DOI:
10.1039/D2QI02631G
(Research Article)
Inorg. Chem. Front., 2023,
10, 1721-1730
Topological control of metal–organic frameworks toward highly sensitive and selective detection of chromate and dichromate†
Received
10th December 2022
, Accepted 30th December 2022
First published on 4th January 2023
Abstract
Luminescent metal–organic frameworks (LMOFs) have been extensively used as sensitive and selective sensors for carcinogenic chromium(VI) oxyanions. However, the correlation between the MOF structure and the Cr(VI) sensing efficacy remains underexplored, hindering the rational design of MOFs for Cr(VI) sensing. Herein, we overcome this challenge by judiciously selecting the rarely touched thorium cations and a tetraphenylethene-based ligand for building two novel thorium-based MOFs, Th-BCTPE-1 and Th-BCTPE-2. Despite being built from identical precursors, Th-BCTPE-1 shows more sensitive luminescence quenching responses to CrO42− and Cr2O72− than Th-BCTPE-2, producing the second highest quenching constants of CrO42− among all LMOF-based Cr(VI) sensors. We further decoupled the influences of different structural variables and the corresponding physiochemical properties, including porosity, BET surface area, photoluminescence quantum yield, and adsorption capacity, showing that the sensing efficacy of Cr(VI) oxyanions is more relevant to the adsorption capacity and the degree of the inner filter effect than the rest of the variables in this system.
Introduction
Chromates and dichromates have been extensively used to improve the corrosion resistance of active metals via the formation of stable chromate conversion coating.1 Moreover, Cr(VI) oxyanions are essential in advanced nuclear cycles since chromate and dichromate have been used as corrosion inhibitors in cooling water for nuclear reactors.2,3 Cooling water with an approximate Cr(VI) concentration of 700 μg kg−1 is discharged from the reactors to natural water bodies after cooling in retention basins.4 As a consequence, high-level Cr(VI) contamination (ranging up to nearly 300 mg kg−1) has been identified in the groundwater at the Hanford site and the nearby Columbia river, which imposes a potential hazard to human health due to its high carcinogenicity.4,5 Furthermore, the presence of chromate in high-level waste (HLW) leads to the formation of undesirable spinel crystals during the vitrification of nuclear waste, which increases the anticipated cost and risk of HLW vitrification.6 Hence, facile detection and the subsequent effective sequestration of chromate and dichromate from contaminated water and HLW are highly indispensable.7–11 The concentration of Cr(VI) solution can be determined colorimetrically by the diphenylcarbazide method.4 However, this technology suffers from low sensitivity (limit of detection of 0.5 mg kg−1). Instrumental techniques including inductively coupled plasma atomic emission spectroscopy (ICP-AES) and inductively coupled plasma mass spectrometry (ICP-MS) are advantageous in terms of limit of detection (LOD)12 but they are unable to distinguish the toxic Cr(VI) from the benign Cr(III) ion, the latter of which is the dominant natural background species of chromium in sediments.
As a class of porous inorganic–organic hybrid materials, metal–organic frameworks (MOFs) are scientifically compelling and functionally evolving with considerable advances made in various fields including separation, sensing, and catalysis.13–18 Incorporating luminescent metal nodes and/or organic linkers within MOFs engenders the sensing capacities of MOFs in a turn-off, turn-on, or fluorochromic manner, making them suitable for chromate/dichromate sensing.19–22 Furthermore, the highly tunable compositions, structures, and pore functionalities of MOFs give rise to a series of chromate/dichromate sensors with high sensitivity and excellent selectivity.23–28 Although significant progress in the development of MOFs for Cr(VI) detection has been achieved, understanding the relationship between the MOF structure and the sensing efficacy remains in its infancy, which hinders the rational design of MOFs for Cr(VI) sensing. The study by Sun and coworkers indicated that modifying the pore shape and volume of MOFs via altering the linker shape have a notable influence on the detection sensitivity of dichromate.25 Moreover, a mixed-ligand strategy gave rise to three cadmium coordination polymers, of which the one-dimensional polymer exhibited the best sensing performance.29 However, both the organic linkers and the topologies of MOFs are changed in these systems, rendering the decoupling of the influence of each variable on the luminescence properties rather difficult. Therefore, an outstanding challenge in obtaining the structure–property correlation is to alter the underlying topologies of MOFs without introducing additional variables.
We have recently undertaken a study on the control of the polymorphism of MOFs by utilizing thorium-based metal–organic frameworks (Th-MOFs) as a platform.30 One of the key merits of Th-MOFs is that the appropriate “hardness” of Th4+ can afford different secondary building units (SBUs) with a wide range of nuclearity and correspondingly diverse topologies.31–35 Besides, the Th4+ cation typically adopts a nine-coordinate capped square antiprism geometry, which provides an additional coordinating site on its capping addenda for creating new structures.36–39 This can be exemplified by a series of Th-MOFs that feature five unique topologies even though they are built from the same 2′-methyl-[1,1′:4′,1′′-terphenyl]-4,4′′-dicarboxylate (MeTPDC2) ligand.38 Such topological polymorphism of Th-MOFs facilitates a better understanding of how the structure of MOF, in isolation, influences the sensing efficacy of Cr(VI) oxyanions.
Herein, we showcase a synthetic modulation approach to obtain two topologically distinct Th-MOFs, [Th6(OH)4(O)4(H2O)6](BCTPE)6·(DMF)18·(H2O)9 (Th-BCTPE-1) and [Th6(OH)4(O)4(H2O)6](BCTPE)5(HCOO)2·(DMF)26·(H2O)32 (Th-BCTPE-2), built from the same (E)-4,4′-(1,2-diphenylethene-1,2-diyl)dibenzoate linker (BCTPE2−). As a π-electron-rich dicarboxylate ligand, H2BCTPE was used as a fluorophore to engender the luminometric sensing capacities of Th-BCTPE-1 and Th-BCTPE-2 for chromate and dichromate. Notably, the UiO-type MOF Th-BCTPE-1 features greater quenching constants (KSV) and a lower limit of detection (LOD) than Th-BCTPE-2 for both chromate and dichromate. The polymorphism of these Th-MOFs and the corresponding different sensing efficacies show the profound effect of structure on Cr(VI) sensing and shed light on the future rational design of highly efficient Cr(VI) sensors.
Results and discussion
By modifying the stoichiometry, modulator, and reaction temperature, solvothermal reactions between the identical precursors of Th(NO3)4·6H2O and (E)-4,4′-(1,2-diphenylethene-1,2-diyl)dibenzoic acid (H2BCTPE) allow for the isolation of two different Th-MOFs, Th-BCTPE-1 and Th-BCTPE-2. When trifluoroacetic acid was selected as a modulator, a reaction at 80 °C gave rise to octahedral single-crystals of Th-BCTPE-1 (Fig. S1a†). Incorporating nitric acid in the synthesis at 120 °C resulted in the crystallization of prismatic crystals of Th-BCTPE-2 (Fig. S1b†).
