DOI:
10.1039/D0RA08027F
(Paper)
RSC Adv., 2020,
10, 39842-39853
Zinc(II)-cyclen coordinative amphiphiles for enhanced gene delivery
Received
20th September 2020
, Accepted 23rd October 2020
First published on 2nd November 2020
Abstract
In this study, we developed coordinative amphiphiles for use as novel non-viral DNA vectors. As a modification of a conventional cationic lipid structure, we replaced the cationic head with a zinc(II)-1,4,7,10-tetraazacyclododecane (Zn-cyclen) complex as a phosphate-directing group, and used biocompatible skeletons (α-tocopherol or cholesterol) as hydrophobic tails. The structure-activity relationship (SAR) was systematically investigated to study the effect of Zn-coordination on the gene transfection between cyclen-based traditional head-tail lipids and Zn(II)-cyclen coordinative amphiphiles. The results reveal that both Zn-free lipids and Zn-containing amphiphiles could condense DNA into nano-sized particles with appropriate size and zeta-potentials. Agarose gel retardation assay and MTS-based cell viability assays demonstrated that the Zn(II)-cyclen complex exhibited slightly lower DNA binding ability and much lower cytotoxicity compared to liposome analogues, respectively. Most importantly, in vitro transfection studies showed that the coordination of zinc(II) to cyclen may dramatically increase the transfection efficiency of the conventional cationic lipid, and α-tocopherol-containing coordinative amphiphile Zn-Cyc-Toc gives the best transfection efficiency, which was enhanced 24.4 times after coordination and was 6.1 times higher than commercial transfection reagent lipofectamine 2000. Mechanism studies confirmed that the DNA complex formed from Zn-Cyc-Toc might induce higher cellular uptake and better endosomal escape ability than the lipoplexes formed from Zn-free lipid Cyc-Toc. This study not only demonstrates that these coordinative amphiphiles might be promising non-viral gene vectors, but also presents a novel strategy to enhance the gene transfection efficiency and biocompatibility of cyclen-based cationic lipids.
Introduction
Over the past decades, gene therapy, which involves the delivery of genetic drugs such as plasmid DNA and antisense oligonucleotides to the targeted cells thus treating the disease at the level of its origin, has made significant progress as a potential method for treating genetic disorders and cancer of both innate and acquired origins.1–3 Development of safe and efficient gene delivery vectors is a critical challenge for the application of gene therapy since free oligonucleotides and DNA are rapidly degraded by serum nucleases in the blood and the unfavorable electrostatic interactions between macromolecular DNA and the biological cell surface (both being negatively charged) could restrict uptake into cells.4,5 Of the many reported gene delivery systems, cationic lipids were the earliest to be described and the most investigated. Conventional cationic lipids typically comprise a cationic head, a linker, and a hydrophobic tail.6,7 The headgroup of cationic lipids play a crucial role for gene delivery in that they can bind to the negatively charged phosphate backbone of nucleic acid, leading to DNA condensation.8 Most structures of cationic lipids reported so far utilize nonspecific amine-based cationic head groups. As a result, the cationic character enhances cellular uptake of nucleic acids due to the resulting cationic surfaces of the complexes, which have greater cell membrane affinity.9 However, redundant positive surface charges might induce nonspecific binding to components in blood, resulting in less efficient transport or higher toxicity.10–12
Besides electrostatic attraction, other chemical interactions between nucleic acids and vectors as alternatives such as covalent bonding,13,14 sequence-specific hybridization,15–17 intercalation18 and coordinative interaction19 have also been investigated as means of binding therapeutic oligonucleotides to delivery carriers. Of these approaches, coordinative interaction is drawing increasing attention. It was reported that Zn(II) ions could bind to the phosphate groups, which induces the nucleic acid to self-assemble to form Zn/DNA or Zn/siRNA nanoclusters.20 Moreover, some zinc complexes, especially Zn(II) coordinated-dipicolyamine complexes (Zn-DPA) have analogously unique properties and been found to show high affinity with phosphate derivatives.21,22 Chen et al. first introduced Zn-DPA to hyaluronic acid (HA) for siRNA delivery by utilizing Zn-DPA to bind therapeutic molecules that contain phosphate groups. The result indicates that siRNA was bound on the HADPA-Zn-NPs by coordination with Zn-DPA.23 Inspired by this, Bang and co-workers designed a series of coordinative amphiphiles with Zn-DPA as a phosphate-directing group and various membrane-directing groups as hydrophobic tails for efficient siRNA delivery.24 Similarly, a series of Zn-DPA based cationic lipids as non-viral gene vectors was carried out by Liu.25 More recently, Guo and co-workers introduced Zn-DPA complex to low molecular weight PEI by biodegradable disulfide bond, realizing efficient gene delivery to primary and stem cells.26 These studies showed that coordinative interaction as alternatives to electrostatic attraction is feasible and Zn coordination may endow benefits in gene delivery processes.
1,4,7,10-Tetraazacyclododecane (cyclen) been frequently introduced into cationic lipids as the hydrophilic headgroup for its strong binding ability toward DNA and non-self-folded conformation in our previous works.27–29 Nevertheless, most of these cyclen-based cationic lipids revealed unsatisfactory transfection efficiency (TE) compared to commercial transfection agents due to the unduly strong electrostatic interactions and subsequent hard DNA release.30,31 Interestingly, the cyclen has unique cyclic structure besides the characteristics mentioned above and has a strong coordination ability toward a wide range of cations, such as transition metal ions (Zn2+, Cu2+ or Co2+) and lanthanide ions.32,33 As a result, cyclen-based metal coordination, particularly that of zinc cyclen (Zn-cyclen) analogues, may provide an option for DNA binding because of its high affinity to phosphodiester moieties.34,35 Additionally, since cell membranes are mainly composed of phosphate-containing components, we anticipate that introducing the function of the Zn-coordinative ligand to cationic lipids would not only greatly strengthen its DNA binding affinity but also drastically enhance the cellular uptake of the cyclen-base conventional cationic lipids, which would be particularly beneficial for the TE subsequently.
Herein, we report the design and synthesis of coordinative amphiphiles with Zn(II)-cyclen (Zn-Cyc) complexes as head-group and biocompatible α-tocopherol or cholesterol as hydrophobic tails to give a highly effective and safe non-viral vector (Zn-Cyc-Toc) or (Zn-Cyc-Cho). This strategy could achieve the transformation of traditional cationic lipids to a highly efficient and safe gene vehicle. The effect of Zn-coordination on the gene transfection of this new-fashioned gene delivery system is discussed in detail. Results reveal that the Zn-coordination could significantly enhance the TE by enhancing the cellular uptake and reduce the cytotoxicity of the conventional liposomes by the unique binding affinity with phospholipid cell membrane. The evidences suggest that our strategy offers a practical and versatile platform to functionalize traditional cationic lipids for high-performance and low cytotoxicity gene transfection.
