DOI:
10.1039/D0QI00294A
(Review Article)
Inorg. Chem. Front., 2020,
7, 3282-3314
Low-temperature wet chemistry synthetic approaches towards ferrites†
Received
9th March 2020
, Accepted 11th May 2020
First published on 12th May 2020
Abstract
Ferrites are a broad class of iron-containing oxides that includes spinel ferrites MFe2O4, perovskites MFeO3, and hexagonal ferrites (hexaferrites) such as BaFe12O19. These materials have a wide array of applications owing to their diverse properties: notable instances include catalysis, piezoelectric components, magnetic components, biomedical applications, heterogeneous catalysis and photocatalysis. Given the growing importance of environmentally friendly, low-temperature methodologies to obtain functional materials, there is a growing interest in synthetic approaches which are compatible with the principles of “green chemistry”. In this context, wet chemistry represents an attractive choice, and furthermore offers the possibility of scale-up for manufacture of materials in volumes for practical application. Though there is a sizeable amount of literature on the synthesis of ferrites, the most common approaches require treatments at temperatures above 200 °C, either as the main synthetic procedure itself (thermal decomposition), or as a post-synthetic step (for example, calcination after sol–gel autocombustion). This review aims at summarising, categorising, classifying and critically discussing the different low-temperature (<200 °C), wet chemistry approaches employed in recent years for the synthesis of ferrites. This will include hydrothermal, solvothermal, sonochemical, and microwave methods, with examples taken from literature making reference to the various sub-classes of ferrites.
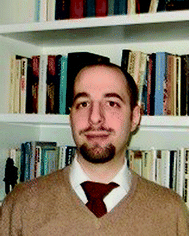 Stefano Diodati | Stefano Diodati is a postdoc fellow in the workgroup of Prof. Silvia Gross. His field of research concerns the synthesis of crystalline inorganic nanostructures (ferrites, oxides, sulphides) through wet chemistry methodologies, with a particular focus on low temperature, green methods such as subcritical hydrothermal synthesis. A further focus of his research is the characterisation of inorganic materials from the compositional (XPS, ICP-MS), structural (powder XRD) and morphological (SEM, AFM) point of view. Most recently he worked on the statistical characterisation of coffee extracts through comparative NMR and other complementary techniques. |
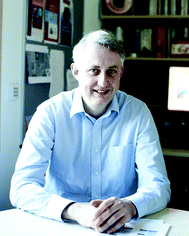 Richard I. Walton | Richard Walton is Professor of Chemistry at the University of Warwick and his research group is working in the field of inorganic materials, focussed on the use of innovative synthesis methods for functional solids. This spans zeotypes and metal–organic frameworks through to condensed oxides, with an emphasis on solution-based synthesis routes and characterisation using diffraction and spectroscopy including at synchrotron and neutron facilities. Practical applications are explored and exploited through various industrial collaborations including in areas such as heterogeneous catalysis and ionic conductors. He is presently an editor of the Inorganic Materials Series published by the Royal Society of Chemistry. |
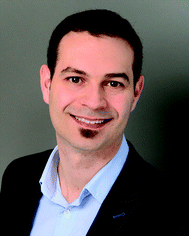 Simone Mascotto | Simone Mascotto (1981, Trento, Italy) is Assistant Professor of Inorganic Chemistry at the University of Hamburg, Germany. He received a MSc degree in Industrial Chemistry from the University of Padua (2006) and a PhD in Physical Chemistry from the Justus-Liebig-University of Giessen (2009). In 2010–2013 he was a Marie Curie postdoctoral fellow at the University of Trento, Italy and research associate at the Helmholtz-Zentrum Berlin, Germany. His research group focuses on the design of metal oxides with tailored surface properties and on the investigation of surface-related phenomena in nanomaterials by scattering methods at large scale facilities. |
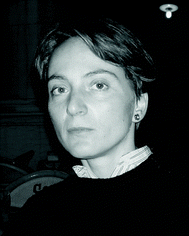 Silvia Gross | Silvia Gross is Professor of Inorganic and Colloid Chemistry at the University of Padova and her research group is working in the field of wet-chemistry and colloidal synthesis of different inorganic materials (oxides, sulphides, metal nanoparticles), with particular focus on low temperature and sustainable routes. A relevant part of the activity is devoted to the thorough structural, morphological and chemico-physical characterisation of the materials, also by time-resolved and/or in situ experiments performed at synchrotron radiation sources. She is DFG Mercator Fellow and regularly visiting professor at the Justus-Liebig University of Giessen. She has authored about 160 ISI papers. |
Ferrites: a useful and multifaceted class of compounds
Ferrites are a broad class of oxides containing iron which in general include spinel ferrites MFe2O4, perovskites, ilmenite (FeTiO3) and hexagonal ferrites (or hexaferrites). These materials have a wide array of applications owing to their diverse properties.1–10 Notable examples include catalysis,11–15 piezoelectric components,16,17 magnetic components,18–21 biomedical applications22,23 and photocatalysis,24–29 photoanodes30 and photocathodes.31 In particular, their magnetic properties make them convenient materials for heterogeneous catalysis as they greatly simplify catalyst isolation and recovery operations. In general, the nature of ferrites as iron-containing oxides endows them with important magnetic properties, which vary depending on the specific structure and nature of non-iron metals involved.32,33 Spinels in particular (due to their specific structure – vide infra) have been shown to display both hard (CoFe2O4)6 and soft33 magnetic behaviour, making them very attractive materials in applications ranging from magnetic recording to magnetic resonance imaging (MRI).6,34,35
Given the importance of ferrites in a wide variety of fields, numerous works have been dedicated to the synthesis and study of these materials.1,3,18,33,34,36–46 The present review will, however, focus specifically on wet chemistry methods which not only afford the desired materials, but also comply with the principles of Green Chemistry47 and can be achieved whilst maintaining low temperatures (≤200 °C) of synthesis, due to the importance for industry to steadily move towards greener and more environmentally friendly approaches (vide infra “Green chemistry: the need for low-temperature wet synthesis approaches”).
Spinels
In general, spinel ferrites can be described as ferrites having the formula MFe2O4, where M is a divalent metal species (such as Fe, Co, Zn, Ni Cu or others).32,48–51 From a crystallographic point of view, spinel ferrites crystallise in a cubic close-packed structure32,34 having Fd
m symmetry with a unit cell containing 8 formula units (M8Fe16O32).21,34 In particular, metal ions within this structure occupy 1/8 of the tetrahedral and 1/2 of the octahedral sites.32,48–50,52 Spinel structures are divided between normal, inverse and partially inverse spinels. In normal spinel ferrites (such as for instance ZnFe2O4)53 the trivalent metal (i.e. Fe) occupies octahedral sites, whereas the divalent metal ions (Zn) lie in tetrahedral sites (Fig. 1). A second class of spinels are known as inverse spinels (such as NiFe2O4);52 in inverse ferrites the iron cations are equally split between tetrahedral and octahedral sites, whereas the divalent atoms occupy in turn the octahedral sites vacated by iron.
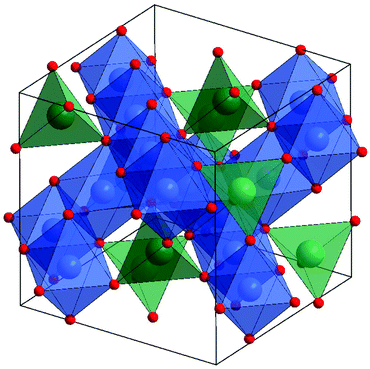 |
| Fig. 1 Crystal structure of normal spinel ferrite ZnFe2O4 highlighting the octahedral Fe (blue) and tetrahedral Zn sites (green) with red oxide anions. | |
A third (much broader) subset of spinels exists where the structure is intermediate between normal and inverse, these are known as partially inverse spinels. In general, therefore, the chemical formula for a spinel ferrite may be written as [M1−iFei]TET(MiFe2−i)OCTO4 where the square and curved brackets labelled TET and OCT represent the tetrahedral and octahedral environments of the ions, respectively and i (where 0 ≤ i ≤ 1) is known as degree of inversion (with 0 indicating a normal and 1 indicating an inverse spinel ferrite structure).52
This distinction is very important because many of the particular properties which make spinel ferrites such interesting functional materials arise directly from this distribution of metal species over crystal sites.52,54–58 Many studies have been carried out on how to control the cation distribution in spinels to affect the final properties of the resulting material.52,54–56,58 While bulk ferrites have a typical degree of inversion, depending on the nature of the M metal, the value of i in nanosized ferrites may vary based upon the synthetic history of the material; in particular, low temperature synthesis may allow access to a different distribution of cations than can be accessed at high temperatures.54,58–60
A subclass of spinel ferrites includes more than one metal. These “doped” or “quaternary” ferrites MxM′1−xFe2O4 can therefore take advantage of the properties of both “parent ferrites” (MFe2O4 and M′Fe2O4).19,42,61–67 Because each M species has its own characteristic electronic structure, the oxides may feature novel or enhanced properties.68–71
This great variety of structural and compositional possibilities endows spinel ferrites with a very broad spectrum of magnetic, electric and functional properties,6,55,56,72–75 making them materials of great interest in a high number of applicative avenues. Nickel and magnesium ferrites have been reported as potential catalysts for water splitting and CO2 decomposition.25,76 The magnetic properties of spinel ferrites have furthermore attracted interest in the fields of spintronics77 and biomedicine,78–81 where they can act as either drug carriers or as foci for hyperthermia treatments. Spinels have further found applications in the fields of sensors,82,83 magnetic adsorbers,21,84 antiparasitic agents85 and as components for magnetic devices.86–88
Perovskites
The ABX3 perovskite structure is one of the most familiar and versatile found in solid-state inorganic materials.89 Named for the mineral CaTiO3, the perovskite structure is adopted by many possible combinations of cations (A and B) and anions (X). The A cation adopts coordination number 12 in the ideal cubic perovskite, while the smaller B cation adopts octahedral coordination, such that a three-dimensional network of corner-shared [BX6] octahedra form a network in which the larger A cations reside, Fig. 2. The versatility in the structure lies in its structurally flexibility: distortions of the structure are possible to accommodate a range of relative ionic sizes, the most common of which are concerted rotations of the corner-shared octahedral to maintain their connectivity, while lowering the coordination number of the A-site cations, Fig. 2b. Another often found distortion is displacement of the B-site cations within their octahedra. This can give rise to polar properties, exploited in ferroelectric devices. For ABO3 oxides, various combinations of differently charged cations are possible to ensure neutrality: commonly A3+B3+O3, A2+B4+O3 and A+B5+O3. Thus for iron in ferrites, the composition A3+Fe3+O3 is expected to be prevalent, with the expectation that replacement of A3+ by A2+ may permit partial oxidation of the Fe to the higher +4 oxidation state.
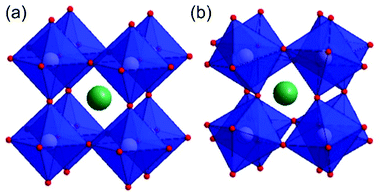 |
| Fig. 2 The ABX3 perovskite structure (a) view of ideal cubic unit cell with blue [BX6] octahedral and green 12-coordinate A sites and (b) the distorted orthorhombic unit cell as found for GdFeO3 where the octahedral are tilted with respect to each other. | |
Ferrite perovskites have attracted interest for their various properties and continue to be heavily researched. Magnetic properties have long been studied in this family, in particular in the rare-earth ferrites (so-called orthoferrites), where A is a lanthanide or Y3+ and B is Fe3+.90 Depending on the rare-earth cation the materials show complex magnetic behaviour associated with orientation of spins of each of the lanthanide and Fe3+ sub-lattices. More recently the interplay of magnetism and polar properties has emerged of being importance.91 Such multiferroicity and enhanced magnetoelectric coupling in ferrites is exemplified by BiFeO3 that has been the focus of intense investigation in this respect.92–94 The coexistence of coexistence of ferroelectricity, ferroelasticity, and ferromagnetism in a single phase is of relevance for the development of electronic devices such as in next generation data storage and BiFeO3 shows antiferromagnetism, ferroelectricity and ferroelasticity over a wide temperature range above room temperature. Photocatalysis by BiFeO3 has also been widely studied, due to its band gap being appropriate for solar light activation, and it has been thoroughly investigated for the degradation of organics in solution.95 The hexagonal ferrite perovskites of the smaller rare earths (Y, Dy–Lu) have also been recently studied for their multiferroic properties.96
Ferrite perovskites have shown promise as heterogeneous catalysts for oxidation reactions, and have been investigated for many years,97 in applications such as methanol oxidation.98,99 With an increasing interest and awareness of environmental concerns and the need for effective catalysts for clean combustion and pollution abatement, the materials continue to be developed and optimised for applications. La1−xCaxFeO3 materials have been shown to be effective for activation of the combustion of propane and ethanol, which was ascribed to the presence of Fe4+.100 A systematic study of AFeO3 (A = La, Nd, Sm) and LaFe1−xMgxO3 found that the materials gave complete conversion of methane and CO, with a correlation between structural chemistry, chemical composition and activity.101 More detailed studies of non-stoichiometry in La1−xFeO3−δ showed that composition dictates activity for hydrocarbon oxidation,102,103 while others have also suggested that the synthesis method is also important.104 Wet chemical approaches have been shown to give higher surface area for LaFeO3.105 This enables LaFeO3 to be used as a support for precious metals to perform effective oxidations.106,107
Other recent examples of catalysis applications include LaFeO3 for activating H2O2 to oxidise methyl orange,108 in three-way catalysis for automotive exhausts,109 for the conversion of CO and NO to dinitrogen,110 and a titanium-doped LaFeO3 has been used for catalytic wet peroxide oxidation.111 Electrocatalytic activity towards oxygen evolution in alkaline media has been long studied with ferrites since systematic work on La1−xSrxFeO3−δ,112,113 and has been tuned by exploring structure–property relationships in much more complex solid-solutions.114 This is of tremendous importance in contemporary applications in rechargeable batteries, fuel cells, and hydrogen production from water splitting.
Other important applications of orthoferrites are as cathode materials in solid-oxide fuel cells: here mixed ionic-electronic conductors are needed, along with catalytic activity for oxygen reduction and thermal expansion that is matched to a solid electrolyte.115,116 To meet this combination of criteria, substitutions into the parent LaFeO3 perovskite has been extensively studied to tune the electronic structure and to induce oxide-ion vacancies to provide anion conduction pathways. This includes materials co-doped with Sr and Ni, or Co.117,118 The material Nd0.5Ba0.5FeO3−δ when doped at the B-site with Cu, Ni or Co has proved to be compatible with proton-conducting electrolytes.119 Related to this, is recent work that shows how the oxygen non-stoichiometry in ferrites can be used for thermochemical air separation, making use of their intrinsic oxygen storage capacity, such as in SrFe0.95Cu0.05O3−δ,120 or the parent SrFeO3−δ.121
Hexagonal ferrites
The ABX3 composition is found in other structure types, which are often labelled as perovskites, but these do not have the essential feature of solely corner-shared octahedral [BX6] units. For example, Fig. 3a and b shows the structure of an example of a so-called ‘hexagonal perovskite’ that contains layers of corner-linked [BX6] trigonal bipyramids, separated by layers of A cations.122 This structure is found for some ferrites, notably ferrites of the small rare-earth cations, as will be discussed below.
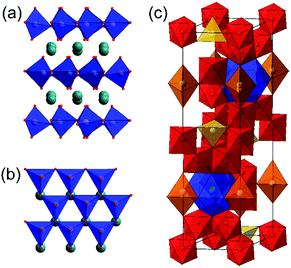 |
| Fig. 3 Example of hexagonal ferrites (a) and (b) shows the ABO3 hexagonal layered perovskite structure as found for YbFeO3 (YMnO3 type), where [BX5] trigonal bipyramids are shown in blue and layers of A cations are the green spheres: (a) view showing stacking of layers and (b) a view perpendicular to the layers. (c) shows the unit cell of the hexaferrite BaFe12O19 with three distinct iron environments: octahedral (red), tetrahedral (yellow) and pyramidal (orange) and 12-fold coordination sites for Ba (blue). | |
A second important type of hexagonal ferrite are the ‘hexaferrites’ exemplified by the barium iron oxide BaFe12O19. This phase, known as the M-type hexaferrite, has a structure containing iron in three distinct coordination environments, octahedral, tetrahedral and pyramidal, Fig. 3c. More complex hexaferrite structures are known, such as the Y-type Ba2Me2Fe12O22, where Me is a divalent metal cation, and the family is further diversified by stacking of M-type, Y-type and spinel motifs.36
The hexaferrites have long been studied for their magnetism, which find widespread technological used in hard permanent magnets in applications such as magnetic recording and data storage materials, but they are also now used as components for mobile and wireless communications at microwave per GHz frequencies, and in electromagnetic wave absorbers.1 Recent interest has been focussed on the formation of composite materials based on hexaferrites, such as with polymer matrices, and for this purpose fine powders of the oxides are needed that can be suitably surface modified.123
Green chemistry: the need for low-temperature wet synthesis approaches to ferrites
From a functional and applicative point of view, ferrites are becoming increasingly relevant. As outlined at the beginning of this review, new application avenues have been disclosed in an endeavour to enhance both the economic and the environmental sustainability of synthetic approaches, thus also complying with one of the most relevant out of the 12 paradigms of Green Chemistry:47 lowering processing time and temperature, which would allow to pursue the goal of reducing energy consumption, a goal which in turn represents one of the major targets in designing future sustainable syntheses of materials.
Lowering temperatures necessarily requires a turn to wet chemistry and solution-based routes, since temperatures below 200 °C are not sufficient to reach the activation energy required for solid state reactions, grain coalescence and coarsening, and eventually the initiation of crystallisation in solid state. In wet-chemistry routes, the limit imposed by the boiling point of the used medium or solvent inherently helps maintain milder synthesis conditions. Despite some exceptions (e.g. polyol synthesis124 and the Pechini125 method), most of the currently used wet chemistry routes affording the crystallisation of inorganic nanostructures are carried out at temperatures ranging from room temperature (e.g. mini- and microemulsion, biogenic synthesis, radiolysis, sonolysis, laser ablation, microfluidics) to 150–200 °C (sol–gel, hydro- and solvothermal, coprecipitation), only rarely exceeding them. There is still space for improvement: the experimental set-up can be optimised to increase the efficiency of the heat and mass transfer during the synthesis, as well as to reduce heat losses, making the overall process more energy efficient.
It should however be pointed out that lowering the synthesis temperature, is not the only relevant aspect involved in reducing the energy consumption: decreasing the processing time, i.e. the time for which energy must be provided to the reaction system, is also of paramount important. A systematic optimisation of the experimental landscape is needed for this aspect as well. A recent work by some of us126 has shown how the crystallisation of ferrites by an already established hydrothermal approach,127 relying on heating a suspension of oxalates at 135 °C for 24 h, could be also achieved by heating for only 1 to 3 hours (depending on the nature of the involved divalent metal cation), which implies a reduction in energy consumption, all other conditions being equal, by a factor of 24 to 8. In this work, the crystallisation of the targeted ferrites was followed, in a time-resolved fashion, by using different analytical tools sensitive to different range orders, from local/atomic (XAS, XPS) to long range (XRD, SAXS) to macroscopic scale (TEM, SEM). It could furthermore be pointed out that the nucleation and growth of the final nanostructures occurs within a very limited time frame, and no further growth occurs by increasing the heating period.
Hydro/solvothermal approaches to ferrites
The term hydrothermal refers to the use of water as a reaction medium when heated to above its boiling point in a sealed vessel. Under these conditions, autogeneous pressure is produced, and the combined role of increased temperature and pressure in the synthesis and crystallisation of solids has been long studied.128,129 It is already known how under these conditions, physical properties of water, such as viscosity, ionic product and dielectric constant, change significantly at high pressure and temperature thus enabling solubilisations, crystallisations and, possibly, chemical reactions that would not occur under normal conditions. This is particularly the case once the supercritical point is exceeded (for water Tc = 647.14 K and pc = 22.06 MPa), but even so most hydrothermal reactions currently reported use milder conditions. The term solvothermal, later coined by Demazeau,130–132 encompasses all possible solvents, with their differing boiling points, viscosities and dielectric constants, and this method widened the use of solution chemistry to allow access to a larger range of materials. These solution-based routes to solids originally developed from studies of the formation of minerals in the Earth's crust and led to synthetic silicates, exemplified by the zeolites.133 The growth of large crystals of a range of materials has benefitted from hydrothermal chemistry,134 and more recently attention has turned to the formation of polycrystalline powders of condensed solids that would usually be prepared using the high temperatures associated with solid-state chemistry.135–141 The ongoing use of hydrothermal chemistry is also driven by the discovery of new materials, with compositions and structure types not seen by other preparative routes, and that may be metastable under conventional synthesis conditions,142 as well as control over crystal form over length-scale from nanometres upwards.143 The key feature of the hydro/solvothermal method is that synthesis and crystallisation of a materials occurs in a single reaction, directly from solution, with no second step of annealing or calcination need to induce the formation of the desired phase. This then means that intricate crystal forms can be isolated, or metastable atomic-scale structure and polymorphs, that would otherwise transform or collapse at elevated temperature. Most solvothermal chemistry for materials synthesis is performed under rather mild conditions, with maximum temperature of around 250 °C, so the pressure generated is not high (typically ten times atmospheric pressure) and arguably not an important synthetic parameter, but under these conditions reagents that would otherwise be insoluble are solubilised to some extent (often with the aid of a mineraliser) to bring about crystallisation from homogeneous solution or to mediate heterogeneous reactions. While some authors report the use of solvothermal chemistry as a processing step, which is followed by a high temperature annealing rather like sol–gel chemistry, in the following sections we will focus on true solvothermal synthesis, where the advantages outlined above are at play. We have chosen examples of materials to show the versatility of the hydrothermal method: this includes a range of chemical compositions and also materials whose properties have been characterised for practical applications.
Solvothermal routes to perovskites
In this section we will review the solvothermal crystallisation of AFeO3 materials, where A = a large trivalent cation. In terms of ferrite perovskites, much work has been done on the hydrothermal crystallisation of BiFeO3, which has been the focus of attention for its multiferroicity and, more recently, its photocatalytic properties.95 There are numerous published reports of the one-step hydrothermal crystallisation of the solid in the past 12 years, and many examples are summarised in Table S1 (ESI),† which contains selected examples of the published work on this chemistry. Chen et al. in 2006 pioneered this work, reporting the phase-pure synthesis of BiFeO3 as submicron crystallites from aqueous 4 M KOH.144 The same year, Han et al. reported that, along with BiFeO3, two further bismuth ferrites could be selectively synthesised as phase-pure powders: the sillenite-structured Bi12Fe0.63O18.945 (close to the ideal composition Bi25FeO40) and Bi2Fe4O9.145 As well as phase selectivity the crystal morphology could be adjusted by temperature and/or pH as shown in Fig. 4. This illustrates the complexity in hydrothermal synthesis if competing phases form from a given set of reagents and shows how optimisation of conditions may be necessary to obtain a desired phase as a pure material.
