DOI:
10.1039/C2FO10248J
(Paper)
Food Funct., 2012,
3, 537-546
Coupling in vitro gastrointestinal lipolysis and Caco-2 cell cultures for testing the absorption of different food emulsions
Received
9th November 2011
, Accepted 18th January 2012
First published on 21st February 2012
Abstract
There is a growing interest in the optimization of dietary emulsions for monitoring postprandial lipid metabolism in the frame of preventing metabolic diseases. Using various emulsions, we investigated in a systematic scheme the combination of (i) in vitro gastrointestinal lipolysis and (ii) absorption and metabolism of lipolysis media in Caco-2 cells. Four emulsions based on either milk fat olein (OL) or rapeseed oil (RA) as the dispersed phase and either lecithin (LE) or sodium caseinate (CA) as the emulsifier were tested. After a sequential incubation of these emulsions with gastric and pancreatic enzymes, lipolysis media were incubated with Caco-2 cells, after dilution (1
:
20) to maintain the barrier integrity. Both gastric and duodenal lipolysis levels were similar to values reported in vivo and the rates of lipolysis were higher with LE-stabilized emulsions than with CA-stabilized emulsions (P < 0.05). TAG secretion by Caco-2 cells was found to be higher using (i) duodenal vs. gastric media (P < 0.001) and (ii) emulsions stabilized with CA vs. LE (P < 0.01). Consistently, gene expression of both FABP2 and FATP4 induced by the duodenal media was (i) higher than that with gastric media (P < 0.001) and (ii) faster than that with model mixed micelles. Using gastric media, TAG secretion of Caco-2 cells after 12 h was higher with RA than with OL (P < 0.001). Moreover, the RA–CA emulsion increased the size of secreted lipoprotein particles (514 nm vs. 61 to 130 nm; P < 0.01). In conclusion, it was possible to observe distinct responses in the lipid metabolism of Caco-2 cells incubated with lipolysis media obtained from different dietary emulsions digested by gastrointestinal lipases in vitro.
Introduction
Obesity, diabetes and atherosclerosis are diseases generally associated to a hyperlipidic diet and have become major public concerns. In this context, particularly in type 2 diabetes, a high postprandial hypertriglyceridemia increases the risk to develop cardiovascular diseases. High fat diets are therefore not recommended. However, lipids are naturally occurring in numerous foodstuffs. They are also widely used as food ingredients because of their capacity to bring texture, flavour and taste. In this respect, oils and fats exist under various forms and structures in food products. In particular, dietary lipids, mainly found as triacylglycerols (TAG), can be present as oil-in-water emulsions with different droplet sizes and stabilized by different surfactant types (phospholipids, proteins, etc.). Until recently, nutritional studies on dietary oils and fats only considered the impact of their global intake and their total fatty acid composition. The effect of their structure (e.g., fatty acid position on the glycerol backbone) and/or their physical state (emulsified or not, droplet size, etc.) remained poorly described. Because of their relevance, these parameters are now a subject of growing research interest.1–6 In rats, Michalski et al. report that the emulsified structure influences the overall fat metabolism kinetics including lipolysis, absorption and oxidation steps.7,8 Similar observations were recently reported in humans.9 Some studies have shown, in healthy subjects and type 2 diabetics, differences in postprandial triglyceridemia after ingestion of butter, milk and cheese.10–12 In rats and in humans, increased fatty acid bioavailability was observed using oil-in-water emulsions.6,13 Moreover, in humans, the fatty acid composition and the emulsion droplet size affect chylomicron size and lipemia kinetics, respectively.14,15
Therefore, it now becomes important to better understand the effect of emulsion structure on lipolysis and absorption steps, considering that little information is available about the effect of oil and surfactant types.16,17 It is necessary to develop in vitro models to rapidly test the digestion and intestinal absorption of emulsions in physiological-like conditions. Meanwhile, the concept of bioavailability involves the fact that only a proportion of the nutrient provided by food is made available to target tissues after ingestion of that food. Therefore, bioavailability is influenced by the different stages of lipid metabolism: enzymatic digestion (the so-called gastrointestinal lipolysis), intestinal absorption, nutrient delivery and use by target tissues. Functional foods are meant to improve a target function or to reduce the risk of developing a pathology. Thus, a primary concern is the control of the metabolic fate of TAG by selecting their form of presentation.
In this context, the objective of the present study was two-fold. First, we studied the influence of the physical form (two types of emulsion) of two dietary lipid sources with different fatty acid compositions on the lipolysis kinetics by lipases of the gastrointestinal tract. Second, using Caco-2 cells, we investigated the intestinal absorption and the lipid metabolism in intestinal cells of the lipolysis products to test the technical feasibility of the model and possible limitations.
Materials and methods
Materials
Two oils were used as the dispersed phase of the oil-in-water (O/W) emulsions: rapeseed oil (RA) (Lesieur, Fleur de colza), and olein issued from the fractionation of milk fat (OL) (Fléchard, France). Sodium caseinate salt (CA) from bovine milk and sodium azide were obtained from Sigma–Aldrich (Germany) and Merck (Germany), respectively. Soya lecithin (LE) (Centrolex D) from Central Soya (USA) was used.
All chemicals, bovine bile and porcine pancreatic extract (PPE) were purchased from Sigma–Aldrich–Fluka Chemistry (St-Quentin-Fallavier, France) except the internal standard (IS; CholE1 = O-cholesteryl ethyleneglycol) used for the analysis of lipolysis products by thin-layer chromatography coupled with flame ionization detection (TLC-FID) kindly provided by Dr Dominique Lafont (ICBMS Lyon, France).
Rabbit gastric extract (RGE) was produced by GERME S.A. company (Marseille, France).
All components of mixed micelles, i.e. oleic acid, 2-oleyglycerol, soybean lecithin (phosphatidylcholine), sodium taurocholate, L-α-lysophosphatidylcholine (lyso-PC) from egg yolk and cholesterol were purchased from Sigma–Aldrich–Fluka Chemistry (St-Quentin-Fallavier, France).
Bulk emulsion preparation
Four O/W emulsions were prepared at room temperature: OL–LE (olein–lecithin), RA–CA (rapeseed–caseinate), OL–CA (olein–caseinate) and RA–LE (rapeseed–lecithin). The formulation protocol was specifically chosen in order to reach similar oil droplet sizes in all emulsions, due to the known importance of emulsion specific surface on lipolysis,14 and according to the gained experience on emulsion stability. For the oil droplets stabilized by sodium caseinate, the emulsions were obtained by introducing the oil phase into a 12 wt% sodium caseinate aqueous solution. This oil phase was dispersed into the aqueous phase to obtain an oil fraction of 70 wt%. For the oil droplets stabilized by soya lecithin, the aqueous solution was poured into the oil phase in which 33 wt% lecithin was previously dissolved. The oil phase was dispersed into the aqueous phase at an oil fraction of 38 wt%. Importantly, the fat content of emulsions was extemporaneously adjusted to the same value for in vitro digestion as described below. All aqueous phases contained 0.08 wt% of sodium azide (bactericide agent). Coarse O/W emulsions were first obtained by fragmentation using an Ultraturax apparatus (Janke & KunKel, equipped with a generator axis: 10 mm S25-N-10G, IKA). The emulsions were then made finer by submitting them to a strong shear in a Couette cell (concentric cylinders geometry, Ademtech SA, France), with a gap of 200 μm. Irrespective of the formulation, the applied shear rate was 6300 s−1. The chemical and physical characteristics of the studied emulsions are described in Table 1.
