DOI:
10.1039/D4EA00078A
(Critical Review)
Environ. Sci.: Atmos., 2024,
4, 1113-1128
Aerosolized algal bloom toxins are not inert
Received
6th June 2024
, Accepted 11th August 2024
First published on 12th August 2024
Abstract
Harmful algal blooms (HABs) are projected to become increasingly prevalent, extending over longer periods and wider geographic regions due to the warming surface ocean water and other environmental factors, including but not limited to nutrient concentrations and runoff for marine and freshwater environments. Incidents of respiratory distress linked to the inhalation of marine aerosols containing HAB toxins have been documented, though the risk is typically associated with the original toxins. However, aerosolized toxins in micrometer and submicrometer particles are vulnerable to atmospheric processing. This processing can potentially degrade HAB toxins and produce byproducts with varying potencies compared to the parent toxins. The inhalation of aerosolized HAB toxins, especially in conjunction with co-morbid factors such as exposure to air pollutants from increased commercial activities in ports, may represent a significant exposure pathway for a considerable portion of the global population. Understanding the chemistry behind the transformation of these toxins can enhance public protection by improving the existing HAB alert systems.
 Eric P. Vejerano | Eric Vejerano is an associate professor at the Center for Environmental Nanoscience and Risk at the Department of Environmental Health Sciences at the University of South Carolina. He contributed to pioneering work on environmentally persistent free radicals. His research investigates the interactions of semi/volatile organic compounds on aerosol and nanoparticles. |
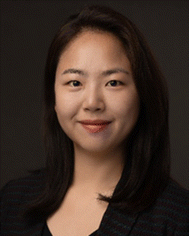 Jeonghyeon Ahn | Jeonghyeon Ahn is a postdoctoral researcher at the University of Iowa, focusing on the development of sampling and analytical methods for indoor and outdoor air pollutants, including volatile organic compounds, per- and polyfluorinated substances, and secondary organic aerosols. Her research interests include risk assessment of exposure to toxic environmental pollutants and the health impacts of emerging contaminants. |
 Geoffrey I. Scott | Geoffrey I. Scott is a clinical professor and Chair of the Department of Environmental Health Sciences at the Arnold School of Public Health, University of South Carolina. Dr Scott is an ecotoxicologist who has directed the research of >63 Masters and PhD students. He has published >200 peer reviewed scientific publications, has authored/co-authored >24 national reports, and has served on numerous federal (White House, National Academy of Science, US Congress), national and international (United Nations; Black Sea Research Institute, Ukraine; Hanse Institute for Advance Learning, Germany) expert panels and research taskforces across the globe. |
Environmental significance
Harmful algal blooms (HABs) are becoming more frequent and widespread due to warming ocean waters and other environmental factors, posing a significant risk to public health. The inhalation of aerosolized HAB toxins has been linked to respiratory distress, yet the potential transformation of these toxins through atmospheric processing, which could yield more potent byproducts, is not well understood. This study highlights the need to address the combined effects of aerosolized HAB toxins and air pollutants, particularly in coastal areas with increased commercial activity. Understanding the chemical transformation of these toxins is crucial for enhancing HAB alert systems and protecting public health in a changing climate and deteriorating ocean health.
|
Introduction
As the climate and ocean health change, inhaling aerosols containing harmful algal bloom toxins (HABs) may be an important unassessed health risk for a significant fraction of the world population living near coastal areas.1–8 In this perspective, we discuss the (1) susceptibility of HAB toxins to transform chemically in nano- and micrometer-sized aerosols; (2) atmospheric processing that may alter the chemistry of the original toxins, modifying their potency; and (3) the impact of this emerging threat to susceptible demographics. Assessing the human health impacts of aerosolized toxins, particularly after atmospheric processing, has been understudied due to the lack of understanding of the chemistry occurring in these aerosols.
As surface ocean temperature increases,9 combined with the contributions from multiple environmental stressors,10 HABs will be prevalent,6 and are expected to worsen (Fig. 1).10–12 The frequency of HAB events has been increasing across temporal and broad geographical coverage.13,14 In 2018–2019, HABs occurred in marine and freshwater systems in Florida, which upended economic and recreational activities related to water bodies.15,16
 |
| Fig. 1 (A) Increase in the incidence of HABs correlating with (B) the trends in the warming of the global sea surface temperature (measured as temperature anomaly) since 1995–2015. Data source. NOAA.9 In panel B, the black curve is the average temperature. The teal and orange curves are uncertainties. Data were taken from four locations: Canada (Ontario), East China Sea, Oman, and USA from 1995 to 2015. (C) Global distribution of HAB events associated with the reported syndromes of poisoning for some HAB toxins. Data was taken from the Harmful Algal Event Database (HEADAT) from 2018 to 2024. Legend: amnesic shellfish poisoning (ASP), azaspiracid shellfish poisoning (AZP), ciguatera fish poisoning (CFP), diarrhetic shellfish poisoning (DSP), neurotoxic shellfish poisoning (NSP), paralytic shellfish poisoning (PSP), and other syndromes (OTHER), which includes effects from aerosolized toxin. | |
Many HAB-forming species are invasive and opportunistic, exploiting altered habitat conditions.17 HAB species continue to be dispersed globally. Species that were once endemic are emerging in new locations. Aureococcus anophagefferens, which was localized in the northeastern US and South Africa, is blooming in coastal China.18Aureoumbra lagunensis is spreading in the Gulf of Mexico to Florida,19 and as far as Cuba.20 Areas that have been previously unaffected now experience recurrent outbreaks. Even freshwater aquatic species can cross over into the marine environment. The dispersal and expansion of these species are driven primarily by warming water temperatures.21–24
Unlike conventional chemical contaminants, whose severity is strongly coupled to emission sources, pollution from HABs is unpredictable because multiple natural and anthropogenic factors modulate their severity. Thus, the magnitude of toxin production is difficult to quantify in a specific waterbody.17 Many HABs are enhanced by high temperatures, hypoxia, eutrophication,13,14 nutrient levels, and other variables.25–30
Fig. 1C shows the global distribution of syndromes associated with HAB toxin exposure from 2018–2024. Syndromes are those associated with amnesic shellfish poisoning (ASP, e.g., domoic acid), azaspiracid shellfish poisoning (AZP, e.g., azaspiracids), ciguatera fish poisoning (CFP, e.g., ciguatoxins), cyanobacterial toxins effects (e.g., microcystins, cylindrospermopsins, and anatoxins), diarrhetic shellfish poisoning (DSP, e.g., okadaic acid and related toxins), neurotoxic shellfish poisoning (NSP, e.g., brevetoxins), paralytic shellfish poisoning (PSP, e.g., saxitoxins), and other syndromes (OTHER), including aerosolized toxin effects. A larger circle denotes more cases of reported syndromes. The map shows the widespread occurrence of reported syndromes across various coastal regions worldwide, specifically along the coastal areas of North America and Europe, the Philippines, and French Polynesia. The map was generated using the Intergovernmental Oceanographic Commission of UNESCO (IOC-UNESCO) Harmful Algae Information System31 and the data contained in it.
Exposure via intake of contaminated fish, shellfish, food, and water containing HAB toxins10 has dominated the discussion. Toxins from some species with adverse human health impacts primarily attributed to ingesting contaminated seafood have garnered attention as they are reported to cause respiratory distress from inhaling marine aerosols.32 Inhaling microdoses of red-tide toxins in sea spray aerosols (SSA) for one hour results in adverse lung function changes in susceptible populations such as asthmatics.33
Marine aerosols from breaking surface ocean waves generate a significant amount of nanoscale SSA that impacts global climate, atmospheric chemistry, and human health.11,34,35 HABs are primarily released from ruptured cells.36 These toxins are aerosolized when breaking ocean waves eject jet and film drops from bursting surface bubbles,37,38 producing SSA.39 Evidence of fragmented aliphatic hydrocarbons that formed an organic film on the particle has been observed in marine aerosol.40 In addition, exposure to HAB toxins can also arise from natural and anthropogenic activities in lakes that release micron-sized lake spray aerosol (LSA).41 During a HAB event, SSA/LSA will contain toxins secreted by algae, cyanobacteria, dinoflagellates, diatoms, and other species.42
Human exposure to HAB toxins in aerosols depends on the particle number concentration of SSA and LSA.43–45 The particle number concentration of SSA/LSA can be as high as ∼5000 cm−3 (ref. 46) depending on the windspeed,47–50 which is 5× the number of particles in indoor air. HAB concentration in air samples has been detected as low as 2 ng m−3. However, brevetoxins, a class of neurotoxins released by Karenia brevis during marine HABs, were detected up to 160 ng m−3 in air samples and as far as 6.4 km inland during the 2018–2019 HAB event in Florida.51 At this concentration, a typical adult would inhale ∼77 ng of brevetoxins in a one-hour exposure. Pumping fresh water for crop irrigation may be an important exposure source that may render HAB toxins airborne, such as cyanotoxin-containing aerosols.52 Inhaling chronic microdoses of brevetoxin, or potentially their degradation products, can impact immune function since they alter human DNA in lymphocytes.53
Physico-chemical conditions existing in aerosols and the dominance of interfacial reactions in the transformation of toxins
The scant studies that investigated the presence of toxins in aerosol have only quantified their concentration levels (Table 1).54 Recently, Shi and colleagues published a number of concentration levels for aerosolized MC congeners.44 Studies on the transformation of aerosolized toxins are scant. Toxins in aerosol should not be assumed inert since nano- and micro-particles are highly reactive vessels.55 Compared to toxins in the bulk ocean water, aerosolized toxins readily undergo atmospheric reactions due to conditions that do not exist in the bulk sea, lake water, or at sea- or lake-atmosphere interface. In aerosols, unique conditions such as enhanced oxidant concentration at the air–liquid interface (ALI), increased uptake of hydrophobic gases, and localized pH differences between the interface and interior may facilitate the toxins' transformation. Such transformation may form byproducts whose potency may differ from the initial toxins. HAB toxins are susceptible to modification via multiple mechanisms similar to those undergone by atmospheric VOCs.56 Reactions in aerosols can occur via (1) interfacial heterogeneous and bulk reactions with diverse gaseous oxidants, (2) pH-catalyzed reactions at the core and interface, and (3) photochemical reactions, primarily at the interface.
