DOI:
10.1039/D3QI01723K
(Research Article)
Inorg. Chem. Front., 2023,
10, 6909-6917
Luminescence detection of CH2Cl2 by varying Cu⋯Cu interactions in a flexible porous coordination polymer†
Received
28th August 2023
, Accepted 8th October 2023
First published on 10th October 2023
Abstract
Owing to the weakness of the interaction between chlorohydrocarbon and the host framework, the development of reversible and vapochromic coordination polymer (CP)-based luminescence sensors for the detection of CH2Cl2 with a fast response is still challenging. In this study, a flexible Cu(I)-CP, [Cu2I2(PPh3)2(PYZ)] (CIPP, PPh3 = triphenylphosphine, PYZ = pyrazine) is reported. The intra-cluster Cu⋯Cu distance in CIPP is quite sensitive to the external stimuli, resulting in the corresponding luminescence color and intensity change. Based on this feature, the fast (11 s), distinguishing (wavelength shift of 45 nm), and reversible luminescence response of CIPP for CH2Cl2 is realized. Crystallographic analysis suggests that the presence/removal of CH2Cl2 can affect the Cu⋯Cu distance, which is the origin of the responsive luminescence. In addition, the multiple weak interactions between CH2Cl2 and the framework afford the strong adsorption of CH2Cl2 into CIPP, which can be maintained for at least 5 minutes when exposed to air, thereby ensuring accuracy in the sensing process.
Introduction
Volatile organic compounds (VOCs) refer to organic compounds with high vapor pressure and low boiling point under ambient conditions, therefore, they have high volatility.1,2 Long-term exposures to VOCs possibly cause damage to human health, such as damage to the liver, kidneys, and nervous system; thus the detection of VOCs is of critical significance.3–5 Emitting units in porous coordination polymers (CPs) or metal–organic frameworks (MOFs) can interact with VOCs, leading to changes in the intensity or the wavelength of the luminescence. For example, by generating C–H⋯O or Cl⋯H interactions between the host and guest molecules, the luminescence detection of tetrahydrofuran (THF), N,N-dimethylformamide (DMF), dimethyl sulfoxide (DMSO), and CH2Cl2 can be realized.6–8 In addition, the process of guest-adsorption/desorption in a porous CP is usually accompanied by the structural transformation, which further exhibits changes in the conformation or the charge transfer of the luminescent chromophore.9,10
Among VOCs, CH2Cl2 is widely used as a solvent or reagent not only in laboratories but also in a wide range of industrial applications, such as the production of paint removers, herbicides, and pesticides.11 However, CH2Cl2 may cause damage to the liver and nervous system, and even potential carcinogenic effects.12,13 Several methods have been developed for the detection of CH2Cl2, such as gas chromatography (GC)-mass spectrometry (GC-MS, 5 ng L−1), gas chromatography-photo-ionization detectors (GC-PID, 1 ppm), and screen-printed electrodes (SPE, 17.3 μmol L−1).14–17 However, they display some shortcomings, such as the use of expensive instruments, tedious sample pretreatment processes, and the inability for in situ analysis. Compared to the above traditional ways, luminescence detection for VOCs based on porous CPs has unique advantages, such as fast response, visualization, and simplicity.18–23 However, in comparison with usual VOCs, CH2Cl2 has difficulty forming strong interactions with the luminescent probes because of its low polarity and rather low boiling points. Therefore, reports about the luminescence response for CH2Cl2 in porous CPs and MOFs are rare.8,24 Moreover, because of the weak interaction between CH2Cl2 and CPs, it is difficult for CH2Cl2 to directly affect the luminescent center in CPs and cause the solvatochromism/vapochromism. Generally, the reported luminescent CP sensors for CH2Cl2 with fast response are based on the emission intensity change. It is rather difficult to design and prepare luminescent CPs with both chromatic and fast responses to CH2Cl2. Compared with the color-changeable luminescence detection, the intensity-depending method is more likely to cause errors due to test conditions and methods.25–27
Cu(I)-CPs have attracted much attention because of their low toxicity, low cost, and broad photophysical behaviors.28–34 In particular, the intra-cluster Cu⋯Cu distance in copper clusters could be varied with external stimulus, resulting in changes in Cu⋯Cu interaction and corresponding emissions.35,36 Based on this principle, we recently reported a series of Cu(I)-CPs with attractive optical properties, i.e., long afterglow and multi-stimuli-responsive, properties, including thermochromism, mechanochromism, and solvatochromism/vapochromism.37–40
Herein, we report a Cu(I)-CP, [Cu2I2(PPh3)2(PYZ)] (CIPP, PPh3 = triphenylphosphine, PYZ = pyrazine), which has a flexible one-dimensional (1D) chain structure. CIPP displays different blue shifts in the luminescence after the adsorption of CH2Cl2, CHCl3, and CH3CN vapors. In order to investigate the vapochromic mechanism, CIPP with corresponding guests was also prepared. Detailed structural analyses show that compared to CIPP, the adsorption of guest molecules makes the Cu⋯Cu distances longer, resulting in luminescent blue shifts and higher emission energy. Meanwhile, CIPP exhibits fast response speed (11 s) and satisfactory reversibility. Furthermore, CIPP can retain CH2Cl2 for a long time, which is helpful for applications in the detection processes.
