Daphnia magna under different test conditions, Toxicol. Environ. Chem., 2017, 99, 665–679 CrossRef CAS.
- Y. Zhang, T. T. Meng, L. Shi, X. Guo, X. H. Si, R. X. Yang and X. Quan, The effects of humic acid on the toxicity of graphene oxide to Scenedesmus obliquus and Daphnia magna, Sci. Total Environ., 2019, 649, 163–171 CrossRef CAS PubMed.
- V. L. Castro, Z. Clemente, C. Jonsson, M. Silva, J. H. Vallim, A. M. Z. de Medeiros and D. S. T. Martinez, Nanoecotoxicity assessment of graphene oxide and its relationship with humic acid, Environ. Toxicol. Chem., 2018, 37, 1998–2012 CrossRef CAS PubMed.
- H. Lin, X. H. Xia, X. M. Jiang, S. Q. Bi, H. T. Wang, Y. W. Zhai, W. Wen and X. J. Guo, Bioavailability of Pyrene Associated with Different Types of Protein Compounds: Direct Evidence for Its Uptake by Daphnia magna, Environ. Sci. Technol., 2018, 52, 9851–9860 CrossRef CAS PubMed.
- N. Singh, E. Tiwari, N. Khandelwal and G. K. Darbha, Understanding the stability of nanoplastics in aqueous environments: effect of ionic strength, temperature, dissolved organic matter, clay, and heavy metals, Environ. Sci.: Nano, 2019, 6, 2968–2976 RSC.
- W. W. Yang, Y. Wang, B. Huang, N. X. Wang, Z. B. Wei, J. Luo, A. J. Miao and L. Y. Yang, TiO2 Nanoparticles Act As a Carrier of Cd Bioaccumulation in the Ciliate Tetrahymena
thermophila, Environ. Sci. Technol., 2014, 48, 7568–7575 CrossRef CAS PubMed.
- H. Joolaei, M. Vossoughi, A. R. M. Abadi and A. Heravi, Removal of humic acid from aqueous solution using photocatalytic reaction on perlite granules covered by Nano TiO2 particles, J. Mol. Liq., 2017, 242, 357–363 CrossRef CAS.
- B. Glomstad, D. Altin, L. Sorensen, J. F. Liu, B. M. Jenssen and A. M. Booth, Carbon Nanotube Properties Influence Adsorption of Phenanthrene and Subsequent Bioavailability and Toxicity to Pseudokirchneriella subcapitata, Environ. Sci. Technol., 2016, 50, 2660–2668 CrossRef CAS PubMed.
- M. Mortimer, K. Kasemets, M. Vodovnik, R. Marinsek-Logar and A. Kahru, Exposure to CuO Nanoparticles Changes the Fatty Acid Composition of Protozoa Tetrahymena thermophila, Environ. Sci. Technol., 2011, 45, 6617–6624 CrossRef CAS PubMed.
- K. Tanneberger, M. Knöbel, F. J. Busser, T. L. Sinnige, J. L. Hermens and K. Schirmer, Predicting fish acute toxicity using a fish gill cell line-based toxicity assay, Environ. Sci. Technol., 2013, 47, 1110–1119 CrossRef CAS PubMed.
- M. Mortimer, E. J. Petersen, B. A. Buchholz, E. Orias and P. A. Holden, Bioaccumulation of Multiwall Carbon Nanotubes in Tetrahymena thermophila by Direct Feeding or Trophic Transfer, Environ. Sci. Technol., 2016, 50, 8876–8885 CrossRef CAS PubMed.
- V. Raffa, G. Ciofani, S. Nitodas, T. Karachalios, D. D'Alessandro, M. Masini and A. Cuschieri, Can the properties of carbon nanotubes influence their internalization by living cells?, Carbon, 2008, 46, 1600–1610 CrossRef CAS.
- J. Chen, Z. Xiu, G. V. Lowry and P. J. Alvarez, Effect of natural organic matter on toxicity and reactivity of nano-scale zero-valent iron, Water Res., 2011, 45, 1995–2001 CrossRef CAS PubMed.
- L. Zhang, C. Lei, J. Chen, K. Yang, L. Zhu and D. Lin, Effect of natural and synthetic surface coatings on the toxicity of multiwalled carbon nanotubes toward green algae, Carbon, 2015, 83, 198–207 CrossRef CAS.
- J. E. Schnitzer, P. Oh, E. Pinney and J. Allard, Filipin-sensitive caveolae-mediated transport in endothelium: reduced transcytosis, scavenger endocytosis, and capillary permeability of select macromolecules, J. Cell Biol., 1994, 127, 1217–1232 CrossRef CAS PubMed.