Experimental section
Materials and methods
All of the common chemicals and reagents were obtained commercially and were used as received. Anhydrous chloroform were dried and purified under nitrogen by using standard methods and were distilled immediately before use. [4,7,10-Tris(tert-butoxycarbonyl)-1,4,7,10-tetraaza-cyclododecan-1-yl] (3Boc-cyclen) were prepared according to the literature.30 1,2-Dioleoyl-sn-glycero-3-phosphoethanolamine (DOPE) was purchased from Avanti Polar Lipids, Inc. pUC19 DNA was purchased from TIANTAI (Chengdu, China). MicroBCA protein assay kit was obtained from Pierce (Rockford, IL, USA). Luciferase assay kit and MTS (3-(4,5-dimethylthiazol-2-yl)-5-(3-carboxymethoxyphenyl)-2-(4-sulfophenyl)-2H tetrazolium, inner salt) were purchased from Promega (Madison, WI, USA). Endotoxin-free plasmid purification kit was purchased from TIANGEN (Beijing, China). The plasmids used in the study were pGL-3 (Promega, Madison, WI, USA) coding for luciferase and pEGFP-N1 (Clontech, Palo Alto, CA, USA) coding for EGFP. The Dulbecco's modified Eagle's medium (DMEM), fetal bovine serum (FBS) and Lipofectamine® 2000 were purchased from Invitrogen Corp. The 1H NMR and 13C NMR spectra were measured on a Bruker AM400 NMR spectrometer. Proton Chemical shifts of NMR spectra were given in ppm relative to internals reference TMS (1H, 0.00 ppm). HRMS spectral data was recorded on a Bruker Daltonics Bio TOF mass spectrometer.
Some usual procedures such as dynamic light scattering (DLS) experiments, transmission electron microscopy (TEM), preparation of cationic liposomes and liposome/DNA complexes (lipoplexes), agarose-gel retardation assay, amplification and purification of plasmid DNA, cell culture, cytotoxicity assays and cellular uptake of plasmid DNA were performed according to our reported literatures.36
Synthesis of the coordinative amphiphiles
Preparation of compound 3. A mixture of epichlorohydrin (5.6 g, 60.5 mmol), R–OH (cholesterol or tocopherol, 20 mmol), sodium hydroxide pellets (2.4 g, 60 mmol), tetrabutylammonium bromide (322 mg, 1 mmol) and water (1 mL, 56 mmol) were stirred for 24 h at 40 °C. Then the reaction mixture was filtered off and the solid was washed with dichloromethane. The combined organic layer was dried with anhydrous sodium sulfate. The solvent and excess epichlorohydrin were distilled off under reduced pressure and the obtained residue was purified by silica gel column chromatography (PE/EA = 40/1, v/v) to give compound 3 as oil.
Compound 3a (yield 55.3%). 1H NMR (CDCl3, 400 MHz): δ (ppm) = 5.37 (s, 1H), 3.74 (m, 1H), 3.48 (m, 1H), 3.24 (m, 1H), 3.16 (m, 1H), 2.83 (t, 1H), 2.64 (m, 1H), 2.39 (m, 1H), 2.24 (m, 1H), 2.02 to 0.87 (m, 38H), 0.68 (s, 3H).
Compound 3b (yield 63.2%). 1H NMR (CDCl3, 400 MHz): δ (ppm) = 3.90 (m, 1H), 3.65 (m, 1H), 3.35 (m, 1H), 2.88 (t, J = 4.6 Hz, 1H), 2.71 (m, 1H), 2.57 (t, J = 6.7 Hz, 2H), 2.18 (s, 3H), 2.14 (s, 3H), 2.08 (s, 3H), 1.80 (m, 2H), 1.60–1.05 (m, 24H), 0.84 (s, 12H).
Preparation of compound 5. 3Boc-cyclen (compound 3, 1.00 mmol), compound 3 (1.20 mmol) and 20 mL of anhydrous ethanol were mixed in a flask with magnetic stirring and refluxed for 60 h in an oil bath. After the reaction, the mixture was concentrated to give the crude product of 5, which were further purified by column chromatography on silica gel (PE/EtOH = 2/1, v/v).
Compound 5a (yield 62.1%). 1H NMR (CDCl3, 400 MHz): δ (ppm) = 5.34 (s, 1H, cholesterol-H), 3.89 (s, 1H, O–H), 3.72–3.13 (m, 16H, cyclen-H, O–CH, O–CH2), 2.98–2.71 (m, 4H, cyclen-H), 2.55–2.52 (d, 2H, N–CH2), 2.40–1.62 (m, 14H, cholesterol-H), 1.46–1.44 (d, 27H, Boc-H), 1.40–0.85 (m, 26H, cholesterol-H), 0.67 (s, 3H, cholesterol-H). 13C NMR (CDCl3, 100 MHz): δ (ppm) = 11.8, 18.7, 19.3, 21.0, 22.5, 22.8, 23.8, 24.2, 27.9, 28.2, 28.3, 28.4, 28.5, 28.6, 31.8, 31.9, 35.7, 36.1, 36.8, 37.1, 39.0, 39.1, 39.5, 39.7, 42.3, 48.2, 50.1, 56.1, 56.7, 57.8, 67.9, 71.1, 79.3, 79.6, 121.7, 140.7, 155.5. HR-MS (ESI): C53H95N4O8 [M + H]+, 915.7144, found: 915.7150.
Compound 5b (yield 46.5%). 1H NMR (CDCl3, 400 MHz): δ (ppm) = 4.11 (m, 1H, O–H), 3.75–3.19 (m, 16H, cyclen-H, O–CH2, O–CH, CH2), 2.92 (s, 3H, CH2, cyclen-H), 2.58–2.48 (m, 4H, N–CH2, cyclen-H), 2.16 (s, 3H, tocopheryl-CH3), 2.12 (s, 3H, tocopheryl-CH3), 2.07 (s, 3H, tocopheryl-CH3), 1.83–1.70 (m, 2H, CH2), 1.53–1.50 (m, 3H, CH), 1.48–1.45 (d, 27H, Boc-H), 1.38–1.36 (m, 3H, CH3), 1.33–1.04 (m, 18H, CH2), 0.88–0.84 (m, 12H, CH3). 13C NMR (CDCl3, 100 MHz): δ (ppm) = 11.7, 11.8, 12.7, 19.6, 19.7, 20.6, 21.0, 22.6, 22.7, 23.8, 24.4, 24.7, 27.9, 28.5, 28.6, 31.3, 32.7, 37.2, 37.4, 39.3, 40.0, 50.1, 58.2, 58.4, 74.8, 75.5, 79.3, 79.7, 117.5, 122.9, 125.6, 127.6, 147.6, 147.9. HR-MS (ESI): C55H99N4O9 [M + H]+, 959.7407, found: 959.7416.
Preparation of compound 6. Compound 5 (0.5 mmol) was suspended in anhydrous dichloromethane (5 mL), whereafter, a solution of trifluoroacetic acid (5 mL) in anhydrous dichloromethane (5 mL) was added dropwise under ice bath. And then, the obtained mixture was stirred at room temperature for 6 h. After the solvent and trifluoroacetic acid were removed, the conventional head-tail type cationic lipids 6 (Cyc-Cho or Cyc-Toc) were directly obtained as light yellow liquid by treating the residues with anhydrous ethyl ether twice.
Compound 6a (Cyc-Cho, yield 95.4%). 1H NMR (CDCl3, 400 MHz): δ (ppm) = 5.32 (s, 1H, cholesterol-H), 3.87 (s, 2H, O–CH, O–CH2), 3.75–3.70 (m, 2H, O–CH, O–CH2), 3.52–2.94 (m, 18H, cyclen-H, N–CH2), 2.68–0.82 (m, 41H, cholesterol-H), 0.67 (s, 2H, cholesterol-H). 13C NMR (CDCl3, 100 MHz): δ (ppm) = 11.8, 13.7, 18.0, 18.1, 18.2, 18.3, 18.7, 19.3, 19.7, 20.2, 21.0, 21.3, 22.5, 22.6, 22.7, 22.8, 22.9, 23.8, 24.2, 25.4, 25.5, 27.9, 28.0, 28.2, 31.4, 31.6, 31.8, 35.5, 35.7, 35.8, 36.1, 36.7, 37.0, 37.9, 39.0, 39.5, 42.3, 43.0, 44.5, 50.1, 50.9, 55.2, 55.6, 56.1, 56.7, 58.4, 64.3, 67.3, 69.9, 70.1, 75.6, 75.8, 79.8, 121.8, 140.4, 141.2. HR-MS (ESI): C38H71N4O2 [M + H]+, 615.5572, found: 615.5569.
