Dual-targeted activatable photosensitizers with aggregation-induced emission (AIE) characteristics for image-guided photodynamic cancer cell ablation†
Received
31st October 2015
, Accepted 16th November 2015
First published on 16th November 2015
Abstract
The currently available photosensitizers (PSs) for photodynamic therapy (PDT) can easily lead to undesirable normal cell death due to their intrinsic photo-toxicity and lack of selectivity for cancer cells. Activatable PSs with high therapeutic efficiency towards cancer cells but minimized side effects on normal cells are thus highly desirable. In this work, we developed a probe with dual-targeted activatable PSs that can recognize and ablate cancer cells with high selectivity. The probe is composed of a fluorophore with aggregation-induced emission (AIE) characteristics which can be used as an imaging agent as well as a PS, a quencher moiety that can be cleaved upon encountering biothiols, and a cyclic arginine–glycine–aspartic acid (cRGD) tripeptide for targeting cancer cells with overexpressed αvβ3 integrin. The probe itself is non-fluorescent and its ability to generate reactive oxygen species (ROS) is prohibited. However, it could be selectively activated to offer specific fluorescence turn-on with efficient ROS generation in the aggregated state, which was used to ablate cancer cells overexpressing both αvβ3 integrin receptors and glutathione. As compared to conventional activatable PSs which show quenched fluorescence and reduced ROS generation in the aggregated state, the dual-selection process with enhanced fluorescence and efficient ROS generation of the activated AIE probe in aggregated state offers a high signal-to-background ratio for MDA-MB-231 cancer cell imaging and ablation. This strategy thus opens up new opportunities for designing activatable PSs with high selectivity and low intrinsic photo-toxicity for photodynamic cancer cell ablation.
Introduction
Photodynamic therapy (PDT) is based on the concept that upon light irradiation, photosensitizers (PSs) can generate cytotoxic reactive oxygen species (ROS), mainly singlet oxygen (1O2), to induce cell death.1 PDT has been recognized as one of the most promising and noninvasive cancer treatment modalities. However, the clinical application of PDT is still restricted due to its intrinsic photo-toxicity and lack of selectivity which can cause severe side-effects on normal tissues.2 Therefore, it is necessary to explore new strategies to enhance the generation efficiency of ROS and cancer cell selectivity of PSs to improve the therapeutic outcome as well as to minimize the side effects.
Recently, activatable PSs that can only be activated in tumor tissue through tumor-associated stimuli have received great attention.3 In these systems, the fluorescence and photosensitizing properties of PSs are quenched by photoinduced electron transfer (PeT), self-quenching or fluorescence resonance energy transfer (FRET) mechanisms.4–15 Upon encountering tumor related enzymes or environments, the separation of quenchers or electron/energy acceptors from the PSs can restore their fluorescence and photosensitizing properties. However, in most cases, the changes in fluorescence and photosensitization activity of PSs before and after activation were less than ten-fold. This is because the background signal is highly dependent on the FRET or quenching efficiency and the activated PSs often show notorious aggregation caused quenching (ACQ) properties,16 leading to fluorescence self-quenching with a low signal output and reduced photoactivity. As the removal of quencher moieties is mainly accomplished by tumor associated enzymes (e.g. matrix metalloproteinase,6,17 β-galactosidase,13etc.) or pH difference,9 these activatable PSs can improve the cancer cell selectivity to a certain extent but cross-activation in normal cells frequently occurs.18 In addition, pH-activatable PSs rely on the protonation of nitrogen atoms at acidic pH in lysosomes to restrict the quenching process,9 however, the low pH in lysosome is a general characteristic of the specific organelle, it is not directly related to tumor cells. There is thus a need to develop novel activatable PSs with high selectivity and a high target-to-background ratio for cancer therapy.
Recently, fluorogens with aggregation-induced emission characteristics (AIEgens) have received great attention in biomedical applications.19–30 Distinct to the conventional fluorophores and PSs that show ACQ properties in aggregates, AIEgens emit intensively in aggregated states due to the restriction of intramolecular motions.31 Their unique properties have allowed the design of light-up probes for sensing and fluorescent nanoparticles to continuously monitor biological processes.17,19 In addition, we and others have developed AIEgen PSs that can efficiently generate ROS even in the aggregated state.32–34 The AIEgen PS nanoparticles (NPs) show high brightness with intrinsic photo-toxicity, which can easily cause side effects on normal cells. To reduce the side effects, tumor-specific ligands have been conjugated to AIEgen PS NPs.32 Although this strategy can improve the selectivity, the results are not very satisfactory as the receptors could exist on both the tumor and normal cells, although they may be overexpressed on the former.