Results and discussion
Syntheses and characteristics
CIPP·CH2Cl2 (CIPP-D) was synthesized by the reaction of CuI, PPh3, and PYZ in a CH2Cl2 solution. After drying under vacuum, the CH2Cl2 guest in CIPP-D was removed and the red CIPP crystals were obtained, referring to the reported method of a similar structure.41 Single-crystal X-ray diffraction (SCXRD) demonstrates that CIPP belongs to the monoclinic C2/c space group. The crystallographic asymmetric unit of CIPP contains one and a half [Cu2(μ-I)2] clusters as nodes, a bridging bidentate PYZ, and three monodentate PPh3 ligands, which directly coordinate with the copper ions (Fig. 1a). In contrast to the similar structure that has been reported,42 there are two different clusters in CIPP with intra-cluster Cu⋯Cu distances of 2.7338(13) Å (Cu1⋯Cu1A) and 2.8557(16) Å (Cu2⋯Cu2), respectively (Fig. S1†). These two types of copper clusters are arranged at the ABAB intervals along the CP chain. The [Cu2(μ-I)2] clusters are interconnected by PYZ as a bridging ligand into the 1D chains (Fig. 1b). These 1D chains are stacked with each other to form the 3D structure via aromatic C–H⋯π interaction (Fig. 1c and S2†). Thermogravimetric (TG) analysis indicated that the CIPP was stable up to 115 °C, and no significant weight loss was observed before the collapse, proving that there was no guest molecule in the CIPP (Fig. S3†).
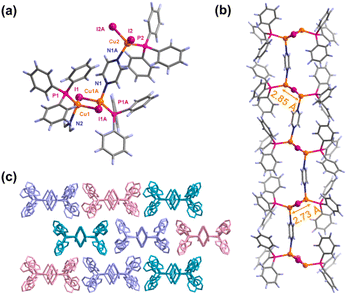 |
| Fig. 1 (a) The asymmetric unit and (b) 1D chain of CIPP. Color codes: Cu, orange; I, purple; N, indigo; P, pink; C, gray; H, light purple. (c) The stacking mode between the chains of CIPP. Different colors are employed to distinguish separate chains. Hydrogen atoms are omitted for clarity. | |
Photophysical properties
UV–vis absorption and emission spectra of CIPP are shown in Fig. 2a. It shows the strong absorption from 200 to 600 nm, and the maximum absorption wavelength (λabs) was centered at approximately 427 nm, which can be assigned to the mixed metal-to-ligand charge transfer (MLCT) and halogen-to-ligand charge transfer (XLCT) transitions.43 Because the Cu⋯Cu distance in CIPP is about 2.8 Å, the strong Cu(I)⋯Cu(I) interaction should be considered, which will affect the mixed MLCT/XLCT transition, and is usually accompanied by the longer wavelength emission. As observed under 365 nm excitation, CIPP shows orange-red luminescence with the maximum emission wavelength (λem) at 663 nm (Fig. S4†). This λem with rather low energy indicates the fairly large MLCT composition and strong Cu(I)⋯Cu(I) interaction. Meanwhile, the emissions of CIPP and CIPP-D do not change when varying the excited wavelength (260–460 nm), demonstrating the same emission center (Fig. S5†). Because the luminescence of Cu(I) clusters is reported to be sensitive to temperature, the emission spectra of CIPP at different temperatures were measured. As shown in Fig. 2b, from 80 K to 120 K, the emission of CIPP displayed both red-shifting and intensity-decreasing tendencies with increasing temperature, which agreed with the common thermal quenching (TQ) phenomenon.44 However, when the the temperature was further increased, contrastingly, the emissions of CIPP showed blue shifts with the gradually enhanced intensity (Fig. 2c). Detailedly, the emission of CIPP shifted from 710 nm to 663 nm (120–300 K), which showed a significant wavelength shift of 47 nm (0.12 eV). Although some studies have promoted that such temperature-dependent luminescence in Cu(I) complexes may be caused by the “thermally activated delayed fluorescence” mechanism,45,46 in this work, we tend to attribute this phenomenon to the slight lengthening of the Cu(I)⋯Cu(I) distance,47,48 as the SCXRD measurements at variable temperatures further confirmed it (Fig. S6 and Table S1†). The increase in the Cu(I)⋯Cu(I) distance weakens the Cu⋯Cu interaction, resulting in the luminescent blue shift. Because the luminescence blue shifts from the near-infrared to the visible region, its non-radiative transition is reduced. Thus, besides the blue shift, it also exhibits luminescence intensity enhancement at the same time.
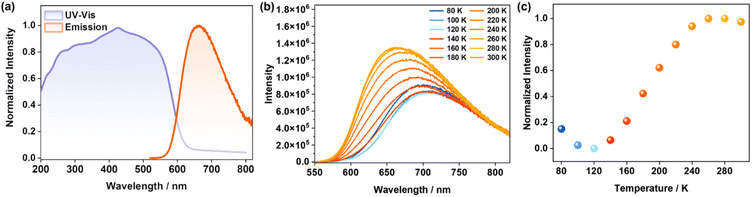 |
| Fig. 2 (a) UV–vis absorption and emission spectra (excited at 365 nm) of CIPP in air at room temperature. (b) Temperature-dependent emission spectra of CIPP, from 80 K to 300 K, excited at 365 nm. (c) A scatter plot of the integral emission intensity of CIPP at the corresponding temperature, based on (b). | |
Interestingly, the obvious vapochromism of CIPP was observed (Fig. 3). Especially, when exposed to CH2Cl2 vapor, the luminescence of CIPP exhibited a distinct blueshift (45 nm), with the emission color change from orange-red (663 nm) to orange-yellow (618 nm). Moreover, other VOC vapors, such as CH3CN and CHCl3, can also result in similar blue shifts but with smaller changes (30 and 38 nm, respectively, Fig. 3a and c, respectively). When CIPP was exposed to other types of halohydrocarbon vapors, for example, CH2Br2, CH2I2, and CHBr3, it exhibited similar luminescent vapochromism behavior, indicating that CIPP can detect the molecules with a similar size to CH2Cl2 (Fig. S7†). On the contrary, exposure to other common VOC vapors did not lead to the obvious luminescence variation for CIPP (Fig. 3b and c), which suggested the selective luminescence sensing and the potential application of halohydrocarbon and CH3CN detection.