Single-crystal X-ray diffraction (SCXRD) study revealed that Th-BCTPE-1 adopts a typical UiO type structure with the cubic Fm
m space group and fcu topology (Table S1†). The asymmetric unit of its framework is composed of one crystallographically unique 1/8 Th4+, 1/6 μ3-OH/μ3-O, 1/8 coordinating H2O (1/48 [Th6(μ3-OH)4(μ3-O)4(H2O)6]12+), and 1/8 BCTPE2−. As shown in Fig. 1a, the secondary building unit (SBU) of Th-BCTPE-1 is built from six Th cations interconnected by four μ3-OH and four μ3-O groups and further decorated by six H2O molecules. The afforded [Th6(μ3-OH)4(μ3-O)4(H2O)6]12+ cluster is bridged by twelve BCTPE2− dicarboxylate linkers with the neighbouring clusters, generating a 12-connected SBU. Two types of voids, namely an octahedral (9.0 Å) and a tetrahedral (6.2 Å) void, were identified. These cavities are occupied by disordered DMF and H2O guest species, accounting for a total volume of 34.1% as estimated by PLATON.40
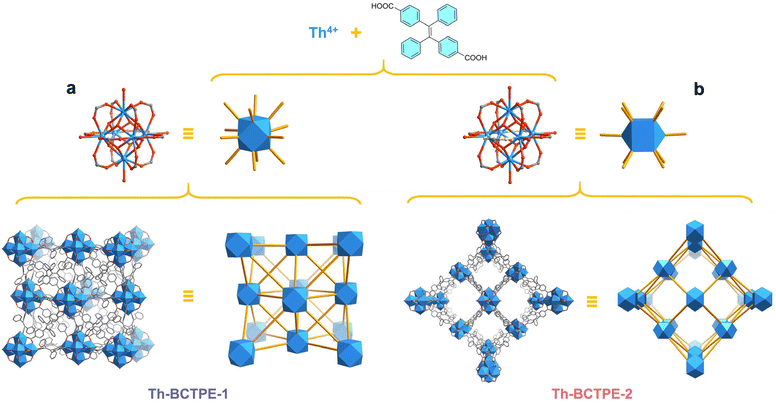 |
| Fig. 1 (a) The 12-connected Th6(μ3-OH)4(μ3-O)4(H2O)6(R-COO)12 SBU, 3D structure, and simplified topology of Th-BCTPE-1. (b) The 10-connected Th6(μ3-OH)4(μ3-O)4(H2O)6(HCOO)2(R-COO)10 SBU, 3D structure, and simplified topology of Th-BCTPE-2. Colour code: Th atoms are shown in blue, O atoms are in red, C atoms from the BCTPE2− ligands are in grey, and O atoms from the HCOO− ligand are in light yellow. | |
Th-BCTPE-2 crystallizes in the P42/mmc space group and its asymmetric unit is assembled from one 1/8 Th6(μ3-OH)4(μ3-O)4(H2O)6(HCOO)2 cluster, one 1/2 and one 1/8 BCTPE2− anion. In sharp contrast to Th-BCTPE-1, the SBU of Th-BCTPE-2 is assembled from a [Th6(μ3-OH)4(μ3-O)4(H2O)6]12+ core chelated with ten BCTPE2− ligands and two HCOO− anions (Fig. 1b). It is worth noting that HCOO− anions originated from the hydrolyzation of DMF molecules in the presence of nitric acid with extended reaction time. Unlike the bitopic BCTPE2− ligands that interconnect the neighbouring Th6 SBUs, the HCOO− groups in Th-BCTPE-2 terminate the bridging of SBUs. Thus, each Th6 SBU is connected to ten neighbouring ones, giving a final three-dimensional porous network with the bcu topology. Such a structure renders open channels of approximately 8.1 Å × 8.1 Å, that result in a much higher solvent-accessible volume of 64.7% than that of Th-BCTPE-1.
Th-BCTPE-1 and Th-BCTPE-2 can be obtained as pure phases, as confirmed by the similar powder X-ray diffraction (PXRD) patterns of the as-synthesized samples and the simulated ones (Fig. S2†). The permanent porosities of these Th-MOFs were examined by N2 adsorption/desorption isotherms measured at 77 K. Both Th-BCTPE-1 and Th-BCTPE-2 exhibited typical type I isotherms with saturated uptakes of 69.8 and 115.4 cm3 g−1, respectively, corresponding to Brunauer–Emmett–Teller (BET) surface areas of 188 and 316 m2 g−1 (Fig. 2). This trend matches well with the tendency of void volumes obtained from PLATON. The desorption branches of both materials feature slight hysteresis loops, which are characteristic of moderate interactions between N2 and the frameworks. Thermogravimetric analysis (TGA) study revealed that Th-BCTPE-1 and Th-BCTPE-2 are stable up to 490 and 510 °C, respectively (Fig. S3†). Both Th-MOFs undergo three stages of weight loss, and the first and second stages correspond to the departure of guest species including DMF and H2O. Th-BCTPE-2 features a greater amount of solvent loss (w% = 39.9%) due to its larger void space than that of Th-BCTPE-1.
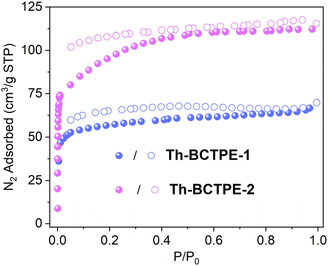 |
| Fig. 2 N2 adsorption/desorption isotherms of Th-BCTPE-1 and Th-BCTPE-2 at 77 K. | |
Despite the tremendous effort focused on the aggregation-induced emission (AIE) of tetraphenylethene, there has been only one MOF report to date integrating the BCTPE2− ligand, a dicarboxylate derivative of TPE.41 The luminescence properties of Th-BCTPE-1 and Th-BCTPE-2 were initially investigated by collecting their emission spectra. Upon 365 nm UV excitation, Th-BCTPE-1 and Th-BCTPE-2 exhibit green luminescence with emission bands centered at 495 and 500 nm, respectively, which are red-shifted in comparison with the blue emission (λmax = 467 nm) of the pure H2BCTPE ligand (Fig. S4†). The luminescence of these Th-MOFs can be ascribed to the matrix coordination-induced emission (MCIE), whereas that of H2BCTPE could result from the AIE effect.42 Moreover, the photoluminescence quantum yields (PLQYs) under ambient conditions were measured to be 50.53% and 53.07% for Th-BCTPE-1 and Th-BCTPE-2, respectively, both of which are higher than 39.19% for H2BCTPE. This observation indicates that the ordered and spatial anchoring of fluorescent BCTPE2− moieties inside rigid Th-MOFs can efficiently eliminate possible dissipative processes and turn on fluorescence.43–45 In addition, Th-BCTPE-1, Th-BCTPE-2 and H2BCTPE show bi-exponential luminescence decays with average lifetimes of 3.21, 2.45, and 2.87 ns, respectively (Fig. S5†).