To overcome the limitations of single-targeting, dual-targeting strategies have been developed to improve the target-to-background ratio for cancer therapy.35,36 These systems often contain a tumor targeting ligand and a tumor specific prodrug activator such as enzymes or pH. In addition, glutathione (GSH) has been widely utilized for selective release and activation of drugs for cancer therapy.37,38 The concentration of GSH in the intracellular region is much higher than that in the extracellular region and cancer cells are known to have much higher concentrations of GSH as compared to normal cells.39,40 The difference provides the possibility to develop GSH activatable PSs that are stable in an extracellular environment but can be selectively activated in cancer cells, which has been achieved by using 2,4-dinitrobenzenesulfonyl as a quencher moiety.11 However, previous studies based on porphyrin PSs have the disadvantage that porphyrin derivatives are prone to aggregate in aqueous media to yield quenched fluorescence and reduced ROS generation as compared to their molecular state.14 By incorporating a target ligand (1st target) and a cancer cell specific activator (2nd target) to a therapeutic agent, the dual selection process will offer a new generation of AIEgen PSs to achieve selective ablation of cancer cells with high specificity and efficient ROS generation in the aggregate state.
In this contribution, we designed a dual-targeted and activatable AIEgen PS probe for selective recognition, imaging and killing of cancer cells (Scheme 1). The fluorescence and photosensitizing activity of the probe were initially quenched by the thiol cleavable quencher moiety 2,4-dinitrobenzenesulfonyl41 but can be restored after receptor (e.g. αvβ3 integrin) mediated endocytosis (1st target), followed by intracellular GSH activation (2nd target). By taking advantage of the AIEgen PS that shows efficient ROS generation in the aggregated state and the dual-process of recognition and activation, the PS can be delivered and activated in a target cancer cell with high selectivity, which has been successfully used for cancer cell ablation.
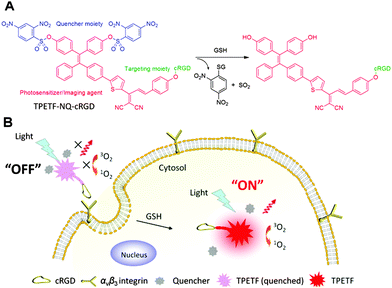 |
| Scheme 1 (A) Schematic illustration of probe TPETF-NQ-cRGD activated by glutathione (GSH) to release the quencher moiety. (B) The probe maintains an “off” state which is non-fluorescent with almost no ROS generation upon light irradiation, but the fluorescence and ROS generation can be turned “on” after receptor mediated endocytosis, followed by intracellular GSH activation. | |
Results and discussion
Design principle of the AIEgen PS
Tetraphenylethylene (TPE) is one of the most commonly used AIEgens but its absorption is in the UV range and it is a poor photosensitizer.31 By introducing the dicyanovinyl group as an electron acceptor and the methoxy group as an electron donor into a TPE core to form a D–π–A structure, it is expected to yield an AIEgen with red shifted absorption/emission maxima and reduced ΔES1–T1 (the energy gap between the lowest singlet excited state (S1) and the lowest triplet excited state (T1)). By reducing ΔES1–T1, one would expect to improve intersystem crossing, which is favorable for ROS generation.42 Upon excitation of the AIEgen PS with white light, the S1 state will switch to the T1 state by intersystem crossing (ISC), which is followed by energy transfer from the T1 state of the PS to surrounding oxygen (3O2) to generate singlet oxygen (1O2). By attaching a quencher moiety to the AIEgen PS, the fluorescence and photosensitizing activity can be quenched in the aggregated state. Once the quencher moiety is released from the AIEgen PS, the AIE characteristic will lead to high brightness and efficient ROS generation even in the aggregated state, which is highly desirable for image-guided PDT.
Synthesis of the probe TPETF-NQ-cRGD
The synthetic route towards TPETF and TPETF-NQ-cRGD is shown in Scheme 2. Compound 1 was obtained by the McMurry reaction between 4-bromobenzophenone and 4,4′-dimethoxybenzophenone in the presence of TiCl4 and Zn.43 Subsequently, compound 1 was reacted with trimethyl borate to yield compound 2. The Suzuki reaction between compound 2 and 2-acetyl-5-bromothiophene yielded compound 3, which was further reacted with malononitrile on the SiO2 support to produce TPETF. Further treatment of TPETF with 4-(3-azidopropoxy)benzaldehyde in the presence of peperidine, followed by de-protection of methoxy groups with boron tribromide (BBr3) in dichloromethane (DCM), yielded azide-functionalized TPETF (TPETF-N3). The hydroxyl groups in TPETF-N3 were then reacted with the quencher moiety of 2,4-dinitrobenzenesulfonyl chloride (NQ) in the presence of N,N-diisopropylethylamine (DIPEA) to yield TPETF-NQ. TPETF-NQ-cRGD was obtained as red powders via the copper(II) sulfate and sodium ascorbate (NaAsc) catalyzed click reaction between TPETF-NQ and alkyne-functionalized cRGD, followed by HPLC purification and freeze drying. It is known that NQ is a strong electron acceptor group which can produce a dark excited state through the intramolecular photoinduced electron transfer (PeT) process, which can be removed upon encountering thiols.41 The probe and the intermediates were characterized by NMR and HRMS to confirm their right structures (Fig. S1–S8, ESI†).