Table 1 Selected toxins emitted by various aquatic and marine organisms and their concentration in aerosols
Toxin, molecular structure |
Selected species responsible for production |
Concentration range in aerosols obtained from field measured |
|
Pseudo-nitzschia (phytoplankton) |
No reports |
Pseudo-nitzschia australis
|
Pseudo-nitzschia multiseries
|
Algae |
|
Dinoflagellate Protoperidinium crassipes57 |
No reports |
Azadinium spinosum
|
|
M. aeruginosa, Cylindrospermopsis raciborskii (freshwater cyanobacteria)58 |
50 ± 20 ng m−3 (ref. 59) |
Anabaena, Microcystis, Planktothrix, Nostoc, Oscillatoria, and Aphanizomenon |
0.61 ± 0.75 ppb (ref. 60) |
|
Karenia brevis (marine dinoflagellates)61 |
0.2–24 ng m−3 (ref. 62) |
|
Karenia brevis (dinoflagellates) |
No reports |
|
Gambierdiscus toxicus (dinoflagellates) and Ostreopsis siamensis (diatoms)63 |
No reports |
|
Gambierdiscus (dinoflagellates)64 |
No reports |
|
Ostreopsis
65–67
|
Detected in aerosols68,69 |
First, oxidants are enriched at the interface. In computational studies,70 gaseous oxidants exhibit minimum free surface energy, resulting in enhanced concentration of aqueous aerosol particles and droplets at the ALI. Hydrophilic gases (e.g., hydroxyl radical (˙OH), H2O2, and ROOR) are enhanced 8× at the ALI relative to the gas phase.70 This result is consistent with the experimental observation that aqueous microdroplets can form H2O2,71 a secondary oxidant, which is localized at the air–water interface.72 The [H2O2] increases with decreasing particle size.55 Even hydrophobic gases, O2 and O3, are concentrated ∼3×, and as much as ∼7× for O3, at the interface relative to the gas phase.70
Note that the modeling study by Vácha et al.70 uses a simple model of a water droplet. The composition of SSA and LSA is more complex, containing various organics, some of which act as surfactants.73 Aerosol particles contain long-chain organics (
C5) that may act as surfactants.74 These organic films may temper toxins' reactions with oxidants (e.g., O3 and ˙OH) at the ALI.75
Second, the localization of gases forms nonhomogeneous pH conditions. Reactions may be different because pH gradients exist in nano/micrometer aerosol particles. As a result of the enhancement of gases at the interface, water-soluble atmospheric gases (e.g., CO2) will alter the pH of aqueous particles, creating a nonhomogeneous condition inside droplets or aerosol particles.76 For nanodroplets, experimental measurement determined that the interfacial pH is ∼2.2 pH units more acidic than in the bulk's interior.77 This result is consistent with computational modeling.78 For micron-sized droplets, the surface is ∼3.6 pH units higher than the core.76 Toxins can undergo pH-dependent reactions depending on their location in aerosol particles. Nonhomogeneous pH conditions may occur easily for LSA but are more complicated for SSA. The high ionic concentration in SSA tempers the solubility of gaseous oxidants,79,80 resulting in a more acidic environment. On the one hand, pH is a function of particle size.81 Aerosol particle size is determined by relative humidity (RH). A typical RH at the marine boundary layer is ∼80%.82 The pH of SSA decreases with increasing RH.79 Ultimately, the pH in aerosol particles is modulated by these confounding factors.
However, pH gradients of aerosol particles, droplets, and films remain an active research area with differing perspectives and ongoing debate.83 For example, de la Puente and Laage84 argue that the air–water interface controls the pH of water droplets and films. Additionally, empirical measurements by Ault and colleagues81 have highlighted the size dependence of pH in aerosol. Thus, until resolved, the overall impact of pH gradients on the reactions of organic compounds in aerosol particles, droplets, and film remains uncertain.
Third, the extent of photochemical reactions of toxins in aerosol particles will be higher than those occurring on the surfaces of sea or lake water systems. Photocatalyzed reactions of toxins may occur easily in airborne particles because of the shorter transmission path length (micrometer down to the nanometer scale). Unlike in oceans, the presence of multiple contaminants in sub-surface ocean water attenuates and scatters light. For nano- and micron-sized particles (<30 μm), the rates of reacting components depend primarily on geometry and dimensionality.55 Hence, the components in nanoparticles (e.g., SSA) are more reactive than those in microparticles (e.g., LSA).76 Indeed, ambient measurement in SSA detected molecular chlorine, formed via heterogeneous photochemical process at the air–particle interface of aerosol particle.85
The current view from modeling and experimental studies is that reactions of gaseous oxidants with aerosol components at the air–particle interface will dominate those in the bulk. Such is the case when ˙OH rapidly oxidizes large aromatic hydrocarbons.86 The ten-fold increase in [˙OH] at the interface results in higher reaction rates than those predicted in the gas and bulk phases.86 It is plausible that large toxins listed in Table 1 will degrade, with oxidation as the primary reaction mechanism. Studies on the degradation of toxins are scant. The few studies only investigated the decay of HAB toxins, such as microcystin-LR (MC-LR), when exposed to O3, ˙OH, and sunlight.87 We posit that the low pH in aerosol will accelerate the rate of breakdown of MC-LR during photolysis as it did in bulk liquid.88
Molecular nature and transformation of HAB toxins
Only a few of the thousands identified marine phytoplankton species produce potent toxins. HAB toxins are of high molecular weight, with large organic structures containing diverse functional groups.10 HAB toxins have over 322 known chemical structures.10 We list in Table 1 the general class of toxins commonly present in water bodies. Some of these toxins exist at relatively high concentrations in aerosol particles (Table 1). Many of these chemical structures contain double bonds, serving as reactive sites for O3 and ˙OH. Table 2 (ref. 89) lists the reaction kinetics of different classes of molecules with O3 and ˙OH. Table 2 depicts that increasing branching enhances reaction rates with ˙OH. Differences in the reaction's rate constant in aerosol particles are to be expected from those reported in the gas phase. We propose reaction pathways that may fragment HAB toxins using the known mechanisms in atmospheric chemistry.90–92
Table 2 Gas-phase rate constants of organic compounds in the atmosphere reacting with ˙OH and O3 at 298 K (ref. 89)
|
Rate constant (k, cm3 per molecule per s) |
Species
|
OH |
O3 |
Alkanes
|
(×10−12) |
|
Methane |
0.00618 |
|
Ethane |
0.254 |
|
Propane |
1.12 |
|
2-Methylbutane |
3.7 |
|
2,3-Dimethylbutane |
5.8 |
|
Alkenes
|
(×10−12) |
(×10−18) |
Isoprene |
101 |
12.8 |
α-Terpinene |
363 |
21 000 |
1,3-Butadiene |
66.6 |
6.3 |
Methyl vinyl ketone |
18.8 |
5.6 |
Methacrolein |
33.5 |
1.2 |
2-Methyl-2-butene |
86.9 |
403 |
Aromatics
|
(×10−12) |
|
Benzene |
1.2 |
|
Toluene |
6.0 |
|
Ethylbenzene |
7.1 |
|
n-Propylbenzene |
6.0 |
|
Others
|
(×10−13) |
|
Acetone |
2.2 |
|
Propionic acid |
12 |
|
Dimethyl ether |
30 |
|
Toxins in aerosol particles are susceptible to oxidation by gaseous oxidants, particularly ˙OH and O3. Here, we described only the reaction of MC-LR and brevetoxin with O3 and ˙OH as they are the primary atmospheric oxidants. In Scheme 1, O3 will preferentially attack the C
C (C*) since increasing substitution near the C
C enhances the rate constant with O3 (Table 2). This reaction forms a primary ozonide (molozonide). The conjugated diene bond of MC-LR is the most reactive site for the reaction with O3.92 Since the primary ozonide is unstable, the peroxy O–O bond and C–C bond cleave simultaneously, forming a Criegee intermediate and carbonyls. In solution, the Criegee intermediate forms a secondary ozonide that eventually forms carbonyl compounds.
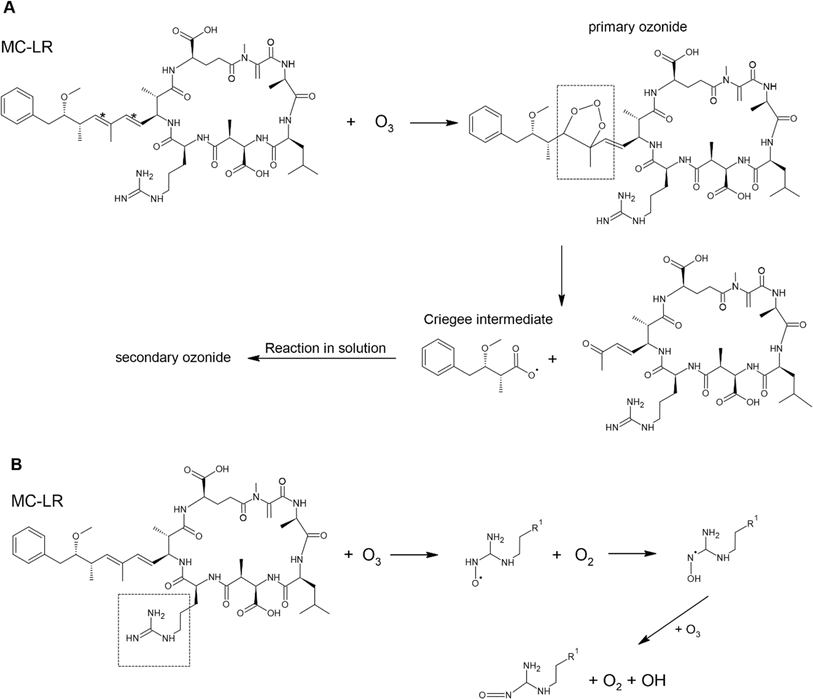 |
| Scheme 1 Possible mechanism of the degradation of MC-LR molecule by O3 due to reaction of (A) C C and (B) C N. | |
Reaction with ˙OH is likely the primary step followed by O3, as depicted in Table 2, subjecting the rings in the HAB toxins to open and fragment.91 A microcosm study demonstrated that under natural sunlight, MC-LR toxin degrades faster than during nighttime due to the involvement of ˙OH. MC-LR in M. aeruginosa aerosol decays with a second-order rate behavior with a lifetime of 54 min.87 In a polluted atmosphere such as those near ports of commerce, [˙OH] is generally taken as 1 × 106 molecules per cm3.90 We used the results from an ab initio model that investigated ˙OH reaction on peptide backbones to determine the potential fragmentation byproducts. These calculations were simulated in the gas phase and used amides, which are less computationally extensive than amino acids.93 We used the results for the acetamides as they are the more relevant model for a cyclic peptide. The designation of the type of carbon (α-, β-, and γ-C) is labeled for MC-LR (Scheme 2).