Given the intense emissions and high PLQYs of both materials, we sought to investigate the potential utility of Th-BCTPE-1 and Th-BCTPE-2 for chromate and dichromate sensing. Moreover, their variety and multiplicity in terms of structures, void spaces, and surface areas make them ideal candidates for elucidating the correlations of the MOF structure with the Cr(VI) sensing efficacy. In this regard, equivalent amounts (0.16 μmol) of Th-BCTPE-1 or Th-BCTPE-2 were dispersed in 2 mL K2CrO4 or K2Cr2O7 solution with increasing concentrations. Both Th-BCTPE-1 and Th-BCTPE-2 were finely ground to make the materials homogeneously dispersed in Cr(VI) solutions and to eliminate the effect of morphology difference. As shown in Fig. 3, the emissions of both Th-MOFs were gradually quenched upon increasing the addition of CrO42− or Cr2O72− and differences in the sensing efficacy of CrO42− can be resolved between the two Th-MOFs. Specifically, the addition of 50 μM CrO42− resulted in 88.1% and 58.0% decreases in the luminescence intensities of Th-BCTPE-1 and Th-BCTPE-2, respectively, suggesting that Th-BCTPE-2 is more robust against quenching than Th-BCTPE-1 (Fig. 4a). To gain deeper insight into the quenching behaviours, the quenching constants (KSV) in the lower concentration range (0.1–1 μM) were derived from the Stern–Volmer (S–V) plots, I0/I = 1 + KSV[Q] − 1, where [Q] is the concentration of the quencher, and I0 and I are emission intensities before and after adding quenchers, respectively.46 As shown in Fig. 4b, the S–V plots for Th-BCTPE-1 and Th-BCTPE-2 are linear in the range of 0.1–1 μM and downward deviations from the linear dependence occur at higher concentrations for both materials. The KSV values were calculated to be 2.4(1) × 105 and 1.30(7) × 105 M−1 for Th-BCTPE-1 and Th-BCTPE-2, respectively, which is indicative of the higher sensing efficacy of Th-BCTPE-1 than that of Th-BCTPE-2. Notably, the KSV of Th-BCTPE-1 for CrO42− is the second highest among all MOF-based chemosensors (Table S2†).47 The limits of detection (LODs) were calculated to be 9.0 and 159 nM (3σ/k) for Th-BCTPE-1 and Th-BCTPE-2, respectively, which agree well with that of KSV (Table S3†).48
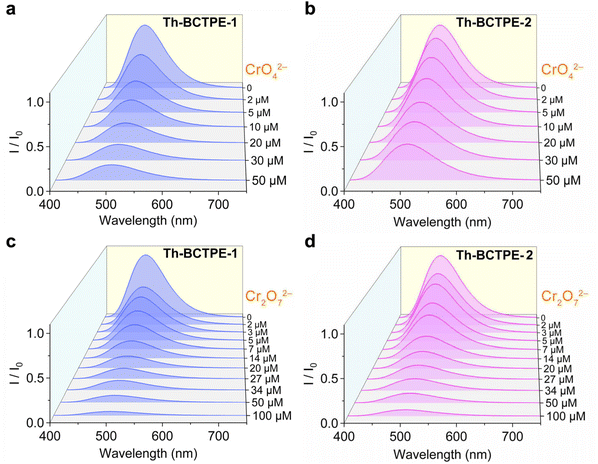 |
| Fig. 3 Normalized luminescence spectra of (a) Th-BCTPE-1 as a function of increasing CrO42− concentration, (b) Th-BCTPE-2 as a function of increasing CrO42− concentration, (c) Th-BCTPE-1 as a function of increasing Cr2O72− concentration, and (d) Th-BCTPE-2 as a function of increasing Cr2O72− concentration. | |
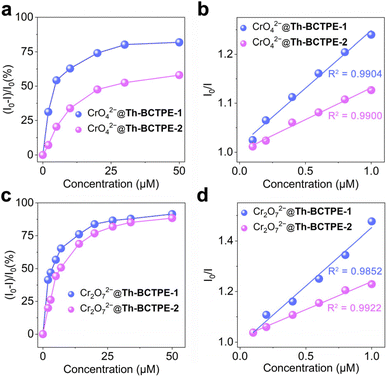 |
| Fig. 4 (a) The quenching rates of Th-BCTPE-1 and Th-BCTPE-2 as a function of CrO42− concentration. (b) Stern–Volmer plots of luminescence quenching of Th-BCTPE-1 and Th-BCTPE-2 in CrO42− solution. (c) The quenching rates of Th-BCTPE-1 and Th-BCTPE-2 as a function of Cr2O72− concentration. (d) Stern–Volmer plots of luminescence quenching of Th-BCTPE-1 and Th-BCTPE-2 in Cr2O72− solution. | |
Aligned with the CrO42− sensing, the sensing capacities of Th-BCTPE-1 and Th-BCTPE-2 for Cr2O72− were also investigated, showing that their difference in Cr2O72− sensing efficacy is relatively trivial in the concentration range of 0–50 μM (Fig. 4c). However, the quenching rate of Th-BCTPE-1 is much faster than that of Th-BCTPE-2 in a lower concentration range as shown in (Fig. S6†), suggesting that Th-BCTPE-2 is more quenching-resistant to Cr2O72− than Th-BCTPE-1. In a similar vein, the quenching constants for Cr2O72− were acquired from the S–V plots, showing a higher KSV (4.6(3) × 105 M−1) of Th-BCTPE-1 than 2.222(9) × 105 M−1 of Th-BCTPE-2. Th-BCTPE-1 again exhibited a higher sensing efficacy for Cr2O72− than Th-BCTPE-2 (Fig. 4d). Moreover, the LODs for Cr2O72− were derived from the linear regression analysis and calculated to be 4.6 and 94 nM for Th-BCTPE-1 and Th-BCTPE-2, respectively, whose trend agrees well with those for CrO42−.
Besides the quenching efficacies, the adsorption capacities of Th-BCTPE-1 and Th-BCTPE-2 for Cr(VI) oxyanions were also comparatively investigated. Scanning electron microscopy and energy dispersive X-ray spectroscopy (SEM-EDS) revealed that Cr was homogeneously dispersed on the surface of CrO42−- and Cr2O72−-soaked crystals (Fig. S7†). Moreover, the sorption isotherms indicated that both Th-BCTPE-1 and Th-BCTPE-2 can capture CrO42− and Cr2O72− anions. The uptake of anionic CrO42− and Cr2O72− by neutral Th-BCTPE-1 and Th-BCTPE-2 could be largely attributed to the electrostatic interactions between cationic SBUs and anions.38,49,50 The sorption isotherms can be better fitted with the Langmuir model than the Freundlich model (Table S4†). Notably, Th-BCTPE-1 featured higher maximum uptake capacities of CrO42− and Cr2O72− (1.58 and 1.05 mol mol−1) than those of Th-BCTPE-2 (1.22 and 0.93 mol mol−1) (Fig. 5a and b). This observation appears to be counterintuitive at first glance since Th-BCTPE-2 has a greater void volume and a larger BET surface area than those of Th-BCTPE-1. However, a recent study by us revealed that the high porosity of MOFs cannot guarantee high adsorption capacity.51 Since Th-BCTPE-1 incorporates fewer solvent species, its open void is more accessible than Th-BCTPE-2, which renders Th-BCTPE-1 a more efficient absorbent for both CrO42− and Cr2O72−.