Table 1 Characteristics of the four emulsions used in the experimental design: composition, droplet volume-averaged diameter (d43), droplet specific surface area per gram of fat a (S), oil fatty acid (FA) composition
Emulsion |
RA–CA |
OL–CA |
RA–LE |
OL–LE |
Oil type |
Rapeseed oil |
Olein fraction of milk fat |
Rapeseed oil |
Olein fraction of milk fat |
Surfactant type |
Sodium caseinate |
Sodium caseinate |
Soybean lecithinb |
Soybean lecithin |
d
43, μm |
9.1 ± 0.8 |
8.8 ± 0.4 |
8.6 ± 0.4 |
9.1 ± 0.1 |
S, m2 g−1 |
0.78 ± 0.05 |
0.79 ± 0.02 |
1.07 ± 0.06 |
1.15 ± 0.03 |
Values are mean ± SD, n = 3.
Soybean lecithin contained 13.2% PC, 29.6% PE, 24.6% PI, 23% Lyso-phospholipids.
|
FA profile (%) |
|
|
|
|
4 : 0 to 12 : 0 |
0 |
18 |
0 |
18 |
14 : 0 |
0 |
12 |
0 |
12 |
14 : 1 |
0 |
1 |
0 |
1 |
16 : 0 |
5 |
23 |
5 |
23 |
16 : 1 |
0 |
2 |
0 |
2 |
18 : 0 |
2 |
7 |
2 |
7 |
18 : 1 |
62 |
28 |
62 |
28 |
18 : 2 n = 6 |
20 |
3 |
20 |
3 |
18 : 3 n = 3 |
8 |
2 |
8 |
2 |
20 : 0 |
0 |
3 |
0 |
3 |
20 : 1 |
1 |
0 |
1 |
0 |
Preparation of a solution mimicking human gastric juice
Because human gastric juice is not commonly available and human gastric lipase (HGL) is not produced in vitro at a large scale, a RGE was prepared according to Moreau et al.18 Rabbit gastric lipase (RGL) and HGL are known to display similar specific activities on various TAG.19
The solution mimicking human gastric juice was prepared by mixing 21 mg of RGE powder (containing 1.41% w/w of RGL) with 3 mL of 10 mmol L−1 MES buffer (2-(N-morpholino)ethanesulfonic acid) and 150 mmol L−1 NaCl buffer, pH 6.0, in order to obtain the gastric lipase (GL) mean concentration of 100 μg mL−1 found in human gastric juice.20,21 The GL solution was prepared freshly on the day of the experiments.
The RGL activity was measured at pH 5.5 under GL standard assay conditions using tributyrin as substrate.19
Preparation of a solution mimicking human pancreatic juice mixed with bile
To replace the human pancreatic juice, 500 mg of porcine pancreatic extracts (PPE; Sigma P7545) containing 1.1% w/w of Porcine Pancreatic Lipase (PPL; deduced from lipase activity measurements) and 1.6 mL bovine bile mixture containing 60 mmol L−1 bile salts (i.e. 68 mg of bovine bile, Sigma B3883, in 1.6 mL of Tris 10 mmol L−1 and NaCl 150 mmol L−1 solution, pH 6.0) were mixed with a 150 mmol L−1 NaCl solution to obtain a total volume of 9.1 mL. Bile salt concentration of this solution was 10.5 mmol L−1. The pH of this solution was adjusted at 6.25.
The final PPL and bile salt concentrations in the incubation vessel were 250 μg mL−1 and 4.4 mmol L−1, respectively, during the simulation of the duodenal phase of digestion. It is important for the bile salt concentration to be greater than the critical micellar concentration (1–2 mmol L−1), as observed in intestinal contents.
In vitro gastro-intestinal lipolysis
Both the gastric and the duodenal phases were sequentially simulated. The choice of the experimental conditions to simulate meal fat digestion in vitro was based on the in vivo data obtained during clinical studies in healthy humans.22 The pH values, lipase concentrations, and meal (emulsion)-to-digestive juice ratios were those observed at 50% gastric emptying of a liquid test meal, in the stomach and in the duodenum.
To minimize bacterial contamination as required for further incubation of Caco-2 cells with lipolysis media, all enzyme and buffer solutions were filtered on 0.22 μm size cut-off membranes. All glass vessels and measuring instruments were washed with alcohol.
Each experiment was performed in a 50 mL thermostated vessel (37 °C), equipped with a pH electrode, and a 1-cm magnetic bar for gentle stirring at 1000 rpm. At time zero, a precise amount of emulsion (1 g for RA–CA and OL–CA; 2 g for RA–LE and OL–LE) was mixed with sterilized water to obtain a total volume of 15 mL so that the same amounts of TAG was provided in all experiments (0.7 g per 15 mL of total volume for each emulsion). Three mL of GL solution was then added and the pH was adjusted at 5.5 to simulate the gastric phase of lipolysis. At t = 30 min, 9.1 mL of pancreatic juice–bile solution were added to the reaction mixture, the pH was adjusted to 6.25 and the reaction went on for 60 additional minutes to simulate the duodenal phase of lipolysis.
The following samples were taken for analysis: (i) 1-mL samples from the reaction mixture were collected immediately at time 0 and then at times 15, 29, 40, 50, 60 and 90 min for total lipid extraction and analysis; (ii) additional samples (6 × 0.5 mL in 2-mL sterile cryotubes) of the reaction mixture were taken at times 29, 60 and 90 min, and were immediately frozen in liquid nitrogen for storage at −80 °C for later incubation with Caco-2 cells.
Each experiment was performed in duplicate. Before each experiment, GL and PL activities were measured by the pH-stat technique using previously published methods19,23 in order to verify the stability of enzymes.
Cell culture and treatments
Caco-2/TC7 cells were kindly provided by Monique Rousset (INSERM UMR 505, Paris, France) at passage 30. Cells were routinely cultured on 75 cm2 flasks (Falcon, Becton Dickinson) in DMEM medium without pyruvate containing 4.5 g L−1 glucose (PAA, Les Mureaux, France) supplemented with 20% heat-inactivated fetal calf serum (FCS, PAA), 1% glutamax (PAA), 1% non-essential amino acids (PAA) and 1% antibiotics (penicillin/streptomycin, PAA) and maintained under a 10% CO2 atmosphere at 37 °C. For experiments, cells were seeded on six-well Transwell (Corning Costar Corp, Cambridge) permeable polyester filters (0.4 μm pore size) at a density of 6 × 104 cells cm−2 and grown to confluence in complete medium. Cells were then cultured for 2 weeks in complete medium containing FCS only in the lower compartment. Media were changed in both compartments every two days until total differentiation was achieved (approximately 21 days after seeding onto the Transwell filters). Transepithelial electrical resistance (TEER) was measured every two days, prior to media change, using a Millicell-ERS apparatus (Millipore Corp, USA) and on the day of experiments, before and after cell treatments.