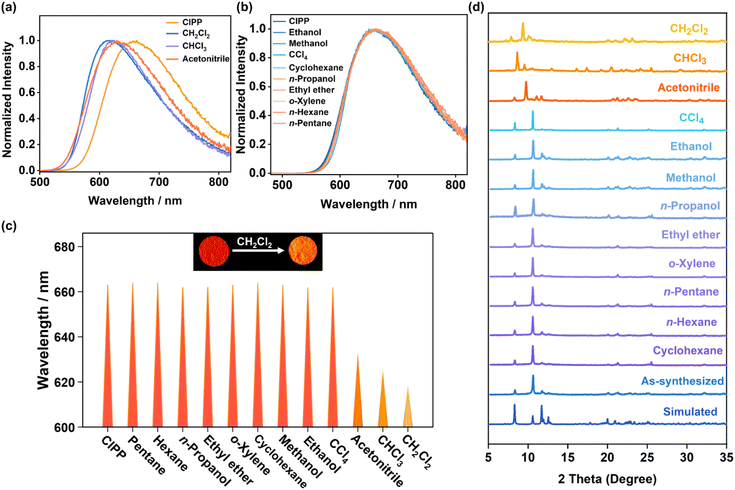 |
| Fig. 3 Emission spectra of CIPP before and after exposure in (a) acetonitrile, CH2Cl2, CHCl3 vapors, and (b) other VOCs. (c) The λem that was obtained from (a) and (b), excited at 365 nm. Insert: a photographs of CIPP before and after exposure in CH2Cl2, under 365 nm UV light. (d) PXRD patterns of CIPP before and after exposure in different VOCs. | |
Mechanism of sensing for VOCs
Powder X-ray diffraction (PXRD) patterns were measured to reveal the structural transformation during the vapochromism. As shown in Fig. 3d, The PXRD results show that the structure of CIPP does not change after exposure to the most common VOC vapors, including CCl4, ethanol, methanol, and cyclohexane, consistent with the corresponding irresponsive emission spectra shown in Fig. 3b and c. It is worth mentioning that the structure of CIPP in the gate-closing state is close-packed, making it difficult to adsorb these VOC molecules. The PXRD patterns in other VOC vapors, including CH2Cl2, CHCl3, and CH3CN, show remarkable changes (Fig. 3d). This means that these organic molecules are able to interact with CIPP by vapor diffusion, which opens the channels, illustrating the structural flexibility of CIPP. Such flexibility enables it to adsorb different molecules to form the structures in the opening state. The luminescence lifetimes of CIPP before and after exposure to VOC vapors are listed in Fig. S8,† which are all μs-scale and assigned to the phosphorescence.
To further clarify the structural transformations of CIPP in the vapochromic processes, the crystallographic structures of CIPP with different guest molecules were analyzed in detail, including CIPP-D (with CH2Cl2 guest), CIPP-C (with CHCl3 guest), and CIPP-A (with acetonitrile guest), which crystallize from the corresponding organic solvents (Fig. S9†). The λem of the as-synthesized CIPP-D is almost the same as that of the fumigated CIPP powder in CH2Cl2 vapor. The comparison of the PXRD patterns of CH2Cl2-fumigated CIPP and CIPP-D indicates that the transformation from CIPP to CIPP-D is mostly complete (Fig. S10†). Meanwhile, the luminescence sensing is still sufficient by using only the surface of the compound, as the excitation light is difficult to deeply penetrate the crystals (Fig. S11†). The completed structural transformation was observed by directly immersing CIPP in CH2Cl2 solvent (Fig. S12†). Similar transformations for CIPP after exposure to CHCl3 and CH3CN can be also observed (Fig. S13 and S14†).
As shown in Fig. 4a and b, the molecular chains in CIPP-D are similar to those in guest-free CIPP, suggesting that they are not reconstructed after absorbing CH2Cl2. For CIPP-D, the gaps between molecular chains are filled with CH2Cl2 molecules, resulting in the expansion of the structure, which exhibits the flexibility of the crystal (Fig. 4b). Each CH2Cl2 molecule in CIPP-D is enclosed by four phenyl rings of PPh3 and two PYZ ligands from the two adjacent chains (Fig. S15†). The chlorine atoms of the CH2Cl2 molecule point toward the pyrazine ligands of two adjacent chains. The dihedral angle between the pyrazine ligands of the two adjacent chains is ca. 25° and creates a void to accommodate a CH2Cl2 molecule (Fig. 4d). The chains of CIPP-D are also stacked by weak intermolecular interactions, such as those of CIPP (Fig. S16†). The structures in CIPP-C with CHCl3 and CIPP-A with CH3CN are similar with those of CIPP-D (Fig. S17†). The CH2Cl2 molecules are ordered in the crystal structure and stabilized by multi-weak interactions with PYZ and PPh3 ligands in the lattice (Fig. 4d and S18†). The closest distance between Cl atoms of CH2Cl2 and H atoms of triphenylphosphine is 3.08 Å, which shows the large tendency of Cl⋯H hydrophobic interaction. In addition, the closest C–H⋯π distance between CH2Cl2 and the aromatic ring is only 3.56 Å. In order to analyze the proportion of multi-weak interactions, Hirshfeld surfaces analysis was used (Fig. S19†).49,50 The 2D fingerprint plots illustrated that the weak host–guest Cl⋯H interactions occupied a higher fraction (58.5%). The theoretical calculation demonstrated that the total binding energy between CH2Cl2 and CIPP framework is 85 kJ mol−1 (Table S2†). Meanwhile, the adjacent inter-chain distance in CIPP-D (11.44 Å) is markedly longer compared with that in CIPP (10.60 Å), due to the insertion of CH2Cl2 (Fig. 4c and d). Furthermore, the average occupancy area per chain for CIPP-D (31.2 Å2) is also larger than that for CIPP (27.9 Å2). Similarly, there is also a difference in the average occupancy areas of the molecular chains for CIPP-C (35.8 Å2 per chain) and CIPP-A (30.3 Å2 per chain), which are mainly dependent on the sizes of the guest molecules (Fig. S20†).