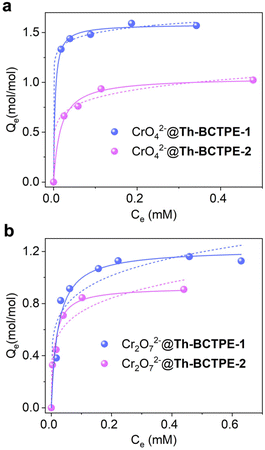 |
| Fig. 5 Adsorption isotherms of Th-BCTPE-1 and Th-BCTPE-2 for (a) CrO42− and (b) Cr2O72− fitted with the Langmuir model (solid line) and the Freundlich model (dashed line). | |
Elucidating the correlation between the structure of MOF and the sensing efficacy of Cr(VI) oxyanions is essential to the rational design of new chemosensors.52–54 Therefore, structural and photophysical parameters, including void volumes, BET surface areas, PLQYs, KSV values, and LODs, as well as the maximum adsorption capacities, are summarized in Table 1. As seen, the porosity, surface area, and PLQY are not necessarily relevant to the sensing efficacy. In contrast, the trend of maximum adsorption capacity is in line with that of KSV, implying that the sensing efficacy could be more strongly associated with Cr(VI) adsorption. Indeed, additional insights into the correlation between the sensing efficacy and sorption capacity can be derived from the S–V plots extending to higher a concentration range (Fig. S8†). For both CrO42− and Cr2O72− sensing, Th-BCTPE-1 showed saturation plateaux at higher concentrations than Th-BCTPE-2. These observations conjointly suggest that the luminophores of Th-BCTPE-1 are more accessible to CrO42− and Cr2O72− than those of Th-BCTPE-2, which could be largely ascribed to the higher adsorption capacities of Th-BCTPE-1 for Cr(VI) oxyanions. In addition, a UV-vis absorption spectroscopy study revealed that the spectra of K2CrO4 and K2Cr2O7 exhibit two intense absorption peaks at 230–310 nm and 310–450 nm, both of which overlap with the excitation spectra of Th-BCTPE-1 and Th-BCTPE-2 (Fig. S9†). Consequently, the emission intensity reduction and eventual luminescence quenching upon titration of chromium oxyanions can be attributed to a strong inner filter effect.55–57 More importantly, the overlap efficacy between the excitation spectrum of Th-BCTPE-1 and the absorption spectrum of Cr(VI) is greater than that of Th-BCTPE-2, which makes Th-BCTPE-1 as a more sensitive sensor for Cr(VI) detections.
Table 1 The void volumes, BET surface areas, photoluminescence quantum yields, KSV values, LODs, and maximum adsorption capacities of Th-BCTPE-1 and Th-BCTPE-2
Products |
Void (%) |
BET surface area (m2 g−1) |
PLQY (%) |
CrO42− |
Cr2O72− |
K
SV (M−1) |
LOD (nM) |
Q
max (mol mol−1) |
K
SV (M−1) |
LOD (nM) |
Q
max (mol mol−1) |
Th-BCTPE-1
|
34.1 |
188 |
50.53 |
2.4(1) × 105 |
9.0 |
1.58 |
4.6(3) × 105 |
4.6 |
1.05 |
Th-BCTPE-2
|
64.7 |
316 |
53.07 |
1.30(7) × 105 |
159 |
1.22 |
2.222(9) × 105 |
94 |
0.93 |
The high sensing efficacy, low LOD, and excellent stability of Th-BCTPE-1 make it well-suited for the practical application of Cr(VI) sensing (Fig. S10†). To evaluate its potential applicability to real-world conditions, the interfering effects from the environmentally relevant cations and anions were examined by dispersing finely ground Th-BCTPE-1 in M(NO3)x (M = Al3+, Cr3+, Mg2+, Ca2+, Sr2+, Cu2+, Zn2+, Mn2+, Ni2+, or K+, 2 mM) and NaxL solutions (L = F−, Cl−, Br−, I−, IO3−, NO3−, HCO3−, SO42−, BO33−, Cr2O72−, and CrO42−, 2 mM) (Fig. 6a). As shown in Fig. 6b, the luminescence of Th-BCTPE-1 was completely quenched by CrO42− and Cr2O72− with a ratio of 99.84% and 99.91%, respectively. In contrast, minor to moderate emission reductions (−3.52% to 23.62%) occurred upon immersing Th-BCTPE-1 in interfering cation or anion solutions. These results are testaments to the excellent sensing selectivity of Th-BCTPE-1 over the environmentally relevant cationic and anionic species.58 To achieve the facile and on-site sensing of the Cr(VI) oxyanion, we further fabricated the MOF-based polyvinylidene fluoride (PVDF) membrane by dispersing finely ground Th-BCTPE-1 in PVDF.59,60 As shown in Fig. 6c, the as-prepared Th-BCTPE-1@PVDF membrane shows green luminescence under a 365 nm UV lamp.
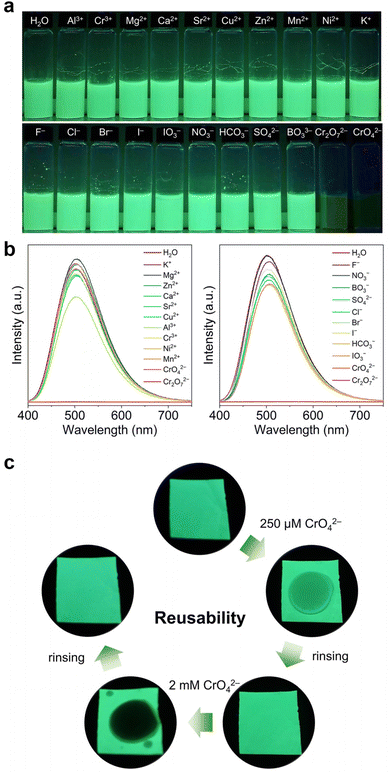 |
| Fig. 6 (a) Photographs of Th-BCTPE-1 immersed in different cation and anion solutions under UV irradiation. (b) Photoluminescence spectra of Th-BCTPE-1 immersed in different cation and anion solutions. (c) Photographs of a Th-BCTPE-1@PVDF membrane showing its excellent reusability. | |
Upon applying CrO42− or Cr2O72− solution, a dark spot was observed and its darkness increased with the increasing Cr(VI) concentration. More strikingly, the quenched luminescence of Th-BCTPE-1@PVDF can be recovered by simply rinsing the membrane with DI water, indicating the excellent reusability of Th-BCTPE-1@PVDF.