Prior to the start of treatment, Caco-2 cells were incubated for 24 h with serum-free complete medium in both compartments. On the experiment day, monolayers were incubated in the apical compartment with emulsion lipolysis media diluted (1
:
20, targeted in preliminary experiments to avoid toxicity while still presenting significant lipid content) in serum-free complete medium. Additional experiments were performed by incubating cells with mixed oleic acid micelles whose preparation was described previously.24 Each plate contained a control sample with DMEM medium only. All solutions were maintained at 37 °C before use. The basolateral compartment received 2.5 mL of serum free complete medium.
The basolateral media were collected after incubations during 4, 8 and 12 h and were stored at −80 °C in sterile tubes for lipid analysis. After the same incubation times, cell lysates were obtained by rinsing cell layers twice with ice-cold phosphate-buffered saline (PBS) (2.7 mmol L−1 KCl, 137 mmol L−1 NaCl in 10 mmol L−1 phosphate buffer, pH 7.5), scraping the cells into 1 mL of TRI Reagent (Ambion/Applied Biosystems, Courtaboeuf, France), freezing and storing at −80 °C until genomic analysis. The experiments were repeated five times for each lipolysis product formulation.
Particle size measurement
Direct visualization of the oil droplets just after preparation was carried out using a phase-contrast microscope (Axiovert 135 with a water immersion × 100 objective; Zeiss, Germany). The size distribution of the oil droplets was determined by static light-scattering, using a Coulter LS 230 apparatus. The refractive index was 1.33 for the dispersant (water), 1.46 for olein and 1.47 for rapeseed oil. From droplet size distribution, the volume-averaged diameter d43 and the specific surface area S were calculated by the software as previously explained.8,25
The hydrodynamic diameter of lipoproteins secreted by Caco-2 cells was measured by photon correlation spectroscopy using a Malvern Zetasizer NanoS (Malvern, UK). Samples were concentrated by centrifugation at 13 000 rpm for 4 h at 10 °C; supernatants were analysed at 37 °C. The viscosity and the refractive index of the DMEM medium at 37 °C were respectively 0.84 cP and 1.445.
Total lipids were extracted from emulsion-containing media before and in the course of lipolysis, and from Caco-2 basolateral culture media after incubation, according to the Folch procedure.26 Quantification of TAG and lipolysis products [free fatty acids (FFA), diacylglycerols (DAG), monoacylglycerols (MAG)] was performed by TLC-FID using a Iatroscan MK6 (Iatron laboratories, Japan) as described previously.27 Lipolysis level during in vitro digestion was calculated by quantifying moles of FFA versus moles of total acyl chains present in residual TAG, DAG, MAG and FFA.
Gene expression analysis by quantitative PCR
Total RNA was extracted from cells with the TRI Reagent® (Ambion/Applied Biosystems). RNA concentration was measured with Nanodrop ND1000 (Labtech, Palaiseau, France). First-strand cDNAs were synthesized from 1 μg of total RNA in the presence of 100 units of Superscript II (Invitrogen) using a mixture of random hexamers and oligo (dT) primers (Promega, Charbonnières, France). Real-time PCR assays were performed using a Rotor-GeneTM 6000 (Qiagen, Courtaboeuf, France) in a final volume of 20 μl containing 5 μl of a 60-fold dilution of the reverse transcription (RT) reaction medium, 10 μl of reaction buffer from the Absolute QPCR SYBR Green ROX Mix (Abgene, Courtaboeuf, France) and 0.375 μM of the specific forward and reverse primers. For quantification, a standard curve was systematically generated with six different amounts of cDNA. Each assay was performed in duplicate, and validation of the real-time PCR runs was assessed by evaluation of the melting temperature of the products and by the slope and error obtained with the standard curve. TATA box binding protein (TBP) mRNA level was determined in each sample and was used as internal standard for normalization of target mRNA expression. The list of the PCR primers and the quantitative PCR assay conditions are available on request (mailto:emmanuelle.meugnier@pop.univ-lyon1.fr).
Statistical analysis
Multivariate ANOVA followed by Fisher PLSD was used to compare results according to the type of treatment (oil type, emulsifier type, gastric vs. duodenal phase) and incubation time. Point comparisons among emulsions were performed by one-way ANOVA or Student's t-test.
Results
Emulsion lipolysis
The data reported in Table 1 show that we succeeded in preparing emulsion droplets of different compositions with similar droplet sizes between 8.6 and 9.1 μm. Regarding in vitro lipolysis of these emulsions, Fig. 1 shows that the amount of FFA increased with time as a result of TAG lipolysis. The lipolysis kinetics clearly reflected the two phases (gastric vs. duodenal): a low lipolysis level until 29 min (gastric phase) followed by a sudden increase in lipolysis level until 90 min (duodenal phase), regardless of emulsion composition. Table 2 presents the corresponding gastric lipolysis levels at t = 29 min and duodenal lipolysis levels at t = 90 min. Table 3 shows the concentration of the different lipid species present in lipolysis medium during three different lipolysis stages. These data provide information about the lipolysis product concentrations that face the intestinal mucosa. As expected, TAG concentration decreased during lipolysis whereas the concentrations of DAG, MAG and FFA gradually increased (Table 3). Regarding FFA kinetics, differences among emulsions are consistent with the patterns of Fig. 1. Regarding intermediate products of lipolysis, DAG began to be generated during the gastric phase whereas MAG began to be detectable at the beginning of the duodenal phase only. As for FFA release, the MAG concentration increase was greater during the duodenal phase for the lecithin-stabilized emulsions compared with the caseinate-stabilized emulsions. Accordingly, the remaining DAG concentration at 90 min was lower for lecithin-stabilized emulsions (Table 3).
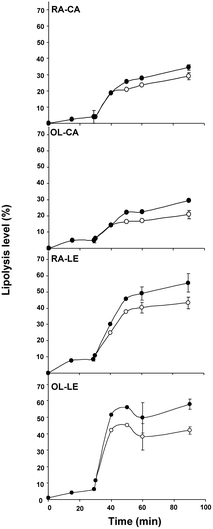 |
| Fig. 1 Lipolysis levels of the different emulsions tested: (○) Free fatty acids (FFA) expressed as a percentage of total FA; (●) FFA and MAG expressed as a percentage of total FA. Values are means ± SD, n = 3. | |
Table 2 Lipolysis levels of different emulsions under in vitro conditions mimicking the gastric and duodenal phases of digestion.a
Emulsion |
Gastric lipolysis (t = 29 min) |
Duodenal lipolysis (t = 90 minb) |
Values are mean ± SD, n = 3.
30 min of gastric-like digestion followed by 60 min of duodenal-like digestion.
P < 0.05 vs. OL–CA, Student's t-test.
|
|
%FFA in total FA
|
RA–CA |
4.0 ± 4.0 |
29.1 ± 2.2 |
OL–CA |
4.8 ± 1.6 |
20.9 ± 2.6 |
RA–LE |
8.4 ± 0.9 |
43.2c ± 3.6 |
OL–LE |
6.2 ± 0.6 |
42.0c ± 2.3 |
Table 3 Variations with time in the lipolysis product concentrations
Emulsion |
Lipolysis Time, min |
Concentration, mmol L−1 |
TAG |
FFA |
DAG |
MAG |
Gastric-like phase.