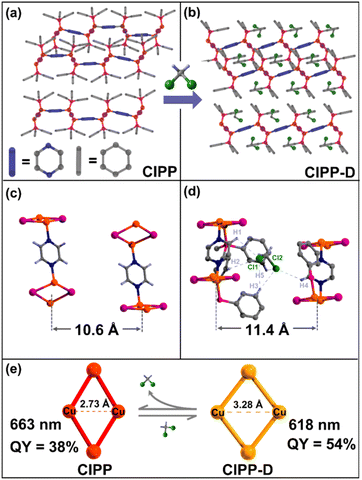 |
| Fig. 4 Molecular chains stacking in (a) CIPP and (b) CIPP-D viewed along the b-axis, the purple cylinders represent the PYZ ligands and the gray ones are for the phenyl rings of PPh3. The distance between adjacent molecular chains in (c) CIPP and (d) CIPP-D. Interactions in CIPP-D: Cl1⋯H1, 3.33 Å; Cl1⋯H2, 3.26 Å; Cl1⋯H3, 3.15 Å; Cl2⋯H3, 3.35 Å; Cl2⋯H4, 3.33 Å; Cl2⋯H5, 3.40 Å. (e) Cu⋯Cu distances of the [Cu2(μ-I)2] clusters in CIPP and CIPP-D. Color codes: Cu, orange; I, purple; Cl, green; N, indigo; P, pink; C, gray; H, light purple. | |
The intra-cluster Cu⋯Cu distance in CIPP is 2.73 Å, indicating a strong Cu⋯Cu interaction that leads to greatly reduced emission energy (1.87 eV). While for CIPP-D, with the lengthening of the inter-chain distance, [Cu2(μ-I)2] cluster stretches and the Cu⋯Cu distances increased to 3.28 Å, and 3.2378(8) Å for CIPP-A and 3.1479(7) Å for CIPP-C (Fig. S21†). For this reason, the intra-cluster Cu⋯Cu interaction in CIPP-D almost disappeared, thus it showed an obvious blueshift emission compared to that of CIPP (Fig. 4e). In addition, the increase of the Cu⋯Cu distance may enhance luminescence efficiency, as the photoluminescence quantum yields (PLQYs) are 38% for CIPP, and 54% for CIPP-D (Fig. 4e). This conclusion also explains why CIPP exhibits thermal luminescence enhancing phenomenon mentioned above, as the Cu⋯Cu distance in CIPP can slightly increase the warming process (120–298 K, Fig. S6†).
CH2Cl2 sensing properties
As CIPP shows the best sensing performance to CH2Cl2 with the greatest change of the luminescence among the VOCs, its responding performance to CH2Cl2 was analyzed in detail. First, when CIPP was exposed to the atmosphere of CH2Cl2 at different concentrations, it displayed a regularly changing tendency. As shown in Fig. 5, with the increase in CH2Cl2 concentration, the emission of CIPP gradually shifted from 663 nm to 618 nm. Moreover, a significant blueshift occurred at the CH2Cl2 concentration of 1 g L−1 (Fig. 5a), suggesting that the channel begins to open at this concentration.
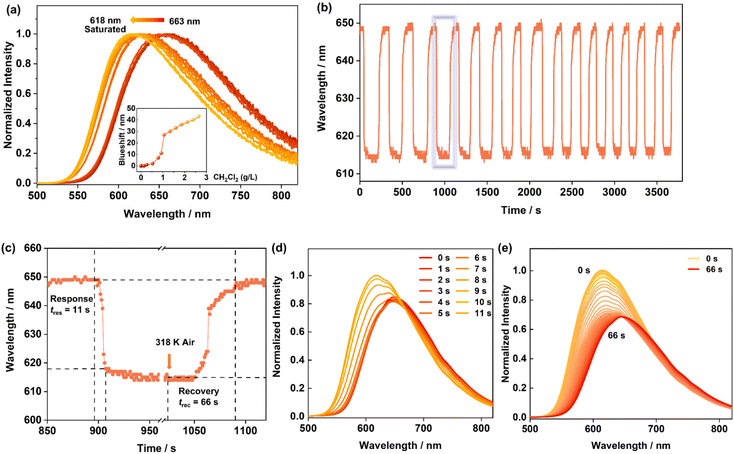 |
| Fig. 5 (a) Emission spectra of CIPP in the atmosphere with various CH2Cl2 concentrations (0, 0.133, 0.265, 0.53, 0.795, 0.928, 1.06, 1.33, 1.59, 1.86, 2.12, 2.39, 2.65 g L−1, from right to left) at room temperature. (b) The cyclic luminescence responses of CIPP under the alternation between saturated CH2Cl2 vapor and hot air (318 K). The vertical coordinate is the λem of the spectra per second. (c) Response curves in the range of purple square in (b) with a time range from 896 s to 907 s. (d) Time-dependent emission spectra of CIPP in saturated CH2Cl2 vapor, from 0 s to 11 s. (e) Time-dependent emission spectra of CH2Cl2-absorbed CIPP sample at 318 K, recorded once per second. | |
The sensing speed and reversibility of CIPP were also tested by in situ experiments. The real-time continuous emission spectra were recorded using a charge-coupled device (CCD). CH2Cl2 molecules absorbed in CIPP could be removed by heating at 318 K, which was proved by both PXRD patterns and emission spectra (Fig. S22 and S23†). Therefore, to test the reversibility, CIPP powder was alternately placed in the atmosphere of saturated CH2Cl2 vapors at room temperature, and warm air without CH2Cl2 at 318 K. It should be noted that CIPP still showed a similar response to CH2Cl2 vapors after 16 cycles and the structure was maintained even after cyclic responses (Fig. 5b and S22†). As shown in Fig. 5d, in the saturated CH2Cl2 vapor, the luminescence of CIPP rapidly blue shifts within 11 s, suggesting a rather fast response. After the stabilization of the luminescence spectra, which suggested the saturation of CH2Cl2 absorption, CH2Cl2 from the sample was removed by blowing heated air (318 K), and the recovery time (back to the luminescence of CIPP phase) was about 66 s (Fig. 5e). The regenerated CIPP-D was able to maintain the emission without significant shift for about 5 min at room temperature (Fig. S24†), indicating that CIPP showed a relatively strong absorption of CH2Cl2, thus reducing the luminescence change in the measurement and improving the accuracy. In addition to CH2Cl2, the response time for CHCl3 and CH3CN vapors were also measured. For CHCl3, the blue shift of λem was completed within 3 min (Fig. S25†). Such wavelength shift is smaller, and the response time is longer compared with that of the CH2Cl2 vapor. While for CH3CN, the luminescence blueshift was completed within 60 s (Fig. S26†). The faster response speed for CH2Cl2 may be attributed to the rather high saturation vapor pressure of CH2Cl2 (46.5 kPa at 293 K), compared with those of CHCl3 (21.28 kPa at 293 K) and CH3CN (9.33 kPa at 293 K).51,52