- Y. Ha, X. Z. Wang, H. M. Liljestrand, J. A. Maynard and L. E. Katz, Bioavailability of Fullerene under Environmentally Relevant Conditions: Effects of Humic Acid and Fetal Bovine Serum on Accumulation in Lipid Bilayers and Cellular Uptake, Environ. Sci. Technol., 2016, 50, 6717–6727 CrossRef CAS PubMed.
- W. Jia and P. Y. Wu, Fast Proton Conduction in Denatured Bovine Serum Albumin-Coated Nafion Membranes, ACS Appl. Mater. Interfaces, 2018, 10, 39768–39776 CrossRef CAS PubMed.
- A. Zaragoza, J. A. Teruel, F. J. Aranda, A. Marques, M. J. Espuny, A. Manresa and A. Ortiz, Interaction of a Rhodococcus sp Trehalose Lipid Biosurfactant with Model Proteins: Thermodynamic and Structural Changes, Langmuir, 2012, 28, 1381–1390 CrossRef CAS PubMed.
- L. Wang, J. Li, J. Pan, X. Jiang, Y. Ji, Y. Li, Y. Qu, Y. Zhao, X. Wu and C. Chen, Revealing the binding structure of the protein corona on gold nanorods using synchrotron radiation-based techniques: understanding the reduced damage in cell membranes, J. Am. Chem. Soc., 2013, 135, 17359–17368 CrossRef CAS PubMed.
- V. Banerjee and K. P. Das, Structure and Functional Properties of a Multimeric Protein alpha A-Crystallin Adsorbed on Silver Nanoparticle Surface, Langmuir, 2014, 30, 4775–4783 CrossRef CAS PubMed.
- X. Wang, W. Fan, Z. Dong, D. Liang and T. Zhou, Interactions of natural organic matter on the surface of PVP-capped silver nanoparticle under different aqueous environment, Water Res., 2018, 138, 224–233 CrossRef CAS PubMed.
- G. Damaschun, H. Damaschun, K. Gast and D. Zirwer, Denatured states of yeast phosphoglycerate kinase, Biochemistry, 1998, 63, 259–275 CAS.
- J. W. Jiang and J. M. Prausnitz, Molecular thermodynamics for partitioning of native and denatured proteins in aqueous two-phase systems, J. Phys. Chem. B, 2000, 104, 7197–7205 CrossRef CAS.
- W. H. Fan, X. L. Wang, M. M. Cui, D. F. Zhang, Y. Zhang, T. Yu and L. Guo, Differential Oxidative Stress of Octahedral and Cubic Cu2O Micro/Nanocrystals to Daphnia magna, Environ. Sci. Technol., 2012, 46, 10255–10262 CrossRef CAS PubMed.
- Q. H. Wang, C. Li, Y. Wang and X. Que, Phytotoxicity of Graphene Family Nanomaterials and Its Mechanisms: A Review, Front. Chem., 2019, 7 CAS.
- S. T. Du, P. Zhang, R. R. Zhang, Q. Lu, L. Liu, X. W. Bao and H. J. Liu, Reduced graphene oxide induces cytotoxicity and inhibits photosynthetic performance of the green alga Scenedesmus obliquus, Chemosphere, 2016, 164, 499–507 CrossRef CAS PubMed.
- L. Verneuil, J. Silvestre, F. Mouchet, E. Flahaut, J. C. Boutonnet, F. Bourdiol, T. Bortolamiol, D. Baqué, L. Gauthier and E. Pinelli, Multi-walled carbon nanotubes, natural organic matter, and the benthic diatom Nitzschia palea: "A sticky story", Nanotoxicology, 2015, 9, 219–229 CrossRef CAS PubMed.
- J. Zhao, X. S. Cao, Z. Y. Wang, Y. H. Dai and B. S. Xing, Mechanistic understanding toward the toxicity of graphene-family materials to freshwater algae, Water Res., 2017, 111, 18–27 CrossRef CAS PubMed.
- S. Sabella, R. P. Carney, V. Brunetti, M. A. Malvindi, N. Al-Juffali, G. Vecchio, S. M. Janes, O. M. Bakr, R. Cingolani, F. Stellacci and P. P. Pompa, A general mechanism for intracellular toxicity of metal-containing nanoparticles, Nanoscale, 2014, 6, 7052–7061 RSC.
- F. You, W. Q. Tang and L. Y. L. Yung, Real-time monitoring of the Trojan-horse effect of silver nanoparticles by using a genetically encoded fluorescent cell sensor, Nanoscale, 2018, 10, 7726–7735 RSC.