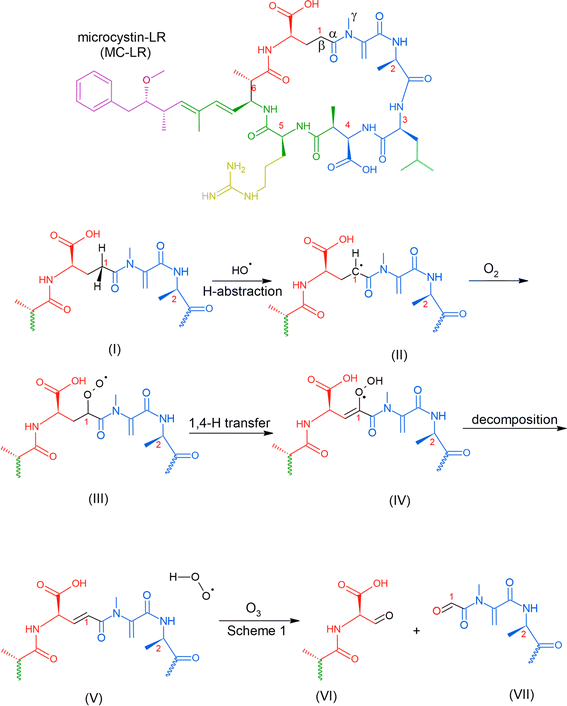 |
| Scheme 2 Possible mechanism of the reaction of MC-LR molecule with ˙OH and O3 that can result in fragmentation. | |
This study obtained multiple H-abstraction pathways: (IA) H-abstraction on the α-C abstraction is kinetically and thermodynamically favored; (IB) a CH3– group on the N results in a secondary pathway that will compete with H-abstraction; (IIA) H-abstraction on the β-C is preferred; (IIB) H-abstraction off the N is the least preferred path unless a CH3– group is present.93 Pathway IB is contrary to that obtained by Štefanić et al.,94 who concluded that the amide N is the preferred site for ˙OH attack on an isolated free glycine and its anion in solution. We used Doan et al.91 because it agreed with an older study conducted in 1970 by Hayon et al.95via an experimental kinetic study performed in an aqueous solution that N is activated for H-abstraction only when methylated (similar to pathway IB).95 In solution, an N-centered radical reaction pathway appears to be favored during ˙OH-induced reaction of peptides and proteins, which releases free amino acids.96
We used this information to determine the possible reaction byproducts for MC-LR, which contains a cyclic heptapeptide after ˙OH and O3 attack (Scheme 2). The carbon centers on the MC-LR peptide backbone that is prone to H-abstraction are labeled 1–6. These carbon centers follow these pathways: C1 (IIA, IB), C2 (IIA, IB), C3 (IIA), C4 (IIA), C5 (IIA, IB), and C6 (IIA). The hypothesized mechanism is depicted in Scheme 2. The first step in the reaction is the abstraction of H by an ˙OH on the β-C on C1, forming a carbon radical (Scheme 2, I). The second step is the addition of an oxygen molecule into the carbon radical (Scheme 2, II). The high concentration of O2 in the aerosol, especially on the interface, suggests O2 addition to be the most likely reaction and is supported by an ab initio modeling study conducted for amides in the gas phase.97 An H atom is abstracted from C adjacent to the β-C, forming a hydroperoxide following a 1,4 H-shift (Scheme 2, III).97 The resulting structure decomposes via hydroperoxide elimination (Scheme 2, IV). Decomposition of hydroperoxide may form a C
C in the structure (Scheme 2, V), which subsequently reacts with O3 following the mechanism illustrated in Scheme 1, yielding carbonyls (Scheme 2, VI and VII). A similar attack by ˙OH on C6 (following a similar mechanism in Scheme 2) and subsequent ozonolysis (Scheme 1) yield (2R)-3-oxo-2-(2-oxopropanamido)propanoic acid as a possible fragment. Applying the mechanisms illustrated in Schemes 1 and 2, we list some potential degradation products due to the ring-opening of the cyclic heptapeptide in MC-LR in Fig. 2. Attack on C
C, yields (2R,3S)-3-methoxy-2-methyl-4-phenylbutanal, attack on C1 and C4 yields 12-methyl-2,4,5,7,8,10,11,13,14-nonaoxo-3,6,9,12-tetraazatetradecan-1-oic acid, and attack on C4, C5, and C6 yield N1-[(2E)-4-oxopent-2-enoyl]-N2-(2-oxopropanoyl)ethanediamide, attack on C5 yields N-(3-oxopropyl)guanidine. An ˙OH attack on C3 yields isobutyraldehyde and ozonolysis on the C
C located between C1 and C2 yields formaldehyde.
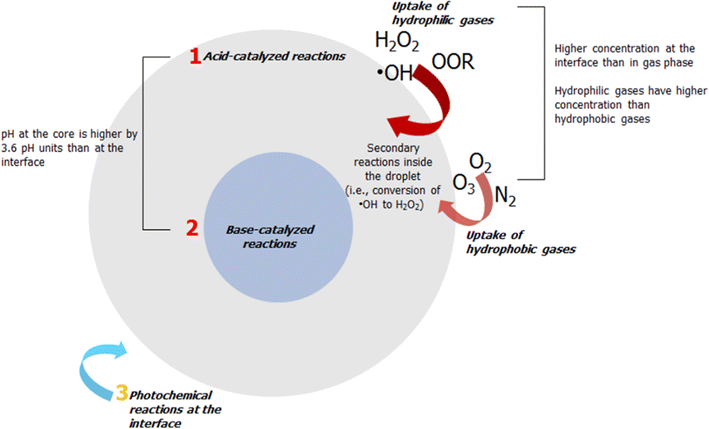 |
| Fig. 2 Conceptual scheme of the possible reaction pathways of the atmospheric processing of toxins in aerosols. These reactions include: (1) acid-catalyzed reactions at the interface and (2) base-catalyzed reactions at the core, (3) photochemical reactions at the interface. Interfacial reactions will dominate those occurring at the bulk as the concentration of small gaseous oxidants (e.g., ˙OH, O3, O2) are enhanced at the interface,70 resulting in nonhomogeneous condition in the aerosol particle. These gaseous oxidants will also form secondary oxidants in the droplets, for instance, the conversion of ˙OH to H2O2.55 | |
The ˙OH can also attack the phenyl ring in MC-LR, as shown in Scheme 3, forming an OH-aromatic adduct by H-abstraction yielding an epoxide. Photolysis of the epoxide will form substituted phenol. Reaction with oxygen can yield hydroperoxyl radicals. The addition of ˙OH to the aromatic ring forms an unstable OH-aromatic adduct. Oxidation of this compound by molecular oxygen via gas-phase reaction produces species such as cresol. The ˙OH reacted with the side chain of the amino acid in MC-LR, which might have formed small molecular fragments (Fig. 3).
 |
| Scheme 3 Reaction of ˙OH on the aromatic ring of MC-LR. | |
 |
| Fig. 3 Potential fragmentation products resulting from the degradation of MC-LR by reaction with ˙OH and O3. | |
Cyclic polyethers are a molecular feature common in ciguatoxins, okadaic acids, palytoxins, and brevetoxins (Table 1). These cyclic polyethers may be amenable to ring openings. Experimental studies conducted with the reaction of ˙OH with some representative ethers suggest that they are relatively facile, with rate constants in similar order (10−12) to those depicted in Table 2 for ˙OH.89
As shown in the two studies previously described, there is an agreement between the gas-phase mechanism derived in solutions. Therefore, we expect the mechanism to apply to polyethers contained in aerosol particles.98 The reaction of cyclic ethers with ˙OH is thought to proceed similarly to those for carboxylic acids, aldehydes, and ketones.98,99 The general mechanism illustrated in Scheme 3 for a tetrahydropyran.100 First, a complex is formed via an H bond between the ˙OH and the O atom on the ether (Scheme 3, II). Then, another H-bond between the O atom of the ˙OH and the H atoms in the ether chain is formed, forming five-, six-, and seven-membered ring structures depending on the position of the C atoms to which the H atom is attached (Scheme 3, III). For HAB toxins in Table 2, these would form a five-membered ring complex (Scheme 3). Intramolecular H transfer will eliminate H2O and produce an oxygenated alkyl radical (Scheme 3, IV).
The rate coefficient for H abstraction from an α-CH2– group in ether is about 2× higher than those for α and γ-CH2– groups because a five-membered ring adduct is more strained.98,99 This information suggests that the cyclic ethers depicted in Table 1 are more likely to react due to the ring strain in the resulting adduct (Scheme 3). However, for ˙OH to attack the O atom in a cyclic ether, it should be accessible (i.e., less sterically hindered). Therefore, the initial ˙OH attack is more plausible on larger ether rings such as the seven- and nine-membered ring in brevetoxins, which are the rings designated as B, D, E, F, and G (Scheme 3). Although limited to determining mass-to-charge ratio, open-ring structures of brevetoxins have been identified in marine aerosols.101 Brevetoxins and other similar systems are hydrophobic, known to form a film in marine aerosol, thus enriched at the interface. Reaction with O3 (Scheme 1) will open rings E and F. Reaction with ˙OH and subsequent acid hydrolysis due to the low pH condition existing at the interface76–78 may convert it to an alcohol (Scheme 3, VI). The possible large fragments are illustrated in Fig. 4, including hexanedial, as a small fragment.