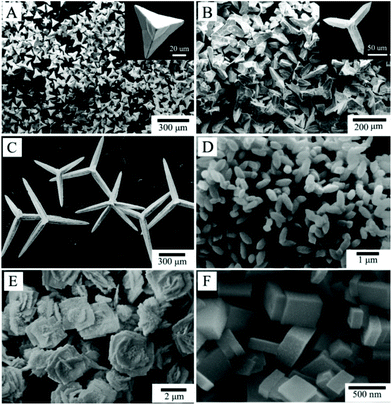 |
| Fig. 4 SEM images of bismuth ferrites prepared by hydrothermal synthesis (A) Bi12Fe0.63O18.945 (pH ∼ 12, heating temperature, 180 °C); (B) Bi12Fe0.63O18.945 (pH ∼ 10, 180 °C); (C) Bi12Fe0.63O18.945 (pH ∼ 8, 180 °C); (D) BiFeO3 (200 °C); (E) Bi2Fe4O9 (180 °C); (F) Bi2Fe4O9 (270 °C). Reprinted with permission from Han et al.145 | |
Li et al. later tuned the crystal form of BiFeO3 on the micron-scale by choice of pH and introduction of a polymer (polyethylene glycol) to the synthesis, as shown in Fig. 5.146
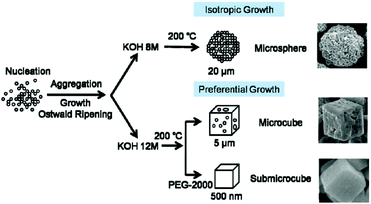 |
| Fig. 5 Crystal morphology control of BiFeO3 proposed by Li et al. and reproduced with permission.146 | |
Table S1 in ESI† shows the frequent observation of competing sillenite Bi25FeO40 and Bi2Fe4O9 materials in the hydrothermal synthesis of BiFeO3. The formation of these may depend on the Bi
:
Fe ratio used in the synthesis, but Cai et al. proposed that Bi25FeO40 is formed early in the hydrothermal reaction and reacts further to give the perovskite product; in their case, the stabilisation of the sillenite was aided using polyvinylalcohol as an additive in solution.147 Lopes et al. performed a detailed study that supported this view, showing that Bi25FeO40 could be formed at room temperature, and then under hydrothermal conditions was formed at short reactions times before the emergence of BiFeO3.148
While most of the work on BiFeO3 has used hydrothermal conditions (i.e. water as solvent) there is some evidence how the presence of a secondary solvent can influence the crystallisation and hence form of the products. For example, Chen et al. found that the addition of acetone lowered the synthesis temperature,149 while Zhang et al. found that addition of polyvinylpyrrolidone in ethanol lead to the assembly of primary crystallites into a hierarchical structure of micron diameter rods.150
Hou et al. performed hydrothermal synthesis of BiFeO3 in an applied magnetic field and found that with increasing field strength up to 12 T the crystallite size decreased from 40 μm to 20 μm and at 12 T chain-link agglomerates of the primary particles were formed, Fig. 6.151 The subsequent magnetic response of the materials was improved for the samples prepared in-field: magnetisation measured under a 1.8 T magnetic field was enhanced from 0.1003 emu g−1 (0 T) to 0.2067 emu g−1 (12 T).
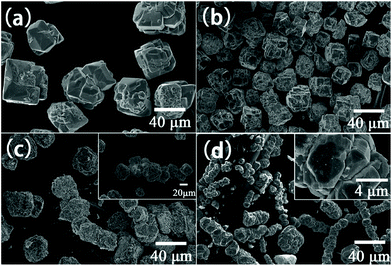 |
| Fig. 6 SEM images of BiFeO3 obtained by hydrothermal synthesis at 200 °C with 8 M KOH for 6 h in various magnetic fields: (a) 0 T; (b) 4 T; (c) 8 T and (d) 12 T. Reproduced with permission from Hou et al.151 | |
The hydrothermal method can be used to crystallise films of oxides on suitable substrates and in the case of BiFeO3, Huang et al. showed how epitaxial growth of the material on orientated surfaces of SrTiO3 was possible to give micron-thick films with favourable dielectric properties.152 This is important for the fabrication of electronic devices, but annealing is necessary to remove and achieve the dielectric properties expected for the material.
Substitutional chemistry of BiFeO3 using hydrothermal crystallisation is also possible in one-step hydrothermal reactions, and this has been done to tune dielectric and ferromagnetic properties. The advantage of the solution mediated reaction is a high homogeneity of atomic mixing, which by solid-state synthesis often requires repetitive cycles of grinding and heating to achieve. Lanthanum-doped BiFeO3 materials, Bi1−xLaxFeO3 with x up to 0.3 have been reported from hydrothermal reactions, with enhanced ferromagnetic and dielectric properties over the parent material,153,154 whilst using microwaves as the heating source allowed rapid synthesis of materials with even higher lanthanum content.155 Replacement of Bi3+ by Ba2+ is also possible,156 while co-doping with both Ca2+ and Sm3+, leads to oxide-ion vacancies as seen by XPS, which may be responsible for the high temperature dielectric anomalies observed in the material.157
The extensive work on the hydrothermal synthesis of BiFeO3, clearly illustrates its ease of implementation over a range of pH, temperature, reaction time, with some choices of reagents possible. In contrast, hydrothermal synthesis of rare-earth (lanthanide, yttrium or scandium) ferrites has received comparatively less attention. Yoshimura and co-workers reported in the 1980s that the hydrothermal synthesis of LaFeO3 required temperatures above 300 °C from lanthanum oxide and hydrated iron oxide in KOH solution, with optimum temperature 450–500 °C.158 This has been verified by Adschiri and co-workers.159 More recent work has shown how additives in solution can bring about hydrothermal crystallisation of LaFeO3 from nitrate precursors KOH solution at around 250 °C. The additives include sodium carbonate,160 urea,161,162 and while others have used citric acid, although a second step of calcination at 800 °C is needed in those cases to crystallise the materials, so these are not strictly hydrothermal reactions but more akin to a co-precipitation.163,164 Interestingly, the rare-earth ferrites of other lanthanides are more readily synthesised: this includes those of all of the elements Pr–Lu (except Pm) that crystallise at 240 °C from KOH in 24 hours without any need for extra mineralisers or additives; the materials are highly crystalline and have magnetic properties that match those prepared by conventional solid-state synthesis.165–169 In the case of YbFeO3, the choice of mineraliser, KOH, NaOH, or mixtures of, was shown to influence crystal morphology, which was rationalised by considering the interaction of solution cations with the growing phases of the crystallites: as shown in Fig. 7, one effect is to adjust the aspect ratio of the cuboid crystals that are formed.170 B-Site doping has also proved possible in the series LaFe1−xCrxO3 (0 ≤ x ≤ 1)171 and REFe0.5Cr0.5O3 (RE = La, Tb, Ho, Er, Yb, Lu and Y)172 prepared directly from hydrothermal solutions at 240 °C in aqueous KOH and their magnetic and ferroelectric properties shown to be highly dependent on composition. Similarly, the series GdFe1−xCrxO3 (0.1 ≤ x ≤ 0.9) has been prepared and characterised.173
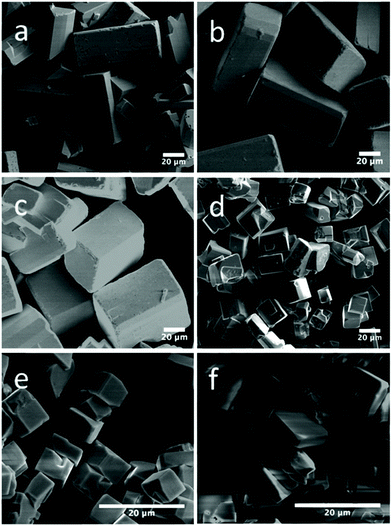 |
| Fig. 7 SEM images of orthorhombic YbFeO3 with NaOH/KOH mixed mineraliser: (a) 25 M/0 M; (b) 20 M/5 M; (c) 15 M/10 M; (d) 10 M/15 M; (e) 5 M/20 M; and (f) 0 M/25 M. Reproduced with permission from Zhang et al.170 | |
The rare-earth ferrite YFeO3 also crystallises directly from solution and although some groups have reported that higher temperatures of 300 °C are needed,174–176 others have used 240 °C and concentrated KOH.177 The fact the some of the rare-earth orthoferrites require high temperature (supercritical) hydrothermal conditions or the use of additives is not understood at present. Feng and co-workers proposed that the role of urea as additive was to release NH4+ into solution where it interacted with the growing faces of the perovskite crystals.161,178,179 Further work is needed to prove this hypothesis and to understand properly the kinetics and mechanism of crystal growth of perovskites from hydrothermal solutions.
For the smaller rare-earth cations, a hexagonal perovskite structure is also possible (see Fig. 3 above) and this may be accessed using solvothermal synthesis selectively over the orthorhombic polymorph. For example, the reaction between rare-earth (RE) acetates (RE = Tm–Lu) and iron acetylacetonate in 1,4-butanediol at 300 °C gave hexagonal REFeO3 materials consisting of submicron particles.180 Upon subsequent calcination, the hexagonal phases irreversibly transformed into the perovskite phase at around 980 °C. This is an important example of how the use of mild synthesis conditions allows accessible to a metastable polymorph that cannot be stabilised at high temperature by classical solid-state synthesis. In the case of YbFeO3, the solvothermal reaction between Yb chlorides and iron acetylacetonate in 1,4-butanediol in the presence of 1,6-hexanediamine yielded the orthorhombic perovskite YbFeO3, in contrast to the hexagonal polymorph prepared using the prior method.181,182
As well as the perovskites, it is here worth mentioning that FeTiO3, the mineral ilmenite, can be prepared via hydrothermal synthesis. Although having a perovskite-like composition, this material has a trigonal corundum structure with alternating layers of octahedral Fe2+ and Ti4+. Guan et al. prepared hexagonal plates of FeTiO3 hydrothermally and tested their electrochemical and catalytic properties,183 Zhang et al. prepared various morphologies at 180 °C, depending on reaction time and solution pH, and demonstrated shape-dependent photocatalysis for the degradation of an organic dye,184 Aparna and Sivasubramanian used a urea-aided hydrothermal method to prepare hexagonal plates (300 nm diameter) that could be as electrochemical sensors for dopamine,185 and Palanisamy et al., prepared nanoflower-shaped particles that were tested as a catalyst for electrochemical CO2 reduction.186 Tao et al. used a different approach for preparing FeTiO3 nanostructures, by ball milling commercially available FeTiO3 followed by hydrothermal treatment.187,188
Hydro/solvothermal approaches to spinels
In this section we will consider spinels consisting of divalent cations in combination with trivalent Fe. The spinels MFe2O4 (M = Mn, Co, Ni, Cu, Zn, Mg) are the most widely studied and form readily under hydrothermal conditions. Early work in the 1970s by Swaddle and co-workers used hydroxides and oxides as precursors in alkali solutions up to 350 °C.189 Milder condition are in fact needed when metal salts are used and these can give fine powders directly from solution at 150 °C, or lower.190 There are many examples of hydrothermal formation of such spinels and some selected examples will be chosen to illustrate the control that can be achieved in synthesis. Rath et al. prepared nanoparticles 9–12 nm in size of Mn0.65Zn0.35Fe2O4 from chloride precursors in ammonia solution at 180 °C: pH above 10 was optimum and the precipitation of an iron hydroxide before reaction with the other metal salts was found to be necessary to isolate the quaternary oxide.191 The work was extended to other compositions Mn1−xZnxFe2O4 (0 ≤ x ≤ 1), and the nano-sized materials were found to have a different distribution of cations over tetrahedral and octahedral sites than after annealing, as evidenced by irreversible changes in magnetic response after heating above 300 °C;192 this illustrates how the hydrothermal synthesis can allow access to metastable polymorphs of materials, not accessible by high temperature synthesis. Xia et al. prepared similar materials from nitrate salts in NaOH solution and found that only with higher Zn content could Fe2O3 impurities be avoided,193 while Nalbandian et al. used careful pH control to prepare homogeneous nanocrystalline powders, Fig. 8, that had low sintering temperatures to allow the formation of dense, fine-grained microstructures for practical application.194 Ni0.5Zn0.5Fe2O4 can also be prepared at a low temperature of 120 °C.195
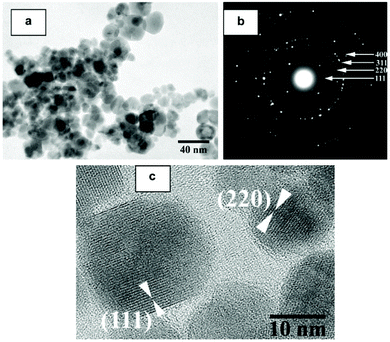 |
| Fig. 8 (a) Bright field TEM micrograph, (b) selected area diffraction and (c) HRTEM image of hydrothermally prepared nanocrystalline Mn0.8Zn0.2Fe2O4. Reprinted with permission from Nalbandian et al.194 | |
Nanorods of MnFe2O4 were prepared using pre-made nanorods of Mn2O3, demonstrating how the form of solid-state precursors might be used to direct the formation of desired morphology of multi-metal oxide via hydrothermal chemistry.196 It should be noted that MnFe2O4 could also be prepared as nanorods directly from metal chlorides treated hydrothermally in sodium hydroxides solution,197 suggesting that further work is needed to understand crystal growth mechanism.
The use of microwave heating for the hydrothermal synthesis of the spinels Co1−xZnxFe2O4 and Ni1−xZnxFe2O4 (x = 0, 0.3, 0.5, 0.7, 1) was shown to be an effective way to give 10 nm diameter crystallites in reactions as short as 10 minutes.64
Nanocrystalline Cu1−xCoxFe2O4 (0 ≤ x ≤ 1) samples were prepared hydrothermally, aided by the surfactant cetyltrimethylammonium bromide (CTAB), and studied for catalysis of the nitration of toluene: the materials with equal amounts of Cu and Co were most active and allowed the regioselective formation of 1,4-dinitrotoluene.198
Tiano et al. developed a general hydrothermal approach to MFe2O4 for M = Mg, Fe, Co, Ni, Cu, and Zn, from simple metal salt precursors in NaOH solution from which crystallite size and shape could be controlled by addition of surfactants.199 This allowed access to materials with crystallite sizes of just a few nanometres up to 10s of nanometres, and a comprehensive set of characterisation measurements allowed structure/property relationships to be established: this included application as magnetically-recoverable photocatalysts and as mimics of the enzymatic activity of peroxidases, where enhanced activity over pure iron oxides was proven. MFe2O4 with M = Co, Ni, Cu, Zn were also studied as magnetically-separable catalysts, for epoxide ring opening reactions, and hydrothermally-prepared nanoparticulate CeFe2O4 was found to be the active catalyst.200
Aside from magnetic and catalysis properties, other applications of hydrothermally prepared ferrite spinels include NiFe2O4 for the detection of liquefied petroleum gas in air,201 magneto-dielectric properties of Ni0.54Zn0.48Fe1.98O4,202 microwave absorbing materials Cox(Cu0.5Zn0.5)1−xFe2O4,203 and the absorption of dye molecules for depollution.204 The spinel β-LiFe5O8 was prepared as a nanocrystalline powder at 140 °C from FeCl3·6H2O, in equimolar amounts of LiOH·H2O and NaOH at 140 °C and their electrochemical capacity was found to be superior to other lithium ferrites.205
Diodati et al. explored a very easy, low-temperature (T = 135 °C), route based on the coprecipitation of metal precursors from an aqueous suspension containing oxalates combined with a low-temperature hydrothermal treatment.54,127
As outlined in the above section concerning green chemistry, time-resolved experiments showed that the formation of crystalline ferrites could be achieved after only 1 to 3 hours of hydrothermal processing,126 thus disclosing the possibility to implement an energy effective route to the up-scaling of these materials.
This route was further also implemented for the synthesis of quaternary spinel ferrites containing both zinc and either nickel (NixZn1−xFe2O4) or cobalt (CoxZn1−xFe2O4).59
Precursors/additives in solvothermal spinel synthesis
Further versatility can be brought to solvothermal synthesis by inclusion of dissolved species, such as salts, surfactants and co–solvents and, in this section, we will consider their benefits for the formation of spinel ferrites. The use of solution additives in solvothermal reactions provides a means to adjust crystal size and shape in the resultant product. Polyethylene glycols, available in a range of molecular weights, are convenient water-compatible solution additives that have been widely studied to control the hydrothermal crystallisation of ferrite spinels: this includes the synthesis of nanocrystalline forms of CoFe2O4,84,206 ZnxNi1−xFe2O4,207 CoxZn1−xFe2O4,208 Mn0.2Ni0.8Fe2O4,209 Ni0.5Zn0.5Fe1.5Cr0.5O4
210 MnxCo1−xFe2O4,211 MnFe2O4,212 CoCrxFe2−xO4
213 MnxNi1−xFe2O4
214 and NiFe2O4.215 The synthesis of magnetic nickel spinel ferrite NiFe2O4 nanospheres by a reverse emulsion-assisted hydrothermal process proved possible, using ethyltrimethylammonium bromide, polyoxyethylene(10)nonylphenyl ether, iso-amyl alcohol and hexane, in an aqueous medium at 120 °C.216 This lead to the formation of nanorods of oxyhydroxides and layered hydroxides at short reaction times, prior to the formation of nanospheres of the ferrite spinel.
The use of oleate complexes as reagents in solvothermal ferrite preparation has been studied and the role of the oleate can be as a surface capping agent on the resulting crystallites so to control the crystallite size and shape. A set of the ferrites MFe2O4 (M = Co, Ni, Mn, Zn) were prepared from mixed-metal (M,Fe)-oleate complexes upon solvothermal treatment in heptane at 140–180 °C: this yielded 5–6 nm crystallites coated with oleic acid.217 For CoFe2O4 the choice of solvent mixture and temperature could be used to tune the crystallite size between 5 and 12 mn.218 In the case of NiFe2O4, various alcohols and urea were investigated as solution additives to control the crystallite size and shape.219 Repko et al. prepared a precursor solution of Fe(oleate)3 and Co(oleate)2 and used this as a reagent for the solvothermal formation of nanoparticles of CoFe2O4 in water–pentanol mixtures at 180 °C, or in pentanol and 1-octanol (or toluene) at 220 °C.220 The particles could subsequently be surface modified with citrate, or with TiO2, via hydrolysis of titanium isopropoxide leading to different dispersions and separations of the nanocrystals, Fig. 9.
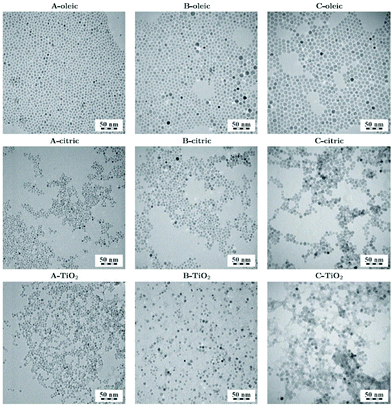 |
| Fig. 9 CoFe2O4 crystallites of three different sizes (A, B and C) coated with oleic acid, citric acid and TiO2. Reproduced with permission from Repko et al.220 | |
Angotzi et al. have recently extended the solvothermal oleate route to prepare nanoscale heterostructures that consist of CoFe2O4 and MnFe2O4 cores with shells of either spinel iron oxide (maghemite/magnetite) or manganese ferrite, added in a second solvothermal reaction, Fig. 10.221 These complex structures contain an intimate intergrowth between hard (cobalt ferrite) and soft magnetic materials with a hydrophobic coating, and provide a possible means to tune magnetic properties for practical applications.
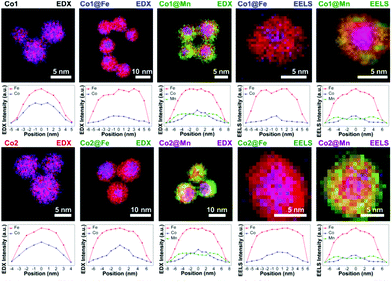 |
| Fig. 10 Energy-dispersive X-ray maps of metal cation concentration in core–shell nanocrystals of cobalt ferrite and manganese ferrite coated with a second spinel oxide, with line-scans below each map. Reproduced with permission from Angotzi et al.221 | |
Acetylacetonates provide convenient precursors for solvothermal synthesis that are soluble in organic solvents. Fe(acac)3 and M(acac)2 (M = Ni, Co, Mn, Mg, Zn) treated in water in aloe-vera extract at 200 °C for 2 hours gave agglomerated nanocrystals ranging from 8–45 nm in diameter, depending on the divalent metal.222 Fe(acac)3 has also been used as a precursor to other ferrites under solvothermal conditions, including manganese ferrites in oleylamine and phenyl ether.223 and gallium ferrites using 1,4-butanediol as solvent.224 MnxFe3−xO4 nanoparticles were prepared in various polyols from trivalent manganese and iron acetylacetonates directly from solution at 200 °C: this lead to organic coated particles that were soluble in water.225
Jolley et al. produced Mn–Fe spinels in hydrothermal reactions in the presence of proteins and found protein-encapsulated Mn-ferrites showed different crystal chemistry compared to the pristine materials: in the absence of proteins a solid-solution between γ-Fe2O3 (maghemite) and Mn3O4 (hausmannite) was found, but within proteins the Mn end-member was a chalcophanite-like layered Mn oxide.226
Heterometallic cluster species provide versatile precursors to mixed-metal oxides since the ratio of metals in the precursors can be pre-defined and intimately mixed prior to the crystal growth of the mixed oxide product. The trinuclear heterometallic acetate clusters [MFe2O(CH3CO2)6(H2O)3]·nH2O 120 °C (M = Ni, Co, Mn, and Zn) provide a good example and these can be hydrothermally decomposed at 120 °C in water, or various alcohols to yield uniform spinel ferrite nanoparticles.227 Monodispersed iron cobalt oxide (Fe2CoO4) and iron manganese oxide (Mn0.43Fe2.57O4) nanoparticles were prepared using bimetallic pivalate clusters of [Fe2CoO-(O2CtBu)6(HO2CtBu)3], [Co4Fe2O2(O2CtBu)10(MeCN)2], and [Fe2MnO(O2CtBu)6(HO2CtBu)3] using a mixture of oleylamine and oleic acid with either diphenyl ether or benzyl ether as solvent at their respective boiling points of 260 and 300 °C.228 A similar approach was adopted for the synthesis of ZnFe2O4 and NiFe2O4 from appropriate heterometallic pivalates.229
The use of non-aqueous solvents provides a way of modifying crystal growth conditions, by adjusting the solubility of reagents, adjusting diffusion, as well as modifying the pressure at a given temperature. The solvent itself may be reactive, for example, providing reducing or oxidising conditions. Deng et al. pioneered the synthesis of MnFe2O4 ferrites in non-aqueous solvothermal reactions, reporting the formation of monodisperse microspheres for M = Co, Mn, Zn from ethylene glycol with addition of sodium acetate and polyethylene glycol.230 The sodium acetate was proposed to provide electrostatic stabilisation, while the polyethylene glycol acted as a surfactant to further prevent particle agglomeration. Ethylene glycol as solvent with PEG additive allows access to some intricate and hierarchical crystal morphologies: for example, 100 nm spherical mesocrystals of Co0.5Zn0.5Fe2O4 constructed from agglomerates of primary nanocrystals.231 Wang et al. prepared hollow CoFe2O4 nanospheres using ethylene glycol as solvent and with urea as an additive, Fig. 11, and the materials were shown to have favourable properties an anodes for lithium-ion batteries.232
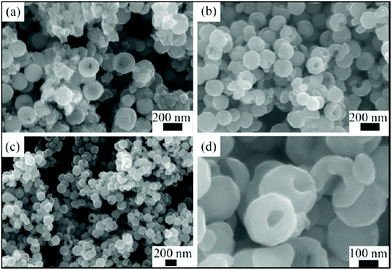 |
| Fig. 11 Scanning electron microscopy images of CoFe2O4 nanospheres prepare via hydrothermal synthesis in the presence of ethylene glycol at reaction times of (a) 6 hours, (b) and (d) 12 hours and (c) 24 hours. Reproduced with permission from Wang et al.232 | |
Otero-Lorenzo et al. recently described a comprehensive investigation of colloidal nanocrystal clusters of Fe3O4 and MnxFe3−xO4 formed in ethylene glycol using polyethylene glycol and sodium acetate as solution additives.233 This led to the conclusion that the spinel oxides formed via a series of poorly crystalline intermediates followed by the assembly of nanocrystals of spinels into the final colloidal clusters.
Continuous routes to ferrite spinels
One advantage of solvothermal routes to oxide materials is the possibility of development of continuous flow methods where solutions of reagents can be pumped through heated pipework that at controlled mixing points gives an industrially-viable production method for the production of nanomaterials at scale.234,235 In the case of ferrites, this approach has been adopted for several compositions. Cabañas and Poliakoff used a continuous hydrothermal reactor to produce various spinel ferrites including Fe3O4, MFe2O4 (M = Co, Ni, Zn) and NixCo1−xFe2O4 from mixtures of Fe(II) acetate and M(II) acetates.236 Electron microscopy TEM showed a bimodal particle size distribution with small particles of ∼10 nm and larger particles up to 100 nm, and possible reaction mechanisms were discussed, to account for the solution redox chemistry. MeFe2O4 (M = Ni, Cu, Zn) and their solid solution with γ-Fe2O3 were prepared in continuous flow at 400 °C, 30 MPa with a particle size that increased with increasing residence time.237 The particles were proposed to form via rapid formation of low-crystallinity γ-Fe2O3 followed by secondary nucleation of the solid solution on its surface with surface dissolution of the primary particles. Stingaciu et al. compared CoFe2O4 samples prepared in a continuous flow reactor with those made in batch reactors and a custom-made spiral reactor; the material made in continuous flow had the narrowest particle size distribution, while magnetic measurements showed a strong interparticle interaction.238 Co1−xNixFe2O4 (x = 0–0.8) nanoparticles were prepared from nitrate precursors in sodium hydroxide solution at 390 °C and 30 MPa: this gave 12–20 nm crystallite with high surface areas and their catalytic properties for CO oxidation and the oxygen evolution reaction were studied.239
Recently, some of us have used a continuous hydrothermal flow synthesis (CHFS) approach59 to prepare crystalline spinel quaternary ferrites MxZn1−xFe2O4 (M = Co, Ni; x = 0.2, 0.35, 0.5, 0.65, 0.8). In particular, the same materials were synthesised both through conventional batch hydrothermal synthesis (HT – as described above) at 135 °C as well as via CHFS. The as prepared compounds were thoroughly characterised from a compositional (ICP-MS, XPS) and structural (XRD) point of view in order to compare the two synthetic approaches and achieve a detailed understanding of how the chosen approach influences the characteristics of the resulting spinel. It could be concluded that CHFS requires higher temperatures and a more complex setup, but is capable of continuous synthesis, and thus can afford a greater quantity of materials over time. In contrast, the simpler HT synthesis was far easier and less time consuming to implement, despite, as a batch method, only being able to yield a limited mass of products in a given time span. Interesting differences were pointed out as far as material composition was concerned: analyses via ICP-MS evidenced that the HT protocol was more successful in affording a good stoichiometric control over the final product by simple adjustment of the nominal stoichiometric ratios between the metal precursors.