30 min gastric-like phase followed by duodenal-like phase.
|
RA–CA |
29a |
26.0 |
7.4 |
4.0 |
0.0 |
60b |
7.1 |
10.9 |
6.2 |
2.3 |
90b |
8.5 |
16.8 |
8.2 |
3.7 |
OL–CA |
29 |
18.1 |
5.1 |
5.1 |
0.0 |
60 |
7.2 |
7.3 |
6.0 |
3.0 |
90 |
6.2 |
8.9 |
7.8 |
5.5 |
RA–LE |
29 |
22.2 |
8.6 |
8.6 |
0.0 |
60 |
5.7 |
26.7 |
5.7 |
5.9 |
90 |
3.8 |
27.8 |
5.7 |
8.5 |
OL–LE |
29 |
15.6 |
3.2 |
3.2 |
0.0 |
60 |
3.2 |
19.7 |
4.0 |
5.4 |
90 |
2.4 |
19.3 |
4.8 |
7.2 |
From these data, the TAG conversion rate into DAG and MAG was calculated (Fig. 2). Considering the lipolysis levels obtained up to 90 min of in vitro lipolysis, the data fit a model where TAG was ultimately converted into MAG and FFA without further MAG hydrolysis at this stage (i.e., maximum lipolysis rate of 66.6% within the explored time range). Here again, we observed the enhanced susceptibility to lipolysis of lecithin-covered emulsion droplets compared with caseinate-covered droplets, regardless of the intradroplet oil composition.
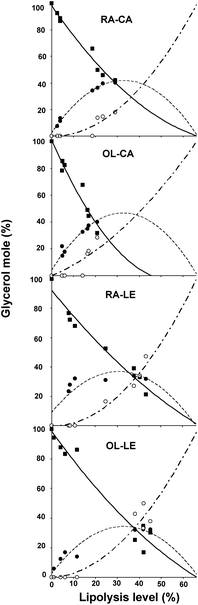 |
| Fig. 2 Triacylglycerol (TAG) conversion into diacylglycerols (DAG) and monoacylglycerols (MAG), represented as percentage of total glycerol esterified onto TAG (■), DAG (●) and MAG (○) during lipolysis. Polynomial trend curves are to guide the eye regarding the kinetics of each species appearance and/or disappearance. Note that 66% on the x-axis represent the highest theoretical lipolysis rate (2 FFA released from 1 TAG). | |
Caco-2 monolayer integrity after incubation with lipolysis media
Fig. 3 shows the evolution of Caco-2 cell monolayer integrity, measured as TEER, during 12 h of incubation with gastric (t = 29 min) or duodenal (t = 60 min) lipolysis media (diluted 1
:
20). TEER values were not affected by incubation with gastric lipolysis media, contrary to duodenal lipolysis media. Indeed, the latter caused a progressive decrease of TEER from 6 to 12 h of incubation, reflecting a loss of cell monolayer integrity possibly due to the presence of components such as bile salts and lysophospholipids.28,29 Consequently, lipid secretion by Caco-2 cells for the 4 duodenal lipolysis media was not studied for incubation times longer than 4 h. In contrast, incubation times of 4, 8 and 12 h were investigated for the media corresponding to the end of the gastric phase (t = 29 min). These conditions were suitable to model phenomena occurring in the upper duodenum. Table 4 presents the composition of lipolysis molecules in the various media incubated with Caco-2 cells.
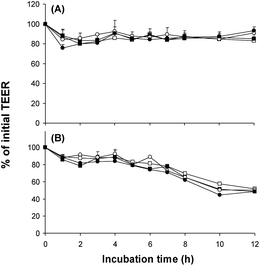 |
| Fig. 3 Effect of apical incubation with different lipolysis media (dilution 1 : 20 in DMEM) on Caco-2 monolayer integrity, expressed as % of initial TEER (transepithelial electrical resistance). (A) Lipolysis media after 29 min of gastric-like digestion; (B) lipolysis media after 60 min of in vitro digestion (30 min of gastric-like digestion followed by 30 min of duodenal-like digestion). (□) RA–CA (rapeseed oil emulsion stabilized with sodium caseinate); (■) OL–CA (olein fraction of milk fat emulsion stabilized with sodium caseinate); (○) RA–LE (rapeseed oil emulsion stabilized with soybean lecithin); (●) OL--LE (olein fraction of milk fat emulsion stabilized with soybean lecithin). Data are means ± SEM, n = 5. | |
Table 4 Lipid composition of lipolysis media incubated on Caco-2 cells and lipid composition of model mixed micelles with oleic acid used in this study and in the literature
Incubation medium |
Concentration, mmol L−1 |
TAG |
FFA |
DAG |
MAG |
Incubation media obtained by 29 min in vitro gastric-like digestion, thus devoid of bile salts (dilution 1 : 20).
Incubation media obtained by 30 min in vitro gastric-like digestion followed by 30 min of duodenal-like digestion of emulsions (dilution 1 : 20), containing 0.22 mmol L−1 of bile salts. Mixed micelles contain 5 mmol L−1 sodium taurocholate only.
Ref. 49.
Ref. 50.
Ref. 51. In each study, oleic acid was used as FFA.
|
29 min lipolysisa |
|
|
|
|
RA–CA |
1.30 |
0.37 |
0.20 |
0 |
OL–CA |
0.91 |
0.25 |
0.25 |
0 |
RA–LE |
1.11 |
0.43 |
0.43 |
0 |
OL–LE |
0.78 |
0.16 |
0.16 |
0 |
60 min lipolysisb |
|
|
|
|
RA–CA |
0.36 |
0.55 |
0.31 |
0.12 |
OL–CA |
0.36 |
0.37 |
0.30 |
0.15 |
RA–LE |
0.29 |
1.33 |
0.29 |
0.29 |
OL–LE |
0.16 |
0.99 |
0.20 |
0.27 |
Mixed micelles |
|
|
|
|
This study |
0 |
0.5 |
0 |
0.2 |
Literature |
0c,d,e |
0.6c; 0.5d; 0.1e |
0c,d,e |
0.2c; 0.03d; 0.1e |
Lipid secretion and gene expression of Caco-2 cells using duodenal vs. gastric media
Fig. 4 shows TAG secretion in basolateral medium by Caco-2 cells after 4 h of incubation with different lipolysis media obtained from the 4 emulsions, in conditions simulating duodenal vs. gastric digestion. Such TAG secretion reflects the extent of lipid absorption and secretion by this cell model. Incubation with duodenal lipolysis media resulted in greater TAG secretion by Caco-2 cells than incubation with gastric lipolysis media (P < 0.001). However, the difference was less pronounced for the RA–LE emulsion. Moreover, the TAG secretion after incubation with duodenal lipolysis media from both caseinate-stabilized emulsions (≥10 μM) was higher than that from lecithin-stabilized emulsions (≤6 μM; P < 0.01).