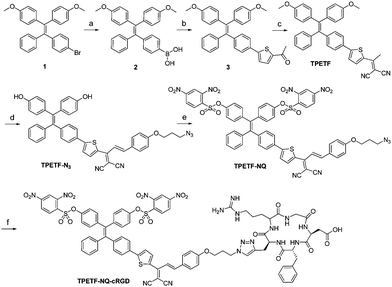 |
| Scheme 2 Synthetic route toward the probe of TPETF-NQ-cRGD. Reagents: (a) n-butyllithium, trimethyl borate, hydrochloric acid; (b) tetrakis(triphenylphosphine)palladium(0), K2CO3, 4-aceyle-5-bromothiophene; (c) malononitrile, ammonium acetate, silica gel; (d) 4-(3-azidopropoxy)benzaldehyde, piperidine, 2-propanol; then boron tribromide; (e) 2,4-dinitrobenzenesulfonyl chloride, triethylamine; (f) alkyne-functionalized cRGD, sodium ascorbate, copper(II) sulphate. | |
Photoproperties of the probe
The AIE properties of TPETF-N3 were first studied by monitoring its fluorescence intensity in water and DMSO mixtures with different water fractions (fw). As shown in Fig. 1A, TPETF-N3 is almost non-emissive in DMSO solution (fw = 0), which should be due to the free rotation of the TPE phenyl rings in the molecularly dissolved state. However, the fluorescence intensity increases steadily with the increase of fw. At fw = 99%, the fluorescence intensity of TPETF-N3 is 110-fold higher than that in DMSO. This should be due to the fact that hydrophobic TPETF-N3 tends to aggregate at a higher water fraction, which leads to the restriction of the intramolecular motion to active the AIE process. The aggregation formation of TPETF-N3 at fw = 99% was confirmed by laser light scattering (LLS) studies which show an average size of 105 ± 13 nm. The UV-vis absorption spectra of TPETF-N3, TPETF-NQ and TPETF-NQ-cRGD are shown in Fig. 1B. Compared with TPETF-N3 which shows an absorption maximum of 430 nm, the conjugation of a quencher moiety (TPETF-NQ) leads to a blue-shift of the absorption spectrum as the NQ moiety weakens charge transfer between the D and A in the original D–π–A structure. Further conjugation of the cRGD moiety does not change its absorption spectrum. The emission spectra of TPETF-N3, TPETF-NQ and TPETF-NQ-cRGD in DMSO/PBS (v/v = 1/199) are very different. As shown in Fig. 1C, TPETF-N3 shows strong red fluorescence, while both TPETF-NQ and TPETF-NQ-cRGD are almost non-fluorescent in the same medium due to the PeT process between TPETF and the quencher moiety. The fluorescence intensity of TPETF-N3 at 650 nm is 280 and 320-fold higher than that of TPETF-NQ and TPETF-NQ-cRGD, respectively. These results clearly demonstrate that the NQ moiety is a very effective fluorescence quencher for TPETF. To study the responsiveness of TPETF-NQ-cRGD toward biothiols, the fluorescence change of TPETF-NQ-cRGD (10 μM) towards GSH (0.1 mM) in DMSO/PBS (v/v = 1/199) was investigated. As shown in Fig. 1D, the fluorescence intensity of TPETF-NQ-cRGD increases gradually upon addition of GSH and a plateau is reached after 2 h incubation. The fluorescence intensity at 650 nm is enhanced by 115-fold as compared to its original value. These should be due to the separation of the NQ quencher moiety from the probe as a result of cleavage of the sulfonate ester by GSH. Under optimized conditions, different concentrations of TPETF-NQ-cRGD were incubated with GSH (0.1 mM) for 90 min and the corresponding emission spectra were investigated. As shown in Fig. 1E, the fluorescence intensity of GSH treated TPETF-NQ-cRGD increases with respect to the probe concentrations. The plot of the PL intensities at 650 nm against the probe concentrations gives a linear fit (R2 = 0.982), indicating the possibility to semi-quantify the activated PS through PL intensity changes (Fig. 1F).
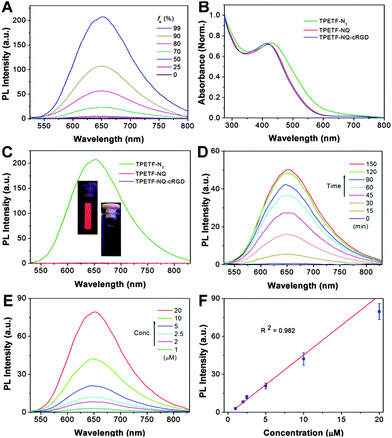 |
| Fig. 1 (A) Photoluminescence (PL) spectra of TPETF-N3 in DMSO and water mixtures at different water fractions (fw). (B) Normalized UV-vis absorption of TPETF-N3, TPETF-NQ and TPETF-NQ-cRGD in DMSO/PBS (v/v = 1/199). (C) PL spectra of TPETF-N3, TPETF-NQ and TPETF-NQ-cRGD in DMSO/PBS (v/v = 1/199). Inset: The corresponding photographs taken under irradiation with a hand-held 365 nm lamp. (D) Time-dependent PL spectra of the probe (10 μM) upon incubation with GSH. (E) PL spectra of the probe at different concentrations after incubation with GSH (0.1 mM) for 90 min. (F) Plot of PL intensities at 650 nm versus the probe concentrations upon incubation with GSH. The excitation wavelength was 430 nm. Data represent mean values ± standard deviation, n = 3. | |
Rationalization of the probe with GSH-induced fluorescence turn-on
To evaluate the reaction mechanism of the probe with GSH, we incubated the probe (10 μM) with GSH (0.1 mM) for 24 h and the reaction mixture was monitored by HPLC at an absorbance of 430 nm. As shown in Fig. S9 (ESI†), the probe shows a single peak with a retention time of 14.9 min, which corresponds to a mass-to-charge ratio (m/z) of 1754.426. After treatment with GSH, a new peak at 8.9 min is observed. The decreased retention time should be due to the release of the hydrophobic quencher from the probe, yielding a relatively more polar AIE residue as compared to the probe itself. This is further confirmed by the mass spectrum which shows an m/z of 1294.106 for the peak at 8.9 min, confirming that the quencher moieties were removed after the treatment with GSH. These results clearly demonstrate that the probe “turn-on” is due to the removal of the quencher moieties through cleavage of the sulfonate ester by GSH.