Compound 6b (Cyc-Toc, yield 92.6%). 1H NMR (CDCl3, 400 MHz): δ (ppm) = 4.13 (s, 1H, O–H), 3.76–3.71 (m, 1H, O–CH), 3.62–3.50 (m, 3H, O–CH2, CH2), 3.08–2.72 (m, 17H, cyclen-H, CH2), 2.55–2.51 (m, 2H, N–CH2), 2.08 (s, 2H, tocopheryl-CH3), 2.05 (s, 5H, tocopheryl-CH3), 1.80–1.71 (m, 2H, tocopheryl-CH3), 1.55–1.41 (m, 2H, CH2), 1.40–1.04 (m, 24H, CH2, CH, CH3), 0.87–0.84 (m, 12H, CH3). 13C NMR (CDCl3, 100 MHz): δ (ppm) = 11.5, 11.7, 12.4, 15.7, 19.6, 19.7, 20.5, 21.0, 22.6, 23.5, 24.4, 24.7, 27.9, 29.6, 31.2, 32.7, 37.2, 37.4, 37.5, 39.3, 40.3, 58.4, 64.3, 67.6, 74.2, 74.9, 117.4, 117.8, 123.1, 125.5, 127.9, 147.1, 148.2. HR-MS (ESI): C40H75N4O3 [M + H]+, 659.5834, found: 659.5839.
Preparation of compound 8. The cationic lipids 6 (0.5 mmol) were dispersed in distilled water, then the pretreated anion exchange resin (Amberjet 4200, Cl form) were added and stirred until the solution turned alkaline. After removing the resin by filtration, the filtrate was lyophilized to remove the water to give spumescent solid 7.The lyophilized products 7 were dissolved in methanol (10 mL). Then the Zn(NO3)2·6H2O (0.6 mmol) was added and stirred for 6 h. After reaction completed, the methanol was evaporated under reduced pressure. The product was redissolved in water and dialyzed (100–500 Da) to remove the redundant zinc ion. Then the water was evaporated under reduced pressure to obtain the faint yellow solid 8 (Zn-Cyc-Cho or Zn-Cyc-Toc).
Compound 8a (Zn-Cyc-Cho, yield 95.4%). 1H NMR (CDCl3, 400 MHz): δ (ppm) = 5.33 (s, 1H, cholesterol-H), 3.87 (s, 2H, O–CH, O–CH2), 3.75–3.69 (m, 2H, O–CH, O–CH2), 3.53–2.94 (m, 18H, cyclen-H, N–CH2), 2.68–0.82 (m, 41H, cholesterol-H), 0.67 (s, 2H, cholesterol-H). HR-MS (ESI): C38H70N5O5Zn [M + Zn + NO3]+, 740.4663, found: 740.4670.
Compound 8b (Zn-Cyc-Toc, yield 92.6%). 1H NMR (CDCl3, 400 MHz): δ (ppm) = 4.12 (s, 1H, O–H), 3.76–3.71 (m, 1H, O–CH), 3.62–3.51 (m, 3H, O–CH2, CH2), 3.08–2.72 (m, 17H, cyclen-H, CH2), 2.55–2.51 (m, 2H, N–CH2), 2.09 (s, 2H, tocopheryl-CH3), 2.05 (s, 5H, tocopheryl-CH3), 1.80–1.71 (m, 2H, tocopheryl-CH3), 1.55–1.41 (m, 2H, CH2), 1.42–1.04 (m, 24H, CH2, CH, CH3), 0.87–0.84 (m, 12H, CH3). HR-MS (ESI): C40H74N5O6Zn [M + Zn + NO3]+, 784.4925, found: 784.4915.
Cell culture
CHO (Chinese hamster ovary cell lines), HepG2 (human liver hepatocellular carcinoma cell lines) and HeLa cells were incubated in Dulbecco's modified Eagle's medium (DMEM) containing 10% fetal bovine serum (FBS) and 1% antibiotics (penicillin 10
000 U mL−1 and streptomycin 10
000 μg mL−1) at 37 °C in a humidified atmosphere containing 5% CO2.
Cytotoxicity assays
Toxicities of lipoplexes toward HepG2 cells and HeLa cells were determined by using MTS reduction assay following literature procedures. About 1.0 × 104 cells per well were seeded into 96-well plates. After 24 h, optimized lipids/DOPE formulations were completed with 0.2 μg of pEGFP-N1 DNA at various N/P ratios for 30 min; 100 μL of lipoplexes were added to the cells in the absence of serum. After 4 h of incubation, lipoplexes solutions were removed, and 100 μL of media with 10% FBS was added. After 24 h, 20 μL MTS and 100 μL PBS were added to each well and the plates were incubated at 37 °C for another 1 h. Then, the absorbance of each sample was measured using an ELISA plate reader (model 680, BioRad) at a wavelength of 490 nm. The cell viability (%) was obtained according to the manufacturer's instruction. Lipoplex prepared from Lipofectamine 2000 was used as control.
In vitro transfection procedure
In order to obtain about 80% confluent cultures at the time of transfection, 24-well plates were seeded with 1.0 × 105 cells per well in 500 μL of antibiotic-free media 24 h before transfection. For the preparation of lipoplexes applied to cells, various amounts of liposomes and DNA were serially diluted separately in anti-biotic-free DMEM culture medium; then, the DNA solutions were added into liposome solutions and mixed briefly by pipetting up and down several times, after which the mixtures were incubated at room temperature for about 30 min to obtain lipoplexes of desired N/P ratios, the final lipoplexes volume was 100 μL, and the DNA was used at a concentration of 0.8 μg per well. After 30 min of complexation, old cell culture medium was removed from the wells, cells were washed once with serum-free DMEM, and the above 100 μL lipoplexes were added to each well. The plates were then incubated for 4 h at 37 °C in a humidified atmosphere containing 5% CO2. At the end of incubation period, medium was removed, and 500 μL of fresh DMEM medium containing 10% FBS was added to each well. Plates were further incubated for a period of 24 h before checking the reporter gene expression.
For fluorescent microscopy assays, cells were transfected by complexes containing pEGFP-N1. After 24 h incubation, the microscopy images were obtained at the magnification of 100 and recorded using Viewfinder Lite (1.0) software. Control transfection was performed in each case using a commercially available transfection reagent Lipofectamine 2000™ based on the standard conditions specified by the manufacture. After 24 h transfection of pEGFP plasmid, lysed cells and take 100 μL lysate to measure the fluorescence intensity. Excitation wavelength was 485 nm and the emission wavelength was 538 nm. Lipofectamine 2000™ was chosen as control.