- W. H. Wu, R. J. Zhang, D. J. McClements, B. Chefetz, T. Polubesova and B. S. Xing, Transformation and Speciation Analysis of Silver Nanoparticles of Dietary Supplement in Simulated Human Gastrointestinal Tract, Environ. Sci. Technol., 2018, 52, 8792–8800 CrossRef CAS PubMed.
- P. Kotchaplai, E. Khan and A. S. Vangnai, Membrane Alterations in Pseudomonas putida F1 Exposed to Nanoscale Zerovalent Iron: Effects of Short-Term and Repetitive nZVI Exposure, Environ. Sci. Technol., 2017, 51, 7804–7813 CrossRef CAS PubMed.
- J. Tournebize, A. Sapin-Minet, G. Bartosz, P. Leroy and A. Boudier, Pitfalls of assays devoted to evaluation of oxidative stress induced by inorganic nanoparticles, Talanta, 2013, 116, 753–763 CrossRef CAS PubMed.
- S. Dalai, S. Pakrashi, R. S. S. Kumar, N. Chandrasekaran and A. Mukherjee, A comparative cytotoxicity study of TiO2 nanoparticles under light and dark conditions at low exposure concentrations, Toxicol. Res., 2012, 1, 116–130 CrossRef CAS.
- D. G. Goodwin, K. M. Marsh, I. B. Sosa, J. B. Payne, J. M. Gorham, E. J. Bouwer and D. H. Fairbrother, Interactions of Microorganisms with Polymer Nanocomposite Surfaces Containing Oxidized Carbon Nanotubes, Environ. Sci. Technol., 2015, 49, 5484–5492 CrossRef CAS PubMed.
- L. Schlagenhauf, T. Buerki-Thurnherr, Y. Y. Kuo, A. Wichser, F. Nuesch, P. Wick and J. Wang, Carbon Nanotubes Released from an Epoxy-Based Nanocomposite: Quantification and Particle Toxicity, Environ. Sci. Technol., 2015, 49, 10616–10623 CrossRef CAS PubMed.
- Y. Liu, E. Yoo, G. J. Mahler and A. L. Doiron, Endothelial barrier dysfunction induced by nanoparticle
exposure through actin remodeling via caveolae/raft-regulated calcium signalling, NANO, 2018, 11, 82–91 Search PubMed.
- Z. H. Xia, A. Woods, A. Quirk, I. J. Burgess and B. L. T. Lau, Interactions between polystyrene nanoparticles and supported lipid bilayers: impact of charge and hydrophobicity modification by specific anions, Environ. Sci.: Nano, 2019, 6, 1829–1837 RSC.
- Y. Li, H. Y. Chen, F. Wang, F. R. Zhao, X. M. Han, H. H. Geng, L. Gao, H. L. Chen, R. F. Yuan and J. Yao, Environmental behavior and associated plant accumulation of silver nanoparticles in the presence of dissolved humic and fulvic acid, Environ. Pollut., 2018, 243, 1334–1342 CrossRef CAS PubMed.
- W. C. Zhang, J. L. Huang, L. Liang, L. H. Yao and T. Fang, Dual impact of dissolved organic matter on cytotoxicity of PVP-Ag NPs to Escherichia coli: Mitigation and intensification, Chemosphere, 2019, 214, 754–763 CrossRef CAS PubMed.
- K. Ouyang, X. Y. Yu, Y. L. Zhu, C. H. Gao, Q. Y. Huang and P. Cai, Effects of humic acid on the interactions between zinc oxide nanoparticles and bacterial biofilms, Environ. Pollut., 2017, 231, 1104–1111 CrossRef CAS PubMed.
- N. Ye, Z. Wang, S. Wang, H. Fang and D. G. Wang, Dissolved organic matter and aluminum oxide nanoparticles synergistically cause cellular responses in freshwater microalgae, J. Environ. Sci. Health, Part A: Toxic/Hazard. Subst. Environ. Eng., 2018, 53, 651–658 CrossRef CAS PubMed.
- C. H. Deng, J. L. Gong, G. M. Zeng, Y. Jiang, C. Zhang, H. Y. Liu and S. Y. Huan, Graphene-CdS nanocomposite inactivation performance toward Escherichia coli in the presence of humic acid under visible light irradiation, Chem. Eng. J., 2016, 284, 41–53 CrossRef CAS.
- Y. L. Xiao, W. J. G. M. Peijnenburg, G. C. Chen and M. G. Vijver, Impact of water chemistry on the particle-specific toxicity of copper nanoparticles to Daphnia magna, Sci. Total Environ., 2018, 610, 1329–1335 CrossRef PubMed.
Footnote |
† Electronic supplementary information (ESI) available: Four tables (Table S1–S4) and six figures (Figure S1–S6). See DOI: 10.1039/c9en01017c |
|
This journal is © The Royal Society of Chemistry 2020 |