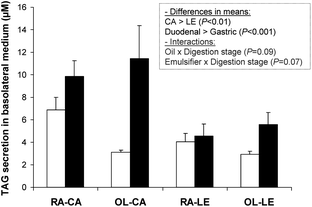 |
| Fig. 4 Triacylglycerols (TAG) secreted by Caco-2 cells after 4 h incubation with different lipolysis media. (□) Lipolysis media after 29 min of gastric-like digestion; (■) lipolysis media after 60 min of in vitro digestion (30 min of gastric-like digestion followed by 30 min of duodenal-like digestion). Data are means ± SEM, n = 5 per treatment. Blank was 2.8 ± 1 mmol L−1. Insert shows results of multivariate ANOVA analysis. | |
Considering the observed differences in TAG secretion, we further explored the impact of the different lipolysis media on the expression of genes related to lipid transport and secretion in Caco-2 cells. Fig. 5 shows the expression of genes coding for fatty acid transporters (FABP2, FATP4) and microsomal triglyceride transfer protein implicated in chylomicron assembly (MTTP), after incubation for 4 h with duodenal vs. 4 h to 8 h with gastric media from the different emulsions. In addition, we compared the gene expression from digestion media with that obtained by incubation of Caco-2 cells with model mixed micelles incubated during 4 and 8 h as usual in the literature. We also measured the gene expression after 12 h of incubation with gastric media, but the results were similar to those obtained after 8 h of incubation regardless of the gene (results not shown).
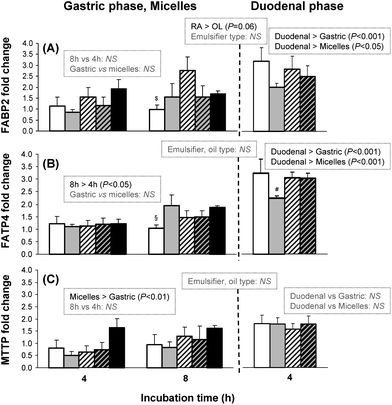 |
| Fig. 5 FABP2, FATP4 and MTTP expressions in Caco-2 cells. Left part: after 4 and 8 h incubation with lipolysis media obtained after 29 min of gastric-like digestion and with mixed micelles. Right part: after 4 h incubation with lipolysis media obtained after 60 min of in vitro digestion (30 min of gastric-like digestion followed by 30 min of duodenal-like digestion). Data are expressed as fold-change vs. blank condition devoid of lipids and are reported as means ± SEM, n = 5 per treatment. Inserts show significant differences observed by multivariate ANOVA analysis (left, gastric media: effect of incubation time, emulsion composition and comparison with micelles; middle, for both gastric and duodenal phases: effect of emulsion composition; right, duodenal media: effect of emulsion composition and comparison with gastric media and with micelles). $P < 0.05 vs. RA–LE; §P < 0.05 vs. OL–CA and micelles; #P < 0.1 vs. other emulsions (one-way ANOVA). (□) RA–CA (rapeseed oil droplets covered with sodium caseinate); ( ) OL–CA (olein fraction of milk fat droplets covered with sodium caseinate); ( ) RA–LE (rapeseed oil droplets covered with soybean lecithin); ( ) OL–LE (olein fraction of milk fat droplets covered with soybean lecithin); (■) mixed micelles. | |
Regarding FABP2, the gene expression tended to be enhanced with RA–CA and RA–LE compared with OL–CA and OL–LE (P < 0.06). FATP4 expression increased with gastric media between 4 and 8 h (Fig. 5; P < 0.05). Duodenal lipolysis media induced increased gene expression of lipid metabolism compared with gastric lipolysis media for both FABP2 and FATP4 (P < 0.001). Most interestingly, incubation with duodenal media induced significant increase of fold-change after 4 h incubation for fatty acid transporters, while at least 8 h of incubation with model mixed micelles were necessary to observe the same effect on gene expression for FABP2 (P < 0.05) and FATP4 (P < 0.001).
However, regarding MTTP expression, no difference was observed among the different emulsions. Mixed micelles with taurocholate were more efficient in inducing MTTP than gastric media, devoid of bile salts. However, duodenal media were not more active than mixed micelles regarding MTTP gene expression.
Lipid secretion by Caco-2 cells using gastric media as a function of incubation time
We further tested lipid secretion by Caco-2 cells using incubation with gastric media, because such media could serve as a model of intestinal content in the upper duodenum where early interactions of lipolysis products with the intestine occur. Fig. 6A shows TAG secretion in the basolateral medium by Caco-2 cells after 4 to 12 h of incubation with different gastric lipolysis media. As soon as 4 h, TAG secretion was higher from lipolysis medium originating from RA–CA emulsion than from other emulsions. After 12 h, RA–LE and OL–CA induced more TAG secretion than OL–LE (Fig. 6A) in particles of similar size (Fig. 6B). In contrast, the size of TAG-containing lipoproteins secreted by Caco-2 cells was significantly higher after 12 h incubation with the latter RA–CA medium (514 ± 162 nm) compared with the media resulting from all other emulsions (Fig. 6B), indicating that RA–CA induced a lower number of larger lipoproteins.
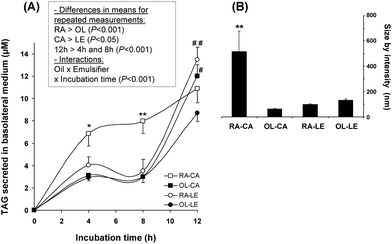 |
| Fig. 6 Lipid secretion by Caco-2 cells after incubation with lipolysis media obtained after 29 min of gastric-like digestion. (A) Triacylglycerols (TAG): (□) RA–CA; (■) OL–CA; (○) RA–LE; (●) OL–LE. Insert shows results of multivariate ANOVA analysis with repeated measurements over incubation time. *P < 0.05 vs. other emulsions, **P < 0.01 vs. other emulsions, #P < 0.05 vs. OL–LE, ##P < 0.01 vs. OL–LE. (B) Corresponding size of lipoproteins secreted after 12h. **P < 0.01 vs. other emulsions. Data are means ± SEM, n = 5 per treatment. | |
Discussion
Emulsion lipolysis
It is worth noting that the emulsion droplet size in the present study was similar to that observed in human stomach and duodenum during gastric digestion of a coarse emulsion.14,30 Regarding lipolysis kinetics, gastric lipolysis levels at t = 29 min (4 to 8.4%; Table 2) for all emulsions tested, were significant and similar to those recorded in vivo in the course of test meal digestion.21,22,31,32
Noticeably, regardless of the oil type, both emulsions stabilized with sodium caseinate underwent similar gastric and duodenal lipolysis kinetics (Fig. 1, RA–CA and OL–CA). This was also the case for both emulsions stabilized with lecithin (Fig. 1, RA–LE and OL–LE). The surfactant type appeared to have a significant effect on the lipolysis levels. Gastric and duodenal lipolysis levels were higher for lecithin-stabilized emulsions than for caseinate-stabilized emulsions (Table 2). Lecithin-stabilized emulsion thus appeared to be better substrates for lipolytic enzymes than sodium caseinate-stabilized emulsions, even though their interfacial area tended to be lower (Table 1). This result is consistent with a previous study showing that oil-in-water emulsions stabilized with lecithin resulted in pancreatic lipolysis levels a few % greater than emulsions stabilized with caseinate using an in vitro model devoid of gastric lipase.33 This observation was interpreted considering that the lipid droplets size in simulated intestinal media was larger in presence of caseinate than in presence of lyso-lecithin;34 differences in flocculation and surface charges were also observed.35 However, the differences we observed in the % of FFA released depending on the emulsifier type were greater using the present and more realistic sequential lipolysis model including gastric and intestinal steps. This result is also consistent with the report that human milk fat globules, of ∼3.5 μm and covered by a phospholipid membrane,36,37 are more efficiently lipolyzed than submicronic fat droplets of infant formula covered with milk proteins.38,39 In cow milk, despite their larger interfacial area, homogenized milk fat droplets are not more efficiently lipolyzed than natural milk fat globules (covered with a phospholipid membrane), possibly because their interface composed mainly of milk proteins slows down lipolytic activity.40In vitro, these emulsion types are usually poor substrates for pancreatic lipase alone and the preliminary action of gastric lipase is necessary for promoting lipolysis.41,42 The differences observed in the lipolysis of caseinate-stabilized and lecithin-stabilized emulsions might therefore mainly result from distinct effects of caseinate and lecithin on gastric lipase activity. Although gastric lipase is more surface-active than pancreatic lipase43 and can adsorb/penetrate at lipid-water interfaces covered by various amphiphiles such as proteins and lecithins, the activity of gastric lipase was shown to be dependent on the nature of these amphiphiles and on interfacial tension.44 Lecithins were found to increase gastric lipase activity on triglyceride emulsions.44 With caseinate-stabilized, the oil/water interfacial tension is ∼12–14 mN m−1 whereas the optimum activity of gastric lipase is recorded at ∼10 mN m−1.44 One can therefore assume that lecithin-stabilized emulsions will be better substrates for gastric lipase activity than caseinate-satbilized emulsions.