Hydro/solvothermal approaches to other ferrites
Hexagonal barium hexaferrite, BaFe12O19, is a material well studied as a hard ferrite used in magnetic recording devices, and hydrothermal routes to the material have been reported, where the aim has been to produce fine powders that can be sintered into magnetic solids.240–244 Liu et al. developed a synthesis route from Fe2+ and Fe3+ precursors, with the former being oxidised in situ but yielding samples free of Fe2O3 and BaFe2O4 impurities.245 Related hexagonal ferrites have been reported via hydrothermal routes including SrFe12O19,246 Sr1−xNdxFe12O19
247 and Sr1−xSmxFe12O19.248 It should be noted that many of the reported hydrothermal routes to the hexaferrite family involve a second step of calcination to induce crystallinity and to optimise magnetic properties, and hence these are best thought of as processing rather than synthesis routes. For example, although BaFe12O19 forms from solution at sufficiently high hydroxide ion concentration, annealing at 1000 °C is needed to improve saturation magnetisation and intrinsic coercivity.239 Recent work has examined how the particle morphology of the hexaferrites may be controlled by hydrothermal synthesis: for example, Jing et al. produced M-type SrFe12O19 directly at 220 °C as flake-like particles with magnetic behaviour that depended on grain size.249 Soria et al. studied SrFe12O19 plates formed by a similar method using a comprehensive set of characterisation methods and found a greater proportion of tetrahedral iron than in reference bulk materials.250 Dong et al. found a large magnetocrystalline anisotropy in SrFe12O19 nanosheets and measured favourable microwave absorbing properties.251
Other multinary iron oxides that have been prepared hydrothermally include the garnet phase Sm3Fe5O12
252 and the sillenite-structured Bi12Fe0.63O18.945 (close to the ideal composition Bi25FeO40) and Bi2Fe4O9.145
Sonochemistry
A relatively little-known approach, which has nevertheless gained significantly more interest in the last five years, is the sonochemical approach. Sonochemistry is based on the formation, growth and collapse of bubbles in a liquid medium (cavitation) by exposure of the system to ultrasonic waves.2,253–255 Within this context, the act of the bubbles collapsing can cause significant, as well as extremely localised, temperature increases (to around 4750 °C) with equally swift cooling (in excess of 1010 °C s−1)253 This can be taken advantage of in several ways: it can be used for instance to instantaneously decompose volatile metallorganic compounds to obtain individual metal atoms, agglomerate them and obtain nanoparticles.253 In other cases (such as many of those examined herein) the localised high temperatures can be used to effectively locally calcine a synthesised material to yield crystalline products without actually needing to bring the entire system to high temperatures;258 this locally confined calcination” provides the additional benefit that, as an extremely localised phenomenon, it does not give rise to agglomeration or coalescence phenomena in the system (unlike more conventional calcination approaches).258 Interested readers are referred to more specific works on the approach, such as those by Thompson and Doraiswamy254 or Mason and Lorimal.255
Despite requiring dedicated equipment to suitably generate the cavitation effects necessary for the reactions to take place,259 the sonochemical approach is, perhaps surprisingly, eminently scalable, able to be employed from the laboratory scale (where a simple ultrasonic rig apparatus may be sufficient)260 to high scale flow reactors;259 The technical and engineering aspects of this issue have been the subject of a work by Gogate et al.261
In general, the reports available in the literature on sonochemistry mainly cover spinel ferrites, with the cobalt, zinc and nickel spinels being the most common. Some reports exist concerning perovskite ferrites (namely BiFeO3), but in those cases a conventional calcination step was always involved to achieve crystalline materials.262,263
Concerning successful and actually low-temperature syntheses, Abbas et al.256,264 were not only able to achieve the synthesis of shape-controlled cobalt spinel ferrite (obtaining both nanocubes and nanospheres – Fig. 12), but also expanded the scope of their research to quaternary ferrites, achieving a mixed Ni/Zn spinel ferrite with controlled stoichiometry. Both these syntheses were carried out at room temperature in deionised water: the metal precursors (chlorides) were dissolved in the aqueous medium and sonicated (Vibra Cell-VCF 1500, Sonics and Materials; 5 cm2 titanium horn; 20 kHz), before adding 3 M NaOH.
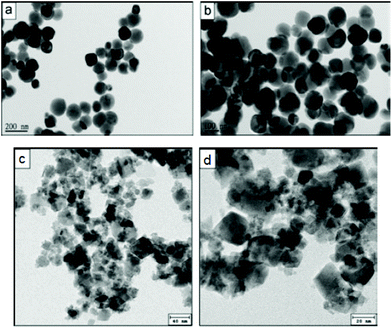 |
| Fig. 12 CoFe2O4 nanospheres (a and b) and nanocubes (c and d) synthesised in water through controlled sonochemistry at room temperature. Reproduced with permission from Abbas et al.256 | |
The effect of the confined calcination mentioned above was the subject of a study by Choudhury et al.258 whereby the chemical intermediate reactions for the formation of ZnFe2O4 from acetate precursors were hypothesised and compared to the obtained experimental results. Reactions were carried out in a conical flask and an ultrasound bath (capacity: 2.5 L, frequency: 35 kHz, Power: 35 W) was employed for sonication, generating a 1.5 bar ultrasound wave. The study concluded that transient cavitation in the reaction medium provides the energy to promote the in situ calcination of oxide particles to yield the ferrite product, with the added benefit of generating smaller size of ferrite crystals compared to conventional calcination. An earlier study by Reddy et al.265 took a more theoretical approach, by coupling experimental results of ZnFe2O4 sonochemical synthesis with simulations of the radial motion of cavitation bubbles. In particular, they concluded that the resulting particle size was mainly governed by the rate of production of OH˙ radicals.
In a more recent work,257 Chen et al. employed sonochemistry to prepare ZnFe2O4 nanocubes embellished with reduced graphene oxide for use in electrochemical sensing of biomarkers (specifically 4-nitroquinoline-N-oxide) (Fig. 13). Syntheses were carried out at room temperature in deionised water by reacting the nitrate metal precursors with ammonium hydroxide (final pH 8.0) in a 100 W, 40 kHz ultrasonic bath. The graphene oxide was then added by drop-casting.
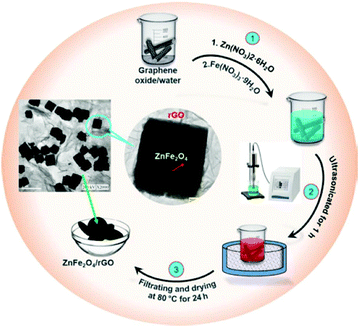 |
| Fig. 13 Reaction scheme for formation of ZnFe2O4/graphene oxide nanocomposite using sonochemistry. Reproduced with permission from Chen et al.257 | |
In work by Harzali et al.,266 the synthesis of the quinary Ni0.4Cu0.2Zn0.4Fe2O4 spinel through a combination of coprecipitation and ultrasound irradiation was described (Fig. 14). The compound was prepared from sulfate metal precursors in deionised water by adjusting the pH to 12 with NaOH and sonicating at 28.03, 38.23 or 46.27 W (equivalent to bulk reaction temperatures of 70, 90 and 100 °C, respectively). The resulting powders were crystalline after drying and required no further high temperature annealing steps.
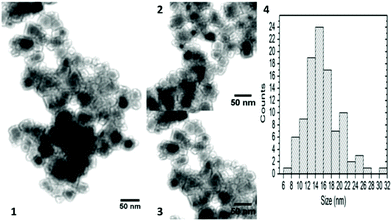 |
| Fig. 14 TEM micrographs and size histogram of Ni0.4Cu0.2Zn0.4Fe2O4 synthesised at T = 90 °C, t = 2 h and for Pdiss = 46.27 W (1, 2, and 3 represent different areas of the sample; 4 the size histogram). Reproduced with permission from Harzali et al.266 | |
Yadav et al.267 were able to prepare CoFe2−xGdxO4 with controlled gadolinium doping levels (x = 0.00, 0.05, 0.10, 0.15 and 0.20). The synthesis was carried out in deionised water at room temperature and the nitrate precursors were sonicated at 20 kHz, 70 W after basification with NaOH. It was shown that doped materials with controlled stoichiometry were afforded by the protocol and the influence of Gd content on structural, magnetic, dielectric, electrical, impedance and modulus spectroscopic features was investigated. The obtained spinels showed potential for applications in microwave and magnetic recording devices and, perhaps more importantly, the method was found to be promising for future synthesis research in the field of rare earth-doped spinel ferrites. Furthermore, the economic and environmental advantages featured by the method make it attractive for possible scaling-up at the industrial level.
Other notable works on the subject include those by Senapati et al. (CoFe2O4),268 Shafi et al. (crystalline CoFe2O4 and amorphous NiFe2O4)269,270 and Wu et al. (Fe3O4).271
Microemulsion and miniemulsion synthesis
A very convenient, albeit not easily implemented at the industrial scale, approach to pursue the crystallisation of nanoscale inorganic materials, with a certain control on size, size distribution and shape, relies on carrying out a reaction within the confined environment of micro- and miniemulsion droplets, which act as “nanoreactors”.272 This class of methods, typically based on the precipitation of the targeted oxide within the confined space of water droplets dispersed in a continuous apolar phase, as described extensively in the following, cannot be classified as fully “green” methods because of the use of organic solvents such as cyclohexane. Nevertheless, they present unique features, such as the exploration of unconventional crystallisation pathways272 and the possibility to pursue the crystallisation of the targeted oxides already at room temperature.273–275 Indeed, also in this case, as in the above described solvo- and hydrothermal routes, formation of phases which would not be affordable by high temperature routes. Micro- and miniemulsions differ not only because of their different typical droplet size, but also regarding their thermodynamics viz. kinetic stability. Microemulsions276 are thermodynamically stable colloidal dispersions, characterised by droplets with an average hydrodynamic diameter of 5–50 nm, that are spontaneously formed by mechanical stirring,277,278 whereas miniemulsions279–282 are kinetically stable systems, consisting of droplets with an average size of 30–300 nm, generated by using high shear forces.279–282 Since the synthesis of oxides is typically performed through precipitation reactions in a water medium, the formation of oxides within droplets is generally carried out in inverse (water-in-oil) emulsions (also referred to as “reverse micelles”), by mixing an emulsion containing an aqueous metal salt precursor solution with a second one containing a precipitating agent (acid/base). Whereas, in the case of microemulsions, the droplet collisions result in the fusion and mixing of the reactants, with slower characteristic exchange times compared to the diffusion of reactants in bulk conditions,283 miniemulsion droplets can instead be considered as independent nanoreactors, in which reactions run in parallel. Miniemulsion droplets are stable against diffusional degradation processes and collisions are hindered. As a consequence, in order to promote the collision and fusion of the droplets and consequently the mixing of the reactants, ultrasounds, providing the high shear forces needed for disrupting the droplets, are necessary.284 For the synthesis of ferrites, microemulsions have been broadly used, whereas only few examples concerning the use of miniemulsion to achieve ferrites have been reported. In the following section, the state-of-the-art in the micro- and miniemulsion synthesis of ferrites is summarised. It is worth highlighting that, in many cases, oxo/hydroxo metal compounds were achieved as a first product of microemulsion synthesis, which in turn needed a further thermal treatment to be converted into crystalline ferrites. Most of these examples, have deliberately not been reported in this contribution, as it is focussed on low temperature routes to ferrites.
Microemulsion and miniemulsion routes to perovskite and spinel ferrites
A very comprehensive review on the use of microemulsion for the synthesis of ferrites was authored by Mathew,34 summarising different protocol examples, typically relying on water-in-oil emulsions, based on a CTAB/1-hexanol/water system. In these routes CTAB acts as the surfactant. Different examples of the microemulsion-based synthesis of various spinel ferrites, such as zinc ferrite, nickel–zinc ferrite, manganese–zinc ferrite and cobalt ferrite, are provided. In some cases, different oil/surfactants formulations were used, as exemplified in Fig. 15.
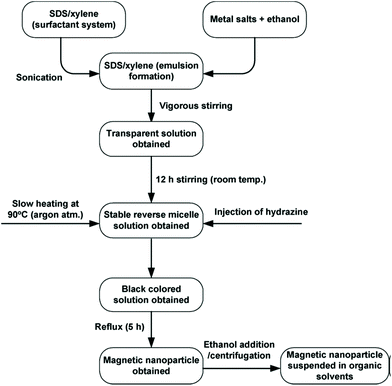 |
| Fig. 15 Flow chart of the typical experimental procedure for the micromulsion synthesis of ferrite nanoparticles (adapted with permission from Mathew et al.).34 SDS: sodium dodecyl sulphate. | |
Very recently, Scano et al.285 have also published a comprehensive review on the preparation of ferrites by microemulsions, presenting a series of advantages with respect to other methods, due to the possibility of confining reactions in nanosized reactors, allowing the preparation in mild synthesis conditions of a wide variety of nanostructured ferrites with controlled composition, particle size and shape.
In a more general work,286,287 the potential of reverse micelles as nanosized aqueous droplets, existing at certain compositions of water-in-oil microemulsions, is presented for the controlled synthesis of nanoparticles, among which also ferrites. The review firstly presents to the reader the basic concepts in the field of reverse micellar synthesis of materials; then the typical synthesis pathways and the correlations between the properties of the microemulsion reaction media (e.g. reagent concentrations, ionic strength, temperature, aging time) and the prepared materials are presented.
A further pivotal report in this regard is the work by Aubery et al.,288 which thoroughly discusses the phase behaviour, dynamics, and structure of water-in-oil (w/o) microemulsions of the aqueous solution/1-hexanol/isooctane system with the goal of determining their effect on Mn–Zn ferrite nanoparticle formation, kinetics and characteristics. The same authors,289 carried out a further fundamental systematic study devoted to investigate the formation of w/o microemulsions with high aqueous phase uptake in a non-ionic surfactant system as a reaction medium for the synthesis of Mn–Zn ferrite nanoparticles. In particular, a comprehensive study, based on the phase behaviour of systems containing precursor salts and the precipitating agent, was carried out to correlate the phase diagrams with the internal structure and dynamics of microemulsions, which in turn could be related to the final features of the resulting Mn–Zn spinel and superparamagnetic ferrites. This study must be considered relevant in this context, since it sets the knowledge base for a deeper understanding of the relationship between the final characteristics of the obtained materials with the properties of the reaction medium, i.e. the microemulsion.
The unusual conditions achieved by using microemulsions as reaction media was the topic of a further investigation by Bellusci et al.290 The authors, through a comprehensive approach, pointed out that the physico-chemical equilibria that influence the precipitation of spinel manganese ferrites from an aqueous solution can be substantially altered when the process is carried out in a microemulsion system consisting of a toluene/water/sodium dodecylbenzenesulfonate system. However, prior to the unavoidable final calcination step, only the presence of nanosized mixed hydroxide compounds could be ascertained, a result which was ascribed to a low metal content and a limited matter exchange among aqueous nanodroplets, likely inhibiting the hydroxide to oxide transformation inside the selected micellar system.
In a further work,291 MgFe2O4 was obtained using a tertiary heptane/Igepal CO 520/H2O, system and the effect of the water-to-surfactant ratio on particle size and magnetic properties was studied, whereas a similar investigation by the same authors292 focussed on the effects which changing the continuous phase had on the particle size and the magnetic properties of the resulting Mg spinel. The same compound was addressed by Holec et al.293
The effect of the experimental parameters on the final features of the resulting ferrites was also the topic of a thorough investigation by Pemartin et al.66 An oil-in-water (o/w) microemulsion was used for the preparation of Mn–Zn ferrite magnetic nanoparticles (NPs). By variating the precipitating agent and the oil phase concentration, Mn–Zn ferrite nanoparticles with different characteristics could be obtained and it could be shown that, at a fixed microemulsion composition (surfactant
:
water weight ratio (S
:
W) 25
:
75, 12 wt% oil phase), the use of NaOH as precipitating agent resulted in smaller nanoparticles compared to when tetramethylammonium hydroxide was used (2.4 nm vs 5.2 nm, respectively). Additionally, particle size was shown to increase with higher oil content (9.4 nm for 20 wt% oil), a result which was ascribed to the higher concentration of precursor in the microemulsion. In conclusion, crystallite size varied depending on microemulsion composition and precipitating agent; consequently the magnetic characteristics (blocking temperature and saturation magnetization) also changed as a function of the precipitating agent and microemulsion composition.
Different spinel ferrites, ranging from CoFe2O4,294–296 to Ni and Zn ferrites,297,298 to Li0.5Fe2.5O4,299 to Mn3+ substituted MnFe2−xMnxO4 ferrite,300 to Ni1−xZnxFe2O4 (x = 1, 0.8, 0.6, 0.5, 0.4, 0.2, 0.0),301,302 to Co0.3Cd0.7Zn1.5xFe2−xO4 (x = 0.0, 0.1, 0.2, 0.3, 0.4, 0.5),303 to NixCo(1−x)Fe2O4 (x = 0.0, 0.2, 0.4, 0.6, 0.8, 1.0),304 to CoCrFeO4,305 to MFe2O4 (M = Co, Fe, Mg, Mn, Cr, Zn) have been prepared by these routes.306
By the microemulsion route, doped spinel ferrites could also be easily achieved, as reported by Baig et al.307 for the rare earth Dy3+ substituted MnFe2O4 nanoparticles, though in this case a final heating step at 400 °C in vacuum was needed to achieve a crystalline nanomaterial.
In an interesting example of combining different synthetic routes, Antonello et al.,15 reported the synthesis of several crystalline first-row-transition-metal (Mn, Fe, Co, Ni, Cu, and Zn) ferrites prepared by a combination of miniemulsion synthesis and solvothermal treatment, pursuing unconventional conditions in terms of space confinement, temperature, and pressure. This synergy allowed to obtain the crystalline ferrites at a much lower temperature (i.e., 80 °C) than usually required and without any post-synthesis thermal treatment. X-ray diffraction revealed that analogous ferrites synthesised by miniemulsion at ambient pressure or in bulk (i.e., from an aqueous macroscopic solution and not in the confined space of the miniemulsion droplets) either at ambient pressure or under solvothermal conditions did not result in similarly highly crystalline products. Concerning their functional behaviour, the synthesised ferrites were superparamagnetic and were found to be active oxidation catalysts, as demonstrated for the oxidation of styrene, which was taken as a model reaction. Thanks to their magnetic properties, the catalysts could be recovered from the reaction medium by magnetic separation and reused for several cycles without loss in activity.
A further combination of approaches was presented by Tajik et al.,308 who prepared CuFe2O4 nanoparticles with an average particle size of 22.3 nm by a combined microemulsion and homogenous precipitation method.
In a report by Ahmad,72 the microemulsion synthesis of spinel nanoferrites having a nominal composition of Sr1−xNdxFe2−yCdyO4 (0.0 ≤ x ≤ 0.1, 0.0 ≤ y ≤ 1.0) and an average crystallite size in the 23–35 nm range is described. Quaternary ferrites were also prepared by using the microemulsion route. Ali et al.309 prepared nanosized Zr–Co doped nickel ferrites with a nominal composition, of NiZrxCoxFe2−2xO4 (x = 0.0, 0.2, 0.4, 0.6, 0.8) by using this method.
Microemulsion and miniemulsion routes to hexaferrites and further ferrites
Ali310 reported a series of complex transition metal/rare earth-based Y-type hexaferrites synthesised by the microemulsion method. Interestingly, the microemulsion route allowed controlled doping, and the effect of doping with manganese (at the tetrahedral site) and with terbium (at octahedral site) could be investigated. It was observed that changes occurred in magnetic properties (such as saturation magnetisation, coercivity, remanence and magnetic moment) due to the cationic stoichiometry and their occupancy in the specific sites.
Differences in the structural and magnetic properties of nanosized barium hexaferrite powders, prepared by single and double microemulsion techniques, were the topic of a study by Koutzarova et al.311,312 In detail, barium hexaferrite powders, featuring nanometric particle sizes, were prepared by either single or double microemulsion (water-in-oil reverse microemulsion system with cetyltrimethylammonium bromide (CTAB) as a cationic surfactant, n-butanol as a co-surfactant, n-hexanol as a continuous oil phase, and an aqueous phase) and the influence of the type of microemulsion technique on the microstructure and on the magnetic properties of the barium hexaferrite powders was studied. The type of microemulsion adopted was shown to affect, inter alia, the average particle size, the shape and the functional properties: ferrites obtained by single microemulsion featured better magnetic characteristics than those obtained by double microemulsion. It should be also pointed out that barium ferries had already been prepared by microemulsion by further authors.313
Murtaza et al.314 further employed the microemulsion approach to prepare different Nd–Mn-substituted hexaferrites having general formula Sr2−xNdxNi0.5Co1.5Fe12−yMnyO22 (x = 0.0, 0.02, 0.04, 0.06, 0.08, 0.10, 0.20, 0.30, y = 0.0, 0.25, 0.50, 0.75, 1.00, 1.25, 1.50, 1.75).
Microwave-assisted synthesis of ferrites
The liquid phase synthesis of nanomaterials using microwave-assisted heating has received considerable attention from the scientific community in the last years because it allows rapid volumetric heating, thus enabling remarkable increase of reaction rate, selectivity and yield compared to conventional approaches. This scenario offers attractive perspectives in the fabrication of materials at low cost, improving energy savings and process efficiency.
Microwaves are electromagnetic radiation with frequencies between 0.3 and 300 GHz and wavelengths ranging from 1 mm to 1 m, positioned between infrared and radio wave in the electromagnetic spectrum. For laboratory and domestic application, the usual frequency for microwave heating is 2.45 GHz, corresponding to a wavelength of 12.24 cm. In general, any material containing mobile electric charges such as polar solvents or electrolytes, can be heated by microwaves. Heat is generated by rotation, friction and collision of polar molecules such as water, which try to orientate towards the oscillating electric field (Fig. 16a). In conventional heating systems the thermal energy needs to be transferred from the heating source to the reactor, to the solvent and ultimately to the reactants, which requires large amount of time and of energy dissipation. In contrast, in microwave-based setups the heating occurs directly at the target system, thus making the use of energy sources and energy transfer media (e.g. oil bath) superfluous and remarkably improving the time and energy savings (Fig. 16b). There is an extensive number of reports in the literature showing that microwave heating largely increases the rate of chemical reactions. In order to explain the rapid temperature increase promoted by the microwave irradiation, the existence of effects called “specific microwave effects” and “non-thermal microwave effects” was postulated.315–317 “Specific microwave effects” consider that the thermal heating effect does not have repercussions on the mechanism of the chemical reaction but only influences the reaction time through the formation of hot spots in the reactor. In contrast, “non-thermal microwave effects” predict direct interactions between the molecules and the microwaves in the solution reaction. Possible implications may regard the reaction activation energy or excitement of rotational/vibrational molecular transitions.318–320
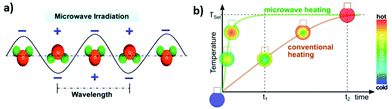 |
| Fig. 16 (a) Water molecules in an alternating electrical field under microwave irradiation (from ref. 323) (b) Time–temperature correlation of conventional and MW-heated reaction vessels as well as the temperature distribution inside the reaction mixture. Reproduced with permission from Schütz et al.324 | |
The heating generated by microwave radiation in a solvent or material is determined by the loss tangent tan
δ = δ′′/δ′, where δ′′ is the dielectric loss, i.e. the amount of radiation converted into heat, and δ′ represents the dielectric constant, which gives the polarisability of molecules in the electric field.315,321 To understand the microwave-matter interaction the penetration depth of the radiation should be also considered. This denotes the depth where the incoming microwave power is reduced to the 37%. The penetration depth is inversely proportional to the loss tangent: it grows with increasing material temperature and decreases by enhancing the radiation frequency.322 For water, which is one the most used solvent in liquid phase syntheses, due to its large availability and environmental-friendly character, the penetration depth at room temperature conditions is approx. 2 cm. Even though this value doubles at 80 °C its dielectric loss drops remarkably, complicating the microwave heating at high temperatures. However, as water is a polar solvent, is considered also a good microwave absorber. When microwave irradiated, the polarised water molecules tend to orientated following the alternating electric field (Fig. 16a). Heat is consequently produced by the rotation, friction, and collision of the molecules. The amount of time required for the molecules to relax is directly correlated to their volume and the extent of the hydrogen-bonding network. As the relaxation time depends on the loss tangent of the solvent, the denser is hydrogen-bonding network, the higher will be the heat developed by irradiation. When electrolyte solutions are considered, the relaxation time decreases at low salt concentrations and then increases again. This behaviour is explained by the initial rupture of the hydrogen bonding network. Even if some molecules are coordinated to the ions, the majority of them do not experience strong intermolecular forces and possess lower relaxation time. At high salt concentration, greater ordering of the water molecules presumably sets in, thus inducing even larger relaxation times than for pure water.321
Owing to the manifold advantages offered by water in the synthesis of nanostructured materials, numerous scientific publications have dealt with the employment of microwave radiation in combination with wet chemistry processes. In the following sections the main progress on the microwave-assisted synthesis of ferrites with spinel and perovskite structures in aqueous and other solvents will be highlighted.