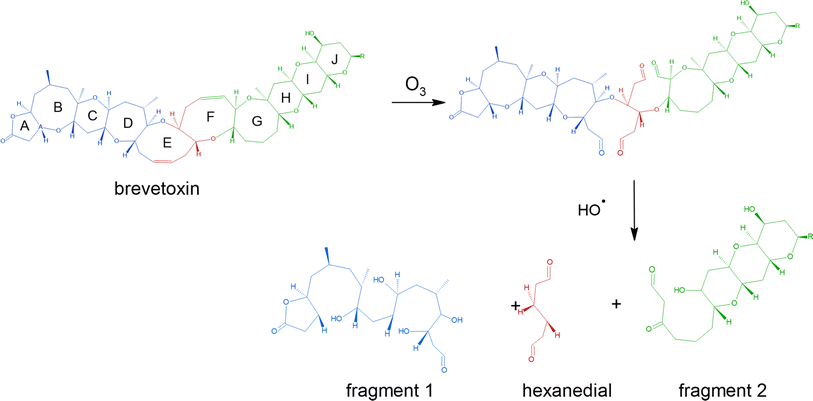 |
| Fig. 4 Possible fragmentation products of brevetoxin with its reaction with O3 and ˙OH. | |
The proposed degradation products primarily consider only the initial step in the bond-breaking process of the toxins with different gaseous oxidants. Succeeding reactions due to these oxidants, internal reactions within the fragments, and reactions between fragments are not discussed to limit the speculative aspect of this critical review.
Variability in aerosol generation and degradation of HAB toxins
The aerosolization of toxins and subsequent atmospheric transport and transformation of these toxins are highly variable across different regions and climates. Factors such as temperature, wind patterns, atmospheric pollutants, and air–sea aerosol dynamics can significantly influence the aerosolization and chemical transformations of HAB toxins in the atmosphere.
In regions with high pollution levels, such as industrialized coastal areas, toxins may partition into airborne particles, increasing their persistence and transport range. In coastal areas with frequent HAB events, warm temperatures can enhance the release and spread of toxins. Higher air temperatures can increase the rate of water evaporation in aerosols, concentrating the toxins. Colder regions may see reduced aerosolization due to lower water temperatures102 and atmospheric dynamics.103 However, climate change is gradually altering these patterns,104 potentially increasing the risk even in historically less affected areas. Humidity levels can alter the size and induce phase separation of aerosol particles.105–108 Low humidity can cause particles to shrink,107 enhancing their inhalation risk.
Windspeeds and patterns47–50 and storm events49,103 further influence the amount of aerosol particle and their dispersal, potentially affecting inland areas. Local wind patterns can also influence toxins' concentration and exposure levels in coastal and inland areas far from the original bloom site.8,51,109 The selective transfer of organic compounds from seawater to the atmosphere significantly varies from particulate organic carbon in the bulk water to those in bubble-bursting aerosols.45 Thus, biological processes and air–sea aerosol dynamics strongly influence aerosol production and marine toxin diffusion.45
Photochemical reactions of HAB toxins as discussed above are influenced by atmospheric chemicals, cloud cover, and air quality. Climate variations, such as El Niño and La Niña, can further impact these processes by altering temperature, precipitation, and storm activity,110 leading to significant year-to-year differences in HAB toxin aerosolization.
Toxicity and fate of degradation products
Although the adverse impact of marine aerosols containing HAB toxins has been documented to result in respiratory distress,111 it is unclear if the toxicity is from the original toxins or includes the contribution from the degradation byproducts. Based on the limited study, it appears that MC-LR in aerosol degrades rapidly.87 We expect that brevetoxins will behave similarly. If so, we posit that adverse effects may substantially modify the potency of the original toxin based on the formation of highly oxidized aldehyde and carbonyl byproducts. As brevetoxins and MC-LR fragment, hexanedial and other aldehydes are produced (Scheme 4). In general, α, β-unsaturated aldehydes—2-butene-1,4-dial and 4-oxo-2-pentenal—are formed when ˙OH opens the rings of benzene and benzene derivatives. This ring-opening reactions were observed in the gas112–114 and liquid phases.115
 |
| Scheme 4 Possible mechanism for the ring opening of a substituted tetrahydropyran (oxane) structure. A plausible ring opening of similar structures in HAB toxins such as those in ciguatoxins, brevetoxins, palytoxins, and okadaic acids. Larger rings are more likely to open because of less steric hindrance for ˙OH attack. | |
Dicarbonyls (e.g., 2-butene-1,4-dial) are known to react with endogenous nucleophiles in the human body, such as N-α-acetyl-lysine, glutathione, and N-acetyl-cysteine, forming highly toxic adducts.115 Such molecular initiating event is a recognized and important mechanism involved in various human health dysfunctions (e.g., the development of cancer and cardiovascular diseases).116,117 The study on phenol degradation suggests that the formation of byproducts can occur in aerosols, which may form toxic adducts in living cells when inhaled.115 The fragmentation products here are shown only for illustrative purposes to describe the potential of a toxin to produce multiple oxidation products. We expect a HAB toxin to form diverse oxidation products as observed for a single VOC after atmospheric processing.56
When HAB toxins undergo fragmentation, their properties can change considerably. The resulting fragmentation products can exhibit different chemical properties, such as altered solubility, stability, and toxicity (less or more potent) relative to the parent toxin. The resulting smaller chemical fragments are often more reactive.
The hepatotoxic microcystin can fragment into various products with differing toxicological profiles. Some known degradation products of microcystin include linearized microcystin and smaller peptides.118 These products can vary in stability and toxicity compared to the parent microcystin.118 Photolysis of MC-LR converts it to the less toxic 6(Z)-Adda MC-LR isomer; however, this reaction is reversible.118–121 Similarly, in bulk water, the neurotoxin saxitoxin degrades into several products, including C8H11N6O2 (223.0937 m/z), C9H13N6O3 (253.1041 m/z), and C10H15N6O4 (283.1149 m/z).122,123 These products have only been identified recently; thus, their toxicities relative to the parent toxin are still unevaluated. Some of these products may be less toxic than the parent compound. For instance, domoic acid can photoisomerize into less toxic isodomoic acids A, B, and C.6,124–127 Recently, domoic acids fragments into smaller compounds as deemed from the smaller m/z ratios.127 The toxicity of this fragments are currently unevaluated. In contrast, others might retain significant or even more potent toxicity, such as the biotransformation of some paralytic shellfish toxins.128
Generally, smaller molecules often possess higher chemical reactivity. Thus, smaller fragments of HAB toxins can form new compounds as they react with those in the environment (i.e., those in the water or aerosol particles) or human body. This increased reactivity can also influence the environmental persistence of these products. Moreover, fragmentation can affect the bioavailability and uptake of these toxins by organisms. The smaller size of fragmentation products can facilitate their absorption through biological membranes, potentially leading to higher internal concentrations and increased adverse effect in the organisms. Additionally, these products may evade detection by conventional monitoring methods, complicating the assessment of HAB-related risks.
Human health impact of increasing HAB events and medical cost of HAB toxins
Airborne exposure to HAB toxins has received increasing attention as recent studies have associated amyotrophic lateral sclerosis with cyanobacterial exposure containing β-methyl-amino-L-alanine (BMAA).129–131 The literature on the neurologic impact of BMAA is conflicting,132 and solid evidence is yet to be established. Similar analogs of the degradation products may mimic the neurodegenerative effects of BMAA due to a potential shift in their toxicity.
During active HAB blooms, reports associated with the incidence of asthma and other respiratory conditions increase by 31–64%. Inhaling aerosols with brevotoxins can result in upper airway symptoms, which can be severe in rare cases.6,111 Emergency room visits and hospital admissions increased 54% in residents within ∼2 km of the coast.6,111 According to The One Health Harmful Algal Bloom System (OHHABS) in the United States, reported in 2019,133 and the Morbidity and Mortality Weekly Report in 2020, most of events occurred in summer and early fall.134 Estimates of the annual costs of brevetoxin illness alone in south FL ranged from $60
000 to $700
000 during periodic blooms.135 Assuming that the average annual illness costs of brevetoxin blooms persist into the future, the capitalized costs of future illnesses would range between $2–24 million annually.33
Vulnerability of susceptible demographics and exacerbation of the impact of HAB toxins along active maritime spaces because of compromised air quality
The human health impact of inhaled HABs affects a large population in coastal areas. In 2010, nearly 2.4 billion people (∼40% of the world's population) lived within 100 km (60 mi) of the coast.1,136 In the US, 40% of Americans living in coastal counties fall into an elevated coastal hazard risk category, including children, the elderly, and those living in poverty.137 Coastal states have a larger senior citizen population than inland states (e.g., FL/US being 24%/18%).137 FL and ME, which experienced frequent and major HAB events, have the highest percentage of senior citizens in the US.138 According to the Global HAB Status Report 2021 by the IOC-UNESCO,139 35–38% of human illness cases affect adults aged 18–45 years, while approximately 40% of those affected are under the age of 18.
More than 80% of global trade occurs in major ports of commerce is located in coastal areas.136 Economic development and specific policies drive coastward migration.140,141 In the US, although coastal regions comprise only 10% of the land area of the lower 48 states,1 they contribute to >50% of the gross domestic product.1 Air and water pollution emanating from activities in commercial ports are higher along the coasts than inland. Exposure to conventional air pollutants such as diesel exhaust particulates (DEP) is highly significant in coastal areas. Compared to roadside vehicle emissions, those from marine vessels are significantly higher due to the longer operating duration and the low quality of fuels.142 The severity of the human health impacts of HABS depends on co-morbidity factors. For instance, cigarette smoking is a risk factor for bacterial and viral infections.143 Severe air pollution levels can significantly increase the risk of death from pathogens such as SARS.144 African Americans and Hispanics are disproportionately exposed to high DEP levels emitted by maritime transportation and increased truck traffic in commercial ports. Approximately 50% of African Americans and Hispanics live near ports, although accounting for only 25% of the US population.142 Such pollution sources can intensify the adverse health impact of HABS in susceptible people.145
Enhancing HAB forecasting system
Currently, alerts warn the public of compromised air quality associated with pollutants such as tropospheric O3 or PM2.5. Current alert warning system for HABs only indicates the location of intense HAB growth conditions in aquatic ecosystems. Generating and coupling these data with existing ground-based and satellite technologies to develop real-time HAB toxin level forecasts is a powerful tool. However, such a system is currently non-existent. The development of such an alert system hinges on determining toxin levels in aerosols, which to date remain scant. Equally important is understanding the complex chemistry that can occur in reactive atmospheric particles due to the atmospheric processing of the toxins. Such forecasts would be a more powerful tool in alerting and protecting the public than the current HAB alert system that only forecasts the location of active blooms.