Conclusions
A Cu(I)-CP named CIPP was synthesized and characterized extensively. CIPP displayed luminescence vapochromic response to CH2Cl2, CHCl3, and CH3CN vapors. Benefitting from the flexibility of CIPP, the intra-cluster Cu⋯Cu distance varies when it absorbs guest molecules and transforms into the gate-opening state. Especially, the interaction with CH2Cl2 allows CIPP to display a remarkable luminescence wavelength for the blue shift (45 nm). Moreover, CIPP exhibits fast luminescence color variation (ca. 11 s) and excellent reversibility for sensing CH2Cl2. Due to the multi-weak interactions, CIPP can hold CH2Cl2 for 5 minutes at room temperature in air.
Experimental
All the chemicals were obtained from commercial sources and used as received without further purification unless otherwise noted. Powder X-ray diffraction (PXRD) patterns were recorded on a Rigaku Mini diffractometer with Cu-Kα (λ = 1.54184 Å) radiation. UV-vis absorption spectra were recorded on a Shimadzu UV-3600i Plus UV-VIS-NIR absorption spectrometer. Thermogravimetric (TG) analyses were performed on a METTLER TOLEDO TGA/DSC 3+ instrument with a ramping rate of 10.0 °C min−1 under a nitrogen atmosphere. Elemental analysis (C, H, and N) was performed on an Elementar Vario EL Cube.
Preparation
For CIPP-D and CIPP: PYZ (16 mg, 0.2 mmol) and PPh3 (105 mg, 0.40 mmol) were completely dissolved in dichloromethane (5 mL), followed by the addition of CuI (76 mg, 0.4 mmol) at room temperature. The orange CIPP-D crystals were formed from the transparent liquid after about 7 hours. The crystals were filtered and washed with CH2Cl2, then dried under vacuum to obtain orange-red CIPP crystals (yield = 60%). Elemental analysis calcd for C20H17CuINP (CIPP): C, 48.75; H, 3.48; N, 2.84. Found: C, 48.80; H, 3.83; N, 2.64.
For CIPP-A: CIPP-A was synthesized by a similar method to that of CIPP-D by replacing the CH2Cl2 solvent with the CH3CN solvent (yield = 54%).
For CIPP-C: PYZ (32 mg, 0.4 mmol) and PPh3 (105 mg, 0.40 mmol) were completely dissolved in CHCl3 (5 mL) at 278 K, followed by the addition of CuI (76 mg, 0.4 mmol). Then, the solution was kept at 278 K for about one day, and orange CIPP-C crystals were observed. The crystals were filtered and washed with cold CHCl3 (yield = 47%). Among these, CIPP-A was reported in the previous literature.36
Single-crystal X-ray diffraction
Diffraction data were collected on a Rigaku XtaLAB single-crystal diffractometer by using Cu-Kα radiation (λ = 1.54184 Å). The structures were solved using the direct methods and refined with the full-matrix least-squares method on F2 using the SHELXTL package.53 Anisotropic thermal parameters were used to refine all non-hydrogen atoms. All hydrogen atoms were generated geometrically. Crystallographic data and details of data collection and refinements are summarized in Table S1.† CCDC 2258386 (CIPP), 2258387 (CIPP-D), 2258388 (CIPP-C), and 2258389 (CIPP-150K) contain supplementary crystallographic data for this work.†
Photoluminescence measurement
Photoluminescence measurements were performed on an Edinburgh FLS1000 fluorescence spectrometer. The emission spectra were recorded on the spectrometer equipped with a continuous Xe lamp. The luminescence decay experiments were performed on the same spectrometer equipped with a variable pulsed laser (VPL) at 375 ± 10 nm as the excitation source. The temperature was controlled using an Oxford temperature controller. The photoluminescence quantum yields (PLQYs) were measured on the same spectrometer in the integrating sphere.
The in situ experiment was recorded using an Ocean QE Pro charge-coupled device (CCD) equipped with a 365 nm LED. Real-time sensing of CH2Cl2 was realized using CH2Cl2 liquid to provide the saturated vapor under ambient conditions. After removing CH2Cl2 using hot air at 318 K, CIPP was cooled to room temperature by blowing normal air (detected by thermometer).
Conflicts of interest
There are no conflicts to declare.