For luciferase assays, cells were transfected by complexes containing pGL-3. For a typical assay in a 24-well plate, 24 h post transfection as described above, the old medium was removed from the wells, and the cells were washed twice with 500 μL of prechilled PBS. According to Luciferase assay kit (Promega) manufacture, 100 μL of 1× cell lysis buffer diluted with PBS was then added to each well, and the cells were lysed for 30 min in a horizontal rocker at room temperature. The cell lysate was transferred completely to Eppendorf tubes and centrifuged (4000 rpm, RT) for 2 min; the supernatant was transferred to Eppendorf tubes and stored in ice. For the assay, 20 μL of this supernatant and 100 μL of luciferase assay substrate (Promega) were used. The lysate and the substrate were both thawed to RT before performing the assay. The substrate was added to the lysate, and the luciferase activity was measured in a luminometer (Turner designs, 20/20, Promega, USA) in standard single-luminescence mode. The integration time of measurement was 10
000 ms. A delay of 2 s was given before each measurement. The protein concentration in the cell lysate supernatant was estimated in each case with Lowry protein assay kit (PIERCE, Rockford, IL, USA). Comparison of the transfection efficiencies of the individual lipids was made based on the data for luciferase expressed as relative light units (RLU) per mg of protein. All the experiments were done in triplicates, and results presented are the average of at least two such independent experiments done on the same days.
Cellular uptake of plasmid DNA
The cellular uptake of the liposome/fluorescein labelled-DNA complexes was analyzed by flow cytometry. The Label IT Cy5 Labeling Kit was used to label pGL-3 with Cy5 according to the manufacturer's protocol. Briefly, HeLa cells were seeded onto 12-well plates (2.0 × 105 cells per well) and allow to attach and grown for 24 h. Before transfection, the medium was replaced with serum-free culture medium. Cells were incubated with Cy5 labelled DNA nanoparticles (1.6 μg DNA per well, optimal N/P ratio of each sample) in media for 4 h at 37 °C. Subsequently, the cells were washed with 1× PBS and harvested with 0.25% trypsin/EDTA and resuspended in 1× PBS. Cy5-labelled plasmid DNA uptake was measured in the FL4 channel using the red diode laser (633 nm). Data from 10
000 events were gated using forward and side scatter parameters to exclude cell debris. The flow cytometer (BD Accuri™ C6) was calibrated for each run to obtain a background level of ∼1% for control samples (i.e., untreated cells).
Confocal laser scanning microscopy (CLSM)
HeLa cells were seeded at a density of 2.5 × 105 cells per well in 35 mm confocal dish (Φ = 15 mm), 24 h prior to transfection. For transfection in the absence of serum, the medium was exchange with serum-free medium (for transfection with serum, the medium was exchanged with serum-containing medium). Complexes of liposomes and Cy5-labelled pGL-3 at a given concentration were added to each well. After incubation at 37 °C for 4 h, cells were rinsed for 3 times with PBS (pH 7.4), fixed with 4% paraformaldehyde (dissolved with PBS buffer) for 10 min, nuclear staining was done with DAPI. The CLSM observation was performed using confocal laser scanning microscope (CLSM, 4) at excitation wavelengths of 405 nm for DAPI (blue), 633 nm for Cy5 (red), respectively. For endosome escape experiment, after incubation at 37 °C for different times (4 h, 8 h, 12 h and 24 h), LysoTracker green (1
:
750) was added into well for another 30 min incubation at 37 °C. Then cells were rinsed for 3 times with PBS (pH 7.4), fixed with 4% paraformaldehyde (dissolved with PBS buffer) for 10 min, lysosome staining was done with LysoTracker green. The CLSM observation was performed using confocal laser scanning microscope (CLSM, ZEISS LSM 780) at excitation wavelengths of 504 nm for LysoTracker green (green), 633 nm for Cy5 (red), respectively.
Results and discussion
Synthesis of the zinc(II)-cyclen coordinative amphiphiles
The structures of target coordinative amphiphiles are extremely simple and their syntheses are straightforward. All the final materials were readily obtained following known procedures for epoxide synthesis, epoxide ring-opening and zinc complexation. As shown in Scheme 1, the epoxidation derivatives 3a–3b were prepared by reacting R–OH (cholesteryl or tocopheryl) with epichlorohydrin in the presence of sodium hydroxide, water and tetrabutylammonium bromide (TBAB), which was used as a phase transfer catalyst. Subsequent epoxide ring-opening and Boc deprotection by CF3COOH gave the cyclen-based cationic lipids 6a–6b (Cyc-Cho or Cyc-Toc). Finally, 6a–6b was treated with anion exchange resin to give free cyclen amines (7a–7b), which were then coordinated with 1.5 equiv of Zn(NO)3·6H20 to yield target Zinc(II)-cyclen coordinative amphiphiles 8a–8b (Zn-Cyc-Cho or Zn-Cyc-Toc). The product was redissolved in water and dialyzed (100–500 Da) to remove the redundant zinc ion. All new compounds in each step were purified and their structures were confirmed by 1H NMR, 13C NMR and HRMS.
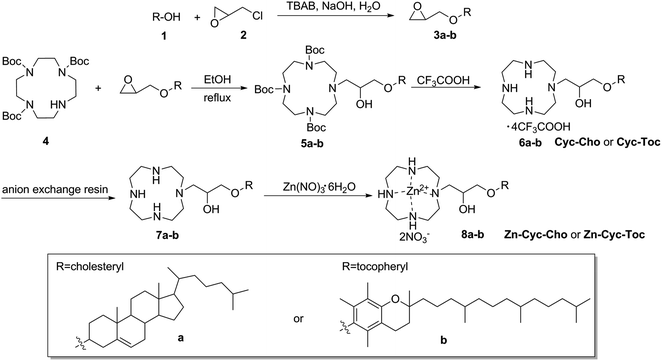 |
| Scheme 1 Synthesis route of target coordinative amphiphiles. | |
Formation of liposomes and their interaction with DNA
Cationic liposomes for gene delivery are more frequently from a combination of cationic lipid and neutral lipids such as 1,2-dioleoyl-sn-glycero-3-phosphoethanolamine (DOPE), which might increase the transfection performance significantly due to its special membrane fusion ability.37 Therefore, the conventional liposomes (Cyc-Cho and Cyc-Toc) and novel liposomes (Zn-Cyc-Cho and Zn-Cyc-Toc) were prepared by the thin film hydration method with the lipids/DOPE molar ratio of 1
:
2. The physical properties of the self-assembly liposomes were firstly investigated by dynamic light scattering (DLS) assay since proper sizes and zeta potentials are important factors for the liposomes used as gene vectors. As shown in Fig. 1A, all of these liposomes were nano-sized particles with diameters around 115 nm. Neither the hydrophobic chain nor the Zn-coordination would have a significant effect on average diameter of the nanoparticles. Similar results were found on the zeta-potential of these liposomes and all of them have a positive surface potential around +55 mV (Fig. 1B), indicating that zinc(II) coordination to the hydrophilic head cyclen will not affect the self-assembly behavior of these cationic lipids, especially on particle sizes and zeta-potential.
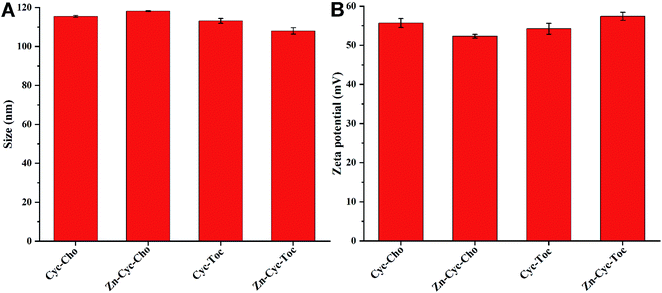 |
| Fig. 1 Mean particle sizes (A) and zeta potentials (B) of four liposomes with the lipids/DOPE molar ratio of 1 : 2 obtained by DLS at 25 °C. Data represent mean ± SD (n = 3). | |
Cationic liposomes can bind and condense plasmid DNA (pUC-19) by electrostatic interactions, which is conducive to avoiding degradation of DNA by DNase and shielding the electrostatic repulsion between DNA and the negatively charged cell membrane. Therefore, the agarose-gel retardation assay was carried out to examine the DNA binding ability of the liposomes. As shown in Fig. 2, cyclen-based cationic liposomes (Cyc-Cho and Cyc-Toc) can effectively bind plasmid DNA and can completely retard DNA migration at the N/P ratio of 4. However, after Zn coordination, the DNA binding abilities of Zn-contained liposomes were much lower than those of the counterparts and complete DNA retardation could be achieved at an N/P of 6, indicating that the coordinative interaction between Zn-cyclen complexes and phosphate group of DNA is weaker than the electrostatic interaction between cyclen and plasmid DNA. Meanwhile, the hydrophobic chain seemed to play little part on DNA binding abilities.
