DOI:
10.1039/C8AY01974F
(Paper)
Anal. Methods, 2019,
11, 78-87
Detection of microplastics in local marine organisms using a multi-technology system
Received
9th September 2018
, Accepted 12th November 2018
First published on 14th November 2018
Abstract
Microplastics with complex polymer compositions are present in a lot of marine organisms. In this study, successive stereo microscopy and micro-Fourier transform infrared spectroscopy equipped with attenuated total reflection (μ-ATR-FTIR) in combination with scanning electron microscopy-energy dispersive spectroscopy (SEM-EDS) were implemented to establish a highly accurate microplastics detection system. The method was applied to analyze microplastics in both the soft tissue and the digestive tract of bivalves and fish collected from the markets in Qingdao and Dongying. The results showed that the individual detection rate of microplastics was higher in the fish than that in the bivalves and that the abundance of microplastics measured in items per individual was significantly higher in the fish than that in the bivalves. Four shapes of microplastics, including fibers, fragments, granules and films, were separated from the organisms above. Fibrous microplastics, being the most dominant ones, accounted for over 70% in different organisms. The average size of the fibrous microplastics was smaller than that of the other three shapes of microplastics. The number of microplastics decreased with increasing microplastics sizes. Microplastics of less than 1 mm obtained from different organisms were in the range of 43% to 78%. Rayon (a semi-synthetic polymer) was the most predominant polymer type of microplastics found, accounting for 48.92%. The demersal fish contained relatively more rayon compared with the pelagic fish samples. Surface chemical components of the microplastics were altered possibly owing to the abiotic oxidation. Large variations of the weathering morphologies were observed in the surface of the differently shaped microplastics originating from the organisms. Some microplastics exhibited a rough surface, broken margins, and pronounced pores. SEM-EDS, as an auxiliary technology, would provide a way for data calibration in microplastics investigation. The combination method can provide complementary data and therefore can be successfully applied to accurately identify microplastics.
1. Introduction
The presence of microplastics (defined as particles <5 mm in diameter1) in marine environments has gained global attention. The sources of microplastics are diverse, mainly including raw materials used in the plastics industry, plastic particles or debris from large plastics degraded by various physical processes, additives from common daily items, and polishing materials used in industrial production.2 Small and ubiquitous plastic particles, microplastics, have been frequently reported in seawater, sediments, biota, sea salt,3 and even in the polar regions.4 Plastics are considered to be quite stable and highly durable, potentially lasting hundreds to thousands of years.5 A potential environmental risk of microplastics is their bioavailability to organisms throughout the food-web.6,7 Adverse effects on hatching, growth, feeding and innate behaviors have been observed in European perch (Perca fluviatilis) larvae after exposure to microplastics in the laboratory.8 In addition, microplastics can adsorb persistent organic pollutants and metals owing to their composition and relatively large surface area.7 Pollutants and plasticizers in microplastics can be released in the gastrointestinal fluids and potentially be transferred to the edible tissue9,10 of organisms. The desorption rate of pollutants in microplastics under gut conditions can be up to 30 times greater than that in seawater alone,11 and the accumulated pollutants are hazardous to biological tissues.12 In addition to the toxic effects on individuals exerted by microplastics, ingested microplastics can transfer from low-level organisms to high-level organisms along the food chain13 and may eventually appear on our tables as seafood. Unfortunately, the implications associated with microplastics on food safety and human health are not yet fully understood.14,15
Microplastics have been detected in various aquatic organisms across multiple trophic levels, including zooplankton,2,16,17 worms,18 bivalves,19–21 fish,22,23 seabirds24 and cetaceans.25,26 In previous published studies, scholars have focused on microplastics abundance, shape, color and polymer type in organisms. In the present study, the abundance of microplastics in organisms varied significantly. For example, the average abundance of microplastics in zooplankton collected from the northern South China Sea2 can reach 131.5 items per m3, and that detected in the digestive tract of natural commercial fish in the Mondego estuary (Portugal)22 can reach 1.67 items per individual. Microplastics identification is one of the crucial steps during microplastics investigation.