Acknowledgements
We gratefully acknowledge the financial support of the National Natural Science Foundation of China (22101211, 21901189), Municipal Science and Technology Bureau (Jiangke 2021-76), MOE Key Laboratory of Bioinorganic and Synthetic Chemistry (BISC2022A04), Scientific Research Ability Improvement Project of Key Discipline Construction from the Education Department of Guangdong Province (2022ZDJS027), and the NSF of Guangdong Province (2017A030310258).
References
- Y. Shen, A. Tissot and C. Serre, Recent progress on MOF-based optical sensors for VOC sensing, Chem. Sci., 2022, 13, 13978–14007 RSC.
- H. Y. Li, S. N. Zhao, S. Q. Zang and J. Li, Functional metal–organic frameworks as effective sensors of gases and volatile compounds, Chem. Soc. Rev., 2020, 49, 6364–6401 RSC.
- S. Joshi, The Sick Building Syndrome, Indian J. Occup. Health, 2008, 12, 61–64 Search PubMed.
- H. Ibrahim, S. Moru, P. Schnable and L. Dong, Wearable Plant Sensor for In Situ Monitoring of Volatile Organic Compound Emissions from Crops, ACS Sens., 2022, 7, 2293–2302 CrossRef CAS PubMed.
- D. S. Li, B. Y. Zhu, K. Pang, Q. Zhang, M. J. Qu, W. T. Liu, Y. Q. Fu and J. Xie, Virtual Sensor Array Based on Piezoelectric Cantilever Resonator for Identification of Volatile Organic Compounds, ACS Sens., 2022, 7, 1555–1563 CrossRef CAS PubMed.
- J. J. Pang, Z. Q. Yao, K. Zhang, Q. W. Li, Z. X. Fu, R. Zheng, W. Li, J. Xu and X. H. Bu, Real-Time In Situ Volatile Organic Compound Sensing by a Dual-Emissive Polynuclear Ln-MOF with Pronounced Ln(III) Luminescence Response, Angew. Chem., Int. Ed., 2023, 62, e202217456 CrossRef CAS PubMed.
- Z. Q. Yao, K. Wang, R. Liu, Y. J. Yuan, J. J. Pang, Q. W. Li, T. Y. Shao, Z. G. Li, R. Feng, B. Zou, W. Li, J. Xu and X. H. Bu, Dynamic Full-Color Tuning of Organic Chromophore in a Multi-Stimuli-Responsive 2D Flexible MOF, Angew. Chem., Int. Ed., 2022, 61, e202202073 CrossRef CAS PubMed.
- C. Y. Liu, X. R. Chen, H. X. Chen, Z. Niu, H. Hirao, P. Braunstein and J. P. Lang, Ultrafast Luminescent Light-Up Guest Detection Based on the Lock of the Host Molecular Vibration, J. Am. Chem. Soc., 2020, 142, 6690–6697 CrossRef CAS PubMed.
- X. Y. Dong, H. L. Huang, J. Y. Wang, H. Y. Li and S. Q. Zang, A Flexible Fluorescent SCC-MOF for Switchable Molecule Identification and Temperature Display, Chem. Mater., 2018, 30, 2160–2167 CrossRef CAS.
- L. Chen, J. W. Ye, H. P. Wang, M. Pan, S. Y. Yin, Z. W. Wei, L. Y. Zhang, K. Wu, Y. N. Fan and C. Y. Su, Ultrafast water sensing and thermal imaging by a metal–organic framework with switchable luminescence, Nat. Commun., 2017, 8, 15985 CrossRef CAS PubMed.
- World Health Organization and International Agency for Research on Cancer, Dichloromethane, IARC Monogr. Eval. Carcinog. Risks Hum., 1999, 71(Part 1), 251–315 Search PubMed.
- A. Hoang, K. Fagan, D. L. Cannon, S. D. G. Rayasam, R. Harrison, D. Shusterman and V. Singla, Assessment of Methylene Chloride-Related Fatalities in the United States, 1980-2018, JAMA Intern. Med., 2021, 181, 797–805 CrossRef CAS PubMed.
- P. M. Schlosser, A. S. Bale, C. F. Gibbons, A. Wilkins and G. S. Cooper, Human health effects of dichloromethane: key findings and scientific issues, Environ. Health Perspect., 2015, 123, 114–119 CrossRef PubMed.
- I. Ueta, S. Kamei and Y. Saito, Needle extraction device for rapid and quantitative gas chromatographic determination of volatile chlorinated hydrocarbons and benzene in soil, J. Chromatogr. A, 2022, 1685, 463586 CrossRef CAS PubMed.
- T. Sakai, Y. Morita and C. Wakui, Biological monitoring of workers exposed to dichloromethane, using head-space gas chromatography, J. Chromatogr. B: Anal. Technol. Biomed. Life Sci., 2002, 778, 245–250 CrossRef CAS PubMed.
- E.-B. Kim, Abdullah, S. Ameen, M. S. Akhtar and H. S. Shin, Environment-friendly and highly sensitive dichloromethane chemical sensor fabricated with ZnO nanopyramids-modified electrode, J. Taiwan Inst. Chem. Eng., 2019, 102, 143–152 CrossRef.
- D. Poli, P. Manini, R. Andreoli, I. Franchini and A. Mutti, Determination of dichloromethane, trichloroethylene and perchloroethylene in urine samples by headspace solid phase microextraction gas chromatography-mass spectrometry, J. Chromatogr. B: Anal. Technol. Biomed. Life Sci., 2005, 820, 95–102 CrossRef CAS PubMed.
- J. R. Askim, M. Mahmoudi and K. S. Suslick, Optical sensor arrays for chemical sensing: the optoelectronic nose, Chem. Soc. Rev., 2013, 42, 8649–8682 RSC.
- F. P. Yang, Q. T. He, H. Jiang, Z. Li, W. Chen, R. L. Chen, X. Y. Tang, Y. P. Cai and X. J. Hong, Rapid and Specific Enhanced Luminescent Switch of Aniline Gas by MOFs Assembled from a Planar Binuclear Cadmium(II) Metalloligand, Inorg. Chem., 2022, 61, 10844–10851 CrossRef CAS PubMed.