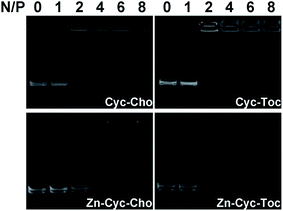 |
| Fig. 2 Electrophoretic gel retardation assays of plasmid DNA (pUC-19) with the presence of liposomes formed from lipid or Zn-cyclen complexes at different N/P ratios. The molar ratio of lipid/DOPE was 1 : 2 and 0.125 μg pUC-19 DNA was used per well. | |
As is known to all that a positive surface charge and an appropriate particle size of lipoplexes are necessary for efficient gene delivery. Then, these physical properties of the liposome-DNA complexes (lipoplexes) formed from Zn-free or Zn-contained liposomes were studied by DLS, and the results are shown in Fig. 2. After binding with plasmid DNA, the size of the lipoplexes particles increased evidently. The particle sizes of Zn-free lipoplexes are decreasing along with the rising of N/P ratios and very stable at room temperature. While, these of Zn-contained lipoplexes were irregular due to the weaker DNA binding ability and incomplete DNA condensation under a lower N/P ratio (≤6), which was consistent with the results obtained in gel electrophoresis. From an N/P ratio of 8, two types of liposomes were able to condense DNA into steady nanosized particles: ∼200 nm and ∼270 nm, respectively (Fig. 3A). Zeta-potentials of the lipoplexes rose along with the increase of the N/P ratio, and finally reached +35–40 mV above an N/P of 10 (Fig. 3B). Moreover, the morphologies of the liposome formed from Zn-Cyc-Toc and Zn-Cyc-Toc/DNA lipoplexes were observed by transmission electron microscopy (TEM). The images revealed that the liposomes formed from Zn-Cyc-Toc gave spherical nanoparticles with diameters about 50 nm (Fig. 3C). The lipoplexes also had spherical shape, while the size was bigger (∼100 nm) than the empty bolasomes (Fig. 3D).
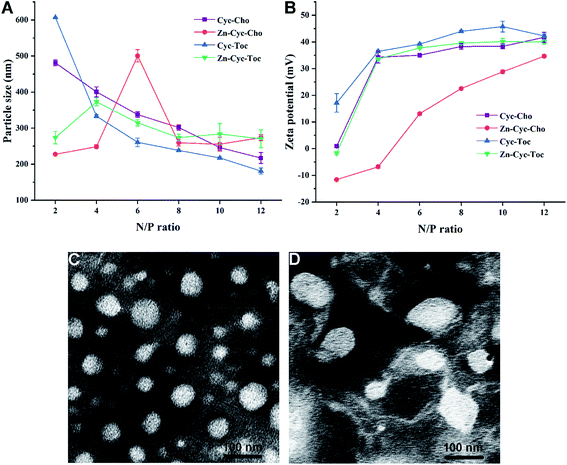 |
| Fig. 3 Characterization of the lipoplexes: mean particle sizes (A) and zeta-potentials (B) of the lipoplexes formed from Zn-free or Zn-contained liposomes and DNA at various N/P ratio measured by DLS at 25 °C. Data represent mean ± SD (n = 3). (C and D) TEM images of liposome and lipoplex (N/P = 8) formed from Zn-Cyc-Toc. | |
In vitro cytotoxicity
Low cytotoxicity of a synthetic gene delivery vector was another critical factor for possible application in further clinical trials. It was reported that Zn coordination could greatly reduce the cytotoxicity of cationic polymers.26 Therefore, the cell viabilities of the lipoplexes prepared at various N/P ratios were evaluated in HeLa, HepG2 and CHO cells by using an MTS assay to demonstrate such superiority of Zn coordination in cationic liposomes materials, and Lipofectamine 2000 was used as control. Results in Fig. 4 reveal that the cytotoxicity of conventional cationic liposomes Cyc-Cho and Cyc-Toc increased along with the increase of N/P ratio and they exhibited obvious toxicity, especially at relatively high N/P ratios (>12). However, after coordination of Zn to the liposomes, both cell lines showed higher tolerance against the Zn-contained materials than the Zn-free liposomes and the positive control. Zn-Cyc-Cho and Zn-Cyc-Toc showed nearly 100% cell viability even if the N/P ratio was as high as 16. Such results reveal that Zn-coordination is an effective method to reduce the cytotoxicity of the cyclen-based biomaterials. We conjecture that zinc cation could decrease the positive charge of the amino groups on the cyclen ring by electro-withdrawing effect, which would refrain from redundant positive surface charges of lipoplexes.
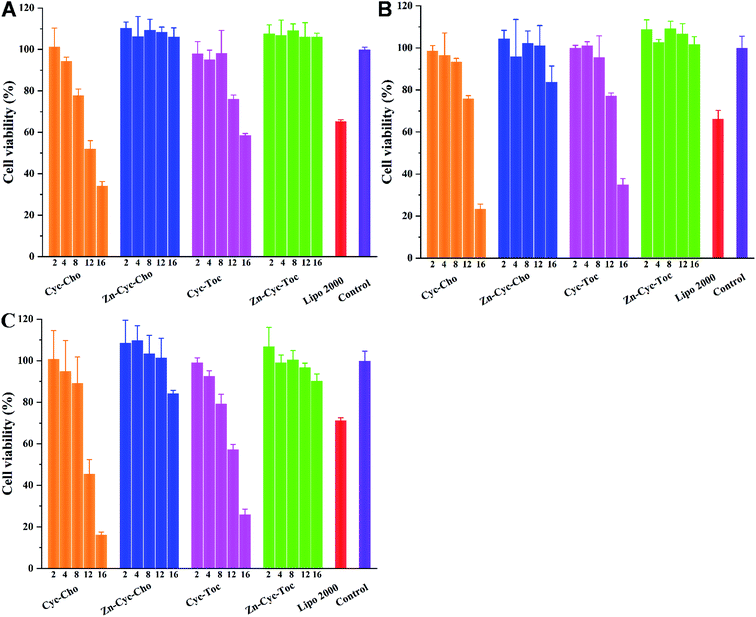 |
| Fig. 4 In vitro cytotoxicity of lipoplexes formed from Zn-free or Zn-contained liposomes at various N/P ratios in HeLa (A), HepG2 (B) and CHO cells (C) for a 24 h incubation. Lipoplexes were prepared with 0.2 μg of pGL-3 plasmid at various N/P ratios. Data represent mean ± SD (n = 3). | |
In vitro gene transfection
To investigate the potential of the Zinc(II)-cyclen coordinative amphiphiles as gene delivery vector, in vitro gene transfection was first evaluated on CHO, HeLa and HepG2 cells by using the enhanced green fluorescent protein (eGFP) reporter gene, and commercial transfection reagent Lipofectamine 2000 was used as positive controls. As shown in Fig. 5, GFP images post transfection distinctly validated that Zn-Cyc-Cho and Zn-Cyc-Toc outperformed the counterparts and the commercial reagent in all three cell lines. The conventional head-tail cationic liposomes Cyc-Cho and Cyc-Toc gave few eGFP expression except in CHO cells and showed high cytotoxicity (Fig. 4), which might be attributed to difficult DNA release from the lipoplexes. However, after Zn-coordination functionalization, the density of the green fluorescence significantly increased, which was comparable to Lipofectamine 2000. Moreover, the luciferase reporter gene (pGL-3) was also used to quantitatively study the transfection efficiency (TE) of the liposomes in CHO and HeLa cells. As shown in Fig. 6, the results were consistent with those obtained in eGPF assays. Zn-cyclen functionalization demonstrated its superiority in benefiting the gene delivery, especially in HeLa cells. At the optimal N/P ratio, up to 10.6- and 24.4-fold higher luciferase activity was observed with Zn-Cyc-Cho and Zn-Cyc-Toc, respectively, compared to Zn-free counterparts. Furthermore, the optimal Zn-Cyc-Toc mediated 6.1-fold enhancements of luciferase activity compared to the commercial Lipofectamine 2000. These results together impart Zn-cyclen based amphiphile as an excellent gene vector and also suggest that Zn coordination may be a promising strategy to improve the gene delivery efficiency of cyclen-based cationic liposomes, hopefully achieving robust gene transfection in those significant but hard-to-transfect cell types, such as primary and stem cells.