The aggregate formation of TPETF-NQ-cRGD in aqueous media before and after treatment with GSH was studied by LLS measurements and TEM imaging. As shown in Fig. 2, TPETF-NQ-cRGD shows an average size of 135 ± 17 nm. The morphology of the probe aggregates was also confirmed by the TEM image, which shows spherical nanoparticles with an average size of about 100 nm. After GSH treatment, the size was slightly increased, due to the loose aggregate formation as the AIE residue is more hydrophilic relative to the probe itself.
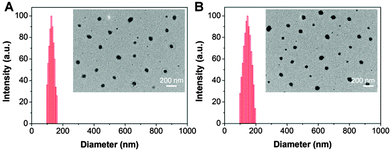 |
| Fig. 2 Size distribution and TEM images (inset) of TPETF-NQ-cRGD before (A) and after (B) treatment with GSH (0.1 mM). | |
Selectivity of the probe
To study the selectivity, the probe was incubated with some cellular relevant biological analytes under identical conditions and the fluorescence intensity change at 650 nm was monitored. As shown in Fig. 3A, the probe shows good selectivity to biothiols over other biologically related analytes. In addition, the effects of pH on the emission of the probe and the GSH treated probe were also investigated. As shown in Fig. 3B, the probe fluorescence remains low in the physiological pH range and the bright fluorescence of the GSH treated probe remains constant. The pH-independent emission indicates that the probe can work in biological systems with limited pH interference.
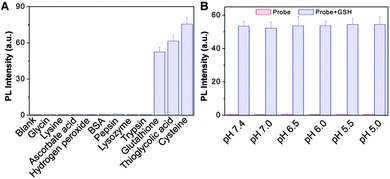 |
| Fig. 3 Fluorescence response of the probe (10 μM) toward different analytes (A) or variation of pH (B). | |
To confirm that GSH plays a critical role in probe activation, we obtained the cell lysate from MDA-MB-231 cancer cells or MDA-MB-231 cells pretreated with buthionine sulfoximine (BSO) to inhibit the cells from synthesizing GSH.44 As shown in Fig. 4, when the lysates were directly incubated with the probe, the PL intensity at 650 nm increased steadily with the incubation time. However, when the cells were pretreated with BSO, the fluorescence increase is greatly inhibited as compared to that in cells without any treatment. These results indicate that although there are different kinds of biothiols in cells, GSH is critical for the PS activation as it is the most abundant biothiols in cells.45
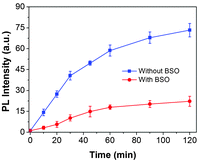 |
| Fig. 4 Time-dependent PL intensity change of the probe at 650 nm upon incubation with cell lysates from MDA-MB-231 cells or MDA-MB-231 cells pretreated with BSO. | |
ROS generation
The ROS generation of PSs upon light irradiation is a critical step for efficient photodynamic therapy. The ROS quantum yield (Φ) of TPETF-N3 in DMSO/PBS (v/v = 1/199) was determined to be 0.33 using Rose Bengal (RB) as a standard photosensitizer (ΦRB = 0.75 in water), which is comparable to the clinically used photosensitizers such as Photofrin® (Φ = 0.28) or Laserphyrin® (Φ = 0.48). The ROS generation of the probe upon white light irradiation was measured and compared with the GSH-pretreated probe using ABDA as a ROS indicator. As shown in Fig. 5A, under white light irradiation, the probe itself shows almost no ROS generation. However, the GSH activated PS is very effective in ROS generation. When vitamin C (a well known ROS scavenger) was added, the greatly decreased ABDA absorbance change further confirmed the ROS generation. The ROS generation is also power density dependent (Fig. 5B), which is revealed by the increased ABDA absorbance drop at a higher light power. The significant difference in ROS generation for the probe before and after GSH treatment demonstrates that NQ is an effective quencher and the quenching process can be recovered upon reaction with biothiols. Moreover, the absorbance of the probe does not change obviously after white light irradiation for 8 min at a power density of 0.25 W cm−2, indicating its good photostability.