Interestingly, the lipolysis level observed in the duodenal phase in our in vitro study was similar to what observed in vivo in humans after ingestion of a coarse emulsion stabilized with both milk proteins and soybean lecithin.14 Moreover, the greater lipolysis activity observed with lecithin vs. caseinate supports the clinical data obtained in a recent human study, in which higher postprandial plasma TAG levels were observed after consumption of egg lecithin-stabilized emulsion compared with those recorded with an emulsion stabilized by caseinate + monoacylglycerols.9 Conversely to the effect of emulsifier type, we observed no significant effect of the oil FA composition (rapeseed vs. milk fat olein) on lipolysis kinetics. This result is different from some reports indicating that the specificity is higher for medium chain fatty triglycerides compared with long-chain triglycerides for bovine lipoprotein lipase45 and for pig pancreatic lipase.46 Importantly, it confirms however that both gastric and pancreatic human lipases can release medium and long chain fatty acids at similar rates.47
Regarding the molecular species generated (Table 3), importantly, the concentrations of FFA released in our in vitro model were similar to those reported in vivo in humans, i.e., 5–20 mmol L−1.14,22,48,49 Identically, the levels of DAG (5–12 mmol L−1) and MAG (0.7–4 mmol L−1) were comparable to those reported in human duodenal fluids during digestion of 10 μm-coarse emulsion.14 The corresponding relative mass% of released FFA in neutral lipid species varied from 17 to 44% (for emulsions OL–CA and RA–LE, respectively) after 90 min of lipolysis. This is also similar to what was observed in vivo in humans at the junction between duodenum and jejunum48 and comparable to what was reported in duodenal fluid, i.e., 16–46%, during digestion of coarse emulsions with the same droplet size as our emulsions.50
In lipolysis media finally incubated onto Caco-2 cells, the concentrations of FFA and MAG in our diluted lipolysis media were in the same range as those used in model mixed micelles (up to 0.6 mmol L−1 for FFA, up to 0.2 mmol L−1 for MAG).51–53 However, the lipolysis media obtained by mimicking real digestion conditions contained TAG and DAG as well as other gastric or duodenal juice components. The latter were thus more realistic than model mixed micelles to mimic the physiological conditions.
Lipid secretion and gene expression of Caco-2 cells using duodenal vs. gastric media
The TAG secretion after 4 h incubation with duodenal lipolysis media is consistent with ∼10 μM reported after 24 h incubation with mixed micelles containing lyso-PC, i.e., 25 nmol per well that is ∼10 μM.54 It is also higher than the secretion of TAG-rich lipoproteins reported after overnight incubation with micelles made of 1.6 mmol L−1 oleic acid and 0.5 mmol L−1 sodium taurocholate, i.e., 2.9 nmol per well or ∼1.5 μM.55 PC was reported to enhance TAG secretion by Caco-2 cells when this phospholipid was included in the model mixed micelles based on oleic acid and sodium taurocholate.56,57 However, in our study, emulsions formulated with sodium caseinate stimulated even more TAG secretion after 4 h than emulsions with lecithin, even if this medium contained less FFA in RA–CA and OL–LA compared with RA–LE and OL–LE (Table 4). The digestion of casein can lead to various bioactive peptides, among which some have been reported to impact Caco-2 cell mitochondrial activity,58 to enhance tight junction barrier,59 to improve calcium absorption,60 and to increase heme iron uptake.61 Therefore, we cannot exclude an impact of casein-derived peptides to account for the observed increased TAG secretion by Caco-2 cells in our in vitro model.
Incubation of Caco-2 cells with duodenal-like media induced enhanced expression of fatty acid transporters (FATP4, FABP2) compared with incubation with model mixed micelles. This was however, not the case for MTTP, a gene involved in chylomicron formation. Interestingly, palmitic acid was reported to down-regulate MTTP expression in Caco-2 cells compared with oleic acid and in absence of lipid.62 It was suggested that oleic acid-rich TAG might present a better ability to form stable lipid droplets than palmitic-rich TAG, which has been shown to be a key factor in enabling core expansion of chylomicron particles in enterocytes.62 Because, in our experiments, cells were exposed to complex lipid profiles including different fatty acids, our data resulted from the combined synergistic effect of different lipids and fatty acids. This could explain the lack of enhancement of MTTP with digested emulsions compared with model mixed micelles. It appears that induction of fatty acid transporters (Fig. 5) was sufficient to provide the observed increase in TAG secretion during incubation with duodenal-like phases (Fig. 4).