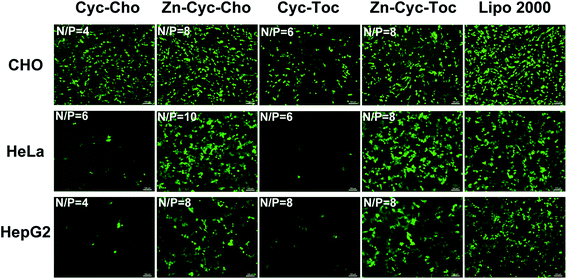 |
| Fig. 5 Fluorescent microscopy images of CHO, HeLa and HepG2 cells transfected by Zn-free or Zn-contained liposomes at the optimal N/P ratio. The molar ratio of lipid/DOPE was 1 : 2, lipofectamine 2000 was used for comparison. The cells were observed by fluorescence microscopy after a 24 h transfection. Scale bar: 100 μm. | |
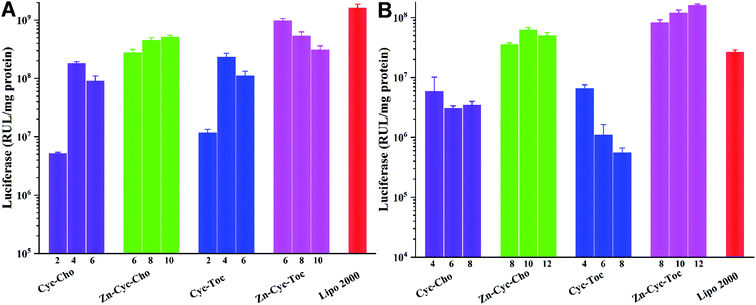 |
| Fig. 6 Luciferase expression in CHO (A) and HeLa (B) cells transfected by Zn-free or Zn-contained liposomes/DNA complexes at various N/P ratios. Data represent mean ± SD (n = 3). | |
In vitro transfection mechanism studies
To investigate the significant promotion effect of Zn coordination on transfection efficiency of Zn-contained liposomes, several mechanism studies were carried out to elucidate the fate of the cargo genes. As one of the main barriers in the gene delivery process, the internalization of the nucleic acid complexes play an important effect on transfection efficiency. Consequently, confocal laser scanning microscopy (CLSM) was primarily applied to visually examine the internalization and intracellular location of Cy5-labelled DNA transferred by Zn-free and Zn-contained liposomes at the optimal N/P ratio in CHO and HeLa cells after 4 h cell incubation. DNA was labelled by Cy5 (red), while the cell nuclei were stained with 4′,6-diamidino-2-phenylindole (DAPI, blue). As shown in Fig. 7, after 4 h of incubation with lipoplexes derived from Zn-free Cyc-Cho and Cyc-Toc, moderate fluorescent labelled DNA accumulated in the perinuclear region, while a considerable amount of signals were observed for the transfection by Zn coordination analogues Zn-Cyc-Cho and Zn-Cyc-Toc in both two cell lines, suggesting a much better cellular uptake. What's more, the red fluorescence induced by Zn-contained liposomes was observably stronger than that by Lipofectamine 2000. The fluorescence activated cell sorting (FACS) technique was applied for further study of the cellular uptake mediated by these liposomes in HeLa cells. The percentage of Cy5-positive cells and the mean fluorescence intensity (MFI) of Cy5 were calculated after a 4 h cell incubation with the lipoplexes at each optimized transfection conditions and shown in Fig. 8. It was confirmed that Zn coordination has superiority for cellular uptake. The results reveal that the percentage of cells positive for Cy5-labelled DNA increased by 15% and 50% for Zn-Cyc-Cho and Zn-Cyc-Toc after Zn coordination, respectively, which is consistent with the CLSM results and might owe to the specific affinity between the Zn(II)-cyclen complex and phosphate-containing cell membrane. From these consequences, it was believed that Zn-contained liposomes might mediate the internalization of DNA cargo with higher efficiency. What is noteworthy is that both cholesterol-based materials (Cyc-Cho and Zn-Cyc-Cho) demonstrated excellent cellular uptake (79.9% and 90.5%, respectively), which might due to the good affinity for cell membrane.38 Nevertheless, Zn-Cyc-Cho with the highest cellular uptake gave lower TE than Zn-Cyc-Toc, also suggesting that good cellular uptake is a necessary but not sufficient condition for high TE. Although positive cell percentage of Zn-Cyc-Toc was lower than that induced by Lipofectamine 2000, the MFI was much higher, indicating a higher amount of labeled DNA was internalized into the cells, thus the result that Zn-Cyc-Toc had a much higher TE than Lipofectamine 2000 might be reasonable.