Ferrites with spinel structure
The microwave heating has been combined with diverse wet chemistry procedures for the synthesis of spinel ferrites. These comprise hydrothermal, solvothermal, sol–gel and polyol methods.
Among these routes, the hydrothermal approach was the first procedure to be coupled with such electromagnetic fields. Komarneni et al.325 showed more than two decades ago how MFe2O4 with M = Mn, Co, Ni, and Zn powders could be obtained from the basic solution of the nitrate salt precursors in few minutes at 164 °C, increasing the reaction kinetics by almost two order of magnitudes with respect to conventional routes. Interestingly, the prepared materials exhibited also high porosity and specific surface areas from 72 to 247 m2 g−1, most probably due to nanoparticle aggregation during the synthesis. A few years later Kim and co-workers64 implemented the work of Komarneni et al. for the preparation of Co–Zn and Ni–Zn ferrite powders from chloride precursors at 100 °C within 30 min. The incorporation of Zn in the lattice positions of Ni and Co was verified by the lattice parameter increase proportional to the Zn amount. In the past years, the use of additives in the hydrothermal process has become common to control finely the properties of the synthesised materials. The addition of ionic liquids to the water-based reaction mixture and the subsequent microwave heating has had a remarkable influence on the crystal structure of the final products. A transition from ZnFe2O4 and α-Fe2O3 mixed phases, to pure ZnFe2O4 and pure β-FeOOH was observed by increasing amount of ionic liquid.326 The spinel structures obtained in such way exhibited also encouraging photocatalytic activity for phenol degradation. ZnFe2O4 nanoparticles were employed as catalyst precursor by Choi et al.327 for the synthesis of hydrocarbons by reducing CO2. The microwave-assisted hydrothermal synthesis produced spinel nanoparticles agglomerates with high surface area (119 m2 g−1) and improved morphology stability under reduction conditions at 400 °C. Microwave heating offers an unconventional way to prepare materials, which usually require elevated temperatures and prolonged reaction time. This is the case of layered metal oxides as sodium/potassium iron oxide nanosheets,328 prepared by microwave-assisted hydrothermal method at 180 °C within 5 minutes. The layered compounds consist of two-dimensional iron oxide building blocks separated by intercalated alkali ions (Fig. 17). Such nanosheets exhibit ferromagnetic properties due to the magnetic anisotropy of the iron oxide layers. Moreover, the lamellar structure enables high ion diffusion and thus promising application in high performance lithium-ion batteries.
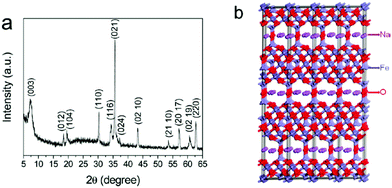 |
| Fig. 17 (a) XRD pattern of sodium iron oxide nanosheets synthesised under microwave irradiation at 180 °C for 5 min. (b) Crystal structure of Na2.4Fe10.99O16.03 viewed perpendicular to the c axis. The pink, red, and grey spheres correspond to Na, O, and Fe atoms, respectively. Reproduced with permission from Wu et al.328 | |
The application of microwave radiation for the hydrothermal synthesis of spinel ferrites resulted also in improving the materials' magnetic properties and their applications as magnetic resonance contrast agents. Thus, Williams and co-workers329 showed that microwave heating induced the formation of small and highly uniform polyelectrolyte functionalized Fe3O4 particles with much larger magnetic saturation than materials prepared by conventional hydrothermal methods. The application of electromagnetic fields allows therefore greater control over the final functionality of the ferrites for magnetic resonance applications.
One of the first examples in exploiting the benefits of the microwave radiation within solvothermal synthesis was reported by Caillot et al. in 2002
330 for the preparation of magnetite nanoparticles. Using a home-made microwave autoclave the authors succeeded in the preparation of Fe3O4 nanoparticles of 20 nm size within 10 minutes starting from ferrous chloride solution in ethanol. Compared to conventional solvothermal procedures the materials were obtained 10 times faster and with lower grain size. The use of surfactants has been implemented in solvothermal synthesis to enable better control of particle growth and morphology. The introduction of cetyltrimethylammonium bromide in an ethanol solution of iron and zinc metalorganic precursors, lead to the rapid synthesis of ZnFe2O4 at temperature below 100 °C with crystallites ranging between 8 and 20 nm, even if in the final material significant organic contaminations originated from the solvent or surfactant molecules were present.332 Diols and in general polyols are in general preferred to surfactants because their bifunctional character to act both as solvent and capping agent, limits the organic content in the reaction mixture. The 1,2-propandiol-based solvothermal synthesis of CoFe2O4 was employed to generate nanoparticles of average size of about 5 nm.333 Also in this case the materials presented organic surface contamination from the solvent, however they showed interesting magnetic behaviour. With respect to bulk and conventionally prepared systems, ferrites prepared through this microwave-assisted method possessed much larger magnetic saturation at 10 K.
The microwave-assisted synthesis of metal ferrites using other polyols, such ethylene glycol, offered an interesting tool to further control nanoparticle microstructure and morphology. Giri et al.334 were among the first to develop a microwave refluxing approach for the preparation of metal ferrites. The bifunctional role of ethylene glycol as solvent and capping agent led to phase pure Co1−xZnxFe2O4 (0 ≤ x ≤ 0.8) nanoparticles within few minutes, the crystallinity of which could be improved by increasing the pH of the starting solution. The group of Kozakova335 showed later how the combination of ethylene glycol and water could be beneficial for directing the structural properties of ferrite materials. They showed that the addition of small portions of water to ethylene glycol in a microwave-assisted environment, lead to Fe3O4 particle size reduction up to one third of the original size and to the formation of nanoparticles in superparamagnetic and ferromagnetic states.
Further works made use of larger polyols as triethylene glycol (TREG)331,336–339 as solvent. The high stabilising properties of TREG enables high dispersion of the nanoparticles without the need of further additives. Solano et al.338 compared the synthesis of MFe2O4 (M = Fe, Co, Mn, Ni, and Zn) through solvothermal and microwave-assisted routes in TREG. With both methods, particles of similar size were obtained. However, the use of microwave heating, besides shortening the reaction time, significantly influenced nanoparticle nucleation, leading to monodisperse size distribution and absence of nanoparticle aggregation (Fig. 18).
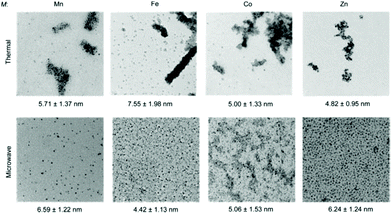 |
| Fig. 18 TEM images of MFe2O4 nanoparticles synthesized by the thermal and microwave synthetic routes. For all the investigated cases, the microwave synthesis produces highly homogeneously dispersed nanoparticles. The numbers below each image display the average nanoparticle size with its deviation. Reproduced with permission from Solano et al.331 | |
In order to better highlight whether microwaves influence the ferrite synthesis, the same authors performed a structural comparison with the solvothermal synthetic route on the same systems prepared in ref.338 using X-ray and neutron diffraction methods.331 With the only exception of ZnFe2O4, for which the microwave synthesis induced much higher inversion degree than the thermal approach, the cationic distribution microstructure and magnetic properties of the materials were very similar independent of the synthesis method used. In particular, neutron diffraction studies demonstrated the absence of H atoms and related organic matter coming from the TREG capping ligand, pointing out the improved dispersion of microwave-assisted materials. Schneider and co-workers demonstrated how the TREG-based microwave synthesis is also suitable for upscaling magnetite nanoparticles from milligram to grams.339 Even though the scalability of the nanoparticles increased their size polydispersity, most likely due temperature gradient issues in the reaction vessel, the materials were produced below 1 h and still possessed high saturation of magnetization.
Besides hydrothermal and solvothermal approaches, which represent the majority of the synthesis processes, the microwave heating for the preparation of spinel ferrites has been combined also with combustion340,341 and sol–gel methods.342–344
The magnetic properties and antimicrobial performance of NiFe2O4 nanopowders prepared with microwave-assisted combustion method were compare to those obtained via conventional approaches.340 The faster synthesis induced by the electromagnetic fields engendered nanoparticles with almost half of the size of those obtained by standard procedures. As a result, the materials possessed improved magnetic properties and antimicrobial character. In another work, conventional and microwave methods were compared using urea and citric acid fuels for the synthesis of CoFe2O4 nanopowders.341 The weaker chelating power of urea with respect to citric acid failed to deliver phase pure ferrites for both approaches. Using citric acid, microwave ignition engendered larger sphere-like ferrite particles with higher inversion degree than those obtained with the conventional approach. For these reasons, and despite small impurities of CoO and CoFe, the microwave-assisted materials also showed better magnetic properties.
The combination of microwave heating with non-aqueous sol–gels offers an intriguing chance for the rational design of spinel ferrite materials beyond the simple synthesis of nanoparticles. Niederberger and co-workers showed how the reaction of metal acetate or acetylacetonates with benzyl alcohol in presence of microwave irradiation yielded Fe3O4, CoFe2O4, MnFe2O4, and NiFe2O4 nanoparticles within 12 minutes at 170 °C.342 The introduction into the reaction mixture of glass substrates or metal nanoparticles enabled the preparation of ferrite thin films and core–shell systems, respectively (Fig. 19).
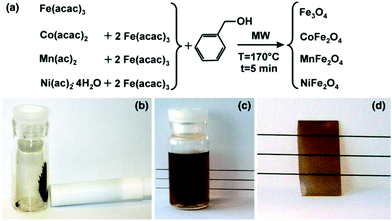 |
| Fig. 19 (a) General reaction scheme displaying the metal oxide precursors employed in the sol–gel synthesis, the solvent, the experimental conditions, and the final composition of the nanoparticles. Photographs of (b) magnetite nanopowder under the influence of a permanent magnet, (c) CoFe2O4 dispersion in ethanol, and (d) CoFe2O4 film on a glass substrate. Reproduced with permission from Bilecka et al.342 | |
This route was implemented by Suchomski et al.343 for the synthesis of monodispersed ZnFe2O4 nanoparticles of spherical shape. Detailed structural and magnetic characterization evidenced an inversion degree of 68% and the presence of a superspin glass state with freezing temperature of about 22 K. In addition, the materials exhibited promising performance as anode material for Li-ion battery with little capacity fading after hundreds of cycles.
Furthermore, the coating capability of the microwave-assisted sol–gel approach has been used for the preparation of ordered macroporous magnetic MnFe2O4 architectures through nanoparticle deposition on sacrificial colloidal crystals.344 The control of synthesis parameters such reaction time enabled tuning of the coating thickness and layer homogeneity.
Ferrites with perovskite structure
In contrast to the spinel structure, perovskites are characterised by large size difference between the A and B cations. This has strong repercussions on their synthesis conditions, such that perovskite systems tend to require higher temperatures for their formation compared to spinels.346 Therefore, the low temperature synthesis of ferrites with the perovskite structure is less common. The main contributions in the literature on this field regard mainly BiFeO3. In combination with microwave irradiation, the hydrothermal method represents the principal low temperature synthetic approach. To ensure homogeneous cationic intermixing, avoid the formation of multiphase impurities, and control the particle morphology, polyelectrolytes or surfactants are generally employed in the reaction mixture. Chybczynska and co-workers showed how the petal size and crystallinity of BiFeO3 flower-like particles increased with the amount of polyethylene glycol used in the microwave-assisted hydrothermal synthesis, which was directly correlated with a decrease of the magnetic properties.347 Besides its interesting magnetic properties, BiFeO3 is a well-known photocatalyst. With respect to conventional hydrothermal method, the preparation of bismuth ferrite with the assistance of microwave heating resulted in materials with higher crystallinity and specific surface area, ascribed to non-thermal effects of microwave during the synthesis.348 As a result, the materials possessed better photocatalytic properties for the photodegradation of organic dyes. Similar photocatalytic tests were performed by Huang et al.349 on BiFeO3 materials prepared by a microwave-assisted hydrothermal approach using different surfactants molecules as capping agents. The employment of polyvinylpirrolidone instead of EDTA engendered materials with almost four times higher specific surface area, which lead to a significant improvement of the material's photocatalytic performance.
The perovskite structure is well-known for its structural flexibility enabling the incorporation of substitutional metal ions. However, there are very few examples of doped perovskite ferrites in literature obtained by low temperature approaches with the assistance of electromagnetic heating. Ultrathin (∼2.5 nm) Cr-doped BiFeO3 ferroelectric films were obtained by hydrothermal approach without any use of mineralizer.350 Due to the microwave-assisted approach, after only 2 cycles irradiation, atomic step terraces were observed on the films indicating the high ordered growth of the film. The resistive switching behaviour of the films was confirmed by electrical characterization and thermoionic emission was demonstrated to be the dominating charge transport behaviour. Ponzoni et al.155 showed how the preparation of A-site substituted Bi1−xLaxFeO3 up to x = 0.45 could be remarkably accelerated using microwave-assisted hydrothermal synthesis with respect to the conventional method. In a further, report solid solutions of SrFeO3 and SrTiO3 in form of nanocubes could be prepared at 140 °C and a constant pressure of 3 bar by a microwave heated hydrothermal approach (Fig. 20).345 X-ray and UV spectroscopies revealed that the Fe2+/Fe3+ ions occupy the Ti4+ sites and that the band gap progressively decreases with the iron amount. In the same work, this synthetic route was also used to prepare films by the tape casting or the suspension methodology.
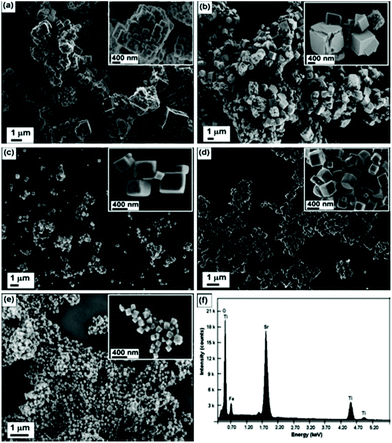 |
| Fig. 20 SEM images of SrTi1−xFexO3 samples prepared via microwave-assisted hydrothermal method: x = 0.0 (a), 0.05 (b), 0.1 (c), 0.25 (d), 0.4 (e); (f) EDX spectrum of the x = 0.25 sample Reproduced with permission from da Silva et al.345 | |
Other approaches
In this section we will provide examples of synthesis approaches that are not sufficiently featured in the literature to merit their own section. Broadly, they belong to one of two subsets: (i) methods that are not particularly popular (generally because other, more widespread approaches are considered preferable and/or less complex) and (ii) methods that generally involve a high-temperature calcination step and therefore only feature very few examples that may qualify as low-temperature (coprecipitation353 is one of these latter approaches).
Biosynthesis, includes syntheses where the chemical reactions are aided by biological systems (e.g. by having bacteria reduce metal cations through anaerobic respiration).351 Byrne et al.351 synthesised zinc-substituted magnetite ZnxFe3−xO4 (x = 0.00, 0.16, 0.42, 0.56, 0.92) through bacterially-assisted synthesis whereby zinc and iron oxyhydroxides were initially coprecipitated from an aqueous solution and then were inoculated with a culture of Geobacter sulfurreducens (0.2 mg mL−1 protein) and acetate (acting as electron donor for the reduction) and incubated in the dark at 30 °C. Crystalline nanoparticles (Fig. 21) with enhanced magnetic properties were thus obtained.
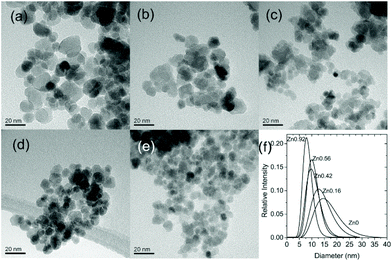 |
| Fig. 21 TEM images showing (a) Zn0, (b) Zn0.16, (c) Zn0.42, (d) Zn0.56, (e) Zn0.92, and (f) size distributions of all samples prepared through bacterially-assisted synthesis. Data obtained by measurement of n = 200 particle diameters per sample. Reproduced from Byrne et al.351 | |
Similarly Céspedes et al.61 employed bacterial synthesis to prepare several different Zn- or Co-doped ferrite spinel nanoparticles with citric acid coating for applications in magnetic hyperthermia therapy MxFe3−xO4 (M = Co, Zn; x = 0.00, 0.16, 0.42, 0.71). As described by Byrne et al., Fe and M oxohydroxide mixtures were coprecipitated from an aqueous medium and then treated with a Geobacter sulfurreducens culture. It was found that the stoichiometry of M in the final ferrite could be controlled by varying the concentration of M in the M–FeIII-oxyhydroxide intermediate species.
Whereas ball-milling is a well-known top-down dry synthesis method for ferrites,354–357 Chen et al.358,359 combined ball-milling and ultrasound technology in an aqueous suspension to obtain nickel and manganese spinel ferrite nanoparticles. In both cases the M precursors (either MnO2 or NiCO3·2Ni(OH)2·4H2O) were milled at room temperature in 1000 ml of ultrapure water together with the iron precursor (either metallic iron powder or iron coming directly from pure iron milling balls). The diameter of the milling balls was 1.0–1.5 mm and a ball to powder mass ratio was 100
:
1. The rotation speed of stirrer was 235 rpm, and the ultrasonic intensity and power were 40 kHz and 200 W, respectively.
The sol–gel auto-combustion approach (and the citrate method in particular), is widely known and applied in the field of ferrite synthesis,360–363 but in most cases either the auto-combustion process must be triggered at high temperatures, or a calcination step is then employed to reach crystallisation. By contrast, Xiao et al.352 achieved the cobalt spinel CoFe2O4 by a modified citrate sol–gel approach, affording auto-combustion and formation of crystalline cobalt ferrite powder already at 200 °C (Fig. 22). The synthesis, from an aqueous mixture of citric acid and metal nitrates was stirred at pH 6 until a sol formed. This sol was changed to a gel at 80 °C and into a xerogel at 120 °C. Finally, auto-combustion was triggered at 200 °C yielding the ferrite powder. The combustion was proven to be a thermally induced anionic redox reaction, with carboxyl group as reductant and nitrate ions as oxidant.
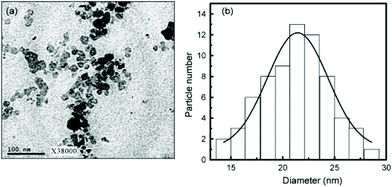 |
| Fig. 22 Cobalt spinel CoFe2O4 synthesised by a modified citrate sol–gel approach: TEM morphology of the as-burnt powder (a) and corresponding grain size histogram obtained by statistical method (b). Reproduced with permission from Gass et al.352 | |
Conclusions
We have collected and reviewed recent contributions concerning different wet-chemistry routes, having as common factor the use of low temperature, for the synthesis of different classes of ferrites, focussing largely on materials belonging to the spinel, perovskite and hexaferrite families. The discussion of the different examples reported illustrates that this relevant family of functional materials can be also addressed by relatively environmentally friendly and, in many cases, simple to implement solution- or suspension-based synthesis approaches. As well as complying with the principles of ‘green chemistry’ the solution-mediated synthetic methods that we have surveyed offer the prospect of industrially-scalable manufacture of materials, as illustrated by the implementation of continuous flow reactors that can feasibly allow efficient production of suitable volumes of powdered ferrites. From the fundamental chemistry point of view, an advantage of the solution synthesis of mixed-metal oxides can lie in the control of distributions of substituent metal cations in solid solutions or during isomorphous replacement. This may allow formation of atomically homogeneous materials, or, on the other hand, the intentional construction of core–shell particles, thus allowing tuning of properties beyond chemical composition and crystal structure. A second benefit of solution crystallisation lies in the possibility of control of crystal morphology with size from the nanoscale to the micron-scale and shape from isotropic spheres and cubes, to highly isotropic rods and plates and multifaceted intricate structures. This can provide novel materials, for example, in the study of nanoscale confinement effects in electronic and magnetic properties, or for use as additives in composites, as well as with surface properties tuned for catalysis.
We hope that our collection of examples will promote further research in this fascinating field, which represents an exciting playground for inorganic materials synthesis. The implementation of syntheses for pursuing anisotropy and shape-control in crystal form is particularly appealing, which is relevant for many applications, such as in heterogeneous catalysis, nanocomposite formation and nanotechnology, as well as in processing of powders towards real applications. Hydrothermal and solvothermal methods uniquely allow the direct formation of oxide materials from solution under very mild conditions, without the use of the extreme temperatures needed for classical oxide preparation. While this is highly advantageous for particle growth, this may mean that only certain compositions and structure are accessible since crystallisation is under strictly kinetic control, without forcing high temperatures to reach all possible stoichiometries. This may be a limitation of soft-chemical methods in general, but on the other hand metastable compositions or structures may be accessible that do not survive extreme temperatures, such as the hexagonal REFeO3 perovskites that irreversibly transform on heating to the classical perovskite structure. The occurrence of competing phases from a given reagent mixture as a function if temperature, time or pH is a further complication when exploring new reaction conditions, as was illustrated in the case of BiFeO3.
Predictability of synthetic chemistry and true control of crystal morphology remains in its infancy but as we have illustrated, with the exploration of a wide variety of solvents and solution additives, progress is being made to allow desired particle size and shape in mixed-oxides to be achieved. The examples we have selected from the literature show how particle morphology can be tuned from the nanoscale to the microscale from highly facetted to anisotropic crystals, but for most of these cases the mechanism of crystal engineering is unknown and not even speculated. Much further work is needed to establish crystal growth mechanism, and this must include both experimental and computation approaches. This leads to the possibility of tailor-made materials with desirable functional properties selected by synthetic chemistry in convenient and scalable processes.
Conflicts of interest
There are no conflicts to declare.
References
- S. Hazra and N. N. Ghosh, Preparation of nanoferrites and their applications, J. Nanosci. Nanotechnol., 2014, 14, 1983–2000 CrossRef CAS PubMed.
- M. K. Singh, Y. Yang and C. G. Takoudis, Synthesis of multifunctional multiferroic materials from metalorganics, Coord. Chem. Rev., 2009, 253, 2920–2934 CrossRef CAS.
- K. K. Kefeni, T. A. M. Msagati and B. B. Mamba, Ferrite nanoparticles: Synthesis, characterisation and applications in electronic device, Mater. Sci. Eng., B, 2017, 215, 37–55 CrossRef CAS.
- E. Casbeer, V. K. Sharma and X.-Z. Li, Synthesis and photocatalytic activity of ferrites under visible light: A review, Sep. Purif. Technol., 2012, 87, 1–14 CrossRef CAS.
- I. H. Gul and A. Maqsood, Structural, magnetic and electrical properties of cobalt ferrites prepared by the sol–gel route, J. Alloys Compd., 2008, 465, 227–231 CrossRef CAS.
- S. Jauhar, J. Kaur, A. Goyal and S. Singhal, Tuning the properties of cobalt ferrite: a road towards diverse applications, RSC Adv., 2016, 6, 97694–97719 RSC.