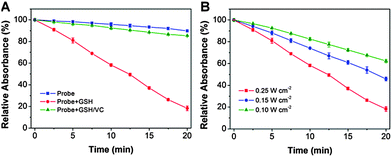 |
| Fig. 5 (A) Time-dependent absorbance changes of ROS indicator ABDA at 400 nm mixed with the probe, GSH-pretreated probe in the presence and absence of ROS-scavenge vitamin C (VC) at a power density of 0.25 W cm−2. (B) ROS generation of the GSH-pretreated probe (10 μM) at different light power densities. The relative absorbance is defined as the absorbance of ABDA at 400 nm after different times of light irradiation as compared to its original value. | |
Cancer cell imaging
To confirm the cell specific imaging, the probe was incubated with αvβ3 integrin overexpressed U87-MG or MDA-MB-231 cancer cells and low αvβ3 integrin expressed MCF-7 cancer cells as well as NIH 3T3 and 293T normal cells as the negative controls. It has been reported that cancer cells show high concentrations of GSH while the GSH concentrations are low in normal cells.11,46 It was anticipated that the probe could be accumulated more in cancer cells that overexpress αvβ3 integrin through receptor mediated endocytosis and subsequently undergo GSH induced release of the quencher moieties, leading to fluorescence “turn-on” in cancer cells. As shown in Fig. 6A–D, the red fluorescent signal attributed to the activated PS in MDA-MB-231 cells increases gradually with incubation time. In addition, the red fluorescent signal in MDA-MB-231 cells is much stronger than that in cells which underwent different pretreatments such as with cRGD to block the αvβ3 integrin or with BSO to inhibit the cells from synthesizing GSH (Fig. 6F and G). The strong red fluorescent signal is also observed in U87-MG cells (Fig. 6E), but it is much weaker in MCF-7 cells (Fig. 6H) and almost no signal is observed in 293T cells (Fig. 6I) and NIH 3T3 cells (Fig. 6J). In addition, the effects of incubation time and different treatments on fluorescence light-up of the probe in MDA-MB-231 cells were further studied by flow cytometry, which agree well with the confocal images (Fig. 7). These results clearly demonstrate that the dual process of receptor mediated endocytosis and the intracellular GSH activation is responsible for PS activation in the cells.
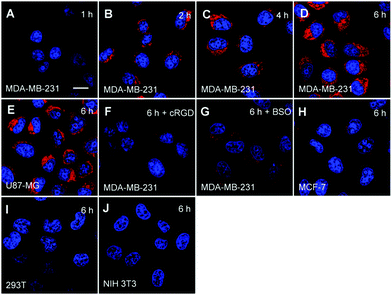 |
| Fig. 6 CLSM images of MDA-MB-231 (A–D, F and G), U87-MG (E), MCF-7 (H), 293T (I), NIH 3T3 (J) and MDA-MB-231 cells pre-incubated with cRGD (F) or BSO (G) and further incubated with the probe (10 μM) for 1 h (A), 2 h (B), 4 h (C), 6 h (D–J). The blue fluorescence is from Hoechst (ex: 405 nm; em: 430–470 nm); the red fluorescence is from AIEgen (ex: 453 nm; em: >560 nm). The scale bar is 20 μm. | |
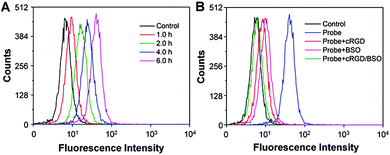 |
| Fig. 7 Flow cytometric analyses of the probe (10 μM) incubated MDA-MB-231 cells for different time duration (A) or pretreated with cRGD/BSO (B). | |
Intracellular ROS generation
The white light induced ROS generation was studied using DCF-DA as a cell permeable fluorescent indicator. DCF-DA is weakly fluorescent but can be reacted with ROS to yield highly fluorescent dichlorofluorescein (DCF). As shown in Fig. 8A, strong DCF fluorescence can be observed in MDA-MB-231 cells when they were incubated with the probe for 6 h and followed by white light irradiation. However, the green fluorescence in MCF-7 and 293T is negligible, indicating little or no ROS generation. When the probe was incubated with MDA-MB-231 cells that pretreated with cRGD or BSO, low green fluorescence was observed which agreed with the confocal studies. In addition, a reduced green fluorescent signal in MDA-MB-231 cells was observed when VC is presented, confirming that the green fluorescence enhancement is due to ROS generation.