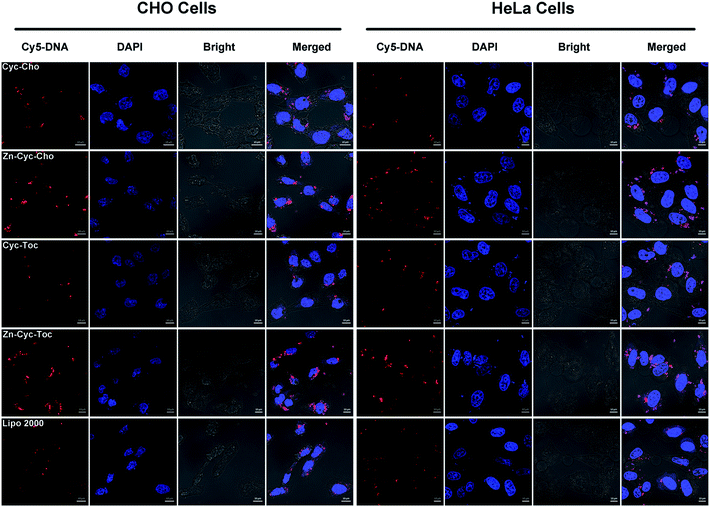 |
| Fig. 7 CLSM images of CHO and HeLa cells transfected with Cy5-labelled DNA by Zn-free or Zn-contained liposomes/DNA complexes at the optimal N/P ratio. For each row, from left to right: Cy5-DNA (red); cell nucleus stained by 4′,6-diamidino-2-phenylindole (DAPI) (blue); bright field and merged image. Scale bar: 10 μm. | |
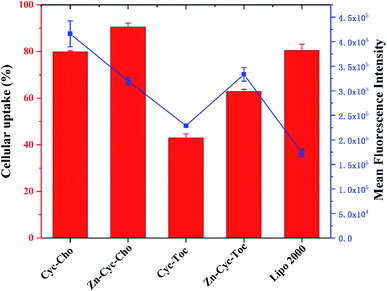 |
| Fig. 8 Cellular uptake (columns, percentage of Cy5-positive cells) and mean fluorescence intensity (dots and lines) of Zn-free or Zn-contained lipoplexes at the optimal N/P ratio in HeLa cells quantified by flow cytometry. Lipofectamine 2000 was used as the control. Data represent mean ± SD (n = 3). | |
To further study the intracellular fate of Zn-Cyc-Toc/DNA lipoplexes, which mediated the highest transfection efficiency after endocytosis, CLSM was applied to visualize the distribution of Cy5-labeled DNA (red) at the optimal N/P ratios in HeLa cells. DNA cargo, lysosome and cell nucleus were labeled/stained with Cy-5, LysoTracker green and DAPI, respectively. As shown in Fig. 9, abundant yellow colocalization fluorescent signals were found after transfection for 4 h with Zn-Cyc-Toc/DNA lipoplexes, indicating that most internalized plasmid DNA or lipoplexes was trapped in late endosomes/lysosomes. With the extension of transfection time, the red signals for labeled pDNA delivered and accumulated around the cell nuclei were markedly increased, suggesting the escape of DNA or Zn-Cyc-Toc/DNA lipoplexes from the endosome. Such results reveal that lipoplex formed from Zn-Cyc-Toc has excellent endosomal escape ability, which may contribute to its outstanding transfection efficiency.
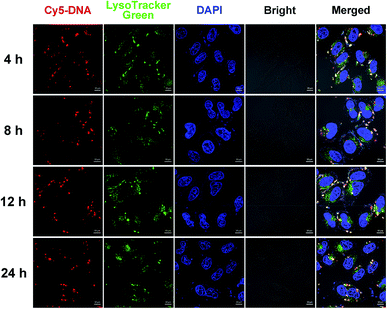 |
| Fig. 9 Confocal laser scanning microscopy (CLSM) images of HeLa cells transfected with Cy5-labelled DNA by Zn-Cyc-Toc at the optimal transfection N/P ratio at different times. For each row, from left to right: Cy5-labelled pGL-3 DNA (red); lysosomes and acidic late endosomes stained by LysoTracker Green (green); cell nucleus stained by DAPI (blue); bright field and merged image. Scale bar: 10 μm. | |
Conclusions
In summary, two conventional cyclen-based cationic lipids and their coordinative amphiphiles by coordinating zinc(II) ion to the cyclen were designed and synthesized. The potentials of these novel structures as non-viral gene delivery vectors were investigated. Results reveal that Zn(II)-cyclen based amphiphiles are all capable of forming liposomes with weaker DNA binding and condensation ability. In vitro transfection studies show that Zn-coordination functionalization not only could significantly reduce the cytotoxicity, but also dramatically enhance the transfection efficiency of the liposomes. The α-tocopherol-contained coordinative compound Zn-Cyc-Toc gives the best transfection efficiency, which enhanced 24.4 times after the coordination and was 6.1 times higher than commercial transfection reagent Lipofectamine 2000. Mechanism studies indicate that lipoplexes formed from Zn-contained liposomes induce the much higher cellular uptake. We speculate that such metal complex head-group might has a specific affinity to cell membranes, thus facilitating the membrane fusion and subsequent endocytosis. This study may give us some clues for the design of novel metal complex based gene vehicles with higher transfection efficiency and biocompatibility.
Conflicts of interest
There are no conflicts to declare.
Acknowledgements
This work was financially supported by the Science and Technology Research Program of Chongqing Municipal Education Commission (Grant No. KJQN201901341 and No. KJQN201901329) and Science & Technology Research Program of Chongqing University of Arts and Sciences (No. R2018SBX12 and R2018SCH09). Zheng Huang thanks Prof. Xiao-Qi Yu and Prof. Ji Zhang from the Key Laboratory of Green Chemistry and Technology (Ministry of Education), College of Chemistry, Sichuan University for their supports.
References
- I. M. Verma and N. Somia, Gene therapy - promises, problems and prospects, Nature, 1997, 389, 239–242 CrossRef CAS.
- M. A. Mintzer and E. E. Simanek, Nonviral Vectors for Gene Delivery, Chem. Rev., 2009, 109, 259–302 CrossRef CAS.
- X. Guo and L. Huang, Recent Advances in Nonviral Vectors for Gene Delivery, Accounts Chem. Res., 2012, 45, 971–979 CrossRef CAS.
- V. V. Kumar, C. Pichon, M. Refregiers, B. Guerin, P. Midoux and A. Chaudhuri, Single histidine residue in head-group region is sufficient to impart remarkable gene transfection properties to cationic lipids: evidence for histidine-mediated membrane fusion at acidic pH, Gene Ther., 2003, 10, 1206–1215 CrossRef CAS.
- Y.-P. Xiao, J. Zhang, Y.-H. Liu, Z. Huang, B. Wang and Y.-M. Zhang, et al. Cross-linked polymers with fluorinated bridges for efficient gene delivery, J. Mater. Chem. B, 2017, 5, 8542–8553 RSC.
- S. Bhattacharya and A. Bajaj, Advances in gene delivery through molecular design of cationic lipids, Chem. Commun., 2009, 4632–4656 RSC.
- D. Zhi, Y. Bai, J. Yang, S. Cui, Y. Zhao and H. Chen, et al. A review on cationic lipids with different linkers for gene delivery, Adv. Colloid Interface Sci., 2017, 253, 117–140 CrossRef.
- D. Zhi, S. Zhang, S. Cui, Y. Zhao, Y. Wang and D. Zhao, The Headgroup Evolution of Cationic Lipids for Gene Delivery, Bioconjugate Chem., 2013, 24, 487–519 CrossRef CAS.
- R. Zu, I. S. Zuhorn and D. Hoekstra, How cationic lipids transfer nucleic acids into cells and across cellular membranes: Recent advances, J. Controlled Release, 2013, 166, 46–56 CrossRef.
- K. Miyata, N. Nishiyama and K. Kataoka, Rational design of smart supramolecular assemblies for gene delivery: chemical challenges in the creation of artificial viruses, Chem. Soc. Rev., 2012, 41, 2562–2574 RSC.
- J. Zhou, J. Liu, C. J. Cheng, T. R. Patel, C. E. Weller and J. M. Piepmeier, et al. Biodegradable poly(amine-co-ester) terpolymers for targeted gene delivery, Nat. Mater., 2012, 11, 82–90 CrossRef CAS.
- S. Liu, J. Yang, H. Ren, J. O'Keeffe-Ahern, D. Zhou and H. Zhou, et al. Multifunctional oligomer incorporation: a potent strategy to enhance the transfection activity of poly(l-lysine), Biomater. Sci., 2016, 4, 522–532 RSC.
- M. G. Sebestyén, J. J. Ludtke, M. C. Bassik, G. Zhang, V. Budker and E. A. Lukhtanov, et al. DNA vector chemistry: The covalent attachment of signal peptides to plasmid DNA, Nat. Biotechnol., 1998, 16, 80–85 CrossRef.