-
Y.-K. Hong and J. Lee, in Recent Advances in Magnetic Insulators – From Spintronics to Microwave Applications, ed. M. Wu and A. Hoffmann, Academic Press, 2013, vol. 64, pp. 237–329 Search PubMed.
- I. V. Lisnevskaya, I. A. Bobrova and T. G. Lupeiko, Synthesis of magnetic and multiferroic materials from polyvinyl alcohol-based gels, J. Magn. Magn. Mater., 2016, 397, 86–95 CrossRef CAS.
- N. R. Ram, M. Prakash, U. Naresh, N. S. Kumar, T. S. Sarmash, T. Subbarao, R. J. Kumar, G. R. Kumar and K. C. B. Naidu, Review on magnetocaloric effect and materials, J. Supercond. Novel Magn., 2018, 31, 1971–1979 CrossRef CAS.
- T. Hyeon, Chemical synthesis of magnetic nanoparticles, Chem. Commun., 2003, 927–934, 10.1039/B207789B.
- V. S. R. Kalluri, S. Melad, S. Aanchal and S. Gaddipati, Catalytic activity of functionalized spinels, Curr. Org. Chem., 2017, 21, 2573–2584 Search PubMed.
- R. Hudson, Y. Feng, R. S. Varma and A. Moores, Bare magnetic nanoparticles: sustainable synthesis and applications in catalytic organic transformations, Green Chem., 2014, 16, 4493–4505 RSC.
- G. Kaur, P. Devi, S. Thakur, A. Kumar, R. Chandel and B. Banerjee, Magnetically separable transition metal ferrites: Versatile heterogeneous nano-catalysts for the synthesis of diverse bioactive heterocycles, ChemistrySelect, 2019, 4, 2181–2199 CrossRef CAS.
- O. Vozniuk, T. Tabanelli, N. Tanchoux, M. J.-M. Millet, S. Albonetti, F. Di Renzo and F. Cavani, Mixed-oxide catalysts with spinel structure for the valorization of biomass: The chemical-loop reforming of bioethanol, Catalysts, 2018, 8, 332 CrossRef.
- A. Antonello, G. Jakob, P. Dolcet, R. Momper, M. Kokkinopoulou, K. Landfester, R. Muñoz-Espí and S. Gross, Synergy of miniemulsion and solvothermal conditions for the low-temperature crystallization of magnetic anostructured transition-metal ferrites, Chem. Mater., 2017, 29, 985–997 CrossRef CAS.
- F. de Lira, M. de Souza Farias, A. de Figueiredo, F. dos Santos Gil, M. dos Santos, B. Malheiros, J. Ferreira, J. Pinheiro, O. Treu-Filho and R. Kondo, Quantum chemical modeling of perovskite: An investigation of piezoelectricity in ferrite of yttrium, J. Mol. Model., 2011, 17, 1621–1624 CrossRef CAS PubMed.
- Q.-H. Jiang, C.-W. Nan and Z.-J. Shen, Synthesis and Properties of Multiferroic La-Modified BiFeO3 Ceramics, J. Am. Ceram. Soc., 2006, 89, 2123–2127 CrossRef CAS.
- H. Shokrollahi and L. Avazpour, Influence of intrinsic parameters on the particle size of magnetic spinel nanoparticles synthesized by wet chemical methods, Particuology, 2016, 26, 32–39 CrossRef CAS.
- R. Masrour, M. Hamedoun and A. Benyoussef, Magnetic properties of B and AB-spinels Zn1−xMxFe2O4 (M=Ni, Mg) materials, J. Alloys Compd., 2010, 503, 299–302 CrossRef CAS.
- A. H. Latham and M. E. Williams, Controlling transport and chemical functionality of magnetic nanoparticles, Acc. Chem. Res., 2008, 41, 411–420 CrossRef CAS PubMed.
- D. H. K. Reddy and Y.-S. Yun, Spinel ferrite magnetic adsorbents: Alternative future materials for water purification?, Coord. Chem. Rev., 2016, 315, 90–111 CrossRef CAS.
- E. A. Périgo, G. Hemery, O. Sandre, D. Ortega, E. Garaio, F. Plazaola and F. J. Teran, Fundamentals and advances in magnetic hyperthermia, Appl. Phys. Rev., 2015, 2, 041302 Search PubMed.
- I. Sharifi, H. Shokrollahi and S. Amiri, Ferrite-based magnetic nanofluids used in hyperthermia applications, J. Magn. Magn. Mater., 2012, 324, 903–915 CrossRef CAS.
- T. Gao, Z. Chen, Q. Huang, F. Niu, X. Huang, L. Qin and Y. Huang, A review: Preparation of bismuth ferrite nanoparticles and its applications in visible-light induced photocatalyses, Rev. Adv. Mater. Sci., 2015, 40, 97–109 CAS.
- S. Chandrasekaran, C. Bowen, P. Zhang, Z. Li, Q. Yuan, X. Ren and L. Deng, Spinel photocatalysts for environmental remediation, hydrogen generation, CO2 reduction and photoelectrochemical water splitting, J. Mater. Chem. A, 2018, 6, 11078–11104 RSC.
- S.-M. Lam, J.-C. Sin and A. R. Mohamed, A newly emerging visible light-responsive BiFeO3 perovskite for photocatalytic applications: A mini review, Mater. Res. Bull., 2017, 90, 15–30 CrossRef CAS.
- B. Zhang, J. Zhang and F. Chen, Preparation and characterization of magnetic TiO2/ZnFe2O4 photocatalysts by a sol–gel method, Res. Chem. Intermed., 2008, 34, 375–380 CrossRef CAS.
- A. Becker, K. Kirchberg and R. Marschall, Magnesium ferrite (MgFe2O4) nanoparticles for photocatalytic antibiotics degradation, Z. Phys. Chem., 2020, 234, 645–654 CAS.
- K. Kirchberg, A. Becker, A. Bloesser, T. Weller, J. Timm, C. Suchomski and R. Marschall, Stabilization of monodisperse, phase-pure MgFe2O4 nanoparticles in aqueous and nonaqueous media and their photocatalytic behavior, J. Phys. Chem. C, 2017, 121, 27126–27138 CrossRef CAS.
- K. Kirchberg, S. Wang, L. Wang and R. Marschall, Mesoporous ZnFe2O4 photoanodes with template-tailored mesopores and temperature-dependent photocurrents, ChemPhysChem, 2018, 19, 2313–2320 CrossRef CAS PubMed.
- K. Kirchberg and R. Marschall, Sol–gel synthesis of mesoporous CaFe2O4 photocathodes with hierarchical pore morphology, Sustainable Energy Fuels, 2019, 3, 1150–1153 RSC.
-
R. Tilley, Understanding Solids - The Science of Materials, J. Wiley & Sons, Chichester, West Sussex, England, 2004 Search PubMed.
- A. Hajalilou and S. A. Mazlan, A review on preparation techniques for synthesis of nanocrystalline soft magnetic ferrites and investigation on the effects of microstructure features on magnetic properties, Appl. Phys. A: Mater. Sci. Process., 2016, 122, 680 CrossRef.
- D. S. Mathew and R.-S. Juang, An overview of the structure and magnetism of spinel ferrite nanoparticles and their synthesis in microemulsions, Chem. Eng. J., 2007, 129, 51–65 CrossRef CAS.
- D. Cruickshank, 1–2 GHz dielectrics and ferrites: overview and perspectives, J. Eur. Ceram. Soc., 2003, 23, 2721–2726 CrossRef CAS.
- R. C. Pullar, Hexagonal ferrites: A review of the synthesis, properties and applications of hexaferrite ceramics, Prog. Mater. Sci., 2012, 57, 1191–1334 CrossRef CAS.
- J. P. Jolivet, C. Chaneac, P. Prene, L. Vayssieres and E. Tronc, Wet chemistry of spinel iron oxide particles, J. Phys. IV, 1997, 7, C1-573–C1-576 CrossRef.
- R. Kesavamoorthi and C. R. Raja, Studies on the properties of manganese substituted nickel ferrite nanoparticles, J. Supercond. Novel Magn., 2016, 29, 2729–2734 CrossRef CAS.
- J.-B. Moussy, From epitaxial growth of ferrite thin films to spin-polarized tunnelling, J. Phys. D: Appl. Phys., 2013, 46, 143001 CrossRef.
- T.-D. Nguyen, From formation mechanisms to synthetic methods toward shape-controlled oxide nanoparticles, Nanoscale, 2013, 5, 9455–9482 RSC.
- V. C. B. Pegoretti, P. R. C. Couceiro, C. M. Goncalves, M. d. F. F. Lelis and J. D. Fabris, Preparation and characterization of tin-doped spinel ferrite, J. Alloys Compd., 2010, 505, 125–129 CrossRef CAS.
- P. Raju and S. R. Murthy, Preparation and characterization of Ni–Zn ferrite + polymer nanocomposites using mechanical milling method, Appl. Nanosci., 2013, 3, 469–475 CrossRef CAS.
- H. H. Sønsteby, H. Fjellvåg and O. Nilsen, Functional perovskites by atomic layer deposition – An overview, Adv. Mater. Interfaces, 2017, 4, 1600903 CrossRef.
- R. Safi and H. Shokrollahi, Physics, chemistry and synthesis methods of nanostructured bismuth ferrite (BiFeO3) as a ferroelectro-magnetic material, Prog. Solid State Chem., 2012, 40, 6–15 CrossRef CAS.
- M. Sugimoto, The past, present, and future of ferrites, J. Am. Ceram. Soc., 1999, 82, 269–280 CrossRef CAS.
- V. Mameli, M. S. Angotzi, C. Cara and C. Cannas, Liquid Phase Synthesis of Nanostructured Spinel Ferrites A-Review, J. Nanosci. Nanotechnol., 2019, 19, 4857–4887 CrossRef PubMed.
-
P. T. Anastas and J. C. Warner, Green Chemistry Theory and Practice, Oxford University Press, New York, Paperback Ed., 2000 Search PubMed.
-
P. A. Cox, Transition Metal Oxides : Introduction to their Electronic Structure and Properties, Oxford University Press, Oxford, U.K., 1995 Search PubMed.
-
G. F. Dionne, Magnetic oxides, Springer, London, 1st edn, 2009 Search PubMed.
-
N. N. Greenwood and A. Earnshaw, Chemistry of the Elements, Pergamon Press, India, 2nd edn, 1998 Search PubMed.
-
A. F. Holleman and E. Wieberg, Lehrbuch der Anorganischen Chemie, Walter de Gruyter, New York, 101th edn, 1995 Search PubMed.
- D. Carta, M. F. Casula, A. Falqui, D. Loche, G. Mountjoy, C. Sangregorio and A. Corrias, A Structural and Magnetic Investigation of the Inversion Degree in Ferrite Nanocrystals MFe2O4 (M = Mn, Co, Ni), J. Phys. Chem. C, 2009, 113, 8606–8615 CrossRef CAS.
- V. Blanco-Gutiérrez, M. J. Torralvo, R. Sáez-Puche and P. Bonville, Magnetic properties of solvothermally synthesized ZnFe2O4 nanoparticles, J. Phys.: Conf. Ser., 2010, 200, 072013 CrossRef.
- P. Dolcet, K. Kirchberg, A. Antonello, C. Suchomski, R. Marschall, S. Diodati, R. Muñoz-Espí, K. Landfester and S. Gross, Exploring wet chemistry approaches to ZnFe2O4 spinel ferrite nanoparticles with different inversion degrees: a comparative study, Inorg. Chem. Front., 2019, 6, 1527–1534 RSC.
- L. I. Granone, R. Dillert, P. Heitjans and D. W. Bahnemann, Effect of the degree of inversion on the electrical conductivity of spinel ZnFe2O4, ChemistrySelect, 2019, 4, 1232–1239 CrossRef CAS.
- L. I. Granone, A. C. Ulpe, L. Robben, S. Klimke, M. Jahns, F. Renz, T. M. Gesing, T. Bredow, R. Dillert and D. W. Bahnemann, Effect of the degree of inversion on optical properties of spinel ZnFe2O4, Phys. Chem. Chem. Phys., 2018, 20, 28267–28278 RSC.
- P. Rajagiri, B. N. Sahu, N. Venkataramani, S. Prasad and R. Krishnan, Effect of substrate temperature on magnetic properties of MnFe2O4 thin films, AIP Adv., 2018, 8, 056112 CrossRef.
- J. Jacob and M. A. Khadar, Investigation of mixed spinel structure of nanostructured nickel ferrite, J. Appl. Phys., 2010, 107, 114310 CrossRef.
- M. Bastianello, S. Diodati, N. Dengo, L. McCafferty, C. Footer, D. Badocco, P. Pastore, J. Darr and S. Gross, Quaternary ferrites by batch and continuous flow hydrothermal synthesis: a comparison, CrystEngComm, 2019, 21, 6801–6809 RSC.
- M. Bastianello, S. Gross and M. T. Elm, Thermal stability, electrochemical and structural characterization of hydrothermally synthesised cobalt ferrite (CoFe2O4), RSC Adv., 2019, 9, 33282–33289 RSC.
- E. Céspedes, J. M. Byrne, N. Farrow, S. Moise, V. S. Coker, M. Bencsik, J. R. Lloyd and N. D. Telling, Bacterially synthesized ferrite nanoparticles for magnetic hyperthermia applications, Nanoscale, 2014, 6, 12958–12970 RSC.
- G. Fan, J. Tong and F. Li, Visible-light-induced photocatalyst based on cobalt-doped zinc ferrite nanocrystals, Ind. Eng. Chem. Res., 2012, 51, 13639–13647 CrossRef CAS.
- C. M. B. Henderson, J. M. Charnock and D. A. Plant, Cation occupancies in Mg, Co, Ni, Zn, Al ferrite spinels: a multi-element EXAFS study, J. Phys.: Condens. Matter, 2007, 19, 076214 CrossRef CAS PubMed.
- C.-K. Kim, J.-H. Lee, S. Katoh, R. Murakami and M. Yoshimura, Synthesis of Co-, Co-Zn and Ni-Zn ferrite powders by the microwave-hydrothermal method, Mater. Res. Bull., 2001, 36, 2241–2250 CrossRef CAS.
- M. Maletin, E. G. Moshopoulou, A. G. Kontos, E. Devlin, A. Delimitis, V. T. Zaspalis, L. Nalbandian and V. V. Srdic, Synthesis and structural characterization of In-doped ZnFe2O4 nanoparticles, J. Eur. Ceram. Soc., 2007, 27, 4391–4394 CrossRef CAS.
- K. Pemartin, C. Solans, J. Alvarez-Quintana and M. Sanchez-Dominguez, Synthesis of Mn–Zn ferrite nanoparticles by the oil-in-water microemulsion reaction method, Colloids Surf., A, 2014, 451, 161–171 CrossRef CAS.
- Y. Zhang and D. Wen, Infrared emission properties of RE (RE=La, Ce, Pr, Nd, Sm, Eu, Gd, Tb, and Dy) and Mn co-doped Co0.6Zn0.4Fe2O4 ferrites, Mater. Chem. Phys., 2012, 131, 575–580 CrossRef CAS.
- R. Mohan, M. P. Ghosh and S. Mukherjee, Size dependent exchange bias in single-phase Zn0.3Ni0.7Fe2O4 ferrite nanoparticles, J. Magn. Magn. Mater., 2018, 458, 193–199 CrossRef CAS.
- A. Yadav and D. Varshney, Structural and temperature dependent dielectric behavior of Cr and Zn doped MnFe2O4 nano ferrites, Superlattices Microstruct., 2018, 113, 153–159 CrossRef CAS.
- C. Virlan, F. Tudorache and A. Pui, Increased sensibility of mixed ferrite humidity sensors by subsequent heat treatment, Int. J. Appl. Ceram. Technol., 2017, 14, 1174–1182 CrossRef CAS.
- J.-S. Kim, J.-R. Ahn, C. W. Lee, Y. Murakami and D. Shindo, Morphological properties of ultra-fine (Ni,Zn)-ferrites and their ability to decompose CO2, J. Mater. Chem., 2001, 11, 3373–3376 RSC.
- I. Ahmad, S. M. Shah, M. N. Ashiq and R. A. Khan, Effect of Nd3+ and Cd2+ ions co-substitution on the dielectric and electron transport properties of spinel strontium nanoferrites, Ceram. Int., 2016, 42, 12763–12770 CrossRef CAS.
- A. K. M. Akther Hossain, M. Seki, T. Kawai and H. Tabata, Colossal magnetoresistance in spinel type Zn1−xNixFe2O4, J. Appl. Phys., 2004, 96, 1273–1275 CrossRef CAS.
- A. S. Albuquerque, J. D. Ardisson, W. A. A. Macedo, J. L. López, R. Paniago and A. I. C. Persiano, Structure and magnetic properties of nanostructured Ni-ferrite, J. Magn. Magn. Mater., 2001, 226–230(Part 2), 1379–1381 CrossRef CAS.
- S. Feng, W. Yang and Z. Wang, Synthesis of porous NiFe2O4 microparticles and its catalytic properties for methane combustion, Mater. Sci. Eng., B, 2011, 176, 1509–1512 CrossRef CAS.
- K.-S. Lin, C.-L. Chiang, P.-J. Hsu, K. Bat-Erdene, C.-Y. Tang and C.-M. Wu, Magnetic separation and recycling of ferrite nanocatalysts for CO2 decomposition with CH4 recovery from steel industrial flyash, Catal. Today, 2018, 307, 260–271 CrossRef CAS.
- U. Luders, A. Barthelemy, M. Bibes, K. Bouzehouane, S. Fusil, E. Jacquet, J.-P. Contour, J.-F. Bobo, J. Fontcuberta and A. Fert, NiFe2O4: a versatile spinel material brings new opportunities for spintronics, Adv. Mater., 2006, 18, 1733–1736 CrossRef CAS.
- T. Dai Lam, L. Van Hong, P. Hoai Linh, H. Thi My Nhung, N. Thi Quy, L. Thien Tai, H. Phuong Thu and N. Xuan Phuc, Biomedical and environmental applications of magnetic nanoparticles, Adv. Nat. Sci.: Nanosci. Nanotechnol., 2010, 1, 045013 Search PubMed.
- H.-M. Fan, J.-B. Yi, Y. Yang, K.-W. Kho, H.-R. Tan, Z.-X. Shen, J. Ding, X.-W. Sun, M. C. Olivo and Y.-P. Feng, Single-Crystalline MFe2O4 Nanotubes/Nanorings Synthesized by Thermal Transformation Process for Biological Applications, ACS Nano, 2009, 3, 2798–2808 CrossRef CAS PubMed.
- M. R. Phadatare, V. M. Khot, A. B. Salunkhe, N. D. Thorat and S. H. Pawar, Studies on polyethylene glycol coating on NiFe2O4 nanoparticles for biomedical applications, J. Magn. Magn. Mater., 2012, 324, 770–772 CrossRef CAS.
- A. Seyfoori, S. A. S. Ebrahimi, S. Omidian and S. M. Naghib, Multifunctional magnetic ZnFe2O4-hydroxyapatite nanocomposite particles for local anti-cancer drug delivery and bacterial infection inhibition: An in vitro study, J. Taiwan Inst. Chem. Eng., 2019, 96, 503–508 CrossRef CAS.
- C. V. Gopal Reddy, S. V. Manorama and V. J. Rao, Semiconducting gas sensor for chlorine based on inverse spinel nickel ferrite, Sens. Actuators, B, 1999, 55, 90–95 CrossRef CAS.
- S. Darshane and I. S. Mulla, Influence of palladium on gas-sensing performance of magnesium ferrite nanoparticles, Mater. Chem. Phys., 2010, 119, 319–323 CrossRef CAS.
- X. F. Wu, W. Wang, F. Li, S. Khaimanov, N. Tsidaeva and M. Lahoubi, PEG-assisted hydrothermal synthesis of CoFe2O4 nanoparticles with enhanced selective adsorption properties for different dyes, Appl. Surf. Sci., 2016, 389, 1003–1011 CrossRef CAS.
- R. P. Sharma, S. D. Raut, V. V. Jadhav, A. S. Kadam and R. S. Mane, Anti-candida and anti-adhesion efficiencies of zinc ferrite nanoparticles, Mater. Lett., 2019, 237, 165–167 CrossRef CAS.
- M. Foerster, J. M. Rebled, S. Estrade, F. Sanchez, F. Peiro and J. Fontcuberta, Distinct magnetism in ultrathin epitaxial NiFe2O4 films on MgAl2O4 and SrTiO3 single crystalline substrates, Phys. Rev. B: Condens. Matter Mater. Phys., 2011, 84, 144422 CrossRef.
- M. Junaid, M. A. Khan, F. Iqbal, G. Murtaza, M. N. Akhtar, M. Ahmad, I. Shakir and M. F. Warsi, Structural, spectral, dielectric and magnetic properties of Tb–Dy doped Li-Ni nano-ferrites synthesized via micro-emulsion route, J. Magn. Magn. Mater., 2016, 419, 338–344 CrossRef CAS.
- S. Sun, H. Zeng, D. B. Robinson, S. Raoux, P. M. Rice, S. X. Wang and G. Li, Monodisperse MFe2O4 (M = Fe, Co, Mn) Nanoparticles, J. Am. Chem. Soc., 2004, 126, 273–279 CrossRef CAS PubMed.
- A. S. Bhalla, R. Y. Guo and R. Roy, The perovskite structure - a review of its role in ceramic science and technology, Mater. Res. Innovations, 2000, 4, 3–26 CrossRef CAS.
- R. L. White, Review of Recent Work on the Magnetic and Spectroscopic Properties of the Rare-Earth Orthoferrites, J. Appl. Phys., 1969, 40, 1061–1069 CrossRef CAS.
- K. K. Bamzai and M. Bhat, Electrical and Magnetic Properties of Some Rare Earth Orthoferrites (RFeO3 where R = Y, Ho, Er) Systems, Integr. Ferroelectr., 2014, 158, 108–122 CrossRef CAS.
- J. Silva, A. Reyes, H. Esparza, H. Camacho and L. Fuentes, BiFeO3: A Review on Synthesis, Doping and Crystal Structure, Integr. Ferroelectr., 2011, 126, 47–59 CrossRef CAS.
- J. G. Wu, Z. Fan, D. Q. Xiao, J. G. Zhu and J. Wang, Multiferroic bismuth ferrite-based materials for multifunctional applications: Ceramic bulks, thin films and nanostructures, Prog. Mater. Sci., 2016, 84, 335–402 CrossRef CAS.
- A. Molak, D. K. Mahato and A. Z. Szeremeta, Synthesis and characterization of electrical features of bismuth manganite and bismuth ferrite: effects of doping in cationic and anionic sublattice: Materials for applications, Prog. Cryst. Growth Charact. Mater., 2018, 64, 1–22 CrossRef CAS.
- S. M. Lam, J. C. Sin and A. R. Mohamed, A newly emerging visible light-responsive BiFeO3 perovskite for photocatalytic applications: A mini review, Mater. Res. Bull., 2017, 90, 15–30 CrossRef CAS.
- X. S. Xu and W. B. Wang, Multiferroic hexagonal ferrites (h-RFeO3, R = Y, Dy-Lu): a brief experimental review, Mod. Phys. Lett. B, 2014, 28, 27 Search PubMed.
- J. J. Zhu, H. L. Li, L. Y. Zhong, P. Xiao, X. L. Xu, X. G. Yang, Z. Zhao and J. L. Li, Perovskite Oxides: Preparation, Characterizations, and Applications in Heterogeneous Catalysis, ACS Catal., 2014, 4, 2917–2940 CrossRef CAS.
- T. Arakawa, S. Tsuchiya and J. Shiokawa, Catalytic activity of rare-earth ortho-ferrites and orthochromites, Mater. Res. Bull., 1981, 16, 97–103 CrossRef CAS.
- T. Arakawa, S. Tsuchiya and J. Shiokawa, Catalytic properties and activity of rare-earth ortho-ferrites in oxidation of methanol, J. Catal., 1982, 74, 317–322 CrossRef CAS.
- B. P. Barbero, J. A. Gamboa and L. E. Cadus, Synthesis and characterisation of La1−xCaxFeO3 perovskite-type oxide catalysts for total oxidation of volatile organic compounds, Appl. Catal., B, 2006, 65, 21–30 CrossRef CAS.
- P. Ciambelli, S. Cimino, S. De Rossi, L. Lisi, G. Minelli, P. Porta and G. Russo, AFeO3 (A = La, Nd, Sm) and LaFe1−xMgxO3 perovskites as methane combustion and CO oxidation catalysts: structural, redox and catalytic properties, Appl. Catal., B, 2001, 29, 239–250 CrossRef CAS.