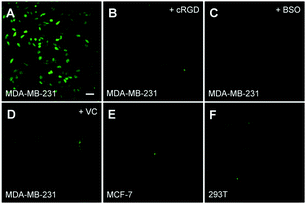 |
| Fig. 8 Intracellular ROS detection using DCF-DA as an indicator in MDA-MB-231 (A–D), MCF-7 (E), 293T (F) or MDA-MB-231 cells pretreated with (B) cRGD, (C) BSO or (D) VC after incubation of the probe (10 μM) for 6 h, followed by white light irradiation. The green fluorescence is attributed to DCF (ex: 488 nm; em: 505–525 nm). The scale bar is 50 μm. | |
Cell death imaging
The probe induced cell death upon light irradiation was monitored by using FITC-tagged Annexin V and propidium iodide (PI) as the indicators.9 Fluorescent dye-tagged Annexin V is commonly used to distinguish apoptotic cells from viable ones as Annexin V can selectively bind to exposed phosphatidylserines on the outer cytoplasmic membrane of apoptotic cells, while PI can selectively stain necrotic cells due to the increased permeability of cell membranes.9 After incubating with the probe for 6 h, the cells in a fresh medium were irradiated with white light for 3 min at a power density of 0.25 W cm−2 and stained with FITC-tagged Annexin V and PI. As shown in Fig. 9A, strong fluorescence of FITC and PI can be observed in MDA-MB-231 cells, demonstrating that the cells are progressed via both apoptosis and the necrotic cell death pathway after light irradiation which agrees with the previous reports.9 However, no significant fluorescence can be observed in MCF-7 or 293T cells, revealing low or no cell death. In addition, the fluorescence is low in cRGD or BSO pretreated MDA-MB-231 cells or in the presence of vitamin C (VC) during light irradiation, which are in accordance with the ROS generation studies. These results indicate that ROS plays an important role in inducing the cell death.
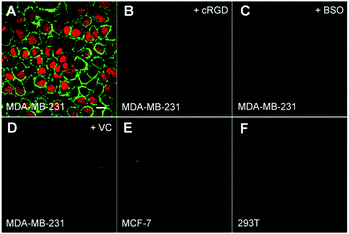 |
| Fig. 9 Confocal images of cell death induced by ROS using FITC-tagged Annexin V and propidium iodide (PI) as indicators. The probe (10 μM) was incubated with MDA-MB-231 (A–D), MCF-7 (E) and 293T (F) cells for 6 h, followed by light irradiation for 3 min at a power density of 0.25 W cm−2 and further incubation in fresh medium for 2 h before staining with Annexin V and PI. For B, C and D, the cells were pretreated with cRGD (B), BSO (C) or in the presence of vitamin C (D). The green fluorescence is attributed to FITC (ex: 488 nm; em: 505–525 nm); the red fluorescence is attributed to PI (ex: 543 nm, em: 610–640 nm). All the images share the same scale bar of 20 μm. | |
Cytotoxicity studies
Low cytotoxicity in dark but high toxicity upon light exposure is essential for phototherapy. The cytotoxicity of MDA-MB-231, MCF-7 and 293T cells incubated with the probe in dark or with light irradiation was evaluated by MTT assay. As shown in Fig. 10A, no significant cytotoxicity is observed for all the three cell lines when the probe concentration is up to 32 μM. However, a dose-dependent cytotoxicity is observed in probe incubated MDA-MB-231 cells after light exposure (Fig. 10B). The probe shows high photocytotoxicity with a half-maximal inhibitory concentration (IC50) value, defined as the drug concentration required to kill 50% of the cells, of 10.3 μM to MDA-MB-231 cells. Only minimum toxicity is observed for MCF-7 cells under the identical experimental conditions. The probe also shows no apparent photocytotoxicity to 293T cells. When the MDA-MB-231 cells were pretreated with cRGD, BSO or in the presence of vitamin C (Fig. 10C), the probe cytotoxicity to the cells is inhibited. In addition, we also confirm that the cytotoxicity of the probe to MDA-MB-231 cells is irradiation time dependent (Fig. 10D), indicating that the phototherapy can be regulated by laser irradiation time.
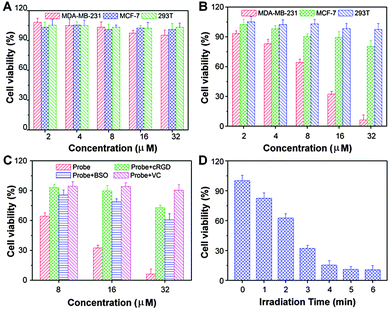 |
| Fig. 10 Cytotoxicity of different cells upon incubation with the probe (A) under dark conditions or (B) under light irradiation (3 min, 0.25 W cm−2). The cells without probe treatment were used as control and normalized to be 100%. (C) Cytotoxicity of the probe to MDA-MB-231 cells upon pretreatment with cRGD, BSO or VC, followed by light irradiation (3 min, 0.25 W cm−2). (D) Cytotoxicity of the probe to MDA-MB-231 cells with different time durations of light irradiation. | |
Conclusions
In conclusion, we have designed a dual-targeted and GSH-activatable probe based on a newly developed AIEgen PS for image-guided photodynamic cancer therapy with high selectivity. Before activation, the probe shows negligible fluorescence and ROS generation ability. Once it is selectively uptaken by cancer cells through receptor mediated endocytosis and further activated by GSH, the fluorescence is turned-on and the photosensitizer activity is restored. In addition, the efficient ROS generation of the AIEgen PS in the aggregated state and the dual targeting process lead to a highly selective image and ablation of αvβ3 integrin overexpressed MDA-MB-231 cancer cells with minimum side effects to normal cells using 293T and NIH 3T3 cells as examples. As compared to the existing systems reported before, our activatable AIEgen PS and the dual-selection process offer a high target-to-background ratio. Although the β-galactosidase activatable PS also shows a high target-to-background ratio and enhanced fluorescence together with efficient ROS generation upon aggregation,13 the system is limited to β-galactosidase expressing cells, which may not be generally applicable to other types of tumors. We believe that this AIEgen PS based GSH activated probe can serve as a promising highly cancer cell selective fluorescent probe and a photosensitizer for cancer therapy. Based on the proof-of-concept probe design in this work, further development of new AIEgens with longer absorption wavelengths as well as simple and more specific cancer cell targeting elements will yield more practical theranostic probes for in vivo applications.