Limitations and challenges of detecting and monitoring aerosolized HABs
Despite technological advancements, significant limitations remain in detecting and monitoring airborne HAB toxins. Current detection methods often lack the sensitivity to identify low concentrations of aerosolized toxins.146–148 Many techniques, such as traditional air sampling followed by laboratory analysis, can detect toxins but may not be sensitive enough to detect low levels that still pose health risks.146 Specificity is another challenge, as many environmental samples contain various substances that can interfere with detecting specific HAB toxins.149
The available technology limits the real-time monitoring of aerosolized HAB toxins. Most current methods involve collecting and analyzing air samples in a laboratory, which is time-consuming and delay the identification of harmful exposure levels.109,147,150 Technologies like remote sensing and automated detection systems are available for identifying and predicting HAB events but not for measuring aerosolized HAB toxins.151
The spatial and temporal coverage of existing monitoring networks is often insufficient.12,152,153 HAB events can be localized and episodic,154 requiring dense monitoring networks and frequent sampling to capture the full extent of aerosolized toxin spread. Remote and underdeveloped regions may lack the infrastructure and resources needed for effective monitoring, leading to gaps in data and delayed responses to HAB events.
Integrating data from various sources, such as satellite imagery, in situ sensors, and laboratory analyses, remains a significant challenge. Different technologies and methodologies can produce data that are difficult to reconcile into a comprehensive understanding of HAB toxin dispersion. Advanced data analysis techniques, including machine learning and predictive modeling, are underutilized in the field, limiting the ability to effectively predict and respond to HAB events. NASA recently developed a new satellite, the Plankton, Aerosol, Cloud, ocean Ecosystem (PACE) mission, launched on February 8, 2024, to observe HABs.155 Additionally, NASA introduced the Cyanobacteria Finder (CyFi), an AI algorithm that uses machine learning to analyze high-resolution satellite imagery from the Sentinel-2 MultiSpectral Instrument. CyFi identifies high-risk areas of cyanobacteria in small water bodies, enhancing the detection and monitoring of HABs in lakes, reservoirs, and rivers.155 To date, PACE and CyFI are limited to cyanobacterial blooms, but future applications might extend them to other causal organisms.
There is a lack of standardized methods and protocols for detecting and monitoring aerosolized HAB toxins.12,156 This inconsistency can lead to variability in data quality and reliability, complicating efforts to compare and interpret results across different studies and regions. Regulatory frameworks are often slow to adapt to new scientific findings and technological advancements that delay the implementation of effective monitoring and response strategies.12,139 Addressing these limitations will require coordinated efforts between scientists, technologists, policymakers, and public health officials to protect communities and ecosystems from the harmful effects of aerosolized HAB toxins.157
Summary
Some HAB toxins excreted by marine and freshwater organisms have been detected in aerosols. Often, it is assumed that the toxicity of the aerosol is due to the original toxin. However, toxins in aerosol are subject to atmospheric processing, unlike those in the bulk water of marine and aquatic ecosystems. Favorable conditions exist in the atmosphere that renders toxins to react and be transformed. This review describes the possible reactions of select toxins with two major atmospheric oxidants and the formation of degradation products resulting from these reactions. We intend that this review serves as an impetus for future studies that will: (1) elucidate the atmospheric processing and mechanism by which toxins in aerosols are transformed and (2) determine adverse human health impact of the aerosolized toxins and their transformation products. Knowledge of the fate and transformation of toxin in aerosol can enhance the current HAB warning system.
Data availability
This critical review has no associated original unpublished data. All relevant information and existing published data are available in the article.
Author contributions
EPV conceptualized the manuscript. EPV and JA conceptualized the reaction mechanisms and drafted the paper. EPV edited the final manuscript. GS contributed and edited some sections.
Conflicts of interest
We declare we have no competing interests.
Acknowledgements
EPV thank the University of South Carolina ASPIRE I for funding. Research reported in this manuscript was supported in part by the National Institute of Environmental Health Sciences of the National Institutes of Health under Award Number P01ES028942.
References
-
Economics and Demographics, https://coast.noaa.gov/states/fast-facts/economics-and-demographics.html, accessed April 15, 2021 Search PubMed.
- B. Kirkpatrick, L. E. Fleming, D. Squicciarini, L. C. Backer, R. Clark, W. Abraham, J. Benson, Y. S. Cheng, D. Johnson, R. Pierce, J. Zaias, G. D. Bossart and D. G. Baden, Harmful Algae, 2004, 3, 99–115 CrossRef PubMed.
- L. C. Backer, W. Carmichael, B. Kirkpatrick, C. Williams, M. Irvin, Y. Zhou, T. B. Johnson, K. Nierenberg, V. R. Hill, S. M. Kieszak and Y.-S. Cheng, Mar. Drugs, 2008, 6, 389–406 CrossRef CAS PubMed.
- B. Kirkpatrick, R. Pierce, Y. S. Cheng, M. S. Henry, P. Blum, S. Osborn, K. Nierenberg, B. A. Pederson, L. E. Fleming, A. Reich, J. Naar, G. Kirkpatrick, L. C. Backer and D. Baden, Harmful Algae, 2010, 9, 186–189 CrossRef CAS PubMed.
- B. Kirkpatrick, L. E. Fleming, J. A. Bean, K. Nierenberg, L. C. Backer, Y. S. Cheng, R. Pierce, A. Reich, J. Naar, A. Wanner, W. M. Abraham, Y. Zhou, J. Hollenbeck and D. G. Baden, Harmful Algae, 2011, 10, 138–143 CrossRef CAS PubMed.
- J. D. Breidenbach, B. W. French, T. T. Gordon, A. L. Kleinhenz, F. K. Khalaf, J. C. Willey, J. R. Hammersley, R. Mark Wooten, E. L. Crawford, N. N. Modyanov, D. Malhotra, J. G. Teeguarden, S. T. Haller and D. J. Kennedy, Environ. Int., 2022, 169, 107531 CrossRef CAS PubMed.
- A. Lad, J. D. Breidenbach, R. C. Su, J. Murray, R. Kuang, A. Mascarenhas, J. Najjar, S. Patel, P. Hegde, M. Youssef, J. Breuler, A. L. Kleinhenz, A. P. Ault, J. A. Westrick, N. N. Modyanov, D. J. Kennedy and S. T. Haller, Life, 2022, 12, 418 CrossRef CAS PubMed.
- C. C. Lim, J. Yoon, K. Reynolds, L. B. Gerald, A. P. Ault, S. Heo and M. L. Bell, EBioMedicine, 2023, 93, 104604 CrossRef CAS.
-
Extended Reconstructed Sea Surface Temperature (ERSST) | National Centers for Environmental Information (NCEI) formerly known as National Climatic Data Center (NCDC), https://www.ncdc.noaa.gov/data-access/marineocean-data/extended-reconstructed-sea-surface-temperature-ersst, accessed April 19, 2021 Search PubMed.
-
D. Anderson, Harmful Algae 2012 Proc. 15th Int. Conf. Harmful Algae Oct. 29 - Novemb. 2 2012, ed. H. G. Kim, B. Reguera, G. M. Hallegraeff, C. K. Lee, M. S. Han and J. K Choi, Gyeongnam, Korea, 2014, vol. 2012, p. 3–17 Search PubMed.
- D. M. Anderson, J. M. Burkholder, W. P. Cochlan, P. M. Glibert, C. J. Gobler, C. A. Heil, R. Kudela, M. L. Parsons, J. E. J. Rensel, D. W. Townsend, V. L. Trainer and G. A. Vargo, Harmful Algae, 2008, 8, 39–53 CrossRef CAS PubMed.
- D. M. Anderson, A. D. Cembella and G. M. Hallegraeff, Ann. Rev. Mar. Sci, 2012, 4, 143–176 CrossRef PubMed.
- B. W. Brooks, J. M. Lazorchak, M. D. A. Howard, M.-V. V. Johnson, S. L. Morton, D. A. K. Perkins, E.
D. Reavie, G. I. Scott, S. A. Smith and J. A. Steevens, Environ. Toxicol. Chem., 2016, 35, 6–13 CrossRef CAS PubMed.
- B. W. Brooks, J. M. Lazorchak, M. D. A. Howard, M.-V. V. Johnson, S. L. Morton, D. A. K. Perkins, E. D. Reavie, G. I. Scott, S. A. Smith and J. A. Steevens, Environ. Toxicol. Chem., 2017, 36, 1125–1127 CrossRef CAS PubMed.
- E. J. Phlips, S. Badylak, N. G. Nelson and K. E. Havens, Sci. Rep., 2020, 10, 1910 CrossRef CAS PubMed.
-
B. H. Rosen, K. A. Loftin, J. L. Graham, K. N. Stahlhut, J. M. Riley, B. D. Johnston and S. Senegal, Understanding the Effect of Salinity Tolerance on Cyanobacteria Associated with a Harmful Algal Bloom in Lake Okeechobee, Florida, U.S. Geological Survey, Reston, VA, 2018, vol. 2018–5092 Search PubMed.
- B. W. Brooks, J. P. Grover and D. L. Roelke, Environ. Toxicol. Chem., 2011, 30, 1955–1964 CrossRef CAS PubMed.
- Q.-C. Zhang, L.-M. Qiu, R.-C. Yu, F.-Z. Kong, Y.-F. Wang, T. Yan, C. J. Gobler and M.-J. Zhou, Harmful Algae, 2012, 19, 117–124 CrossRef.
- C. J. Gobler, F. Koch, Y. Kang, D. L. Berry, Y. Z. Tang, M. Lasi, L. Walters, L. Hall and J. D. Miller, Harmful Algae, 2013, 27, 29–41 CrossRef.
- F. Koch, Y. Kang, T. A. Villareal, D. M. Anderson and C. J. Gobler, Appl. Environ. Microbiol., 2014, 80, 4947–4957 CrossRef CAS PubMed.
- A. M. Michalak, E. J. Anderson, D. Beletsky, S. Boland, N. S. Bosch, T. B. Bridgeman, J. D. Chaffin, K. Cho, R. Confesor, I. Daloğlu, J. V. DePinto, M. A. Evans, G. L. Fahnenstiel, L. He, J. C. Ho, L. Jenkins, T. H. Johengen, K. C. Kuo, E. LaPorte, X. Liu, M. R. McWilliams, M. R. Moore, D. J. Posselt, R. P. Richards, D. Scavia, A. L. Steiner, E. Verhamme, D. M. Wright and M. A. Zagorski, Proc. Natl. Acad. Sci. U. S. A., 2013, 110, 6448–6452 CrossRef CAS PubMed.