- A. Delmastro, D. Mazza, S. Ronchetti, M. Vallino, R. Spinicci, P. Brovetto and M. Salis, Synthesis and characterization of non-stoichiometric LaFeO3 perovskite, Mater. Sci. Eng., B, 2001, 79, 140–145 CrossRef.
- R. Spinicci, A. Tofanari, A. Delmastro, D. Mazza and S. Ronchetti, Catalytic properties of stoichiometric and non-stoichiometric LaFeO3 perovskite for total oxidation of methane, Mater. Chem. Phys., 2002, 76, 20–25 CrossRef CAS.
- G. Pecchi, P. Reyes, R. Zamora, C. Campos, L. E. Caduus and B. P. Barbero, Effect of the preparation method on the catalytic activity of La1−xCaxFeO3 perovskite-type oxides, Catal. Today, 2008, 133, 420–427 CrossRef.
- P. V. Gosavi and R. B. Biniwale, Pure phase LaFeO3 perovskite with improved surface area synthesized using different routes and its characterization, Mater. Chem. Phys., 2010, 119, 324–329 CrossRef CAS.
- J. M. Giraudon, A. Elhachimi and G. Leclercq, Catalytic oxidation of chlorobenzene over Pd/perovskites, Appl. Catal., B, 2008, 84, 251–261 CrossRef CAS.
- Y. C. Wei, J. A. Liu, Z. Zhao, Y. S. Chen, C. M. Xu, A. J. Duan, G. Y. Jiang and H. He, Highly Active Catalysts of Gold Nanoparticles Supported on Three-Dimensionally Ordered Macroporous LaFeO3 for Soot Oxidation, Angew. Chem., Int. Ed., 2011, 50, 2326–2329 CrossRef CAS PubMed.
- J. Li, J. Miao, X. G. Duan, J. Dai, Q. W. Liu, S. B. Wang, W. Zhou and Z. P. Shao, Fine-Tuning Surface Properties of Perovskites via Nanocompositing with Inert Oxide toward Developing Superior Catalysts for Advanced Oxidation, Adv. Funct. Mater., 2018, 28, 12 Search PubMed.
- D. Blanck, A. Schon, A. S. Mamede, C. Dujardin, J. P. Dacquin, P. Granger, J. F. Paul and E. Berrier, In situ Raman spectroscopy evidence of an accessible phase potentially involved in the enhanced activity of La -deficient lanthanum orthoferrite in 3-way catalysis (TWC), Catal. Today, 2017, 283, 151–157 CrossRef CAS.
- Z. X. Li, F. B. Shi and C. H. Yan, Controllable Assembly of Hierarchical Macroporous-Mesoporous LnFeO3 and Their Catalytic Performance in the CO plus NO Reaction, Langmuir, 2015, 31, 8672–8679 CrossRef CAS PubMed.
- P. Garcia-Munoz, C. Lefevre, D. Robert and N. Keller, Ti-substituted LaFeO3 perovskite as photoassisted CWPO catalyst for water treatment, Appl. Catal., B, 2019, 248, 120–128 CrossRef CAS.
- A. Wattiaux, J. C. Grenier, M. Pouchard and P. Hagenmuller, Electrolytic oxygen evolution in alkaline-medium on La1−xSrxFeO3−y perovskite-related ferrites. 1. Electrochemical study, J. Electrochem. Soc., 1987, 134, 1714–1718 CrossRef CAS.
- A. Wattiaux, J. C. Grenier, M. Pouchard and P. Hagenmuller, Electrolytic oxygen evolution in alkaline-medium on La1−xSrxFeO3−y perovskite-related ferrites. 2. Influence of bulk properties, J. Electrochem. Soc., 1987, 134, 1718–1724 CrossRef CAS.
- J. Suntivich, K. J. May, H. A. Gasteiger, J. B. Goodenough and Y. Shao-Horn, A Perovskite Oxide Optimized for Oxygen Evolution Catalysis from Molecular Orbital Principles, Science, 2011, 334, 1383–1385 CrossRef CAS PubMed.
- S. P. Simner, J. R. Bonnett, N. L. Canfield, K. D. Meinhardt, J. P. Shelton, V. L. Sprenkle and J. W. Stevenson, Development of lanthanum ferrite SOFC cathodes, J. Power Sources, 2003, 113, 1–10 CrossRef CAS.
- A. Mai, V. A. C. Haanappel, S. Uhlenbruck, F. Tietz and D. Stover, Ferrite-based perovskites as cathode materials for anode-supported solid oxide fuel cells Part I. Variation of composition, Solid State Ionics, 2005, 176, 1341–1350 CrossRef CAS.
- K. Huang, H. Y. Lee and J. B. Goodenough, Sr- and Ni-doped LaCaO3 and LaFeO3 perovskites - New cathode materials for solid-oxide fuel cells, J. Electrochem. Soc., 1998, 145, 3220–3227 CrossRef CAS.
- S. P. Jiang, Development of lanthanum strontium cobalt ferrite perovskite electrodes of solid oxide fuel cells - A review, Int. J. Hydrogen Energy, 2019, 44, 7448–7493 CrossRef CAS.
- J. Lyagaeva, N. Danilov, A. Tarutin, G. Vdovin, D. Medvedev, A. Demin and P. Tsiakaras, Designing a protonic ceramic fuel cell with novel electrochemically active oxygen electrodes based on doped Nd0.5Ba0.5FeO3-δ, Dalton Trans., 2018, 47, 8149–8157 RSC.
- J. Vieten, B. Bulfin, F. Call, M. Lange, M. Schmucker, A. Francke, M. Roeb and C. Sattler, Perovskite oxides for application in thermochemical air separation and oxygen storage, J. Mater. Chem. A, 2016, 4, 13652–13659 RSC.
- B. Bulfin, J. Lapp, S. Richter, D. Guban, J. Vieten, S. Brendelberger, M. Roeb and C. Sattler, Air separation and selective oxygen pumping via temperature and pressure swing oxygen adsorption using a redox cycle of SrFeO3 perovskite, Chem. Eng. Sci., 2019, 203, 68–75 CrossRef CAS.
- S. Hosokawa, Hexagonal Rare Earth-Iron Mixed Oxides (REFeO3): Crystal Structure, Synthesis, and Catalytic Properties, Front. Chem., 2019, 7, 8 CrossRef CAS PubMed.
- L. Valko, P. Bucek, R. Dosoudil and M. Usakova, Magnetic Properties of Ferrite-Polymer Composites, J. Electr. Eng., 2003, 54, 100–103 CAS.
- H. Dong, Y. C. Chen and C. Feldmann, Polyol synthesis of nanoparticles: status and options regarding metals, oxides, chalcogenides, and non-metal elements, Green Chem., 2015, 17, 4107–4132 RSC.
- J. Lin, M. Yu, C. Lin and X. Liu, Multiform oxide optical materials via the versatile Pechini-type sol−gel process: Synthesis and characteristics, J. Phys. Chem. C, 2007, 111, 5835–5845 CrossRef CAS.
- P. Dolcet, S. Diodati, F. Zorzi, P. Voepel, C. Seitz, B. M. Smarsly, S. Mascotto, F. Nestola and S. Gross, Very fast crystallisation of MFe2O4 spinel ferrites (M = Co, Mn, Ni, Zn) under low temperature hydrothermal conditions: a time-resolved structural investigation, Green Chem., 2018, 20, 2257–2268 RSC.
- S. Diodati, L. Pandolfo, S. Gialanella, A. Caneschi and S. Gross, Green and low temperature synthesis of nanocrystalline transition metal ferrites by simple wet chemistry routes, Nano Res., 2014, 7, 1027–1042 CrossRef CAS.
- A. Rabenau, The Role of Hydrothermal Synthesis in Preparative Chemistry, Angew. Chem., Int. Ed. Engl., 1985, 24, 1026–1040 CrossRef.
- M. Yoshimura and K. Byrappa, Hydrothermal processing of materials: past, present and future, J. Mater. Sci., 2008, 43, 2085–2103 CrossRef CAS.
- G. Demazeau, Solvothermal processes: a route to the stabilization of new materials, J. Mater. Chem., 1999, 9, 15–18 RSC.
- G. Demazeau, Solvothermal reactions: an original route for the synthesis of novel materials, J. Mater. Sci., 2008, 43, 2104–2114 CrossRef CAS.
- G. Demazeau, Solvothermal Processes: Definition, Key Factors Governing the Involved Chemical Reactions and New Trends, Z. Naturforsch., B: J. Chem. Sci., 2010, 65, 999–1006 CAS.
- C. S. Cundy and P. A. Cox, The Hydrothermal Synthesis of Zeolites: History and Development from the Earliest Days to the Present Time, Chem. Rev., 2003, 103, 663–701 CrossRef CAS PubMed.
- R. A. Laudise, Hydrothermal synthesis of crystals, Chem. Eng. News, 1987, 65, 30–43 CrossRef CAS.
- S. Somiya and R. Roy, Hydrothermal synthesis of fine oxide powders, Bull. Mater. Sci., 2000, 23, 453–460 CrossRef CAS.
- R. E. Riman, W. L. Suchanek and M. M. Lencka, Hydrothermal crystallization of ceramics, Ann. Chim.-Sci. Mat., 2002, 27, 15–36 CrossRef CAS.
- R. I. Walton, Subcritical solvothermal synthesis of condensed inorganic materials, Chem. Soc. Rev., 2002, 31, 230–238 RSC.
- S. Komarneni, Nanophase materials by hydrothermal, microwave-hydrothermal and microwave-solvothermal methods, Curr. Sci., 2003, 85, 1730–1734 CAS.
- M. Niederberger, G. Garnweitner, J. H. Ba, J. Polleux and N. Pinna, Nonaqueous synthesis, assembly and formation mechanisms of metal oxide nanocrystals, Int. J. Nanotechnol., 2007, 4, 263–281 CrossRef CAS.
- D. R. Modeshia and R. I. Walton, Solvothermal synthesis of perovskites and pyrochlores: crystallisation of functional oxides under mild conditions, Chem. Soc. Rev., 2010, 39, 4303–4325 RSC.
- S. Hosokawa, Synthesis of metal oxides with improved performance using a solvothermal method, J. Ceram. Soc. Jpn., 2016, 124, 870–874 CrossRef CAS.
- S. H. Feng and R. R. Xu, New materials in hydrothermal synthesis, Acc. Chem. Res., 2001, 34, 239–247 CrossRef CAS PubMed.
- C. I. Hiley and R. I. Walton, Controlling the crystallisation of oxide materials by solvothermal chemistry: tuning composition, substitution and morphology of functional solids, CrystEngComm, 2016, 18, 7656–7670 RSC.
- C. Chen, J. R. Cheng, S. W. Yu, L. J. Che and Z. Y. Meng, Hydrothermal synthesis of perovskite bismuth ferrite crystallites, J. Cryst. Growth, 2006, 291, 135–139 CrossRef CAS.
- J. T. Han, Y. H. Huang, X. J. Wu, C. L. Wu, W. Wei, B. Peng, W. Huang and J. B. Goodenough, Tunable synthesis of bismuth ferrites with various morphologies, Adv. Mater., 2006, 18, 2145–2148 CrossRef CAS.
- S. Li, Y. H. Lin, B. P. Zhang, Y. Wang and C. W. Nan, Controlled Fabrication of BiFeO3 Uniform Microcrystals and Their Magnetic and Photocatalytic Behaviors, J. Phys. Chem. C, 2010, 114, 2903–2908 CrossRef CAS.
- D. R. Cai, J. M. Li, T. Tong, D. R. Jin, S. W. Yu and J. R. Cheng, Phase evolution of bismuth ferrites in the process of hydrothermal reaction, Mater. Chem. Phys., 2012, 134, 139–144 CrossRef CAS.
- A. M. L. Lopes, J. P. Araujo and S. Ferdov, Room temperature synthesis of Bi25FeO39 and hydrothermal kinetic relations between sillenite- and distorted perovskite-type bismuth ferrites, Dalton Trans., 2014, 43, 18010–18016 RSC.
- Z. W. Chen and W. L. Jin, Low-temperature acetone-assisted hydrothermal synthesis and characterization of BiFeO3 powders, J. Mater. Sci.: Mater. Electron., 2014, 25, 4039–4045 CrossRef CAS.
- L. Zhang, X. F. Cao, Y. L. Ma, X. T. Chen and Z. L. Xue, Polymer-directed synthesis and magnetic property of nanoparticles-assembled BiFeO3 microrods, J. Solid State Chem., 2010, 183, 1761–1766 CrossRef CAS.
- L. Hou, K. H. Zuo, Q. B. Sun, Z. M. Ren, Y. P. Zeng and X. Li, Effects of external magnetic field on the morphology and magnetic property of BiFeO3 particles prepared by hydrothermal synthesis, Appl. Phys. Lett., 2013, 102, 4 Search PubMed.
- A. Huang, A. D. Handoko, G. K. L. Goh, P. K. Pallathadka and S. Shannigrahi, Hydrothermal synthesis of (001) epitaxial BiFeO3 films on SrTiO3 substrate, CrystEngComm, 2010, 12, 3806–3814 RSC.
- Y. Du, Z. X. Cheng, M. Shahbazi, E. W. Collings, S. X. Dou and X. L. Wang, Enhancement of ferromagnetic and dielectric properties in lanthanum doped BiFeO3 by hydrothermal synthesis, J. Alloys Compd., 2010, 490, 637–641 CrossRef CAS.
- A. Chaudhuri and K. Mandal, Enhancement of ferromagnetic and dielectric properties of lanthanum doped bismuth ferrite nanostructures, Mater. Res. Bull., 2012, 47, 1057–1061 CrossRef CAS.
- C. Ponzoni, M. Cannio, D. N. Boccaccini, C. R. H. Bahl, K. Agersted and C. Leonelli, Ultrafast microwave hydrothermal synthesis and characterization of Bi1−xLaxFeO3 micronized particles, Mater. Chem. Phys., 2015, 162, 69–75 CrossRef CAS.
- A. Chaudhuri and K. Mandal, Study of structural, ferromagnetic and ferroelectric properties of nanostructured barium doped Bismuth Ferrite, J. Magn. Magn. Mater., 2014, 353, 57–64 CrossRef CAS.
- K. K. Bharathi, G. Ramesh, L. N. Patro, N. R. C. Raju and D. K. Kim, Enhanced ferromagnetic properties and high temperature dielectric anomalies in Bi0.9Ca0.05Sm0.05FeO3 prepared by hydrothermal method, Mater. Res. Bull., 2015, 62, 5–10 CrossRef CAS.
- M. Yoshimura, K. Yamasawa and S. Somiya, Formation of LaFeO3 Crystals under Hydrothermal Conditions, Nippon. Seram. Kyo. Gak., 1982, 90, 521–527 CAS.
- Y. Abe, I. Satou, T. Aida and T. Adschiri, Formation of La-based perovskite compounds in supercritical water, Ceram. Int., 2018, 44, 12996–13003 CrossRef CAS.
- W. J. Zheng, R. H. Liu, D. K. Peng and G. Y. Meng, Hydrothermal synthesis of LaFeO3 under carbonate-containing medium, Mater. Lett., 2000, 43, 19–22 CrossRef CAS.
- L. Yuan, K. K. Huang, S. Wang, C. M. Hou, X. F. Wu, B. Zou and S. H. Feng, Crystal Shape Tailoring in Perovskite Structure Rare-Earth Ferrites REFeO3 (RE = La, Pr, Sm, Dy, Er, and Y) and Shape-Dependent Magnetic Properties of YFeO3, Cryst. Growth Des., 2016, 16, 6522–6530 CrossRef CAS.
- E. M. Kostyukhin, A. L. Kustov and L. M. Kustov, One-step hydrothermal
microwave-assisted synthesis of LaFeO3 nanoparticles, Ceram. Int., 2019, 45, 14384–14388 CrossRef CAS.
- S. Thirumalairajan, K. Girija, N. Y. Hebalkar, D. Mangalaraj, C. Viswanathan and N. Ponpandian, Shape evolution of perovskite LaFeO3 nanostructures: a systematic investigation of growth mechanism, properties and morphology dependent photocatalytic activities, RSC Adv., 2013, 3, 7549–7561 RSC.
-
R. D. Kumar and R. Jayavel, in Solid State Physics: Proceedings of the 58th Dae Solid State Physics Symposium 2013, Pts a & B, ed. C. Murli, D. Bhattacharyya and S. C. Gadkari, 2014, vol. 1591, pp. 315–317 Search PubMed.
- Y. J. Zhang, A. Zheng, X. Z. Yang, H. M. He, Y. Fan and C. P. Yao, Cubic GdFeO3 particle by a simple hydrothermal synthesis route and its photoluminescence and magnetic properties, CrystEngComm, 2012, 14, 8432–8439 RSC.
- Z. Q. Zhou, L. Guo, H. X. Yang, Q. Liu and F. Ye, Hydrothermal synthesis and magnetic properties of multiferroic rare-earth orthoferrites, J. Alloys Compd., 2014, 583, 21–31 CrossRef CAS.
- M. Zhou, H. Yang, T. Xian, J. Y. Ma, H. M. Zhang, W. J. Feng, Z. Q. Wei and J. L. Jiang, Morphology-controlled synthesis of orthorhombic LuFeO3 particles via a hydrothermal route, J. Alloys Compd., 2014, 617, 855–862 CrossRef CAS.
- Y. Wang, X. C. Yan, J. Chen, J. X. Deng, R. B. Yu and X. R. Xing, Shape controllable synthesis of NdFeO3 micro single crystals by a hydrothermal route, CrystEngComm, 2014, 16, 858–862 RSC.
- C. Y. Zhang, M. Y. Shang, M. L. Liu, T. S. Zhang, L. Ge, H. M. Yuan and S. H. Feng, Multiferroicity in SmFeO3 synthesized by hydrothermal method, J. Alloys Compd., 2016, 665, 152–157 CrossRef CAS.
- J. Q. Zhang, K. K. Huang, L. Yuan and S. H. Feng, Mineralizer effect on facet-controllable hydrothermal crystallization of perovskite structure YbFeO3 crystals, CrystEngComm, 2018, 20, 470–476 RSC.
- W. W. Hu, Y. Chen, H. M. Yuan, G. H. Zhang, G. H. Li, G. S. Pang and S. H. Feng, Hydrothermal synthesis, characterization and composition-dependent magnetic properties of LaFe1−xCrxO3 system (0 ≤ x ≤ 1), J. Solid State Chem., 2010, 183, 1582–1587 CrossRef CAS.
- L. Yuan, K. K. Huang, C. M. Hou, W. C. Feng, S. Wang, C. P. Zhou and S. H. Feng, Hydrothermal synthesis and magnetic properties of REFe0.5Cr0.5O3 (RE = La, Tb, Ho, Er, Yb, Lu and Y) perovskite, New J. Chem., 2014, 38, 1168–1172 RSC.
- Y. Qiao, Y. F. Zhou, S. Wang, L. Yuan, Y. Y. Du, D. Y. Lu, G. B. Che and H. N. Che, Composition dependent magnetic and ferroelectric properties of hydrothermally synthesized GdFe1−xCrxO3 (0.1 ≤ x ≤ 0.9) perovskites, Dalton Trans., 2017, 46, 5930–5937 RSC.
- V. I. Popkov and O. V. Almjasheva, Formation mechanism of YFeO3 nanoparticles under the hydrothermal conditions, Nanosyst.: Phys., Chem., Math., 2014, 5, 703–708 Search PubMed.
- A. V. Racu, D. H. Ursu, O. V. Kuliukova, C. Logofatu, A. Leca and M. Miclau, Direct low temperature hydrothermal synthesis of YFeO3 microcrystals, Mater. Lett., 2015, 140, 107–110 CrossRef CAS.
- V. I. Popkov, O. V. Almjasheva, A. S. Semenova, D. G. Kellerman, V. N. Nevedomskiy and V. V. Gusarov, Magnetic properties of YFeO3 nanocrystals obtained by different soft-chemical methods, J. Mater. Sci.: Mater. Electron., 2017, 28, 7163–7170 CrossRef CAS.
- M. Y. Shang, C. Y. Zhang, T. S. Zhang, L. Yuan, L. Ge, H. M. Yuan and S. H. Feng, The multiferroic perovskite YFeO3, Appl. Phys. Lett., 2013, 102, 3 Search PubMed.
- C. M. Hou, W. C. Feng, L. Yuan, K. K. Huang and S. H. Feng, Crystal facet control of LaFeO3, LaCrO3, and La0.75Sr0.25MnO3, CrystEngComm, 2014, 16, 2874–2877 RSC.
- K. K. Huang, L. Yuan and S. H. Feng, Crystal facet tailoring arts in perovskite oxides, Inorg. Chem. Front., 2015, 2, 965–981 RSC.
- M. Inoue, T. Nishikawa, T. Nakamura and T. Inui, Glycothermal reaction of rare-earth acetate and iron acetylacetonate: Formation of hexagonal REFeO3, J. Am. Ceram. Soc., 1997, 80, 2157–2160 CrossRef CAS.
- S. Hosokawa, H. J. Jeon, S. Iwamoto and M. Inoue, Synthesis of Rare Earth Iron-Mixed Oxide Nanoparticles by Solvothermal Methods, J. Am. Ceram. Soc., 2009, 92, 2847–2853 CrossRef CAS.
- S. Hosokawa, H. J. Jeon and M. Inoue, Thermal stabilities of hexagonal and orthorhombic YbFeO3 synthesized by solvothermal method and their catalytic activities for methane combustion, Res. Chem. Intermed., 2011, 37, 291–296 CrossRef CAS.
- X. F. Guan, J. Zheng, M. L. Zhao, L. P. Li and G. S. Li, Synthesis of FeTiO3 nanosheets with {0001} facets exposed: enhanced electrochemical performance and catalytic activity, RSC Adv., 2013, 3, 13635–13641 RSC.
- F. Zhang, C. Wei, Y. Hu and H. Wu, Zinc ferrite catalysts for ozonation of aqueous organic contaminants: phenol and bio-treated coking wastewater, Sep. Purif. Technol., 2015, 156(Part 2), 625–635 CrossRef CAS.
- T. K. Aparna and R. Sivasubramanian, FeTiO3 nanohexagons based electrochemical sensor for the detection of dopamine in presence of uric acid, Mater. Chem. Phys., 2019, 233, 319–328 CrossRef CAS.
- S. Palanisamy, S. Srinivasan, A. P. Shyma, N. Rajendhran, K. Subramani, V. Murugan and R. Venkatachalam, Influence of nanoflower FeTiO3 in carbon dioxide reduction, SN Appl. Sci., 2019, 1, 10 Search PubMed.
- T. Tao, A. M. Glushenkov, H. W. Liu, Z. W. Liu, X. J. J. Dai, H. Chen, S. P. Ringer and Y. Chen, Ilmenite FeTiO3 Nanoflowers and Their Pseudocapacitance, J. Phys. Chem. C, 2011, 115, 17297–17302 CrossRef CAS.
- T. Tao, Y. Chen, Y. H. Chen, D. S. Fox, H. Z. Zhang, M. Q. Zhou, M. Raveggi, A. J. Barlow and A. M. Glushenkov, Two-Dimensional Metal Oxide Nanoflower-Like Architectures: A General Growth Method and Their Applications in Energy Storage and as Model Materials for Nanofabrication, ChemPlusChem, 2017, 82, 295–302 CrossRef CAS PubMed.
- A. R. Gainsford, M. J. Sisley, T. W. Swaddle and P. Bayliss, Hydrothermal formation of ferrite spinels, Can. J. Chem., 1975, 53, 12–19 CrossRef CAS.
- Y. Xie, Y. T. Qian, J. Li, Z. Y. Chen and Y. Li, Hydrothermal preparation and characterization of ultrafine powders of ferrite spinels MFe2O4 (M=Fe, Zn and Ni), Mater. Sci. Eng., B, 1995, 34, L1–L3 CrossRef.
- C. Rath, K. K. Sahu, S. Anand, S. K. Date, N. C. Mishra and R. P. Das, Preparation and characterization of nanosize Mn-Zn ferrite, J. Magn. Magn. Mater., 1999, 202, 77–84 CrossRef CAS.
- C. Rath, S. Anand, R. P. Das, K. K. Sahu, S. D. Kulkarni, S. K. Date and N. C. Mishra, Dependence on cation distribution of particle size, lattice parameter, and magnetic properties in nanosize Mn-Zn ferrite, J. Appl. Phys., 2002, 91, 2211–2215 CrossRef CAS.
- A. L. Xia, S. K. Liu, C. G. Jin, L. Chen and Y. H. Lv, Hydrothermal Mg1−xZnxFe2O4 spinel ferrites: Phase formation and mechanism of saturation magnetization, Mater. Lett., 2013, 105, 199–201 CrossRef CAS.