Acknowledgements
We gratefully acknowledge the financial support from SMART (R279-000-378-592), the Ministry of Education (R279-000-391-112), Singapore, NRF Investigatorship (R-279-000-444-281, R-279-000-444-282) and the Institute of Materials Research and Engineering of Singapore (IMRE/14-8P1110).
Notes and references
- L. Cheng, C. Wang, L. Z. Feng, K. Yang and Z. Liu, Chem. Rev., 2014, 114, 10869–10939 CrossRef CAS PubMed.
- P. Agostinis, K. Berg, K. A. Cengel, T. H. Foster, A. W. Girotti, S. O. Gollnick, S. M. Hahn, M. R. Hamblin, A. Juzeniene, D. Kessel, M. Korbelik, J. Moan, P. Mroz, D. Nowis, J. Piette, B. C. Wilson and J. Golab, Ca-Cancer J. Clin., 2011, 61, 250–281 CrossRef PubMed.
- J. F. Lovell, T. W. B. Liu, J. Chen and G. Zheng, Chem. Rev., 2010, 110, 2839–2857 CrossRef CAS PubMed.
- E. Clo, J. W. Snyder, N. V. Voigt, P. R. Ogilby and K. V. Gothelf, J. Am. Chem. Soc., 2006, 128, 4200–4201 CrossRef CAS PubMed.
- Y. Choi, R. Weissleder and C. H. Tung, Cancer Res., 2006, 66, 7225–7229 CrossRef CAS PubMed.
- G. Zheng, J. Chen, K. Stefflova, M. Jarvi, H. Li and B. C. Wilson, Proc. Natl. Acad. Sci. U. S. A., 2007, 104, 8989–8994 CrossRef CAS PubMed.
- Y. Zhang, J. Qian, D. Wang, Y. Wang and S. He, Angew. Chem., Int. Ed., 2013, 52, 1148–1151 CrossRef CAS PubMed.
- Q. Yuan, Y. Wu, J. Wang, D. Q. Lu, Z. L. Zhao, T. Liu, X. B. Zhang and W. H. Tan, Angew. Chem., Int. Ed., 2013, 52, 13965–13969 CrossRef CAS PubMed.
- J. W. Tian, L. Ding, H. J. Xu, Z. Shen, H. X. Ju, L. Jia, L. Bao and J. S. Yu, J. Am. Chem. Soc., 2013, 135, 18850–18858 CrossRef CAS PubMed.
- Y. Cho, H. Kim and Y. Choi, Chem. Commun., 2013, 49, 1202–1204 RSC.
- I. S. Turan, F. P. Cakmak, D. C. Yildirim, R. Cetin-Atalay and E. U. Akkaya, Chem. – Eur. J., 2014, 20, 16088–16092 CrossRef CAS PubMed.
- K. Lou and J. F. Lovell, Chem. Commun., 2014, 50, 3231–3233 RSC.
- Y. Ichikawa, M. Kamiya, F. Obata, M. Miura, T. Terai, T. Komatsu, T. Ueno, K. Hanaoka, T. Nagano and Y. Urano, Angew. Chem., Int. Ed., 2014, 53, 6772–6775 CrossRef CAS PubMed.
- S. Park, H. Baik, Y. Oh, K. Oh, Y. Youn and E. Lee, Angew. Chem., Int. Ed., 2011, 50, 1644–1647 CrossRef CAS PubMed.
- S. Kolemen, M. Isik, G. M. Kim, D. Kim, H. Geng, M. Buyuktemiz, T. Karatas, X. F. Zhang, Y. Dede, J. Yoon and E. U. Akkaya, Angew. Chem., Int. Ed., 2015, 54, 5340–5344 CrossRef CAS PubMed.
-
J. B. Birks, Photophysics of aromatic molecules, Wiley, London, 1970 Search PubMed.
- B. Jang and Y. Choi, Theranostics, 2012, 2, 190–197 CrossRef CAS PubMed.
- D. Balinsky, C. E. Platz and J. W. Lewis, J. Natl. Cancer Inst., 1984, 72, 217–224 CAS.
- R. T. Kwok, C. W. Leung, J. W. Lam and B. Z. Tang, Chem. Soc. Rev., 2015, 44, 4228–4238 RSC.
- X. Zhang, X. Zhang, L. Tao, Z. Chi, J. Xu and Y. Wei, J. Mater. Chem. B, 2014, 2, 4398–4414 RSC.
- D. Ding, K. Li, B. Liu and B. Z. Tang, Acc. Chem. Res., 2013, 46, 2441–2453 CrossRef CAS PubMed.
- K. Li and B. Liu, Chem. Soc. Rev., 2014, 43, 6570–6597 RSC.
- Y. Y. Yuan, R. T. K. Kwok, B. Z. Tang and B. Liu, J. Am. Chem. Soc., 2014, 136, 2546–2554 CrossRef CAS PubMed.