Conclusions
In summary, LMOFs have been considered a promising material platform for sensing carcinogenic chromate and dichromate. Therefore, unravelling the correlation between the MOF structure and the sensing efficacy is essential to the rational design of high-performance MOF-based sensors. In this work, a simple synthetic modulation approach has given rise to two luminescent Th-MOFs, Th-BCTPE-1 and Th-BCTPE-2, which are built from similar hexanuclear SBUs and identical BCTPE2− linkers. Such polymorphism of Th-MOFs in terms of topology allows for elucidating how the structure of MOF, in isolation, influences the sensing efficacy of Cr(VI) oxyanions. Indeed, we find that the UiO-type MOF Th-BCTPE-1 has greater KSV and lower LOD for both CrO42− and Cr2O72− than the more porous Th-BCTPE-2. Th-BCTPE-1 features the second highest KSV of CrO42− (2.84(4) × 105) among all MOF-based Cr(VI) sensors. Moreover, the sensing efficacies of CrO42− and Cr2O72− have proved to be positively correlated with the adsorption capacity and degree of the inner filter effect; the higher ones for Th-BCTPE-1 render its luminophores more accessible to Cr(VI) oxyanions. In contrast, other physiochemical properties, including porosity, BET surface area, and photoluminescence quantum yield, appear to play a much less significant role in determining the sensing efficacy. In addition, Th-BCTPE-1 exhibits excellent sensing selectivity for CrO42− and Cr2O72−, which is a key merit for the deployment of LMOFs in environmentally related conditions.
Experimental section
Synthesis
Th-BCTPE-1
.
A mixture of Th(NO3)4·6H2O (4.7 mg, 0.008 mmol), 4,4′-(1,2-diphenylethene-1,2-diyl)dibenzoic acid (H2BCTPE, 1.7 mg, 0.004 mmol), DMF (0.308 mL), MeOH (0.2 mL), and trifluoroacetic acid (0.04 mL) in a capped vial was heated at 80 °C for 48 h. Colourless octahedral crystals were obtained, filtered, washed with MeOH and Et2O, and dried at room temperature. The pH values before and after crystallization of Th-BCTPE-1 were 0.91 and 5.71, respectively. Yield: 47% based on H2BCTPE. Anal. calcd for [Th6(μ3-OH)4(μ3-O)4(BCTPE)6(H2O)6]·(C3H7NO)18·(H2O)9, C222H268N18Th6O65, C, 47.44%; H, 4.81%; N, 4.49%. Found: C, 47.08%; H, 4.48%; N, 4.77%.
Th-BCTPE-2
.
A mixture of Th(NO3)4·6H2O (4.7 mg, 0.008 mmol), 4,4′-(1,2-diphenylethene-1,2-diyl)dibenzoic acid (H2BCTPE, 0.9 mg, 0.002 mmol), DMF (0.879 mL), and concentrated nitric acid (0.025 mL) in a capped vial was heated at 120 °C for 96 h. Colourless needle crystals were obtained, filtered, washed with MeOH and Et2O, and dried at room temperature. The pH values before and after the crystallization of Th-BCTPE-2 were 1.12 and 5.33, respectively. Yield: 44% based on H2BCTPE. Anal. calcd for [Th6(μ3-OH)4(μ3-O)4(BCTPE)5(HCOO)2(H2O)6]·(C3H7NO)26·(H2O)32, C220H354N26Th6O96, C, 42.00%; H, 5.67%; N, 5.79%. Found: C, 41.51%; H, 5.33%; N, 5.99%.
Characterization
Single-crystal X-ray diffraction data were collected on a Bruker D8-Venture single-crystal X-ray diffractometer equipped with an IμS 3.0 microfocus X-ray source (Mo-Kα radiation, λ = 0.71073 Å) and a CMOS detector. The data frames were collected using the APEX3 program and processed using the SAINT routine. The empirical absorption correction was applied using the SADABS program.61 The structure was solved by intrinsic phasing with ShelXT and refined with ShelXL using OLEX2.62–64 All the non-H atoms were subjected to anisotropic refinement by a full-matrix program. Contributions to scattering due to these highly disordered solvent molecules were removed using the SQUEEZE routine of PLATON.40 Structures were then refined again using the data generated. Crystal data and details of the data collection are given in Table S1.† Powder X-ray diffraction (PXRD) data were collected from 2 to 40° with a step of 0.02° on a Bruker D8 Advance diffractometer with Cu Kα radiation (λ = 1.54178 Å). The calculated PXRD pattern was produced from the CIFs using the Mercury 1.4.2 program. The N2 adsorption isotherms were obtained at 77 K by using a Micromeritics ASAP 2020. The freshly prepared Th-MOFs were soaked in 20 mL of DCM three times over 1 h (20 min each) and subsequently immersed in 20 mL n-hexane three times over 1 h (20 min each). The excitation and luminescence spectra were collected on an Edinburgh Instruments FS5 fluorescence spectrometer. The photoluminescence lifetimes and quantum yields were obtained on an Edinburgh Instruments FLS 980 spectrometer. Scanning electron microscopy (SEM) images and energy-dispersive spectroscopy (EDS) data were collected on a Zeiss Merlin Compact LEO 1530 VP scanning electron microscope with the energy of the electron beam being 15 kV. Thermalgravimetric analysis (TGA) was carried out on a NETZSCH STA 449 F3 Jupiter instrument in the range of 30–800 °C under a nitrogen flow at a heating rate of 10 °C min−1.
Author contributions
J. L. conceived and designed the research. Z. J. L., Y. J., and X. L. synthesized the materials and performed the luminescence study. Z. J. L. and Y. L. solved the crystal structures. X. L. W. performed the BET study. J. L., Z. J. L., J. Q., J. Q. W., Z. H. Z., M. Y. H., and L. Z. analysed the data and wrote the manuscript. All authors have given approval to the manuscript.
Conflicts of interest
The authors declare no competing financial interests.
Acknowledgements
This work was supported by the National Natural Science Foundation of China (22076196, U22B20139, 21906163, and 21876182), the Strategic Priority Research Program of the Chinese Academy of Sciences (XDA21000000), and the K. C. Wong Education Foundation (GJTD-2018-10).
References
- B. Dhal, H. N. Thatoi, N. N. Das and B. D. Pandey, Chemical and microbial remediation of hexavalent chromium from contaminated soil and mining/metallurgical solid waste: A review, J. Hazard. Mater., 2013, 250–251, 272–291 CrossRef CAS PubMed.