- L. Nalbandian, A. Delimitis, V. T. Zaspalis, E. A. Deliyanni, D. N. Bakoyannakis and E. N. Peleka, Hydrothermally prepared nanocrystalline Mn-Zn ferrites: Synthesis and characterization, Microporous Mesoporous Mater., 2008, 114, 465–473 CrossRef CAS.
- H. W. Wang and S. C. Kung, Crystallization of nanosized Ni-Zn ferrite powders prepared by hydrothermal method, J. Magn. Magn. Mater., 2004, 270, 230–236 CrossRef CAS.
- X. Y. Hou, J. Feng, X. D. Xu and M. L. Zhang, Synthesis and characterizations of spinel MnFe2O4 nanorod by seed-hydrothermal route, J. Alloys Compd., 2010, 491, 258–263 CrossRef CAS.
- J. Wang, Q. W. Chen, B. Y. Hou and Z. M. Peng, Synthesis and magnetic properties of single-crystals of MnFe2O4 nanorods, Eur. J. Inorg. Chem., 2004, 1165–1168, DOI:10.1002/ejic.200300555.
- R. Fareghi-Alamdari, F. Zandi and M. H. Keshavarz, Copper-cobalt synergy in Cu1−xCoxFe2O4 spinel ferrite as a highly efficient and regioselective nanocatalyst for the synthesis of 2,4-dinitrotoluene, RSC Adv., 2015, 5, 71911–71921 RSC.
- A. L. Tiano, G. C. Papaefthymiou, C. S. Lewis, J. Han, C. Zhang, Q. Li, C. Y. Shi, A. M. M. Abeykoon, S. J. L. Billinge, E. Stach, J. Thomas, K. Guerrero, P. Munayco, J. Munayco, R. B. Scorzelli, P. Burnham, A. J. Viescas and S. S. Wong, Correlating Size and Composition-Dependent Effects with Magnetic, Mossbauer, and Pair Distribution Function Measurements in a Family of Catalytically Active Ferrite Nanoparticles, Chem. Mater., 2015, 27, 3572–3592 CrossRef CAS.
- A. Sheoran, M. Dhiman, S. Bhukal, R. Malik, J. Agarwal, B. Chudasama and S. Singhal, Development of magnetically retrievable spinel nanoferrites as efficient catalysts for aminolysis of epoxides with amines, Mater. Chem. Phys., 2019, 222, 207–216 CrossRef CAS.
- L. Satyanarayana, K. M. Reddy and S. V. Manorama, Synthesis of nanocrystalline Ni1−xCoxMnxFe2−xO4: a material for liquefied petroleum gas sensing, Sens. Actuators, B, 2003, 89, 62–67 CrossRef CAS.
- X. Shen, Y. X. Wang, X. Yang, L. Q. Lu and L. Huang, 0.3–3 GHz magneto-dielectric properties of nanostructured NiZnCo ferrite from hydrothermal process, J. Mater. Sci.: Mater. Electron., 2010, 21, 630–634 CrossRef CAS.
- Y. A. Pu, X. Tao, J. Zhai and J. F. Chen, Synthesis and electromagnetic properties of microwave absorbing material: Cox(Cu0.5Zn0.5)1−xFe2O4 (0 < x < 1) nanoparticles, Mater. Sci. Eng., B, 2011, 176, 163–166 CrossRef CAS.
- L. X. Wang, J. C. Li, Y. Q. Wang, L. J. Zhao and Q. Jiang, Adsorption capability for Congo red on nanocrystalline MFe2O4 (M = Mn, Fe, Co, Ni) spinel ferrites, Chem. Eng. J., 2012, 181, 72–79 CrossRef.
- X. Wang, L. S. Gao, L. Li, H. G. Zheng, Z. D. Zhang, W. C. Yu and Y. T. Qian, Low temperature synthesis of metastable lithium ferrite: magnetic and electrochemical properties, Nanotechnology, 2005, 16, 2677–2680 CrossRef CAS.
- Z. T. Chen and L. Gao, Synthesis and magnetic properties of CoFe2O4 nanoparticles by using PEG as surfactant additive, Mater. Sci. Eng., B, 2007, 141, 82–86 CrossRef CAS.
- H. Kavas, A. Baykal, M. S. Toprak, Y. Koseoglu, M. Sertkol and B. Aktas, Cation distribution and magnetic properties of Zn doped NiFe2O4 nanoparticles synthesized by PEG-assisted hydrothermal route, J. Alloys Compd., 2009, 479, 49–55 CrossRef CAS.
- F. Gozuak, Y. Koseoglu, A. Baykal and H. Kavas, Synthesis
and characterization of CoxZn1−xFe2O4 magnetic nanoparticles via a PEG-assisted route, J. Magn. Magn. Mater., 2009, 321, 2170–2177 CrossRef.
- Y. Koseoglu, M. Bay, M. Tan, A. Baykal, H. Sozeri, R. Topkaya and N. Akdogan, Magnetic and dielectric properties of Mn0.2Ni0.8Fe2O4 nanoparticles synthesized by PEG-assisted hydrothermal method, J. Nanopart. Res., 2011, 13, 2235–2244 CrossRef.
- M. Tan, Y. Koseoglu, F. Alan and E. Senturk, Overlapping large polaron tunneling conductivity and giant dielectric constant in Ni0.5Zn0.5Fe1.5Cr0.5O4 nanoparticles (NPs), J. Alloys Compd., 2011, 509, 9399–9405 CrossRef CAS.
- Y. Koseoglu, F. Alan, M. Tan, R. Yilgin and M. Ozturk, Low temperature hydrothermal synthesis and characterization of Mn doped cobalt ferrite nanoparticles, Ceram. Int., 2012, 38, 3625–3634 CrossRef CAS.
- T. R. Bastami, M. H. Entezari, Q. H. Hu, S. B. Hartono and S. Z. Qiao, Role of polymeric surfactants on the growth of manganese ferrite nanoparticles, Chem. Eng. J., 2012, 210, 157–165 CrossRef CAS.
- Y. Koseoglu, M. I. O. Oleiwi, R. Yilgin and A. N. Kocbay, Effect of chromium addition on the structural, morphological and magnetic properties of nano-crystalline cobalt ferrite system, Ceram. Int., 2012, 38, 6671–6676 CrossRef CAS.
- Y. Koseoglu, Structural, magnetic, electrical and dielectric properties of MnxNi1−xFe2O4 spinel nanoferrites prepared by PEG assisted hydrothermal method, Ceram. Int., 2013, 39, 4221–4230 CrossRef CAS.
- B. Paul, D. D. Purkayastha and S. S. Dhar, Size-controlled synthesis of NiFe2O4 nanospheres via a PEG assisted hydrothermal route and their catalytic properties in oxidation of alcohols by periodic acid, Appl. Surf. Sci., 2016, 370, 469–475 CrossRef CAS.
- J. L. Zhang, J. X. Shi and M. L. Gong, Synthesis of magnetic nickel spinel ferrite nanospheres by a reverse emulsion-assisted hydrothermal process, J. Solid State Chem., 2009, 182, 2135–2140 CrossRef CAS.
- B. Bateer, C. G. Tian, Y. Qu, S. C. Du, Y. Yang, Z. Y. Ren, K. Pan and H. G. Fu, Synthesis, size and magnetic properties of controllable MnFe2O4 nanoparticles with versatile surface functionalities, Dalton Trans., 2014, 43, 9885–9891 RSC.
- M. Fantauzzi, F. Secci, M. S. Angotzi, C. Passiu, C. Cannas and A. Rossi, Nanostructured spinel cobalt ferrites: Fe and Co chemical state, cation distribution and size effects by X-ray photoelectron spectroscopy, RSC Adv., 2019, 9, 19171–19179 RSC.
- L. Wang, J. W. Ren, Y. G. Wang, X. H. Liu and Y. Q. Wang, Controlled synthesis of magnetic spinel-type nickel ferrite nanoparticles by the interface reaction and hydrothermal crystallization, J. Alloys Compd., 2010, 490, 656–660 CrossRef CAS.
- A. Repko, J. Vejpravova, T. Vackova, D. Zakutna and D. Niznansky, Oleate-based hydrothermal preparation of CoFe2O4 nanoparticles, and their magnetic properties with respect to particle size and surface coating, J. Magn. Magn. Mater., 2015, 390, 142–151 CrossRef CAS.
- M. S. Angotzi, A. Musinu, V. Mameli, A. Ardu, C. Cara, D. Niznansky, H. L. Xin and C. Cannas, Spinel Ferrite Core-Shell Nanostructures by a Versatile Solvothermal Seed-Mediated Growth Approach and Study of Their Nanointerfaces, ACS Nano, 2017, 11, 7889–7900 CrossRef PubMed.
- S. Phumying, S. Labuayai, E. Swatsitang, V. Amornkitbamrung and S. Maensiri, Nanocrystalline spinel ferrite (MFe2O4, M = Ni, Co, Mn, Mg, Zn) powders prepared by a simple aloe vera plant-extracted solution hydrothermal route, Mater. Res. Bull., 2013, 48, 2060–2065 CrossRef CAS.
- K. Vamvakidis, M. Katsikini, D. Sakellari, E. C. Paloura, O. Kalogirou and C. Dendrinou-Samara, Reducing the inversion degree of MnFe2O4 nanoparticles through synthesis to enhance magnetization: evaluation of their H-1 NMR relaxation and heating efficiency, Dalton Trans., 2014, 43, 12754–12765 RSC.
- D. S. Cook, R. J. Kashtiban, K. Krambrock, G. M. de Lima, H. O. Stumpf, L. R. S. Lara, J. D. Ardisson and R. I. Walton, Nanocrystalline Transition-Metal Gallium Oxide Spinels from Acetylacetonate Precursors via Solvothermal Synthesis, Materials, 2019, 12, 838 CrossRef CAS PubMed.
- K. Vamvakidis, M. Katsikini, G. Vourlias, M. Angelakeris, E. C. Paloura and C. Dendrinou-Samara, Composition and hydrophilicity control of Mn-doped ferrite (MnxFe3−xO4) nanoparticles induced by polyol differentiation, Dalton Trans., 2015, 44, 5396–5406 RSC.
- C. Jolley, V. Pool, Y. Idzerda and T. Douglas, All in the Packaging: Structural and Electronic Effects of Nanoconfinement on Metal Oxide Nanoparticles, Chem. Mater., 2011, 23, 3921–3929 CrossRef CAS.
- L. Y. Chen, Y. M. Shen and J. F. Bai, Large-scale synthesis of uniform spinel ferrite nanoparticles from hydrothermal decomposition of trinuclear heterometallic oxo-centered acetate clusters, Mater. Lett., 2009, 63, 1099–1101 CrossRef CAS.
- K. O. Abdulwahab, M. A. Malik, P. O'Brien, G. A. Timco, F. Tuna, C. A. Muryn, R. E. P. Winpenny, R. A. D. Pattrick, V. S. Coker and E. Arenholz, A One-Pot Synthesis of Monodispersed Iron Cobalt Oxide and Iron Manganese Oxide Nanoparticles from Bimetallic Pivalate Clusters, Chem. Mater., 2014, 26, 999–1013 CrossRef CAS.
- K. O. Abdulwahab, M. A. Malik, P. O'Brien, G. A. Timco, F. Tuna, R. E. P. Winpenny, R. A. D. Pattrick, V. S. Coker and E. Arenholz, Hot injection thermolysis of heterometallic pivalate clusters for the synthesis of monodisperse zinc and nickel ferrite nanoparticles, J. Mater. Chem. C, 2014, 2, 6781–6789 RSC.
- H. Deng, X. L. Li, Q. Peng, X. Wang, J. P. Chen and Y. D. Li, Monodisperse magnetic single-crystal ferrite microspheres, Angew. Chem., Int. Ed., 2005, 44, 2782–2785 CrossRef CAS PubMed.
- C. Y. Hou, H. Yu, Q. H. Zhang, Y. G. Li and H. Z. Wang, Preparation and magnetic property analysis of monodisperse Co-Zn ferrite nanospheres, J. Alloys Compd., 2010, 491, 431–435 CrossRef CAS.
- Y. Wang, D. W. Su, A. Ung, J. H. Ahn and G. X. Wang, Hollow CoFe2O4 nanospheres as a high capacity anode material for lithium ion batteries, Nanotechnology, 2012, 23, 055402 CrossRef CAS PubMed.
- R. Otero-Lorenzo, M. A. Ramos-Docarripo, B. Rodriguez-Gonzalez, M. Comesana-Hermo and V. Salgueirino, Solvothermal Clustering of Magnetic Spinel Ferrite Nanocrystals: A Raman Perspective, Chem. Mater., 2017, 29, 8729–8736 CrossRef CAS.
- H. Hayashi and Y. Hakuta, Hydrothermal synthesis of metal oxide nanoparticles in supercritical water, Materials, 2010, 3, 3794–3817 CrossRef CAS PubMed.
- B. Stieberova, M. Zilka, M. Ticha, F. Freiberg, P. Caramazana-Gonzalez, J. McKechnie and E. Lester, Sustainability assessment of continuous-flow hydrothermal synthesis of nanomaterials in the context of other production technologies, J. Cleaner Prod., 2019, 241, 10 CrossRef.
- A. Cabañas and M. Poliakoff, The continuous hydrothermal synthesis of nano-particulate ferrites in near critical and supercritical water, J. Mater. Chem., 2001, 11, 1408–1416 RSC.
- T. Sato, K. Sue, W. Suzuki, M. Suzuki, K. Matsui, Y. Hakuta, H. Hayashi, K. Arai, S. I. Kawasaki, A. Kawai-Nakamura and T. Hiaki, Rapid and continuous production of ferrite nanoparticles by hydrothermal synthesis at 673 K and 30 MPa, Ind. Eng. Chem. Res., 2008, 47, 1855–1860 CrossRef CAS.
- M. Stingaciu, H. L. Andersen, C. Granados-Miralles, A. Mamakhel and M. Christensen, Magnetism in CoFe2O4 nanoparticles produced at sub- and near-supercritical conditions of water, CrystEngComm, 2017, 19, 3986–3996 RSC.
- Y. Xu, F. Ozcan, P. Zielke, S. Becker, M. Heimann, J. Heese, K. Chakrapani, M. Behrens, S. B. Simonsen, P. Norby, P. V. Hendriksen and R. Kiebach, Continuous Hydrothermal Flow Synthesis of Co1−xNixFe2O4 (x = 0–0.8) Nanoparticles and Their Catalytic Properties for CO Oxidation and Oxygen Evolution Reaction, Z. Anorg. Allg. Chem., 2018, 644, 1727–1733 CrossRef CAS.
- H. Tumazawa, Y. Maeda and E. Sada, Further consideration of hydrothermal synthesis of barium ferrite fine particles, J. Mater. Sci. Lett., 1995, 14, 68–70 CrossRef.
- X. Y. Liu, J. Wang, L. M. Gan and S. C. Ng, Improving the magnetic properties of hydrothermally synthesized barium ferrite, J. Magn. Magn. Mater., 1999, 195, 452–459 CrossRef CAS.
- S. Che, J. Wang and Q. W. Chen, Soft magnetic nanoparticles of BaFe12O19 fabricated under mild conditions, J. Phys.: Condens. Matter, 2003, 15, L335–L339 CrossRef CAS.
- G. V. Duong, R. S. Turtelli, B. D. Thuan, D. V. Linh, N. Hanh and R. Groessinger, Magnetic properties of nanocrystalline BaFe12O19 prepared by hydrothermal method, J. Non-Cryst. Solids, 2007, 353, 811–813 CrossRef CAS.
- T. Yamauchi, Y. Tsukahara, T. Sakata, H. Mori, T. Chikata, S. Katoh and Y. Wada, Barium ferrite powders prepared by microwave-induced hydrothermal reaction and magnetic property, J. Magn. Magn. Mater., 2009, 321, 8–11 CrossRef CAS.
- Y. Liu, M. G. B. Drew, J. P. Wang, M. L. Zhang and Y. Liu, Efficiency and purity control in the preparation of pure and/or aluminum-doped barium ferrites by hydrothermal methods using ferrous ions as reactants, J. Magn. Magn. Mater., 2010, 322, 366–374 CrossRef CAS.
- A. L. Xia, C. H. Zuo, L. Chen, C. G. Jin and Y. H. Lv, Hexagonal SrFe12O19 ferrites: Hydrothermal synthesis and their sintering properties, J. Magn. Magn. Mater., 2013, 332, 186–191 CrossRef CAS.
- H. Mocuta, L. Lechevallier, J. M. Le Breton, J. F. Wang and I. R. Harris, Structural and magnetic properties of hydrothermally synthesised Sr1−xNdxFe12O19 hexagonal ferrites, J. Alloys Compd., 2004, 364, 48–52 CrossRef CAS.
- L. Lechevallier, J. M. Le Breton, J. F. Wang and I. R. Harris, Structural analysis of hydrothermally synthesized Sr1−xSmxFe12O19 hexagonal ferrites, J. Magn. Magn. Mater., 2004, 269, 192–196 CrossRef CAS.
- Y. Jing, L. Jia, Y. Zheng and H. Zhang, Hydrothermal synthesis and competitive growth of flake-like M-type strontium hexaferrite, RSC Adv., 2019, 9, 33388–33394 RSC.
- G. D. Soria, P. Jenus, J. F. Marco, A. Mandziak, M. Sanchez-Arenillas, F. Moutinho, J. E. Prieto, P. Prieto, J. Cerdá, C. Tejera-Centeno, S. Gallego, M. Foerster, L. Aballe, M. Valvidares, H. B. Vasili, E. Pereiro, A. Quesada and J. de la Figuera, Strontium hexaferrite platelets: a comprehensive soft X-ray absorption and Mössbauer spectroscopy study, Sci. Rep., 2019, 9, 11777 CrossRef CAS PubMed.
- S. Dong, C. Lin and X. Meng, One-pot synthesis and microwave absorbing properties of ultrathin SrFe12O19 nanosheets, J. Alloys Compd., 2019, 783, 779–784 CrossRef CAS.
- W. Shen, B. Y. Ren, W. Wang and J. P. Liu, Formation of Samarium Ferrites With Controllable Morphology by Changing the Addition of KOH, IEEE Trans. Magn., 2019, 55, 5 Search PubMed.
- K. S. Suslick, M. Fang and T. Hyeon, Sonochemical synthesis of iron colloids, J. Am. Chem. Soc., 1996, 118, 11960–11961 CrossRef CAS.
- L. H. Thompson and L. K. Doraiswamy, Sonochemistry: Science and engineering, Ind. Eng. Chem. Res., 1999, 38, 1215–1249 CrossRef CAS.
-
T. J. Mason and J. P. Lorimal, Applied sonochemistry, Wiley, New York, 2002 Search PubMed.
- M. Abbas, B. Parvatheeswara Rao, M. Nazrul Islam, K. W. Kim, S. M. Naga, M. Takahashi and C. Kim, Size-controlled high magnetization CoFe2O4 nanospheres and nanocubes using rapid one-pot sonochemical technique, Ceram. Int., 2014, 40, 3269–3276 CrossRef CAS.
- T.-W. Chen, U. Rajaji, S.-M. Chen, M. M. Al Mogren, M. Hochlaf, S. D. A. Al Harbi and R. J. Ramalingam, A novel nanocomposite with superior electrocatalytic activity: A magnetic property based ZnFe2O4 nanocubes embellished with reduced graphene oxide by facile ultrasonic approach, Ultrason. Sonochem., 2019, 57, 116–124 CrossRef CAS PubMed.
- H. A. Choudhury, A. Choudhary, M. Sivakumar and V. S. Moholkar, Mechanistic investigation of the sonochemical synthesis of zinc ferrite, Ultrason. Sonochem., 2013, 20, 294–302 CrossRef CAS PubMed.
- K. S. Suslick, Sonochemistry, Science, 1990, 247, 1439 CrossRef CAS PubMed.
- J. H. Bang and K. S. Suslick, Applications of Ultrasound to the Synthesis of Nanostructured Materials, Adv. Mater., 2010, 22, 1039–1059 CrossRef CAS PubMed.
- P. R. Gogate and A. B. Pandit, Sonochemical reactors: scale up aspects, Ultrason. Sonochem., 2004, 11, 105–117 CrossRef CAS PubMed.
- N. Das, R. Majumdar, A. Sen and H. S. Maiti, Nanosized bismuth ferrite powder prepared through sonochemical and microemulsion techniques, Mater. Lett., 2007, 61, 2100–2104 CrossRef CAS.
- D. P. Dutta, B. P. Mandal, R. Naik, G. Lawes and A. K. Tyagi, Magnetic, ferroelectric, and magnetocapacitive properties of sonochemically synthesized Sc-doped BiFeO3 nanoparticles, J. Phys. Chem. C, 2013, 117, 2382–2389 CrossRef CAS.
- M. Abbas, B. Parvatheeswara Rao and C. Kim, Shape and size-controlled synthesis of Ni Zn ferrite nanoparticles by two different routes, Mater. Chem. Phys., 2014, 147, 443–451 CrossRef CAS.
- B. R. Reddy, T. Sivasankar, M. Sivakumar and V. S. Moholkar, Physical facets of ultrasonic cavitational synthesis of zinc ferrite particles, Ultrason. Sonochem., 2010, 17, 416–426 CrossRef CAS PubMed.
- H. Harzali, F. Saida, A. Marzouki, A. Megriche, F. Baillon, F. Espitalier and A. Mgaidi, Structural and magnetic properties of nano-sized NiCuZn ferrites synthesized by co-precipitation method with ultrasound irradiation, J. Magn. Magn. Mater., 2016, 419, 50–56 CrossRef CAS.
- R. S. Yadav, I. Kuřitka, J. Vilcakova, J. Havlica, L. Kalina, P. Urbánek, M. Machovsky, D. Skoda, M. Masař and M. Holek, Sonochemical synthesis of Gd3+ doped CoFe2O4 spinel ferrite nanoparticles and its physical properties, Ultrason. Sonochem., 2018, 40, 773–783 CrossRef CAS PubMed.
- K. K. Senapati, C. Borgohain and P. Phukan, Synthesis of highly stable CoFe2O4 nanoparticles and their use as magnetically separable catalyst for Knoevenagel reaction in aqueous medium, J. Mol. Catal. A: Chem., 2011, 339, 24–31 CrossRef CAS.
- K. V. P. M. Shafi, A. Gedanken, R. Prozorov and J. Balogh, Sonochemical preparation and size-dependent properties of nanostructured CoFe2O4 particles, Chem. Mater., 1998, 10, 3445–3450 CrossRef CAS.
- K. V. P. M. Shafi, Y. Koltypin, A. Gedanken, R. Prozorov, J. Balogh, J. Lendvai and I. Felner, Sonochemical preparation of nanosized amorphous NiFe2O4 particles, J. Phys. Chem. B, 1997, 101, 6409–6414 CrossRef CAS.
- W. Wu, Q. He and C. Jiang, Magnetic iron oxide nanoparticles: synthesis and surface functionalization strategies, Nanoscale Res. Lett., 2008, 3, 397–415 CrossRef CAS PubMed.
- R. Muñoz-Espì, Y. Mastai, S. Gross and K. Landfester, Colloidal systems for crystallization processes from liquid phase, CrystEngComm, 2013, 15, 2175–2191 RSC.
- P. Dolcet, F. Latini, M. Casarin, A. Speghini, E. Tondello, C. Foss, S. Diodati, L. Verin, A. Motta and S. Gross, Inorganic Chemistry in a Nanoreactor: Doped ZnO Nanostructures by Miniemulsion, Eur. J. Inorg. Chem., 2013, 2013, 2291–2300 CrossRef CAS.
- N. A. Heutz, P. Dolcet, A. Birkner, M. Casarin, K. Merz, S. Gialanella and S. Gross, Inorganic chemistry in a nanoreactor: Au/TiO2 nanocomposites by photolysis of a single-source precursor in miniemulsion, Nanoscale, 2013, 5, 10534–10541 RSC.
- P. Dolcet, M. Casarin, C. Maccato, L. Bovo, G. Ischia, S. Gialanella, F. Mancin, E. Tondello and S. Gross, Miniemulsions as chemical nanoreactors for the room temperature synthesis of inorganic crystalline nanostructures: ZnO colloids, J. Mater. Chem., 2012, 22, 1620–1626 RSC.
-
M. Fanun, Microemulsions: Properties and Applications, CRC Press, Boca Raton, 1st edn, 2019 Search PubMed.
- M. Sanchez-Dominguez, K. Pemartin and M. Boutonnet, Preparation of inorganic nanoparticles in oil-in-water microemulsions: A soft and versatile approach, Curr. Opin. Colloid Interface Sci., 2012, 17, 297–305 CrossRef CAS.