- I. S. Krytchankou, I. O. Koshevoy, V. V. Gurzhiy, V. A. Pomogaev and S. P. Tunik, Luminescence Solvato- and Vapochromism of Alkynyl-Phosphine Copper Clusters, Inorg. Chem., 2015, 54, 8288–8297 CrossRef CAS PubMed.
- R. W. Huang, Y. S. Wei, X. Y. Dong, X. H. Wu, C. X. Du, S. Q. Zang and T. C. W. Mak, Hypersensitive dual-function luminescence switching of a silver-chalcogenolate cluster-based metal–organic framework, Nat. Chem., 2017, 9, 689–697 CrossRef CAS PubMed.
- W. M. He, Z. Zhou, Z. Han, S. Li, Z. Zhou, L. F. Ma and S. Q. Zang, Ultrafast Size Expansion and Turn-On Luminescence of Atomically Precise Silver Clusters by Hydrogen Sulfide, Angew. Chem., Int. Ed., 2021, 60, 8505–8509 CrossRef CAS PubMed.
- Y. Yu, J. P. Ma, C. W. Zhao, J. Yang, X. M. Zhang, Q. K. Liu and Y. B. Dong, Copper(I) Metal–Organic Framework: Visual Sensor for Detecting Small Polar Aliphatic Volatile Organic Compounds, Inorg. Chem., 2015, 54, 11590–11592 CrossRef CAS PubMed.
- L. Guan, Z. Jiang, Y. Cui, Y. Yang, D. Yang and G. Qian, An MOF-Based Luminescent Sensor Array for Pattern Recognition and Quantification of Metal Ions, Adv. Opt. Mater., 2021, 9, 2002180 CrossRef CAS.
- Z. Han, Y. Si, X. Y. Dong, J. H. Hu, C. Zhang, X. H. Zhao, J. W. Yuan, Y. Wang and S. Q. Zang, Smart Reversible Transformations between Chiral Superstructures of Copper Clusters for Optical and Chiroptical Switching, J. Am. Chem. Soc., 2023, 145, 6166–6176 CrossRef CAS PubMed.
- W. Cao, T. Xia, Y. Cui, Y. Yu and G. Qian, Lanthanide metal–organic frameworks with nitrogen functional sites for the highly selective and sensitive detection of NADPH, Chem. Commun., 2020, 56, 10851–10854 RSC.
- J. Wang, D. Li, Y. Ye, Y. Qiu, J. Liu, L. Huang, B. Liang and B. Chen, A Fluorescent Metal–Organic Framework for Food Real-Time Visual Monitoring, Adv. Mater., 2021, 33, 2008020 CrossRef CAS PubMed.
- J. Troyano, F. Zamora and S. Delgado, Copper(I)–iodide cluster structures as functional and processable platform materials, Chem. Soc. Rev., 2021, 50, 4606–4628 RSC.
- X. Hei, W. Liu, K. Zhu, S. J. Teat, S. Jensen, M. Li, D. M. O'Carroll, K. Wei, K. Tan, M. Cotlet, T. Thonhauser and J. Li, Blending Ionic and Coordinate Bonds in Hybrid Semiconductor Materials: A General Approach toward Robust and Solution-Processable Covalent/Coordinate Network Structures, J. Am. Chem. Soc., 2020, 142, 4242–4253 CrossRef CAS PubMed.
- J. P. Zobel, A. M. Wernbacher and L. González, Efficient Reverse Intersystem Crossing in Carbene-Copper-Amide TADF Emitters via an Intermediate Triplet State, Angew. Chem., Int. Ed., 2023, 62, e202217620 CrossRef CAS PubMed.
- J. J. Wang, C. Chen, W. G. Chen, J. S. Yao, J. N. Yang, K. H. Wang, Y. C. Yin, M. M. Yao, L. Z. Feng, C. Ma, F. J. Fan and H. B. Yao, Highly Luminescent Copper Iodide Cluster Based Inks with Photoluminescence Quantum Efficiency Exceeding 98%, J. Am. Chem. Soc., 2020, 142, 3686–3690 CrossRef CAS PubMed.
- B. L. Han, Z. Liu, L. Feng, Z. Wang, R. K. Gupta, C. M. Aikens, C. H. Tung and D. Sun, Polymorphism in Atomically Precise Cu23 Nanocluster Incorporating Tetrahedral [Cu4]0 Kernel, J. Am. Chem. Soc., 2020, 142, 5834–5841 CrossRef CAS PubMed.
- P. P. Sun, B. L. Han, H. G. Li, C. K. Zhang, X. Xin, J. M. Dou, Z. Y. Gao and D. Sun, Real-Time Fluorescent Monitoring of Kinetically Controlled Supramolecular Self-Assembly of Atom-Precise Cu8 Nanocluster, Angew. Chem., Int. Ed., 2022, 61, e202200180 CrossRef CAS PubMed.
- C. Zhang, Z. Wang, W. D. Si, L. Wang, J. M. Dou, Z. Y. Gao, C. H. Tung and D. Sun, Solvent-Induced Isomeric Cu13 Nanoclusters: Chlorine to Copper Charge Transfer Boosting Molecular Oxygen Activation in Sulfide Selective Oxidation, ACS Nano, 2022, 16, 9598–9607 CrossRef CAS PubMed.
- J. Conesa-Egea, F. Zamora and P. Amo-Ochoa, Perspectives of the smart Cu-Iodine coordination polymers: A portage to the world of new nanomaterials and composites, Coord. Chem. Rev., 2019, 381, 65–78 CrossRef CAS.