- I. Dovydenko, I. Tarassov, A. Venyaminova and N. Entelis, Method of carrier-free delivery of therapeutic RNA importable into human mitochondria: Lipophilic conjugates with cleavable bonds, Biomaterials, 2016, 76, 408–417 CrossRef CAS.
- T. Roulon, C. Hélène and C. Escudé, Coupling of a Targeting Peptide to Plasmid DNA Using a New Type of Padlock Oligonucleotide, Bioconjugate Chem., 2002, 13, 1134–1139 CrossRef CAS.
- C. Srinivasan, J. Lee, F. Papadimitrakopoulos, L. K. Silbart, M. Zhao and D. J. Burgess, Labeling and Intracellular Tracking of Functionally Active Plasmid DNA with Semiconductor Quantum Dots, Mol. Ther., 2006, 14, 192–201 CrossRef CAS.
- X. Lu, T.-H. Tran, F. Jia, X. Tan, S. Davis and S. Krishnan, et al. Providing Oligonucleotides with Steric Selectivity by Brush-Polymer-Assisted Compaction, J. Am. Chem. Soc., 2015, 137, 12466–12469 CrossRef CAS.
- P. T. Wong, K. Tang, A. Coulter, S. Tang, J. R. Baker and S. K. Choi, Multivalent Dendrimer Vectors with DNA Intercalation Motifs for Gene Delivery, Biomacromolecules, 2014, 15, 4134–4145 CrossRef CAS.
- G.-Y. Li, R.-L. Guan, L.-N. Ji and H. Chao, DNA condensation induced by metal complexes, Coord. Chem. Rev., 2014, 81, 100–113 CrossRef.
- K. S. Lim, D. Y. Lee, G. M. Valencia, Y.-W. Won and D. A. Bull, Nano-Self-Assembly of Nucleic Acids Capable of Transfection without a Gene Carrier, Adv. Funct. Mater., 2015, 25, 5445–5451 CrossRef CAS.
- H.-W. Rhee, S. H. Lee, I.-S. Shin, S. J. Choi, H. H. Park and K. Han, et al. Detection of Kinase Activity Using Versatile Fluorescence Quencher Probes, Angew. Chem. Int. Ed., 2010, 49, 4919–4923 CrossRef CAS.
- B. A. Smith, W. J. Akers, W. M. Leevy, A. J. Lampkins, S. Xiao and W. Wolter, et al. Optical Imaging of Mammary and Prostate Tumors in Living Animals using a Synthetic Near Infrared Zinc(II)-Dipicolylamine Probe for Anionic Cell Surfaces, J. Am. Chem. Soc., 2010, 132, 67–69 CrossRef CAS.
- G. Liu, K. Y. Choi, A. Bhirde, M. Swierczewska, J. Yin and S. W. Lee, et al. Sticky Nanoparticles: A Platform for siRNA Delivery by a Bis(zinc(II) dipicolylamine)-Functionalized, Self-Assembled Nanoconjugate, Angew. Chem. Int. Ed., 2012, 51, 445–449 CrossRef CAS.
- J. B. Kim, Y. M. Lee, J. Ryu, E. Lee, W. J. Kim and G. Keum, et al. Coordinative Amphiphiles as Tunable siRNA Transporters, Bioconjugate Chem., 2016, 27, 1850–1856 CrossRef CAS.
- R.-C. Su, Q. Liu, W.-J. Yi and Z.-G. Zhao, Zn(II)-dipicolylamine-based metallo-lipids as novel non-viral gene vectors, J. Biol. Inorg Chem., 2017, 22, 867–879 CrossRef CAS.
- S. Liu, D. Zhou, J. Yang, H. Zhou, J. Chen and T. Guo, Bioreducible Zinc(II)-Coordinative Polyethylenimine with Low Molecular Weight for Robust Gene Delivery of Primary and Stem Cells, J. Am. Chem. Soc., 2017, 139, 5102–5109 CrossRef CAS.
- Q. Liu, Q.-Q. Jiang, W.-J. Yi, J. Zhang, X.-C. Zhang and M.-B. Wu, et al. Novel imidazole-functionalized cyclen cationic lipids: Synthesis and application as non-viral gene vectors, Bioorg. Med. Chem., 2013, 21, 3105–3313 CrossRef CAS.
- Q.-F. Zhang, W.-J. Yi, B. Wang, J. Zhang, L. Ren and Q.-M. Chen, et al. Linear polycations by ring-opening polymerization as non-viral gene delivery vectors, Biomaterials, 2013, 34, 5391–5401 CrossRef CAS.
- W.-J. Yi, Q.-F. Zhang, J. Zhang, Q. Liu, L. Ren and Q.-M. Chen, et al. Cyclen-based lipidic oligomers as potential gene delivery vehicles, Acta Biomater., 2014, 10, 1412–1422 CrossRef CAS.
- Z. Huang, Y.-H. Liu, Y.-M. Zhang, J. Zhang, Q. Liu and X.-Q. Yu, Cyclen-based cationic lipids containing a pH-sensitive moiety as gene delivery vectors, Org. Biomol. Chem., 2015, 13, 620–630 RSC.
- H.-J. Wang, X. He, Y. Zhang, J. Zhang, Y.-H. Liu and X.-Q. Yu, Hydroxyl-containing non-viral lipidic gene vectors with macrocyclic polyamine headgroups, RSC Adv., 2015, 5, 59417–59427 RSC.
- C.-Q. Xia, N. Jiang, J. Zhang, S.-Y. Chen, H.-H. Lin and X.-Y. Tan, et al. The conjugates of uracil–cyclen Zn(II) complexes: Synthesis, characterization, and their interaction with plasmid DNA, Bioorg. Med. Chem., 2006, 14, 5756–5764 CrossRef CAS.
- X.-B. Yang, J. Feng, J. Zhang, Z.-W. Zhang, H.-H. Lin and L.-H. Zhou, et al. Synthesis, DNA binding and cleavage activities of the copper (II) complexes of estrogen-macrocyclic polyamine conjugates, Bioorg. Med. Chem., 2008, 16, 3871–3877 CrossRef CAS.
- Q.-Y. Yu, Y. Guo, J. Zhang, Z. Huang and X.-Q. Yu, Zn(ii) coordination to cyclen-based polycations for enhanced gene delivery, J. Mater. Chem. B, 2019, 7, 451–459 RSC.
- Y. Guo, Q.-Y. Yu, J. Zhang, H.-Z. Yang, Z. Huang and X.-Q. Yu, Zn(ii)-cyclen complex-based liposomes for gene delivery: the advantage of Zn coordination, New J. Chem., 2019, 43, 16138–16147 RSC.
- Z. Huang, D.-M. Zhao, X. Deng, J. Zhang, Y.-M. Zhang and X.-Q. Yu, Functionalized Asymmetric Bola-Type Amphiphiles for Efficient Gene and Drug Delivery, Nanomaterials, 2018, 8, 115 CrossRef.
- I. Koltover, T. Salditt, J. O. Rädler and C. R. Safinya, An Inverted Hexagonal Phase of Cationic Liposome-DNA Complexes Related to DNA Release and Delivery, Science, 1998, 281, 78–81 CrossRef CAS.
- D. Zhi, S. Zhang, B. Wang, Y. Zhao, B. Yang and S. Yu, Transfection Efficiency of Cationic Lipids with Different Hydrophobic Domains in Gene Delivery, Bioconjugate Chem., 2010, 21, 563–577 CrossRef CAS.
|
This journal is © The Royal Society of Chemistry 2020 |
Click here to see how this site uses Cookies. View our privacy policy here.