- S. S. Galley, A. A. Arico, T.-H. Lee, X. Deng, Y.-X. Yao, J. M. Sperling, V. Proust, J. S. Storbeck, V. Dobrosavljevic, J. N. Neu, T. Siegrist, R. E. Baumbach, T. E. Albrecht-Schmitt, N. Kaltsoyannis and N. Lanatà, Uncovering the Origin of Divergence in the CsM(CrO4)2 (M = La, Pr, Nd, Sm, Eu; Am) Family through Examination of the Chemical Bonding in a Molecular Cluster and by Band Structure Analysis, J. Am. Chem. Soc., 2018, 140, 1674–1685 CrossRef CAS PubMed.
- USDOE Hanford Site First Five Year Review Report, USDOE Hanford Site First Five Year Review Report, DOE-RL-2011-56, 2001.
-
P. E. Dresel, M. J. Truex and M. D. Sweeney, Review of Techniques to Characterize the Distribution of Chromate Contamination in the Vadose Zone of the 100 Areas at the Hanford Site, United States, 2007 Search PubMed.
- A. M. Zayed and N. Terry, Chromium in the environment: factors affecting biological remediation, Plant Soil, 2003, 249, 139–156 CrossRef CAS.
- P. Izak, P. Hrma, B. W. Arey and T. J. Plaisted, Effect of feed melting, temperature history, and minor component addition on spinel crystallization in high-level waste glass, J. Non-Cryst. Solids, 2001, 289, 17–29 CrossRef CAS.
- H. Fei, C. H. Pham and S. R. J. Oliver, Anion exchange of the cationic layered material [Pb2F2]2+, J. Am. Chem. Soc., 2012, 134, 10729–10732 CrossRef CAS PubMed.
- H. Fei, M. R. Bresler and S. R. J. Oliver, A New Paradigm for Anion Trapping in High Capacity and Selectivity: Crystal-to-Crystal Transformation of Cationic Materials, J. Am. Chem. Soc., 2011, 133, 11110–11113 CrossRef CAS PubMed.
- H. Yang and H. Fei, Exfoliation of a two-dimensional cationic inorganic network as a new paradigm for high-capacity CrVI-anion capture, Chem. Commun., 2017, 53, 7064–7067 RSC.
- X. Chen, X. Dai, R. Xie, J. Li, A. Khayambashi, L. Xu, C. Yang, N. Shen, Y. Wang, L. He, Y. Zhang, C. Xiao, Z. Chai and S. Wang, Chromate separation by selective crystallization, Chin. Chem. Lett., 2020, 31, 1974–1977 CrossRef CAS.
- H. Fei, C. S. Han, J. C. Robins and S. R. J. Oliver, A Cationic Metal–Organic Solid Solution Based on Co(II) and Zn(II) for Chromate Trapping, Chem. Mater., 2013, 25, 647–652 CrossRef CAS.
- J. G. Farmer, R. P. Thomas, M. C. Graham, J. S. Geelhoed, D. G. Lumsdon and E. Paterson, Chromium speciation and fractionation in ground and surface waters in the vicinity of chromite ore processing residue disposal sites, J. Environ. Monit., 2002, 4, 235–243 RSC.
- Z. Hu, B. J. Deibert and J. Li, Luminescent metal–organic frameworks for chemical sensing and explosive detection, Chem. Soc. Rev., 2014, 43, 5815–5840 RSC.
- Y. Bai, Y. Dou, L.-H. Xie, W. Rutledge, J.-R. Li and H.-C. Zhou, Zr-based metal–organic frameworks: design, synthesis, structure, and applications, Chem. Soc. Rev., 2016, 45, 2327–2367 RSC.
- Q. Wang, Q. Gao, A. M. Al-Enizi, A. Nafady and S. Ma, Recent advances in MOF-based photocatalysis: environmental remediation under visible light, Inorg. Chem. Front., 2020, 7, 300–339 RSC.
- X. Wang, P. C. Lan and S. Ma, Metal–Organic Frameworks for Enzyme Immobilization: Beyond Host Matrix Materials, ACS Cent. Sci., 2020, 6, 1497–1506 CrossRef CAS PubMed.
- Y. Ye, S. Xian, H. Cui, K. Tan, L. Gong, B. Liang, T. Pham, H. Pandey, R. Krishna, P. C. Lan, K. A. Forrest, B. Space, T. Thonhauser, J. Li and S. Ma, Metal-Organic Framework Based Hydrogen-Bonding Nanotrap for Efficient Acetylene Storage and Separation, J. Am. Chem. Soc., 2022, 144, 1681–1689 CrossRef CAS PubMed.
- Z. Niu, Z. Fan, T. Pham, G. Verma, K. A. Forrest, B. Space, P. K. Thallapally, A. M. Al-Enizi and S. Ma, Self-Adjusting Metal-Organic Framework for Efficient Capture of Trace Xenon and Krypton, Angew. Chem., Int. Ed., 2022, 61, 1433–7851 Search PubMed.
- W. Liu, Y. Wang, Z. Bai, Y. Li, Y. Wang, L. Chen, L. Xu, J. Diwu, Z. Chai and S. Wang, Hydrolytically Stable Luminescent Cationic Metal Organic Framework for Highly Sensitive and Selective Sensing of Chromate Anions in Natural Water Systems, ACS Appl. Mater. Interfaces, 2017, 9, 16448–16457 CrossRef CAS PubMed.
- Z.-J. Li, M. Lei, H. Bao, Y. Ju, H. Lu, Y. Li, Z.-H. Zhang, X. Guo, Y. Qian, M.-Y. He, J.-Q. Wang, W. Liu and J. Lin, A cationic thorium–organic framework with triple single-crystal-to-single-crystal transformation peculiarities for ultrasensitive anion recognition, Chem. Sci., 2021, 12, 15833–15842 RSC.
- Z.-J. Li, X. Wang, L. Zhu, Y. Ju, Z. Wang, Q. Zhao, Z.-H. Zhang, T. Duan, Y. Qian, J.-Q. Wang and J. Lin, Hydrolytically Stable Zr-Based Metal–Organic Framework as a Highly Sensitive and Selective Luminescent Sensor of Radionuclides, Inorg. Chem., 2022, 61, 7467–7476 CrossRef CAS PubMed.
- Z.-H. Zhu, Z. Ni, H.-H. Zou, G. Feng and B. Z. Tang, Smart Metal-Organic Frameworks with Reversible Luminescence/Magnetic Switch Behavior for HCl Vapor Detection, Adv. Funct. Mater., 2021, 31, 2106925 CrossRef CAS.
- X. Li, H. Xu, F. Kong and R. Wang, A Cationic Metal–Organic Framework Consisting of Nanoscale Cages: Capture, Separation, and Luminescent Probing of Cr2O72− through a Single-Crystal to Single-Crystal Process, Angew. Chem., Int. Ed., 2013, 52, 13769–13773 CrossRef CAS.