- S. Sunagawa, L. P. Coelho, S. Chaffron, J. R. Kultima, K. Labadie, G. Salazar, B. Djahanschiri, G. Zeller, D. R. Mende, A. Alberti, F. M. Cornejo-Castillo, P. I. Costea, C. Cruaud, F. d’Ovidio, S. Engelen, I. Ferrera, J. M. Gasol, L. Guidi, F. Hildebrand, F. Kokoszka, C. Lepoivre, G. Lima-Mendez, J. Poulain, B. T. Poulos, M. Royo-Llonch, H. Sarmento, S. Vieira-Silva, C. Dimier, M. Picheral, S. Searson, S. Kandels-Lewis, T. O. Coordinators, C. Bowler, C. de Vargas, G. Gorsky, N. Grimsley, P. Hingamp, D. Iudicone, O. Jaillon, F. Not, H. Ogata, S. Pesant, S. Speich, L. Stemmann, M. B. Sullivan, J. Weissenbach, P. Wincker, E. Karsenti, J. Raes, S. G. Acinas and P. Bork, Science, 2015, 348 DOI:10.1126/science.1261359.
- C. M. Gibble, M. B. Peacock and R. M. Kudela, Harmful Algae, 2016, 59, 59–66 CrossRef CAS.
- C. J. Gobler, T. K. Hattenrath-Lehmann, O. M. Doherty, A. W. Griffith, Y. Kang and R. W. Litaker, Proc. Natl. Acad. Sci. U. S. A., 2017, 114, E9765–E9766 CAS.
- J. M. O'Neil, T. W. Davis, M. A. Burford and C. J. Gobler, Harmful Algae, 2012, 14, 313–334 CrossRef.
- H. W. Paerl and V. J. Paul, Water Res., 2012, 46, 1349–1363 CrossRef CAS PubMed.
- S. K. Moore, V. L. Trainer, N. J. Mantua, M. S. Parker, E. A. Laws, L. C. Backer and L. E. Fleming, Environ. Health, 2008, 7, S4 CrossRef PubMed.
- J. Heisler, P. M. Glibert, J. M. Burkholder, D. M. Anderson, W. Cochlan, W. C. Dennison, Q. Dortch, C. J. Gobler, C. A. Heil, E. Humphries, A. Lewitus, R. Magnien, H. G. Marshall, K. Sellner, D. A. Stockwell, D. K. Stoecker and M. Suddleson, Harmful Algae, 2008, 8, 3–13 CrossRef CAS PubMed.
- M. S. Wetz and D. W. Yoskowitz, Mar. Pollut. Bull., 2013, 69, 7–18 CrossRef CAS PubMed.
- D. M. Anderson, P. M. Glibert and J. M. Burkholder, Estuaries, 2002, 25, 704–726 CrossRef.
-
Harmful Algal Bloom (HAB) portal, https://data.hais.ioc-unesco.org/, accessed July 12, 2024 Search PubMed.
- L. Rhodes, Toxicon, 2011, 57, 400–407 CrossRef CAS PubMed.
- L. E. Fleming, B. Kirkpatrick, L. C. Backer, J. A. Bean, A. Wanner, A. Reich, J. Zaias, Y. S. Cheng, R. Pierce, J. Naar, W. M. Abraham and D. G. Baden, Chest, 2007, 131, 187–194 CrossRef CAS PubMed.
- J. R. Pierce and P. J. Adams, J. Geophys. Res.: Atmos., 2006, 111 DOI:10.1029/2005JD006186.
- D. Rosenfeld, R. Lahav, A. Khain and M. Pinsky, Science, 2002, 297, 1667–1670 CrossRef CAS PubMed.
-
R. H. Pierce, M. Henry, P. Blum and S. Payne, Harmful Algal Blooms, 2000, pp. 421–424 Search PubMed.
-
D. C. Blanchard, in Applied Chemistry at Protein Interfaces, American Chemical Society, 1975, vol. 145, pp. 360–387 Search PubMed.
-
R. H. Pierce, M. S. Henry, L. S. Proffitt and P. A. Hasbrouck, Toxic Mar. Phytoplankton, 1990, pp. 397–402 Search PubMed.
- H. R. Pruppacher and J. D. Klett, Nature, 1980, 284, 88 CrossRef.
- H. Tervahattu, K. Hartonen, V.-M. Kerminen, K. Kupiainen, P. Aarnio, T. Koskentalo, A. F. Tuck and V. Vaida, J. Geophys. Res.: Atmos., 2002, 107, AAC 1-1–AAC 1-8 Search PubMed.
- N. W. May, N. E. Olson, M. Panas, J. L. Axson, P. S. Tirella, R. M. Kirpes, R. L. Craig, M. J. Gunsch, S. China, A. Laskin, A. P. Ault and K. A. Pratt, Environ. Sci. Technol., 2018, 52, 397–405 CrossRef CAS PubMed.
- G. A. Codd, L. F. Morrison and J. S. Metcalf, Toxicol. Appl. Pharmacol., 2005, 203, 264–272 CrossRef CAS PubMed.
- S. A. Wood and D. R. Dietrich, J. Environ. Monit., 2011, 13, 1617–1624 RSC.
- J. H. Shi, N. E. Olson, J. A. Birbeck, J. Pan, N. J. Peraino, A. L. Holen, I. R. Ledsky, S. J. Jacquemin, L. C. Marr, D. G. Schmale, J. A. Westrick and A. P. Ault, Environ. Sci. Technol., 2023, 57, 21801–21814 CrossRef CAS PubMed.
- N. I. Medina-Pérez, M. Dall'Osto, S. Decesari, M. Paglione, E. Moyano and E. Berdalet, Environ. Sci. Technol., 2021, 55, 468–477 CrossRef PubMed.
- K. Moore, M. Krudysz, P. Pakbin, N. Hudda and C. Sioutas, Aerosol Sci. Technol., 2009, 43, 587–603 CrossRef CAS.
- J. H. Slade, T. M. VanReken, G. R. Mwaniki, S. Bertman, B. Stirm and P. B. Shepson, Geophys. Res. Lett., 2010, 37 DOI:10.1029/2010GL043852.
- G. de Leeuw, E. L. Andreas, M. D. Anguelova, C. W. Fairall, E. R. Lewis, C. O’Dowd, M. Schulz and S. E. Schwartz, Rev. Geophys., 2011, 49 DOI:10.1029/2010RG000349.
- P. K. Quinn, D. B. Collins, V. H. Grassian, K. A. Prather and T. S. Bates, Chem. Rev., 2015, 115, 4383–4399 CrossRef CAS PubMed.
- N. W. May, M. J. Gunsch, N. E. Olson, A. L. Bondy, R. M. Kirpes, S. B. Bertman, S. China, A. Laskin, P. K. Hopke, A. P. Ault and K. A. Pratt, Environ. Sci. Technol. Lett., 2018, 5, 405–412 CrossRef CAS.
- R. H. Pierce, M. S. Henry, P. C. Blum, J. Lyons, Y. S. Cheng, D. Yazzie and Y. Zhou, Bull. Environ. Contam. Toxicol., 2003, 70, 161 CrossRef CAS.
- Z. A. Mohamed and A. M. Al Shehri, Toxicon, 2010, 55, 1346–1352 CrossRef CAS PubMed.
- A. Sayer, Q. Hu, A. J. Bourdelais, D. G. Baden and J. E. Gibson, Arch. Toxicol., 2005, 79, 683–688 CrossRef CAS PubMed.
- R. H. Pierce, Toxicon, 1986, 24, 955–965 CrossRef CAS PubMed.
- C. Zhu and J. S. Francisco, Proc. Natl. Acad. Sci. U. S. A., 2019, 116, 19222–19224 CrossRef CAS PubMed.
- D. Grosjean, E. L. Williams, E. Grosjean, J. M. Andino and J. H. Seinfeld, Environ. Sci. Technol., 1993, 27, 2754–2758 CrossRef CAS.
-
X. Wu, L. Hou, X. Lin and Z. Xie, in Novel Nanomaterials for Biomedical, Environmental and Energy Applications, ed. X. Wang and X. Chen, Elsevier, 2019, pp. 353–414 Search PubMed.
- C. M. Schreidah, K. Ratnayake, K. Senarath and A. Karunarathne, Chem. Res. Toxicol., 2020, 33, 2225–2246 Search PubMed.
- N. E. Olson, M. E. Cooke, J. H. Shi, J. A. Birbeck, J. A. Westrick and A. P. Ault, Environ. Sci. Technol., 2020, 54, 4769–4780 CrossRef CAS PubMed.
- A. M. Schaefer, L. Yrastorza, N. Stockley, K. Harvey, N. Harris, R. Grady, J. Sullivan, M. McFarland and J. S. Reif, Harmful Algae, 2020, 92, 101769 CrossRef CAS PubMed.
- D. G. Baden, A. J. Bourdelais, H. Jacocks, S. Michelliza and J. Naar, Environ. Health Perspect., 2005, 113, 621–625 CrossRef CAS PubMed.
- R. H. Pierce, M. S. Henry, P. C. Blum, S. L. Hamel, B. Kirkpatrick, Y. S. Cheng, Y. Zhou, C. M. Irvin, J. Naar, A. Weidner, L. E. Fleming, L. C. Backer and D. G. Baden, Harmful Algae, 2005, 4, 965–972 CrossRef CAS.
- K. D. Cusick and G. S. Sayler, Mar. Drugs, 2013, 11, 991–1018 CrossRef CAS PubMed.
- M. A. Friedman, M. Fernandez, L. C. Backer, R. W. Dickey, J. Bernstein, K. Schrank, S. Kibler, W. Stephan, M. O. Gribble, P. Bienfang, R. E. Bowen, S. Degrasse, H. A. Flores Quintana, C. R. Loeffler, R. Weisman, D. Blythe, E. Berdalet, R. Ayyar, D. Clarkson-Townsend, K. Swajian, R. Benner, T. Brewer and L. E. Fleming, Mar. Drugs, 2017, 15, 72 CrossRef PubMed.