- Q. Benito, X. F. Le Goff, S. Maron, A. Fargues, A. Garcia, C. Martineau, F. Taulelle, S. Kahlal, T. Gacoin, J. P. Boilot and S. Perruchas, Polymorphic Copper Iodide Clusters: Insights into the Mechanochromic Luminescence Properties, J. Am. Chem. Soc., 2014, 136, 11311–11320 CrossRef CAS PubMed.
- L. Chen, X. B. Dong, H. Y. Liao, W. J. Zhang, Z. W. Mo, H. P. Wang, J. W. Ye and X. M. Chen, Long-Range Rigidity Induced Ultralong Cluster-Centered Phosphorescence, Chem. Mater., 2022, 34, 9182–9189 CrossRef CAS.
- L. Chen, X. B. Dong, Z. W. Mo, H. P. Wang, J. W. Ye, K. Zhang and X. M. Chen, Efficient Restraint of Intra-Cluster Aggregation-Caused Quenching Effect Lighting Room-Temperature Photoluminescence, Adv. Opt. Mater., 2021, 9, 2100757 CrossRef CAS.
- W. T. Chen, L. Chen, Z. Y. Liang, Z. W. Mo, J. W. Ye and X. M. Chen, Multiple Flexibilities Trigger Luminescent Piezochromism of Closely Packed Cu(I) Coordination Polymers, Adv. Opt. Mater., 2023, 11, 2202771 CrossRef CAS.
- W. T. Chen, C. H. Li, W. Q. Zhou, J. T. Huang, J. W. Ye and L. Chen, A One-Dimensional Cu(I) Coordination Polymer with Optical Sensing of Oxygen and Temperature, Inorganics, 2022, 10, 253 CrossRef CAS.
- H. Araki, K. Tsuge, Y. Sasaki, S. Ishizaka and N. Kitamura, Luminescence ranging from red to blue: a series of copper(I)-halide complexes having rhombic {Cu2(μ-X)2} (X = Br and I) units with N-heteroaromatic ligands, Inorg. Chem., 2005, 44, 9667–9675 CrossRef CAS PubMed.
- M. Henary, J. L. Wootton, S. I. Khan and J. I. Zink, Structure and Assignment of the Luminescence of a New Mixed-Ligand Copper(I) Polymer, Inorg. Chem., 1997, 36, 796–801 CrossRef CAS.
- S. Perruchas, C. Tard, X. F. Le Goff, A. Fargues, A. Garcia, S. Kahlal, J. Y. Saillard, T. Gacoin and J. P. Boilot, Thermochromic Luminescence of Copper Iodide Clusters: The Case of Phosphine Ligands, Inorg. Chem., 2011, 50, 10682–10692 CrossRef CAS PubMed.
- Y. H. Kim, P. Arunkumar, B. Y. Kim, S. Unithrattil, E. Kim, S. H. Moon, J. Y. Hyun, K. H. Kim, D. Lee, J. S. Lee and W. B. Im, A zero-thermal-quenching phosphor, Nat. Mater., 2017, 16, 543–550 CrossRef CAS PubMed.
- H. Yersin, R. Czerwieniec, M. Z. Shafikov and A. F. Suleymanova, TADF Material Design: Photophysical Background and Case Studies Focusing on CuIand AgIComplexes, ChemPhysChem, 2017, 18, 3508–3535 CrossRef CAS PubMed.
- M. El Sayed Moussa, S. Evariste, H. L. Wong, L. Le Bras, C. Roiland, L. Le Polles, B. Le Guennic, K. Costuas, V. W. W. Yam and C. Lescop, A solid state highly emissive Cu(i) metallacycle: promotion of cuprophilic interactions at the excited states, Chem. Commun., 2016, 52, 11370–11373 RSC.
- T. H. Kim, Y. W. Shin, J. H. Jung, J. S. Kim and J. Kim, Crystal-to-Crystal Transformation between Three CuI Coordination Polymers and Structural Evidence for Luminescence Thermochromism, Angew. Chem., Int. Ed., 2008, 47, 685–688 CrossRef CAS PubMed.
- D. Sun, S. Yuan, H. Wang, H. F. Lu, S. Y. Feng and D. F. Sun, Luminescence thermochromism of two entangled copper-iodide networks with a large temperature-dependent emission shift, Chem. Commun., 2013, 49, 6152–6154 RSC.
- F. F. Gao, H. Song, Z. G. Li, Y. Qin, X. Li, Z. Q. Yao, J. H. Fan, X. Wu, W. Li and X. H. Bu, Pressure-Tuned Multicolor Emission of 2D Lead Halide Perovskites with Ultrahigh Color Purity, Angew. Chem., Int. Ed., 2023, 62, e202218675 CrossRef CAS PubMed.
- P. R. Spackman, M. J. Turner, J. J. McKinnon, S. K. Wolff, D. J. Grimwood, D. Jayatilaka and M. A. Spackman, CrystalExplorer: a program for Hirshfeld surface analysis, visualization and quantitative analysis of molecular crystals, J. Appl. Crystallogr., 2021, 54, 1006–1011 CrossRef CAS PubMed.
- Y. Tian, H. Ding, Q. Jiao and Y. Shi, Influence of Solvents on the Formation of Honeycomb Films by Water Droplets Templating, Macromol. Chem. Phys., 2006, 207, 545–553 CrossRef CAS.
- N. P. Lebedeva and L. Boon-Brett, Considerations on the Chemical Toxicity of Contemporary Li-Ion Battery Electrolytes and Their Components, J. Electrochem. Soc., 2016, 163, A821–A830 CrossRef CAS.
- G. M. Sheldrick, SHELXT– Integrated space-group and crystal-structure determination, Acta Crystallogr., Sect. A: Found. Adv., 2015, 71, 3–8 CrossRef PubMed.
|
This journal is © the Partner Organisations 2023 |
Click here to see how this site uses Cookies. View our privacy policy here.