- B. Parmar, Y. Rachuri, K. K. Bisht and E. Suresh, Mixed-Ligand LMOF Fluorosensors for Detection of Cr(VI) Oxyanions and Fe3+/Pd2+ Cations in Aqueous Media, Inorg. Chem., 2017, 56, 10939–10949 CrossRef CAS PubMed.
- F.-Y. Yi, J.-P. Li, D. Wu and Z.-M. Sun, A Series of Multifunctional Metal–Organic Frameworks Showing Excellent Luminescent Sensing, Sensitization, and Adsorbent Abilities, Chem. – Eur. J., 2015, 21, 11475–11482 CrossRef CAS PubMed.
- C. Zhang, L. Sun, Y. Yan, H. Shi, B. Wang, Z. Liang and J. Li, A novel photo- and hydrochromic europium metal-organic framework with good anion sensing properties, J. Mater. Chem. C, 2017, 5, 8999–9004 RSC.
- T.-Y. Gu, M. Dai, D. J. Young, Z.-G. Ren and J.-P. Lang, Luminescent Zn(II) Coordination Polymers for Highly Selective Sensing of Cr(III) and Cr(VI) in Water, Inorg. Chem., 2017, 56, 4668–4678 CrossRef PubMed.
- R. Lv, J. Wang, Y. Zhang, H. Li, L. Yang, S. Liao, W. Gu and X. Liu, An amino-decorated dual-functional metal–organic framework for highly selective sensing of Cr(iii) and Cr(vi) ions and detection of nitroaromatic explosives, J. Mater. Chem. A, 2016, 4, 15494–15500 RSC.
- Y. Lin, X. Zhang, W. Chen, W. Shi and P. Cheng, Three Cadmium Coordination Polymers with Carboxylate and Pyridine Mixed Ligands: Luminescent Sensors for Fe-III and Cr-VI Ions in an Aqueous Medium, Inorg. Chem., 2017, 56, 11768–11778 CrossRef CAS PubMed.
- Z.-J. Li, X. Guo, J. Qiu, H. Lu, J.-Q. Wang and J. Lin, Recent advances in the applications of thorium-based metal–organic frameworks and molecular clusters, Dalton Trans., 2022, 51, 7376–7389 RSC.
- K. Lv, S. Fichter, M. Gu, J. März and M. Schmidt, An updated status and trends in actinide metal-organic frameworks (An-MOFs): From synthesis to application, Coord. Chem. Rev., 2021, 446, 214011 CrossRef CAS.
- E. A. Dolgopolova, O. A. Ejegbavwo, C. R. Martin, M. D. Smith, W. Setyawan, S. G. Karakalos, C. H. Henager, H.-C. zur Loye and N. B. Shustova, Multifaceted Modularity: A Key for Stepwise Building of Hierarchical Complexity in Actinide Metal–Organic Frameworks, J. Am. Chem. Soc., 2017, 139, 16852–16861 CrossRef CAS PubMed.
- E. A. Dolgopolova, A. M. Rice and N. B. Shustova, Actinide-Based MOFs: a Middle Ground in Solution and Solid-State Structural Motifs, Chem. Commun., 2018, 54, 6472–6483 RSC.
- O. A. Ejegbavwo, C. R. Martin, O. A. Olorunfemi, G. A. Leith, R. T. Ly, A. M. Rice, E. A. Dolgopolova, M. D. Smith, S. G. Karakalos, N. Birkner, B. A. Powell, S. Pandey, R. J. Koch, S. T. Misture, H.-C. z. Loye, S. R. Phillpot, K. S. Brinkman and N. B. Shustova, Thermodynamics and Electronic Properties of Heterometallic Multinuclear Actinide-Containing Metal–Organic Frameworks with “Structural Memory”, J. Am. Chem. Soc., 2019, 141, 11628–11640 CrossRef CAS PubMed.
- C. R. Martin, G. A. Leith, P. Kittikhunnatham, K. C. Park, O. A. Ejegbavwo, A. Mathur, C. R. Callahan, S. L. Desmond, M. R. Keener, F. Ahmed, S. Pandey, M. D. Smith, S. R. Phillpot, A. B. Greytak and N. B. Shustova, Heterometallic Actinide-Containing Photoresponsive Metal-Organic Frameworks: Dynamic and Static Tuning of Electronic Properties, Angew. Chem., Int. Ed., 2021, 60, 8072–8080 CrossRef CAS PubMed.
- Z.-J. Li, S. Guo, H. Lu, Y. Xu, Z. Yue, L. Weng, X. Guo, J. Lin and J.-Q. Wang, Unexpected Structural Complexity of Thorium Coordination Polymers and Polyoxo Cluster Built from Simple Formate Ligands, Inorg. Chem. Front., 2020, 7, 260–269 RSC.
- Y. Ju, Z.-J. Li, H. Lu, Z. Zhou, Y. Li, X.-L. Wu, X. Guo, Y. Qian, Z.-H. Zhang, J. Lin, J.-Q. Wang and M.-Y. He, Interpenetration Control in Thorium Metal–Organic Frameworks: Structural Complexity toward Iodine Adsorption, Inorg. Chem., 2021, 60, 5617–5626 CrossRef CAS PubMed.
- Z.-J. Li, Y. Ju, Z. Zhang, H. Lu, Y. Li, N. Zhang, X.-L. Du, X. Guo, Z.-H. Zhang, Y. Qian, M.-Y. He, J.-Q. Wang and J. Lin, Unveiling the Unique Roles of Metal Coordination and Modulator in the Polymorphism Control of Metal-Organic Frameworks, Chem. – Eur. J., 2021, 27, 17586–17594 CrossRef CAS PubMed.
- Z.-J. Li, S. Guo, H. Lu, Y. Xu, Z. Yue, L. Weng, X. Guo, J. Lin and J.-Q. Wang, Unexpected structural complexity of thorium coordination polymers and polyoxo cluster built from simple formate ligands, Inorg. Chem. Front., 2020, 7, 260–269 RSC.
- A. Spek, PLATON SQUEEZE: a tool for the calculation of the disordered solvent contribution to the calculated structure factors, Acta Crystallogr., Sect. C: Struct. Chem., 2015, 71, 9–18 CrossRef CAS PubMed.
- X.-G. Liu, C.-L. Tao, H.-Q. Yu, B. Chen, Z. Liu, G.-P. Zhu, Z. Zhao, L. Shen and B. Z. Tang, A new luminescent metal–organic framework based on dicarboxyl-substituted tetraphenylethene for efficient detection of nitro-containing explosives and antibiotics in aqueous media, J. Mater. Chem. C, 2018, 6, 2983–2988 RSC.
- N. B. Shustova, B. D. McCarthy and M. Dincă, Turn-On Fluorescence in Tetraphenylethylene-Based Metal–Organic Frameworks: An Alternative to Aggregation-Induced Emission, J. Am. Chem. Soc., 2011, 133, 20126–20129 CrossRef CAS PubMed.