- P. Durando, F. Ansaldi, P. Oreste, P. Moscatelli, L. Marensi, C. Grillo, R. Gasparini, G. Icardi and Collaborative Group for the Ligurian Syndromic Algal Surveillance, Eurosurveillance, 2007, 12, E070607 Search PubMed.
- L. Tichadou, M. Glaizal, A. Armengaud, H. Grossel, R. Lemée, R. Kantin, J.-L. Lasalle, G. Drouet, L. Rambaud, P. Malfait and L. de Haro, Clin. Toxicol., 2010, 48, 839–844 CrossRef PubMed.
- P. Ciminiello, C. Dell'Aversano, E. D. Iacovo, E. Fattorusso, M. Forino, L. Tartaglione, G. Benedettini, M. Onorari, F. Serena, C. Battocchi, S. Casabianca and A. Penna, Environ. Sci. Technol., 2014, 48, 3532–3540 CrossRef CAS PubMed.
-
Suspected Palytoxin Inhalation Exposures Associated with Zoanthid Corals in Aquarium Shops and Homes — Alaska, 2012–2014, https://www.cdc.gov/mmwr/preview/mmwrhtml/mm6431a4.htm, accessed July 25, 2024 Search PubMed.
-
M.-T. Fernández-Sánchez, D. Cabrera-García, A. Pérez-Gómez and A. Novelli, in Advances in Neurotoxicology, ed. A. Novelli, M.-T. Fernández-Sánchez, M. Aschner and L. G. Costa, Academic Press, 2021, vol. 6, pp. 223–273 Search PubMed.
- R. Vácha, P. Slavíček, M. Mucha, B. J. Finlayson-Pitts and P. Jungwirth, J. Phys. Chem. A, 2004, 108, 11573–11579 CrossRef.
- J. K. Lee, K. L. Walker, H. S. Han, J. Kang, F. B. Prinz, R. M. Waymouth, H. G. Nam and R. N. Zare, Proc. Natl. Acad. Sci. U. S. A., 2019, 116, 19294–19298 CrossRef CAS PubMed.
- S. Du, J. S. Francisco and S. Kais, J. Chem. Phys., 2009, 130, 124312 CrossRef PubMed.
- T. H. Bertram, R. E. Cochran, V. H. Grassian and E. A. Stone, Chem. Soc. Rev., 2018, 47, 2374–2400 RSC.
- S. H. Jones, M. D. King, A. D. Ward, A. R. Rennie, A. C. Jones and T. Arnold, Atmos. Environ., 2017, 161, 274–287 CrossRef CAS.
- J. F. Davies, R. E. H. Miles, A. E. Haddrell and J. P. Reid, Proc. Natl. Acad. Sci. U. S. A., 2013, 110, 8807–8812 CrossRef CAS PubMed.
- H. Wei, E. P. Vejerano, W. Leng, Q. Huang, M. R. Willner, L. C. Marr and P. J. Vikesland, Proc. Natl. Acad. Sci. U. S. A., 2018, 115, 7272–7277 CrossRef CAS PubMed.
- P.-C. Lin, Z.-H. Wu, M.-S. Chen, Y.-L. Li, W.-R. Chen, T.-P. Huang, Y.-Y. Lee and C. C. Wang, J. Phys. Chem. B, 2017, 121, 1054–1067 CrossRef CAS PubMed.
- A. Malevanets and S. Consta, J. Chem. Phys., 2013, 138, 184312 CrossRef PubMed.
- R. von Glasow and R. Sander, Geophys. Res. Lett., 2001, 28, 247–250 CrossRef CAS.
- K. J. Angle, D. R. Crocker, R. M. C. Simpson, K. J. Mayer, L. A. Garofalo, A. N. Moore, S. L. Mora Garcia, V. W. Or, S. Srinivasan, M. Farhan, J. S. Sauer, C. Lee, M. A. Pothier, D. K. Farmer, T. R. Martz, T. H. Bertram, C. D. Cappa, K. A. Prather and V. H. Grassian, Proc. Natl. Acad. Sci. U. S. A., 2021, 118, e2018397118 CrossRef CAS PubMed.
- A. P. Ault, Acc. Chem. Res., 2020, 53, 1703–1714 CrossRef CAS PubMed.
- A. Laîné, H. Nakamura, K. Nishii and T. Miyasaka, Clim. Dynam., 2014, 42, 2745–2761 CrossRef.
- H. O. T. Pye, A. Nenes, B. Alexander, A. P. Ault, M. C. Barth, S. L. Clegg, J. L. Collett Jr, K. M. Fahey, C. J. Hennigan, H. Herrmann, M. Kanakidou, J. T. Kelly, I.-T. Ku, V. F. McNeill, N. Riemer, T. Schaefer, G. Shi, A. Tilgner, J. T. Walker, T. Wang, R. Weber, J. Xing, R. A. Zaveri and A. Zuend, Atmos. Chem. Phys., 2020, 20, 4809–4888 CrossRef CAS PubMed.
- M. de la Puente and D. Laage, J. Am. Chem. Soc., 2023, 145, 25186–25194 CrossRef CAS PubMed.
- E. M. Knipping, M. J. Lakin, K. L. Foster, P. Jungwirth, D. J. Tobias, R. B. Gerber, D. Dabdub and B. J. Finlayson-Pitts, Science, 2000, 288, 301–306 CrossRef CAS PubMed.
- A. Tabazadeh, R. J. Yokelson, H. B. Singh, P. V. Hobbs, J. H. Crawford and L. T. Iraci, Geophys. Res. Lett., 2004, 31 DOI:10.1029/2003GL018775.
- M. Jang, D. E. Berthold, Z. Yu, C. Silva-Sanchez, H. D. Laughinghouse IV, N. D. Denslow and S. Han, Environ. Sci. Technol. Lett., 2020, 7, 740–745 CrossRef CAS.
- W. Song, S. Bardowell and K. E. O'Shea, Environ. Sci. Technol., 2007, 41, 5336–5341 CrossRef CAS PubMed.
-
Chemistry of the Upper and Lower Atmosphere, 1st edn, https://www.elsevier.com/books/chemistry-of-the-upper-and-lower-atmosphere/finlayson-pitts/978-0-12-257060-5, accessed April 19, 2021 Search PubMed.
- R. G. Prinn, R. F. Weiss, B. R. Miller, J. Huang, F. N. Alyea, D. M. Cunnold, P. J. Fraser, D. E. Hartley and P. G. Simmonds, Science, 1995, 269, 187–192 CrossRef CAS PubMed.
- H. Q. Doan, A. C. Davis and J. S. Francisco, J. Phys. Chem. A, 2010, 114, 5342–5357 CrossRef CAS PubMed.
- X. He, M. Pelaez, J. A. Westrick, K. E. O'Shea, A. Hiskia, T. Triantis, T. Kaloudis, M. I. Stefan, A. A. de la Cruz and D. D. Dionysiou, Water Res., 2012, 46, 1501–1510 CrossRef CAS PubMed.
- H. Q. Doan, A. C. Davis and J. S. Francisco, J. Phys. Chem. A, 2010, 114, 5342–5357 CrossRef CAS PubMed.
- I. Štefanić, I. Ljubić, M. Bonifačić, A. Sabljić, K.-D. Asmus and D. A. Armstrong, Phys. Chem. Chem. Phys., 2009, 11, 2256–2267 RSC.
- E. Hayon, T. Ibata, N. N. Lichtin and M. Simic, J. Am. Chem. Soc., 1970, 92, 3898–3903 CrossRef CAS.
- F. Liu, S. Lai, H. Tong, P. S. J. Lakey, M. Shiraiwa, M. G. Weller, U. Pöschl and C. J. Kampf, Anal. Bioanal. Chem., 2017, 409, 2411–2420 CrossRef CAS PubMed.
- N. Borduas, G. da Silva, J. G. Murphy and J. P. D. Abbatt, J. Phys. Chem. A, 2015, 119, 4298–4308 CrossRef CAS PubMed.
- J. Moriarty, H. Sidebottom, J. Wenger, A. Mellouki and G. Le Bras, J. Phys. Chem. A, 2003, 107, 1499–1505 CrossRef CAS.
- I. W. M. Smith and A. R. Ravishankara, J. Phys. Chem. A, 2002, 106, 4798–4807 CrossRef CAS.
- A. Oku, M. Okano and R. Oda, Bull. Inst. Chem. Res., Kyoto Univ., 1965, 43, 303–313 CAS.
- R. H. Pierce, M. S. Henry, P. C. Blum, S. E. Osborn, Y.-S. Cheng, Y. Zhou, C. M. Irvin, A. J. Bourdelais, J. Naar and D. G. Baden, J. Plankton Res., 2011, 33, 343–348 CrossRef CAS PubMed.
- M. E. Salter, E. D. Nilsson, A. Butcher and M. Bilde, J. Geophys. Res.: Atmos., 2014, 119, 9052–9072 CrossRef.
- N. Sahu and A. D. Tangutur, Aerobiologia, 2015, 31, 89–97 CrossRef.
- A. W. Griffith and C. J. Gobler, Harmful Algae, 2020, 91, 101590 CrossRef PubMed.
- Y. You, M. L. Smith, M. Song, S. T. Martin and A. K. Bertram, Int. Rev. Phys. Chem., 2014, 33, 43–77 Search PubMed.
- M. Song, P. F. Liu, S. J. Hanna, R. A. Zaveri, K. Potter, Y. You, S. T. Martin and A. K. Bertram, Atmos. Chem. Phys., 2016, 16, 8817–8830 CrossRef CAS.
- M. A. Freedman, Chem. Soc. Rev., 2017, 46, 7694–7705 RSC.
- Y. Wang, A. Voliotis, Y. Shao, T. Zong, X. Meng, M. Du, D. Hu, Y. Chen, Z. Wu, M. R. Alfarra and G. McFiggans, Atmos. Chem. Phys., 2021, 21, 11303–11316 CrossRef CAS.
- L. Bilyeu, B. Bloomfield, R. Hanlon, J. González-Rocha, S. J. Jacquemin, A. P. Ault, J. A. Birbeck, J. A. Westrick, H. Foroutan, S. D. Ross, C. W. Powers and D. G. Schmale, Environ. Sci.: Atmos., 2022, 2, 1351–1363 Search PubMed.