Lipid secretion by Caco-2 cells using gastric media as a function of incubation time
The lipoprotein particle sizes (from 61 ± 7 nm for OL–CA to 130 ± 12 nm for OL–LE) were consistent with those reported for large (162 nm) and small chylomicrons (104 nm) secreted by Caco-2 cells after incubation with 1.6 mmol L−1 oleic acid and 0.5 mmol L−1 taurocholate.55 Interestingly, an overall rapeseed oil effect was observed on TAG secretion compared with milk fat olein, which is consistent with the effect of rapeseed oil on FABP2 expression (Fig. 5). Moreover, we observed that the sharp increase in TAG secretion between 8 h and 12 h (i) for RA–LE was associated with an increased expression of FABP2 at 8 h and (ii) for OL–CA, with an increased expression of FATP4 at 8 h. Jackson et al. reported that 96 h-incubation with mixed micelles induced higher TAG secretion using monounsaturated FA (MUFA) (36.6 μM TAG secretion in basolateral medium) compared with polyunsaturated (26.6 μM) and saturated FA (25.3 μM),63 resulting from larger triglyceride-rich lipoproteins as revealed by increased TAG/ApoB ratio using micelles composed of MUFA and taurocholate. Palmitic acid and stearic acid were also reported to lower TAG secretion of Caco-2 cells compared with oleic acid.62 Therefore, the higher TAG secretion observed with RA emulsions could be due to the fact that rapeseed oil contained 3 times less palmitic acid and twice more oleic acid as in milk fat olein (Table 1). However, intracellular accumulation of acylglycerols, i.e. lipid uptake by Caco-2 cells, was found to be increased using capric, lauric or myristic acid compared with oleic, palmitic or stearic acids.53 Therefore, the sudden increase in TAG secretion after 8 h of incubation with olein-based emulsions might be partly due to an enhancing effect of medium-chain fatty acids (Table 1).
We would like to emphasize that RA–CA emulsion was stimulating efficiently TAG secretion by Caco-2 cells, both after gastric and duodenal-like digestion, while this emulsion was not providing the highest lipolysis rate (Fig. 1; Table 2). Results obtained using gastric-like phases showed that it was possible to use such an in vitro model devoid of bile salts to test emulsions.
Conclusions
Caco-2 cells are widely used as an in vitro model for studying fatty acid absorption and intestinal lipid metabolism. However, to be metabolized by Caco-2 cells, lipids must be exposed to realistic intestinal conditions. This is why mixed lipid micelles composed of fatty acids, monoacylglycerols, cholesterol and bile salts are most often used to test lipid absorption and metabolism by Caco-2 cells. However, a new research field has emerged these last few years by acknowledging the importance of the physico-chemical and supramolecular structure of fats and oils in food products on their digestion in the gut and further intestinal absorption.1,2,7,8,14,24 These new hypotheses cannot be studied with Caco-2 cells using model mixed micelles. In this study, it was demonstrated the the in vitro digestion fluids resulting from food emulsions could be used to incubate on Caco-2 cells as a new model for testing emulsion digestion and absorption. Moreover, we provided experimental evidence that the emulsion composition influenced the activation of lipid metabolism and TAG secretion. Further work is now to be performed in order to elucidate the impact of the food emulsion structure on its fate in digestive conditions and ultimate lipid absorption.
Acknowledgements
This work received the financial support of the Institut Carnot LISA (Lipides pour l’Industrie et la Santé) and Agence Nationale de la Recherche (ANR-07-CARN-009-01). Dr Mathieu Schue (EIPL CNRS-UMR7282, Marseille) is acknowledged for contributing to the lipid analysis by TLC-FID. We thank Dr Fernando Leal-Calderon for his careful reading of the manuscript.
References
- D. J. McClements, E. A. Decker, Y. Park and J. Weiss, Food Biophys., 2008, 3, 219–228 CrossRef.
- H. Singh, A. Q. Ye and D. Horne, Prog. Lipid Res., 2009, 48, 92–100 CrossRef CAS.
- M. C. Michalski, Eur. J. Lipid Sci. Technol., 2009, 111, 413–431 CrossRef CAS.
- L. Marciani, R. Faulks, M. S. Wickham, D. Bush, B. Pick, J. Wright, E. F. Cox, A. Fillery-Travis, P. A. Gowland and R. C. Spiller, Br. J. Nutr., 2008, 101, 919–928 CrossRef.
- D. J. McClements and Y. Li, Adv. Colloid Interface Sci., 2010, 159, 213–228 CrossRef CAS.
- L. Couedelo, C. Boue-Vaysse, L. Fonseca, E. Montesinos, S. Djoukitch, N. Combe and M. Cansell, Br. J. Nutr., 2010, 105, 1026–1035 CrossRef.
- M. C. Michalski, V. Briard, M. Desage and A. Geloen, Eur. J. Nutr., 2005, 44, 436–444 CrossRef CAS.
- M. C. Michalski, A. F. Soares, C. Lopez, N. Leconte, V. Briard and A. Geloen, Eur. J. Nutr., 2006, 45, 215–224 CrossRef CAS.
- J. B. Keogh, T. J. Wooster, M. Golding, L. Day, B. Otto and P. M. Clifton, J. Nutr., 2011, 141, 809–815 CrossRef CAS.
- A. S. Biong, H. Müller, I. Seljeflot, M. B. Veierod and J. I. Pedersen, Br. J. Nutr., 2004, 92, 791–797 CrossRef CAS.
- G. Clemente, M. Mancini, F. Nazzaro, G. Lasorella, A. Rivieccio, A. M. Palumbo, A. A. Rivellese, L. Ferrara and R. Giacco, Nutr., Metab. Cardiovasc. Dis., 2003, 13, 377–383 CrossRef CAS.
- T. Tholstrup, C. E. Hoy, L. N. Andersen, R. D. K. Christensen and B. Sandstrom, J. Am. Coll. Nutr., 2004, 23, 169–176 Search PubMed.
- I. Garaiova, I. Guschina, S. Plummer, J. Tang, D. Wang and N. Plummer, Nutr. J., 2007, 6, 4 CrossRef.
- M. Armand, B. Pasquier, M. Andre, P. Borel, M. Senft, J. Peyrot, J. Salducci, H. Portugal, V. Jaussan and D. Lairon, Am. J. Clin. Nutr., 1999, 70, 1096–1106 CAS.
- N. Mekki, M. Charbonnier, P. Borel, J. Leonardi, C. Juhel, H. Portugal and D. Lairon, J. Nutr., 2002, 132, 3642–3649 CAS.
- D. J. McClements and Y. Li, Food Funct., 2010, 1, 32–59 CAS.
- N. A. Malaki, A. J. Wright and M. Corredig, Colloids Surf., B, 2011, 83, 321–330 CrossRef.
-
H. Moreau, R. Verger, D. Lecat and J. L. Junien, Eur. Pat., No. 261 016 A1, 1988 Search PubMed.
- H. Moreau, Y. Gargouri, D. Lecat, J. L. Junien and R. Verger, Biochim. Biophys. Acta, Lipids Lipid Metab., 1988, 960, 286–293 CrossRef CAS.
- E. Ville, F. Carriere, C. Renou and R. Laugier, Digestion, 2002, 65, 73–81 CAS.
- F. Carriere, J. A. Barrowman, R. Verger and R. Laugier, Gastroenterology, 1993, 105, 876–888 CAS.
- F. Carriere, C. Renou, V. Lopez, J. De Caro, F. Ferrato, H. Lengsfeld, A. De Caro, R. Laugier and R. Verger, Gastroenterology, 2000, 119, 949–960 CrossRef CAS.
- C. Erlanson and B. Borgström, Scand. J. Gastroenterol., 1970, 5, 293–295 CAS.