- D. F. Sava Gallis, L. E. S. Rohwer, M. A. Rodriguez and T. M. Nenoff, Efficient Photoluminescence via Metal–Ligand Alteration in a New MOFs Family, Chem. Mater., 2014, 26, 2943–2951 CrossRef CAS.
- C. Peng, X. Song, J. Yin, G. Zhang and H. Fei, Intrinsic White-Light-Emitting Metal–Organic Frameworks with Structurally Deformable Secondary Building Units, Angew. Chem., Int. Ed., 2019, 58, 7818–7822 CrossRef CAS PubMed.
- Z. Wei, Z.-Y. Gu, R. K. Arvapally, Y.-P. Chen, R. N. McDougald, J. F. Ivy, A. A. Yakovenko, D. Feng, M. A. Omary and H.-C. Zhou, Rigidifying Fluorescent Linkers by Metal–Organic Framework Formation for Fluorescence Blue Shift and Quantum Yield Enhancement, J. Am. Chem. Soc., 2014, 136, 8269–8276 CrossRef CAS PubMed.
- M. H. Gehlen, The centenary of the Stern-Volmer equation of fluorescence quenching: From the single line plot to the SV quenching map, J. Photochem. Photobiol., C, 2020, 42, 100338 CrossRef CAS.
- B. Parmar, K. K. Bisht, Y. Rachuri and E. Suresh, Zn(ii)/Cd(ii) based mixed ligand coordination polymers as fluorosensors for aqueous phase detection of hazardous pollutants, Inorg. Chem. Front., 2020, 7, 1082–1107 RSC.
- Y.-J. Yang, Y.-H. Li, D. Liu and G.-H. Cui, A dual-responsive luminescent sensor based on a water-stable Cd(ii)-MOF for the highly selective and sensitive detection of acetylacetone and Cr2O72− in aqueous solutions, CrystEngComm, 2020, 22, 1166–1175 RSC.
- M. B. Luo, Y. Y. Xiong, H. Q. Wu, X. F. Feng, J. Q. Li and F. Luo, The MOF+ Technique: A Significant Synergic Effect Enables High Performance Chromate Removal, Angew. Chem., Int. Ed., 2017, 56, 16376–16379 CrossRef CAS PubMed.
- S. R. J. Oliver, Cationic inorganic materials for anionic pollutant trapping and catalysis, Chem. Soc. Rev., 2009, 38, 1868–1881 RSC.
- Z.-J. Li, Y. Ju, B. Yu, X. Wu, H. Lu, Y. Li, J. Zhou, X. Guo, Z.-H. Zhang, J. Lin, J.-Q. Wang and S. Wang, Modulated synthesis and isoreticular expansion of Th-MOFs with record high pore volume and surface area for iodine adsorption, Chem. Commun., 2020, 56, 6715–6718 RSC.
- X. Chen, Y. Yu, C. Yang, J. Yin, X. Song, J. Li and H. Fei, Fabrication of Robust and Porous Lead Chloride-Based Metal-Organic Frameworks toward a Selective and Sensitive Smart NH3 Sensor, ACS Appl. Mater. Interfaces, 2021, 13, 52765–52774 CrossRef CAS PubMed.
- S. E. Crawford, J. E. Ellis, P. R. Ohodnicki and J. P. Baltrus, Influence of the Anionic Zinc-Adeninate Metal-Organic Framework Structure on the Luminescent Detection of Rare Earth Ions in Aqueous Streams, ACS Appl. Mater. Interfaces, 2021, 13, 7268–7277 CrossRef CAS PubMed.
- H. Yuan, N. Li, W. Fan, H. Cai and D. Zhao, Metal-Organic Framework Based Gas Sensors, Adv. Sci., 2021, 9, 2104374 CrossRef PubMed.
- A. S. Tanwar, L. R. Adil, M. A. Afroz and P. K. Iyer, Inner Filter Effect and Resonance Energy Transfer Based Attogram Level Detection of Nitroexplosive Picric Acid Using Dual Emitting Cationic Conjugated Polyfluorene, ACS Sens., 2018, 3, 1451–1461 CrossRef CAS PubMed.
- P. Daga, S. Sarkar, P. Majee, D. K. Singha, S. Hui, P. Mahata and S. K. Mondal, A selective detection of nanomolar-range noxious anions in water by a luminescent metal-organic framework, Mater. Adv., 2021, 2, 985–995 RSC.
- L. Qiu, Z. Ma, P. Li, X. Hu, C. Chen, X. Zhu, M. Liu, Y. Zhang, H. Li and S. Yao, Sensitive and selective detection of chromium(VI) based on two-dimensional luminescence metal organic framework nanosheets via the mechanism integrating chemical oxidation-reduction and inner filter effect, J. Hazard. Mater., 2021, 419, 126443 CrossRef CAS PubMed.
- X. Wang, Z.-J. Li, Y. Ju, X. Li, J. Qian, M.-Y. He, J.-Q. Wang, Z.-H. Zhang and J. Lin, A MOF-based luminometric sensor for ultra-sensitive and highly selective detection of chromium oxyanions, Talanta, 2023, 252, 123894 CrossRef CAS PubMed.
- C. Liang, S. Zhang, L. Cheng, J. Xie, F. Zhai, Y. He, Y. Wang, Z. Chai and S. Wang, Thermoplastic Membranes Incorporating Semiconductive Metal–Organic Frameworks: An Advance on Flexible X-ray Detectors, Angew. Chem., Int. Ed., 2020, 59, 11856–11860 CrossRef CAS PubMed.
- M. S. Denny Jr., J. C. Moreton, L. Benz and S. M. Cohen, Metal-organic frameworks for membrane-based separations, Nat. Rev. Mater., 2016, 1, 16078 CrossRef.
-
G. M. Sheldrick, SADABS, program for empirical absorption correction of area detector data, University of Göttingen, Göttingen, Germany, 1996 Search PubMed.
- G. M. Sheldrick, SHELXT – Integrated space-group and crystal-structure determination, Acta Crystallogr., Sect. A: Found. Adv., 2015, 71, 3–8 CrossRef PubMed.
- G. M. Sheldrick, Crystal structure refinement with SHELXL, Acta Crystallogr., Sect. C: Struct. Chem., 2015, 71, 3–8 Search PubMed.
- O. V. Dolomanov, L. J. Bourhis, R. J. Gildea, J. A. K. Howard and H. Puschmann, OLEX2: a complete structure solution, refinement and analysis program, J. Appl. Crystallogr., 2009, 42, 339–341 CrossRef CAS.
Footnote |
† Electronic supplementary information (ESI) available: PXRD, TGA, excitation and PL spectra, photoluminescence lifetimes, quenching rates, SEM-EDS, and crystallographic data. CCDC 2213561 and 2213562. For ESI and crystallographic data in CIF or other electronic format see DOI: https://doi.org/10.1039/d2qi02631g |
|
This journal is © the Partner Organisations 2023 |
Click here to see how this site uses Cookies. View our privacy policy here.