- M. M. Rogers and R. K. Stanley, Environ. Sci. Technol., 2023, 57, 5501–5503 CrossRef CAS PubMed.
- B. Kirkpatrick, J. A. Bean, L. E. Fleming, G. Kirkpatrick, L. Grief, K. Nierenberg, A. Reich, S. Watkins and J. Naar, Harmful Algae, 2010, 9, 82–86 CrossRef PubMed.
- R. Wu, S. Pan, Y. Li and L. Wang, J. Phys. Chem. A, 2014, 118, 4533–4547 CrossRef CAS PubMed.
- J. F. Hamilton, A. C. Lewis, C. Bloss, V. Wagner, A. P. Henderson, B. T. Golding, K. Wirtz, M. Martin-Reviejo and M. J. Pilling, Atmos. Chem. Phys., 2003, 3, 1999–2014 CrossRef CAS.
- C. Bloss, V. Wagner, M. E. Jenkin, R. Volkamer, W. J. Bloss, J. D. Lee, D. E. Heard, K. Wirtz, M. Martin-Reviejo, G. Rea, J. C. Wenger and M. J. Pilling, Atmos. Chem. Phys., 2005, 5, 641–664 CrossRef CAS.
- C. Prasse, B. Ford, D. K. Nomura and D. L. Sedlak, Proc. Natl. Acad. Sci. U. S. A., 2018, 115, 2311–2316 CrossRef CAS PubMed.
- C. Jenkinson, R. E. Jenkins, J. L. Maggs, N. R. Kitteringham, M. Aleksic, B. K. Park and D. J. Naisbitt, Chem. Res. Toxicol., 2009, 22, 1172–1180 Search PubMed.
- S. M. Rappaport, H. Li, H. Grigoryan, W. E. Funk and E. R. Williams, Toxicol. Lett., 2012, 213, 83–90 CrossRef CAS PubMed.
- I. Gągała and M.-B. Joanna, Pol. J. Environ. Stud., 2012, 21, 1125–1139 Search PubMed.
- J. Goldberg, H. Huang, Y. Kwon, P. Greengard, A. C. Nairn and J. Kuriyan, Nature, 1995, 376, 745–753 CrossRef CAS PubMed.
- K. Tsuji, S. Naito, F. Kondo, N. Ishikawa, M. F. Watanabe, M. Suzuki and K. Harada, Environ. Sci. Technol., 1994, 28, 173–177 CrossRef CAS PubMed.
- K.-I. Harada and K. Tsuji, J. Toxicol., Toxin Rev., 1998, 17, 385–403 CrossRef CAS.
- N. O. G. Jørgensen, R. Podduturi, C. F. Michelsen, T. Jepsen and M. de A. B. Moraes, Water, 2022, 14, 3556 CrossRef.
- K. E. Norris, T. Kurtz, S. Wang, T. Zeng, F. Leresche and F. L. Rosario-Ortiz, ACS ES&T Water, 2024, 4, 346–354 Search PubMed.
- E. F. S. Authority (EFSA), EFSA J., 2009, 7, 1181 CrossRef.
- K. Zabaglo, E. Chrapusta, B. Bober, A. Kaminski, M. Adamski and J. Bialczyk, Algal Res., 2016, 13, 94–101 CrossRef.
- R. Munday, P. T. Holland, P. McNabb, A. I. Selwood and L. L. Rhodes, Toxicon, 2008, 52, 954–956 CrossRef CAS PubMed.
- H. Jin, L. Lian, H. Zhou, S. Yan and W. Song, Chemosphere, 2018, 209, 328–337 CrossRef CAS PubMed.
- M. Wiese, P. M. D'Agostino, T. K. Mihali, M. C. Moffitt and B. A. Neilan, Mar. Drugs, 2010, 8, 2185–2211 CrossRef CAS PubMed.
- W. G. Bradley and D. C. Mash, Amyotrophic Lateral Scler., 2009, 10, 7–20 CrossRef CAS PubMed.
- P. A. Cox, R. Richer, J. S. Metcalf, S. A. Banack, G. A. Codd and W. G. Bradley, Amyotrophic Lateral Scler., 2009, 10, 109–117 CrossRef CAS PubMed.
- J. Pablo, S. A. Banack, P. A. Cox, T. E. Johnson, S. Papapetropoulos, W. G. Bradley, A. Buck and D. C. Mash, Acta Neurol. Scand., 2009, 120, 216–225 CrossRef CAS PubMed.
- D. Nunes-Costa, J. D. Magalhães, M. G-Fernandes, S. M. Cardoso and N. Empadinhas, Front. Aging Neurosci., 2020, 12 DOI:10.3389/fnagi.2020.00026.
-
CDC, Summary Report – One Health Harmful Algal Bloom System (OHHABS), United States, 2021, https://www.cdc.gov/ohhabs/data/summary-report-united-states-2021.html, accessed July 22, 2024 Search PubMed.
- V. A. Roberts, M. Vigar, L. Backer, G. E. Veytsel, E. D. Hilborn, E. I. Hamelin, K. L. Vanden Esschert, J. Y. Lively, J. R. Cope, M. C. Hlavsa and J. S. Yoder, Morb. Mortal. Wkly. Rep., 2020, 69, 1889–1894 CrossRef PubMed.
- P. Hoagland, D. Jin, A. Beet, B. Kirkpatrick, A. Reich, S. Ullmann, L. E. Fleming and G. Kirkpatrick, Environ. Int., 2014, 68, 144–153 CrossRef CAS PubMed.
-
Review of Maritime Transport 2018 | UNCTAD, https://unctad.org/webflyer/review-maritime-transport-2018, accessed April 15, 2021 Search PubMed.
-
Florida Department of Elder Affairs, Florida Profile 2021, https://elderaffairs.org/wp-content/uploads/Florida-Profile-2021.pdf, accessed August 13, 2024 Search PubMed.
-
U. C. Bureau, Population and Housing Unit Estimates, https://www.census.gov/popest, accessed April 15, 2021 Search PubMed.
- G. Hallegraeff, H. Enevoldsen and A. Zingone, Harmful Algae, 2021, 102, 101992 CrossRef PubMed.
- G. McGranahan, D. Balk and B. Anderson, Environ. Urban., 2007, 19, 17–37 CrossRef.
- B. Neumann, A. T. Vafeidis, J. Zimmermann and R. J. Nicholls, PLoS One, 2015, 10, e0118571 CrossRef PubMed.
- A. Rosenbaum, S. Hartley and C. Holder, Am. J. Public Health, 2011, 101, S217–S223 CrossRef PubMed.
- L. Arcavi and N. L. Benowitz, Arch. Intern. Med., 2004, 164, 2206–2216 CrossRef PubMed.
- Y. Cui, Z.-F. Zhang, J. Froines, J. Zhao, H. Wang, S.-Z. Yu and R. Detels, Environ. Health, 2003, 2, 15 CrossRef PubMed.
- D. Mueller, S. Uibel, M. Takemura, D. Klingelhoefer and D. A. Groneberg, J. Occup. Med. Toxicol., 2011, 6, 31 CrossRef CAS PubMed.
- J. J. Chin Chwan Chuong, M. Rahman, N. Ibrahim, L. Y. Heng, L. L. Tan and A. Ahmad, Sensors, 2022, 22, 3144 CrossRef CAS PubMed.
- J. C. Ho, A. M. Michalak and J. Gt, Lakes Reservoirs, 2015, 41, 317–325 Search PubMed.
- K. F. Smith, J. Stuart and L. L. Rhodes, Front. Protistol., 2024, 1 DOI:10.3389/frpro.2023.1305634.
- S. M. Feist and R. F. Lance, Harmful Algae, 2021, 110, 102124 CrossRef CAS PubMed.
- L. C. Backer, D. Manassaram-Baptiste, R. LePrell and B. Bolton, Toxins, 2015, 7, 1048–1064 CrossRef CAS PubMed.
- J. L. Wolny, M. C. Tomlinson, S. Schollaert Uz, T. A. Egerton, J. R. McKay, A. Meredith, K. S. Reece, G. P. Scott and R. P. Stumpf, Front. Mar. Sci., 2020, 7 DOI:10.3389/fmars.2020.00337.
- D. M. Anderson, E. Fensin, C. J. Gobler, A. E. Hoeglund, K. A. Hubbard, D. M. Kulis, J. H. Landsberg, K. A. Lefebvre, P. Provoost, M. L. Richlen, J. L. Smith, A. R. Solow and V. L. Trainer, Harmful Algae, 2021, 102, 101975 CrossRef CAS PubMed.
- C. E. Binding, L. Pizzolato and C. Zeng, Ecol. Indic., 2021, 121, 106999 CrossRef CAS.
- M. L. Wells, B. Karlson, A. Wulff, R. Kudela, C. Trick, V. Asnaghi, E. Berdalet, W. Cochlan, K. Davidson, M. De Rijcke, S. Dutkiewicz, G. Hallegraeff, K. J. Flynn, C. Legrand, H. Paerl, J. Silke, S. Suikkanen, P. Thompson and V. L. Trainer, Harmful Algae, 2020, 91, 101632 CrossRef PubMed.
-
J. Blumenfeld, Applying Machine Learning to Harmful Algal Blooms | Earthdata, https://www.earthdata.nasa.gov/learn/blog/cyanobacteria-finder, accessed July 12, 2024 Search PubMed.
-
Monitoring and management strategies for harmful algal blooms in coastal waters - UNESCO Digital Library, https://unesdoc.unesco.org/ark:/48223/pf0000140545, accessed July 15, 2024 Search PubMed.
-
V. G. Christensen, C. J. Crawford, R. J. Dusek, M. J. Focazio, L. R. Fogarty, J. L. Graham, C. A. Journey, M. E. Lee, J. H. Larson, S. M. Stackpoole, V. Mazzei, E. J. Pindilli, B. A. Rattner, E. T. Slonecker, K. B. McSwain, T. J. Reilly and A. E. Lopez, Interdisciplinary Science Approach for Harmful Algal Blooms (HABs) and Algal Toxins—A Strategic Science Vision for the U.S. Geological Survey, U.S. Geological Survey, 2024 Search PubMed.
|
This journal is © The Royal Society of Chemistry 2024 |
Click here to see how this site uses Cookies. View our privacy policy here.