- Q. L. Hu, M. Gao, G. X. Feng and B. Liu, Angew. Chem., Int. Ed., 2014, 53, 14225–14229 CrossRef CAS PubMed.
- X. D. Xue, Y. Y. Zhao, L. R. Dai, X. Zhang, X. H. Hao, C. Q. Zhang, S. D. Huo, J. Liu, C. Liu, A. Kumar, W. Q. Chen, G. Z. Zou and X. J. Liang, Adv. Mater., 2014, 26, 712–717 CrossRef CAS PubMed.
- X. R. Wang, J. M. Hu, G. Y. Zhang and S. Y. Liu, J. Am. Chem. Soc., 2014, 136, 9890–9893 CrossRef CAS PubMed.
- Y. Yuan, C. J. Zhang and B. Liu, Angew. Chem., Int. Ed., 2015, 54, 11419–11423 CrossRef CAS PubMed.
- A. Shao, Z. Guo, S. Zhu, S. Zhu, P. Shi, H. Tian and W. Zhu, Chem. Sci., 2014, 5, 1383–1389 RSC.
- A. D. Shao, Y. S. Xie, S. J. Zhu, Z. Q. Guo, S. Q. Zhu, J. Guo, P. Shi, T. D. James, H. Tian and W. H. Zhu, Angew. Chem., Int. Ed., 2015, 54, 7275–7280 CrossRef CAS PubMed.
- Z. L. Xie, C. J. Chen, S. D. Xu, J. Li, Y. Zhang, S. W. Liu, J. R. Xu and Z. G. Chi, Angew. Chem., Int. Ed., 2015, 54, 7181–7184 CrossRef CAS PubMed.
- J. Mei, Y. N. Hong, J. W. Y. Lam, A. J. Qin, Y. H. Tang and B. Z. Tang, Adv. Mater., 2014, 26, 5429–5479 CrossRef CAS PubMed.
- Y. Y. Yuan, G. X. Feng, W. Qin, B. Z. Tang and B. Liu, Chem. Commun., 2014, 50, 8757–8760 RSC.
- F. Hu, Y. Y. Huang, G. X. Zhang, R. Zhao, H. Yang and D. Q. Zhang, Anal. Chem., 2014, 86, 7987–7995 CrossRef CAS PubMed.
- E. G. Zhao, H. Q. Deng, S. J. Chen, Y. N. Hong, C. W. T. Leung, J. W. Y. Lam and B. Z. Tang, Chem. Commun., 2014, 50, 14451–14454 RSC.
- X. Tian, K. H. Baek and I. Shin, Chem. Sci., 2013, 4, 947–956 RSC.
- J. Z. Du, X. J. Du, C. Q. Mao and J. Wang, J. Am. Chem. Soc., 2011, 133, 17560–17563 CrossRef CAS PubMed.
- F. H. Meng, W. E. Hennink and Z. Zhong, Biomaterials, 2009, 30, 2180–2198 CrossRef CAS PubMed.
- M. H. Lee, Z. Yang, C. W. Lim, Y. H. Lee, S. Dongbang, C. Kang and J. S. Kim, Chem. Rev., 2013, 113, 5071–5109 CrossRef CAS PubMed.
- P. Kuppusamy, H. Q. Li, G. Ilangovan, A. J. Cardounel, J. L. Zweier, K. Yamada, M. C. Krishna and J. B. Mitchell, Cancer Res., 2002, 62, 307–312 CAS.
- R. A. Cairns, I. S. Harris and T. W. Mak, Nat. Rev. Cancer, 2011, 11, 85–95 CrossRef CAS PubMed.
- H. Maeda, H. Matsuno, M. Ushida, K. Katayama, K. Saeki and N. Itoh, Angew. Chem., Int. Ed., 2005, 44, 2922–2925 CrossRef CAS PubMed.
- S. Y. Lee, T. Yasuda, Y. S. Yang, Q. S. Zhang and C. Adachi, Angew. Chem., Int. Ed., 2014, 53, 6402–6406 CrossRef CAS PubMed.
- X. G. Gu, J. J. Yao, G. X. Zhang, C. Zhang, Y. L. Yan, Y. S. Zhao and D. Q. Zhang, Chem. – Asian J., 2013, 8, 2362–2369 CrossRef CAS PubMed.
- M. Hultberg and B. Hultberg, Chem.-Biol. Interact., 2006, 163, 192–198 CrossRef CAS PubMed.
- S. C. Lu, Curr. Top. Cell. Regul., 2000, 36, 95–116 CAS.
- Y. Chang, K. Yang, P. Wei, S. Huang, Y. Pei, W. Zhao and Z. Pei, Angew. Chem., Int. Ed., 2014, 53, 13126–13130 CrossRef CAS PubMed.
Footnotes |
† Electronic supplementary information (ESI) available: Synthesis and characterization of the intermediates; molecular orbital data. See DOI: 10.1039/c5tb02270c |
‡ These authors contributed equally. |
|
This journal is © The Royal Society of Chemistry 2016 |
Click here to see how this site uses Cookies. View our privacy policy here.