- I. Danielsson and B. Lindman, The definition of microemulsion, Colloids Surf., 1981, 3, 391–392 CrossRef CAS.
- K. Landfester, Synthesis of colloidal particles in miniemulsions, Annu. Rev. Mater. Res., 2006, 36, 231–279 CrossRef CAS.
- K. Landfester, The generation of nanoparticles in miniemulsions, Adv. Mater., 2001, 13, 765–768 CrossRef CAS.
-
K. Landfester, in Colloid Chemistry II, ed. M. Antonietti, Springer Berlin Heidelberg, Berlin, Heidelberg, 2003, pp. 75–123, DOI:10.1007/3-40-36412-9_4.
- Z. Cao and U. Ziener, Synthesis of nanostructured materials in inverse miniemulsions and their applications, Nanoscale, 2013, 5, 10093–10107 RSC.
- M. A. López-Quintela, C. Tojo, M. C. Blanco, L. García Rio and J. R. Leis, Microemulsion dynamics and reactions in microemulsions, Curr. Opin. Colloid Interface Sci., 2004, 9, 264–278 CrossRef.
- K. Landfester, Recent developments in miniemulsions — formation and stability mechanisms, Macromol. Symp., 2000, 150, 171–178 CrossRef CAS.
- A. Scano, V. Cabras, M. Pilloni and G. Ennas, Microemulsions: The Renaissance of Ferrite Nanoparticle Synthesis, J. Nanosci. Nanotechnol., 2019, 19, 4824–4838 CrossRef CAS PubMed.
- V. Uskokovic and M. Drofenik, A mechanism for the formation of nanostructured NiZn ferrites via a microemulsion-assisted precipitation method, Colloids Surf., A, 2005, 266, 168–174 CrossRef CAS.
- V. Uskokovic and M. Drofenik, Synthesis of materials within reverse micelles, Surf. Rev. Lett., 2005, 12, 239–277 CrossRef CAS.
- C. Aubery, C. Solans, S. Prevost, M. Gradzielski and M. Sanchez-Dominguez, Microemulsions as reaction media for the synthesis of mixed oxide nanoparticles: Relationships between microemulsion structure, reactivity, and nanoparticle characteristics, Langmuir, 2013, 29, 1779–1789 CrossRef CAS PubMed.
- C. Aubery, C. Solans and M. Sanchez-Dominguez, Tuning high aqueous phase uptake in nonionic water-in-oil microemulsions for the synthesis of Mn–Zn ferrite nanoparticles: Phase behavior, characterization, and nanoparticle synthesis, Langmuir, 2011, 27, 14005–14013 CrossRef CAS PubMed.
- M. Bellusci, S. Canepari, G. Ennas, A. La Barbera, F. Padella, A. Santini, A. Scano, L. Seralessandri and F. Varsano, Phase evolution in synthesis of manganese ferrite nanoparticles, J. Am. Ceram. Soc., 2007, 90, 3977–3983 CAS.
- J. Chandradass, A. H. Jadhav and H. Kim, Surfactant modified MgFe2O4 nanopowders by reverse micelle processing: Effect of water to surfactant ratio (R) on the particle size and magnetic property, Appl. Surf. Sci., 2012, 258, 3315–3320 CrossRef CAS.
- J. Chandradass and K. H. Kim, Solvent effects in the synthesis of MgFe2O4 nanopowders by reverse micelle processing, J. Alloys Compd., 2011, 509, L59–L62 CrossRef CAS.
- P. Holec, J. Plocek, D. Niznansky and J. P. Vejpravova, Preparation of MgFe2O4 nanoparticles by microemulsion method and their characterization, J. Sol-Gel Sci. Technol., 2009, 51, 301–305 CrossRef CAS.
- E. J. Choi, Y. Ahn and Y. D. Her, Size dependence of magnetic properties of Co-ferrite nanoparticles, J. Korean Phys. Soc., 2007, 50, 460–463 CrossRef CAS.
- A. J. Rondinone, A. C. S. Samia and Z. J. Zhang, Superparamagnetic Relaxation and Magnetic Anisotropy Energy Distribution in CoFe2O4 Spinel Ferrite Nanocrystallites, J. Phys. Chem. B, 1999, 103, 6876–6880 CrossRef CAS.
- A. J. Rondinone, A. C. S. Samia and Z. J. Zhang, A chemometric approach for predicting the size of magnetic spinel ferrite nanoparticles from the synthesis conditions, J. Phys. Chem. B, 2000, 104, 7919–7922 CrossRef CAS.
- R. D. K. Misra, A. Kale, B. J. Kooi and J. T. M. De Hosson, Some aspects of nanocrystalline nickel and zinc ferrites processed using microemulsion technique, Mater. Sci. Technol., 2003, 19, 1617–1621 CrossRef CAS.
- R. D. K. Misra, A. Kale, R. S. Srivastava and O. N. Senkov, Synthesis of nanocrystalline nickel and zinc ferrites by microemulsion technique, Mater. Sci. Technol., 2003, 19, 826–830 CrossRef CAS.
- M. A. Dar, J. Shah, W. A. Siddiqui and R. K. Kotnala, Influence of synthesis approach on structural and magnetic properties of lithium ferrite nanoparticles, J. Alloys Compd., 2012, 523, 36–42 CrossRef.
- M. Hashim, S. E. Shirsath, S. S. Meena, M. L. Mane, S. Kumar, P. Bhatt, R. Kumar, N. K. Prasad, S. K. Alla, J. Shah, R. K. Kotnala, K. A. Mohammed, E. Senturk and Alimuddin, Manganese ferrite prepared using reverse micelle process: Structural and magnetic properties characterization, J. Alloys Compd., 2015, 642, 70–77 CrossRef CAS.
- S. Kumar, P. Kumar, V. Singh, U. K. Mandal and R. K. Kotnala, Synthesischaracterization and magnetic properties of monodisperse Ni, Zn-ferrite nanocrystals, J. Magn. Magn. Mater., 2015, 379, 50–57 CrossRef CAS.
- S. Kumar, V. Singh, S. Aggarwal, U. K. Mandal and R. K. Kotnala, Synthesis of nanocrystalline Ni0.5Zn0.5Fe2O4 ferrite and study of its magnetic behavior at different temperatures, Mater. Sci. Eng., B, 2010, 166, 76–82 CrossRef CAS.
- A. Parveen, H. Asghar, M. Khalid, Z. A. Gilani, S. Aslam, M. Saleem, F. A. Shaikh and J. Rehman, Dielectric, impedance and modulus spectroscopic studies of Co0.3Cd0.7Zn1.5xFe2−xO4 nanoparticles, Appl. Phys. A: Mater. Sci. Process., 2019, 125, 731 CrossRef.
- C. Singh, A. Goyal and S. Singhal, Nickel-doped cobalt ferrite nanoparticles: efficient catalysts for the reduction of nitroaromatic compounds and photo-oxidative degradation of toxic dyes, Nanoscale, 2014, 6, 7959–7970 RSC.
- C. R. Vestal and Z. J. Zhang, Synthesis of CoCrFeO4 nanoparticles using microemulsion methods and size-dependent studies of their magnetic properties, Chem. Mater., 2002, 14, 3817–3822 CrossRef CAS.
- C. R. Vestal and Z. J. Zhang, Magnetic spinel ferrite nanoparticles from microemulsions, Int. J. Nanotechnol., 2004, 1, 240–263 CrossRef CAS.
- M. M. Baig, M. A. Yousuf, M. F. Warsi, P. O. Agboola, M. Sher and I. Shakir, Surfactant assisted synthesis of rare earth Dy3+ substituted MnFe2O4 nanoparticles, Ceram. Int., 2019, 45, 18014–18022 CrossRef CAS.
- S. Tajik and S. Khodabakhshi, Novel and feasible synthetic routes to copper ferrite nanoparticles: Taguchi optimization and photocatalytic application, J. Mater. Sci.: Mater. Electron., 2016, 27, 5175–5182 CrossRef CAS.
- R. Ali, M. A. Khan, A. Manzoor, M. Shahid, S. Haider, A. S. Malik, M. Sher, I. Shakir and M. FarooqWarsi, Investigation of structural and magnetic properties of Zr-Co doped nickel ferrite nanomaterials, J. Magn. Magn. Mater., 2017, 429, 142–147 CrossRef CAS.
- I. Ali, M. U. Islam, M. N. Ashiq, M. A. Iqbal, N. Karamat, M. S. Awan and S. Naseem, Role of Tb-Mn substitution on the magnetic properties of Y-type hexaferrites, J. Alloys Compd., 2014, 599, 131–138 CrossRef CAS.
- T. Koutzarova, S. Kolev, C. Ghelev, I. Nedkov, B. Vertruen, R. Cloots, C. Henrist and A. Zaleski, Differences in the structural and magnetic properties of nanosized barium hexaferrite powders prepared by single and double microemulsion techniques, J. Alloys Compd., 2013, 579, 174–180 CrossRef CAS.
-
T. Koutzarova, S. Kolev, K. Grigorov, C. Ghelev, A. Zaleski, R. E. Vandenberghe, M. Ausloos, C. Henrist, R. Cloots and I. Nedkov, in Nanostructured Materials, Thin Films and Hard Coatings for Advanced Applications, ed. L. Kolakieva and R. Kakanakov, 2010, vol. 159, pp. 57–62 Search PubMed.
- V. Pillai, P. Kumar, M. S. Multani and D. O. Shah, Structure and magnetic-properties of nanoparticles of barium ferrite synthesized using microemulsion processing, Colloids Surf., A, 1993, 80, 69–75 CrossRef CAS.
- G. Murtaza, R. Ahmad, T. Hussain, R. Ayub, I. Ali, M. A. Khan and M. N. Akhtar, Structural and magnetic properties of Nd-Mn substituted Y-type hexaferrites synthesized by microemulsion method, J. Alloys Compd., 2014, 602, 122–129 CrossRef CAS.
- M. Baghbanzadeh, L. Carbone, P. D. Cozzoli and C. O. Kappe, Microwave-assisted synthesis of colloidal inorganic nanocrystals, Angew. Chem., Int. Ed., 2011, 50, 11312–11359 CrossRef CAS PubMed.
- Y. Li and W. Yang, Microwave synthesis of zeolite membranes: A review, J. Membr. Sci., 2008, 316, 3–17 CrossRef CAS.
- C. O. Kappe, Controlled microwave heating in modern organic synthesis, Angew. Chem., Int. Ed. Engl., 2004, 43, 6250–6284 CrossRef PubMed.
- S. A. Galema, Microwave chemistry, Chem. Soc. Rev., 1997, 26, 233–238 RSC.
- L. Perreux and A. Loupy, A tentative rationalization of microwave effects in organic synthesis according to the reaction medium, and mechanistic considerations, Tetrahedron, 2001, 57, 9199–9223 CrossRef CAS.
- A. de la Hoz, A. Diaz-Ortiz and A. Moreno, Microwaves in organic synthesis. Thermal and non-thermal microwave effects, Chem. Soc. Rev., 2005, 34, 164–178 RSC.
- C. Gabriel, S. Gabriel, E. H. Grant, B. S. J. Halstead, P. Michael and D. Mingos, Dielectric parameters relevant to microwave dielectric heating, Chem. Soc. Rev., 1998, 27, 213–223 RSC.
- S. Horikoshi, F. Sakai, M. Kajitani, M. Abe and N. Serpone, Microwave frequency effects on the photoactivity of TiO2: Dielectric properties and the degradation of 4-chlorophenol, bisphenol A and methylene blue, Chem. Phys. Lett., 2009, 470, 304–307 CrossRef CAS.
- Y. J. Zhu and F. Chen, Microwave-assisted preparation of inorganic nanostructures in liquid phase, Chem. Rev., 2014, 114, 6462–6555 CrossRef CAS PubMed.
- M. B. Schütz, L. Xiao, T. Lehnen, T. Fischer and S. Mathur, Microwave-assisted synthesis of nanocrystalline binary and ternary metal oxides, Int. Mater. Rev., 2017, 63, 341–374 CrossRef.
- S. Komarneni, M. C. D'Arrigo, C. Leonelli, G. C. Pellacani and H. Katsuki, Microwave-Hydrothermal Synthesis of Nanophase Ferrites, J. Am. Ceram. Soc., 1998, 81, 3041–3043 CrossRef CAS.
- S. W. Cao, Y. J. Zhu, G. F. Cheng and Y. H. Huang, ZnFe2O4 nanoparticles: microwave-hydrothermal ionic liquid synthesis and photocatalytic property over phenol, J. Hazard. Mater., 2009, 171, 431–435 CrossRef CAS PubMed.
- Y. H. Choi, E. C. Ra, E. H. Kim, K. Y. Kim, Y. J. Jang, K. N. Kang, S. H. Choi, J. H. Jang and J. S. Lee, Sodium-Containing Spinel Zinc Ferrite as a Catalyst Precursor for the Selective Synthesis of Liquid Hydrocarbon Fuels, ChemSusChem, 2017, 10, 4764–4770 CrossRef CAS PubMed.
- L. Wu, H. Yao, B. Hu and S.-H. Yu, Unique Lamellar Sodium/Potassium Iron Oxide Nanosheets: Facile Microwave-Assisted Synthesis and Magnetic and Electrochemical Properties, Chem. Mater., 2011, 23, 3946–3952 CrossRef CAS.
- M. J. Williams, E. Sánchez, E. R. Aluri, F. J. Douglas, D. A. MacLaren, O. M. Collins, E. J. Cussen, J. D. Budge, L. C. Sanders, M. Michaelis, C. M. Smales, J. Cinatl, S. Lorrio, D. Krueger, R. T. M. de Rosales and S. A. Corr, Microwave-assisted synthesis of highly crystalline, multifunctional iron oxide nanocomposites for imaging applications, RSC Adv., 2016, 6, 83520–83528 RSC.
- T. Caillot, D. Aymes, D. Stuerga, N. Viart and G. Pourroy, Microwave flash synthesis of iron and magnetite particles by disproportionation of ferrous alcoholic solutions, J. Mater. Sci., 2002, 37, 5153–5158 CrossRef CAS.
- E. Solano, C. Frontera, T. Puig, X. Obradors, S. Ricart and J. Ros, Neutron and X-ray diffraction study of ferrite nanocrystals obtained by microwave-assisted growth. A structural comparison with the thermal synthetic route, J. Appl. Crystallogr., 2014, 47, 414–420 CrossRef CAS.
- R. Sai, S. D. Kulkarni, K. J. Vinoy, N. Bhat and S. A. Shivashankar, ZnFe2O4: Rapid and sub-100 °C synthesis and anneal-tuned magnetic properties, J. Mater. Chem., 2012, 22, 2149–2156 RSC.
- F. Bensebaa, F. Zavaliche, P. L'Ecuyer, R. W. Cochrane and T. Veres, Microwave synthesis and characterization of Co-ferrite nanoparticles, J. Colloid Interface Sci., 2004, 277, 104–110 CrossRef CAS PubMed.
- J. Giri, T. Sriharsha and D. Bahadur, Optimization of parameters for the synthesis of nano-sized Co1−xZnxFe2O4, (0 ≤ x ≤ 0.8) by microwave refluxing, J. Mater. Chem., 2004, 14, 875–880 RSC.
- Z. Kozakova, I. Kuritka, N. E. Kazantseva, V. Babayan, M. Pastorek, M. Machovsky, P. Bazant and P. Saha, The formation mechanism of iron oxide nanoparticles within the microwave-assisted solvothermal synthesis and its correlation with the structural and magnetic properties, Dalton Trans., 2015, 44, 21099–21108 RSC.
- A. Garzón-Manjón, E. Solano, M. de la Mata, R. Guzmán, J. Arbiol, T. Puig, X. Obradors, R. Yáñez, S. Ricart and J. Ros, Induced shape controllability by tailored precursor design in thermal and microwave-assisted synthesis of Fe3O4 nanoparticles, J. Nanopart. Res., 2015, 17, 291 CrossRef.
- E. Bartolomé, P. Cayado, E. Solano, S. Ricart, J. Gázquez, B. Mundet, M. Coll, T. Puig, X. Obradors, M. Valvidares, J. Herrero-Martín, P. Gargiani and E. Pellegrin, Magnetic stability against calcining of microwave-synthesized CoFe2O4 nanoparticles, New J. Chem., 2016, 40, 6890–6898 RSC.
- E. Solano, L. Perez-Mirabet, F. Martinez-Julian, R. Guzmán, J. Arbiol, T. Puig, X. Obradors, R. Yañez, A. Pomar, S. Ricart and J. Ros, Facile and efficient one-pot solvothermal and microwave-assisted synthesis of stable colloidal solutions of MFe2O4 spinel magnetic nanoparticles, J. Nanopart. Res., 2012, 14, 1034 CrossRef.
- T. Schneider, A. Löwa, S. Karagiozov, L. Sprenger, L. Gutiérrez, T. Esposito, G. Marten, K. Saatchi and U. O. Häfeli, Facile microwave synthesis of uniform magnetic nanoparticles with minimal sample processing, J. Magn. Magn. Mater., 2017, 421, 283–291 CrossRef CAS.
- J. Nandhini, P. Neeraja, S. Rex Jeya Rajkumar, V. Umapathy and S. Suresh, Comparative Studies of Microwave and Sol–Gel-Assisted Combustion Methods of NiFe2O4 Nanostructures: Synthesis, Structural, Morphological, Opto-magnetic, and Antimicrobial Activity, J. Supercond. Novel Magn., 2016, 30, 1213–1220 CrossRef.
- S. Javadi, S. M. Masoudpanah and A. Zakeri, Conventional versus microwave combustion synthesis of CoFe2O4 nanoparticles, J. Sol-Gel Sci. Technol., 2016, 79, 176–183 CrossRef CAS.
- I. Bilecka, M. Kubli, E. Amstad and M. Niederberger, Simultaneous formation of ferrite nanocrystals and deposition of thin films via a microwave-assisted nonaqueous sol–gel process, J. Sol-Gel Sci. Technol., 2010, 57, 313–322 CrossRef.
- C. Suchomski, B. Breitung, R. Witte, M. Knapp, S. Bauer, T. Baumbach, C. Reitz and T. Brezesinski, Microwave synthesis of high-quality and uniform 4 nm ZnFe2O4 nanocrystals for application in energy storage and nanomagnetics, Beilstein J. Nanotechnol., 2016, 7, 1350–1360 CrossRef CAS PubMed.
- O. Pascu, M. Gich, G. Herranz and A. Roig, 2D Magnetic Frames Obtained by the Microwave-Assisted Chemistry Approach, Eur. J. Inorg. Chem., 2012, 2012, 2656–2660 CrossRef CAS.
- L. F. da Silva, W. Avansi, M. L. Moreira, J. Andrés, E. Longo and V. R. Mastelaro, Novel SrTi1−xFexO3 nanocubes synthesized by microwave-assisted hydrothermal method, CrystEngComm, 2012, 14, 4068–4073 RSC.
- J. Martynczuk, M. Arnold, H. Wang, J. Caro and A. Feldhoff, How (Ba0.5Sr0.5)(Fe0.8Zn0.2)O3−δ and (Ba0.5Sr0.5)(Co0.8Fe0.2)O3−δ perovskites form via an EDTA/citric acid complexing method, Adv. Mater., 2007, 19, 2134–2140 CrossRef CAS.
- K. Chybczynska, M. Blaszyk, B. Hilczer, T. Lucinski, M. Matczak and B. Andrzejewski, PEG-controlled thickness of BiFeO3 crystallites in microwave hydrothermal synthesis, Mater. Res. Bull., 2017, 86, 178–185 CrossRef CAS.
- S. Li, G. Zhang, H. Zheng, N. Wang, Y. Zheng and P. Wang, Microwave-assisted synthesis of BiFeO3 nanoparticles with high catalytic performance in microwave-enhanced Fenton-like process, RSC Adv., 2016, 6, 82439–82446 RSC.
- J. Huang, G. Tan, W. Yang, L. Zhang, H. Ren and A. Xia, Microwave hydrothermal synthesis of BiFeO3: Impact of different surfactants on the morphology and photocatalytic properties, Mater. Sci. Semicond. Process., 2014, 25, 84–88 CrossRef CAS.
- G. Kolhatkar, F. Ambriz-Vargas, B. Huber, R. Thomas and A. Ruediger, Thermionic Emission Based Resistive Memory with Ultrathin Ferroelectric BiFe1−xCrxO3 Films Deposited by Mineralizer-Free Microwave-Assisted Hydrothermal Synthesis, Cryst. Growth Des., 2018, 18, 1864–1872 CrossRef CAS.
- J. M. Byrne, V. S. Coker, E. Cespedes, P. L. Wincott, D. J. Vaughan, R. A. D. Pattrick, G. van der Laan, E. Arenholz, F. Tuna, M. Bencsik, J. R. Lloyd and N. D. Telling, Biosynthesis of zinc substituted magnetite nanoparticles with enhanced magnetic properties, Adv. Funct. Mater., 2014, 24, 2518–2529 CrossRef CAS.
- S. H. Xiao, W. F. Jiang, L. Y. Li and X. J. Li, Low-temperature auto-combustion synthesis and magnetic properties of cobalt ferrite nanopowder, Mater. Chem. Phys., 2007, 106, 82–87 CrossRef CAS.
- S. Diodati, L. Nodari, M. M. Natile, A. Caneschi, C. de Julián Fernández, C. Hoffmann, S. Kaskel, A. Lieb, V. Di Noto, S. Mascotto, R. Saini and S. Gross, Coprecipitation of oxalates: an easy and reproducible wet-chemistry synthesis route for transition metal ferrites., Eur. J. Inorg. Chem., 2014, 875–887 CrossRef CAS.
- J. Gass, H. Srikanth, N. Kislov, S. S. Srinivasan and Y. Emirov, Magnetization and magnetocaloric effect in ball-milled zinc ferrite powder, J. Appl. Phys., 2008, 103, 07B309 CrossRef.
- M. Ghaffari, P. Y. Tan, M. E. Oruc, O. K. Tan, M. S. Tse and M. Shannon, Effect of ball milling on the characteristics of nanostructure SrFeO3 powder for photocatalytic degradation of methylene blue under visible light irradiation and its reaction kinetics, Catal. Today, 2011, 161, 70–77 CrossRef CAS.
- R. M. Glaister, N. A. Allen and N. J. Hellicar, Comparison of methods for preparing fine ferrite powders, Proc. Br. Ceram. Soc., 1965,(3), 67–80 CAS.
- V. G. Harris, D. J. Fatemi, J. O. Cross, E. E. Carpenter, V. M. Browning, J. P. Kirkland, A. Mohan and G. J. Long, One-step processing of spinel ferrites via the high-energy ball milling of binary oxides, J. Appl. Phys., 2003, 94, 496–501 CrossRef CAS.
- D. Chen and H.-y. Liu, One-step synthesis of nickel ferrite nanoparticles by ultrasonic wave-assisted ball milling technology, Mater. Lett., 2012, 72, 95–97 CrossRef CAS.
- D. Chen, H. y. Liu and L. Li, One-step synthesis of manganese ferrite nanoparticles by ultrasonic wave-assisted ball milling technology, Mater. Chem. Phys., 2012, 134, 921–924 CrossRef CAS.
- M. A. Gabal, A. A. Al-Juaid, S. M. Al-Rashed, M. A. Hussein and F. Al-Marzouki, Synthesis, characterization and electromagnetic properties of Zn-substituted CoFe2O4 via sucrose assisted combustion route, J. Magn. Magn. Mater., 2017, 426, 670–679 CrossRef CAS.
- S. A. Saafan, S. T. Assar and S. F. Mansour, Magnetic and electrical properties of Co1−xCaxFe2O4 nanoparticles synthesized by the auto combustion method, J. Alloys Compd., 2012, 542, 192–198 CrossRef CAS.
- A. Sutka and G. Mezinskis, Sol-gel auto-combustion synthesis of spinel-type ferrite nanomaterials, Front. Mater. Sci., 2012, 6, 128–141 CrossRef.
- F. Deganello, L. F. Liotta, A. Longo, M. P. Casaletto and M. Scopelliti, Cerium effect on the phase structure, phase stability and redox properties of Ce-doped strontium ferrates, J. Solid State Chem., 2006, 179, 3406–3419 CrossRef CAS.
Footnote |
† Electronic supplementary information (ESI) available: Table summarising examples of hydrothermal syntheses of BiFeO3. See DOI: 10.1039/d0qi00294a |
|
This journal is © the Partner Organisations 2020 |
Click here to see how this site uses Cookies. View our privacy policy here.