- F. Laugerette, C. Vors, A. Geloen, M. A. Chauvin, C. Soulage, S. Lambert-Porcheron, N. Peretti, M. Alligier, R. Burcelin, M. Laville, H. Vidal and M. C. Michalski, J. Nutr. Biochem., 2011, 22, 53–59 CrossRef CAS.
- M. C. Michalski, F. Michel and C. Geneste, Dairy Sci. Technol., 2002, 82, 193–208 CrossRef CAS.
- J. Folch, M. Lees and G. H. Sloane Stanley, J. Biol. Chem., 1957, 226, 497–509 CAS.
- J. F. Cavalier, D. Lafont, P. Boullanger, D. Houisse, J. Giallo, J. M. Ballester and F. Carriere, J. Chromatogr., A, 2009, 1216, 6543–6548 CrossRef CAS.
- G. P. Martin, L. M. el-Hariri and C. Marriott, J. Pharm. Pharmacol., 1992, 44, 646–650 CrossRef CAS.
- M. L. Lind, J. Jacobsen, R. Holm and A. Mullertz, Eur. J. Pharm. Sci., 2007, 32, 261–270 CrossRef CAS.
- M. Armand, Sci. Aliments, 2008, 28, 84–98 CrossRef CAS.
-
H. Lengsfeld, G. Beaumier-Gallon, H. Chahinian, A. De Caro, R. Verger, R. Laugier and F. Carriere, in Lipases and Phospholipases in Drug Development, ed. S. Petry and G. Müller, Wiley-VCH, New York, 2004, pp. 195–229 Search PubMed.
- F. Carriere, P. Grandval, C. Renou, A. Palomba, F. Priéri, J. Giallo, F. Henniges, S. Sander-Struckmeier and R. Laugier, Clin. Gastroenterol. Hepatol., 2005, 3, 28–38 CrossRef CAS.
- M. Hu, Y. Li, E. A. Decker and D. J. McClements, Food Hydrocolloids, 2010, 24, 719–725 CrossRef CAS.
- S. J. Hur, E. A. Decker and D. J. McClements, Food Chem., 2009, 114, 253–262 CrossRef CAS.
- S. Mun, E. A. Decker and D. J. McClements, Food Res. Int., 2007, 40, 770–781 CrossRef CAS.
- M. C. Michalski, V. Briard, F. Michel, F. Tasson and P. Poulain, J. Dairy Sci., 2005, 88, 1927–1940 CrossRef CAS.
- C. Lopez and O. Menard, Colloids Surf., B, 2011, 83, 29–41 CrossRef CAS.
- M. Armand, M. Hamosh, N. R. Mehta and P. A. Angelus, Pediatr. Res., 1994, 35, A124–A124 CrossRef.
- G. Favé, T. C. Coste and M. Armand, Cell. Mol. Biol., 2004, 50, 815–831 Search PubMed.
- A. Berton, C. Sebban-Kreuzer, S. Rouvellac, C. Lopez and I. Crenon, Mol. Nutr. Food Res., 2009, 53, 1592–1602 CAS.
- Y. Gargouri, G. Pieroni, C. Rivière, P. A. Lowe, J. F. Saunière, L. Sarda and R. Verger, Biochim. Biophys. Acta, Lipids Lipid Metab., 1986, 879, 419–423 CrossRef CAS.
- S. Bernbäck, L. Bläckberg and O. Hernell, Biochim. Biophys. Acta, Lipids Lipid Metab., 1989, 1001, 286–293 CrossRef.
- L. De La Fournière, M. Ivanova, J. P. Blond, F. Carriere and R. Verger, Colloids Surf., B, 1994, 2, 585–593 CrossRef.
- Y. Gargouri, G. Pieroni, P. A. Lowe, L. Sarda and R. Verger, Eur. J. Biochem., 1986, 156, 305–310 CrossRef CAS.
- M. Hultin, A. Mullertz, M. A. Zundel, G. Olivecrona, T. T. Hansen, R. J. Deckelbaum, Y. A. Carpentier and T. Olivecrona, J. Lipid Res., 1994, 35, 1850–1860 CAS.
- M. Bonnet, M. Cansell, A. Berkaoui, M. H. Ropers, M. Anton and F. Leal-Calderon, Food Hydrocolloids, 2009, 23, 92–101 CrossRef CAS.
- Y. Gargouri, G. Pieroni, C. Rivière, J. F. Saunière, P. A. Lowe, L. Sarda and R. Verger, Gastroenterology, 1986, 91, 919–925 CAS.
- B. Borgstrom, A. Dahlqvist, G. Lundh and J. Sjovall, J. Clin. Invest., 1957, 36, 1521–1536 CrossRef CAS.
- O. Hernell, J. E. Staggers and M. C. Carey, Biochemistry, 1990, 29, 2041–2056 CrossRef CAS.
- M. Armand, Curr. Opin. Clin. Nutr. Metab. Care, 2007, 10, 156–164 CrossRef CAS.
- E. Morel, S. Demignot, D. Chateau, J. Chambaz, M. Rousset and F. Delers, Mol. Biol. Cell, 2003, 15, 132–141 CrossRef.
- S. Salvini, M. Charbonnier, C. Defoort, C. Alquier and D. Lairon, Br. J. Nutr., 2002, 87, 211–217 CrossRef CAS.
- W. Tsuzuki, Lipids, 2007, 42, 613–619 CrossRef CAS.
- D. Chateau, T. Pauquai, F. Delers, M. Rousset, J. Chambaz and S. Demignot, J. Cell. Physiol., 2005, 202, 767–776 CrossRef CAS.
- J. Luchoomun and M. M. Hussain, J. Biol. Chem., 1999, 274, 19565–19572 CrossRef CAS.
- D. M. Karpf, R. Holm, C. Garafalo, E. Levy, J. Jacobsen and A. Mullertz, J. Pharm. Sci., 2006, 95, 45–55 CrossRef CAS.
- S. N. Mathur, E. Born, S. Murthy and F. J. Field, Biochem. J., 1996, 314, 569–575 CAS.
- N. M. O'Brien, M. Phelan, S. A. Aherne, D. O'Sullivan and R. J. FitzGerald, J. Dairy Res., 2010, 77, 176–182 CrossRef.
- S. Tanabe and H. Yasumatsu, Br. J. Nutr., 2010, 104, 951–956 CrossRef.
- A. Ferraretto, S. Cosentino, C. Gravaghi, E. Donetti, B. M. Donida, G. Lombardi, M. Bedoni, A. Fiorilli and G. Tettamanti, J. Nutr. Biochem., 2010, 21, 247–254 CrossRef.
- P. Villarroel, S. Flores, F. Pizarro, D. L. de Romana and M. Arredondo, Eur. J. Nutr., 2011, 50, 637–643 CrossRef CAS.
- P. A. Bateman, K. G. Jackson, V. Maitin, P. Yaqoob and C. M. Williams, Biochim. Biophys. Acta, Mol. Cell Biol. Lipids, 2007, 1771, 475–485 CrossRef CAS.
- K. G. Jackson, P. A. Bateman, P. Yaqoob and C. M. Williams, Lipids, 2009, 44, 1081–1089 CrossRef CAS.
|
This journal is © The Royal Society of Chemistry 2012 |
Click here to see how this site uses Cookies. View our privacy policy here.