Challenges arise in microplastics identification. Various approaches to validate microplastics are provided in the literature. Commonly used approaches include visual identification27 (with the aid of a microscope), Nile red staining,28 Fourier Transform Infrared (FT-IR) spectroscopy,29 Raman spectrometry,30 scanning electron microscopy-energy dispersive spectroscopy (SEM-EDS),31 and pyrolysis gas chromatography-mass spectrometry (py-GC-MS).32 However, no single technique mentioned above can obtain the physical (shape, color, and size) and chemical (polymer type) features at the same time. Interference factors, size limitation and time constraints can all limit the application of a single method. The minimal size of the microplastics to be identified is a critical factor to consider when selecting the identification tool.33 Hence, a method that reliably conducts the physical and chemical characterization is suitable for microplastics analysis.33
SEM-EDS can not only provide high-resolution images of the surface texture of microplastics, but also analyze the elemental composition of particles, particularly identifying carbon-dominant plastics from inorganic particles. It is useful to observe smaller particles by SEM which may be missed on using an optical microscope. FT-IR was commonly used for microplastics identification in previous studies. Attenuated total reflection (ATR) mode is available in FT-IR analysis for microplastics and was successfully used to analyze filtered microplastics samples by Song et al.34 Additionally, smaller microplastics (e.g., 7 μm) are detectable by μ-ATR-FTIR rather than FT-IR. To our knowledge, microplastics analysis by FT-IR spectroscopy as well as by SEM-EDS has been described in a previous study.20 However, this report used SEM-EDS to characterize the surface morphology of fibrous and fragmented microplastics as well as to distinguish diatoms from microplastics, except with regard to the systematic application of the method combining SEM-EDS and FT-IR.
The present study investigated the occurrence and characteristics of microplastics in bivalves and fish which are of high commercial value. The species selected included organisms from different habitats and with different feeding strategies, i.e. sessile mollusks, and demersal and pelagic fish species, reflecting microplastics contamination in different aquatic environments. Polymer type identification, surface morphology characterization and elemental composition analysis were conducted by combining multiple technologies. The aim of this research is to provide basic data and scientific evidence for environmental/ecological risk assessment and toxicology research.
2. Experimental
2.1 Materials and reagents
Live bivalves and frozen fish were used for our experiments. Mytilus galloprovincialis, Ruditapes philippinarum, Pseudosciaena polyactis (pelagic fish), and Pleuronichthys cornutus (demersal fish) were purchased from the local fish market in Qingdao, China, in December 2017. We also purchased Mytilus galloprovincialis, Mactra veneriformis, Sebastes schlegelii (demersal fish), and Liza haematocheila (demersal fish) from the biggest fish market in Dongying, China at the same time. The samples were individually wrapped in aluminium foil and transferred to the laboratory and stored at −20 °C prior to microplastics analysis. Potassium hydroxide (AR grade) was purchased from Sinopharm Chemical Reagent Co., Ltd (Shanghai, China). Ultrapure water (18.2 MΩ cm) was prepared using a Milli-Q system (Millipore Co., USA).
2.2 Microplastics extraction from the biological samples
In the laboratory, the biological samples were thawed before examination. Bivalves were selected based on similar specifications in terms of basic parameters including the shell length and whole body wet weight. The shell length of bivalves was measured using a vernier caliper. The whole body was weighed with an electronic balance (ME104/02, Shanghai, China). The soft tissue removed from the shell was characterized, and the results are shown in Table 1. The microplastics were separated from the organisms following our previously published methods.35 Briefly, the removed soft tissue was placed in a 250 mL conical flask. After adding 100 mL of 10% KOH solution, the flask was covered with aluminium foil. The conical flask was sonicated for 5 min using a high-power digital ultrasonic instrument (KQ-400KDE, Kunshan, China) and then placed in an oscillation incubator (Zhichu ZQLY-180S, Shanghai, China) at 90 rpm for 24 h at 60 °C in order to remove the organic matter. The digested solution was filtered through 0.7 μm pore size and 47 mm diameter glass fiber membranes (Whatman GF/F, UK) using a vacuum system (SHB-IIIA, Zhengzhou, China) without cooling. The membranes were then placed in clean Petri dishes and dried in an electric thermostat blast drying oven (Shanghai, China) set at 50 °C. Fish samples were selected based on similar body lengths (length from the snout to the end of the tail vertebrae) and body wet weights. The specifications are shown in Table 2. For each fish, the complete digestive tract (esophagus, stomach and intestine) was removed, weighed and placed in a 250 mL conical flask. The pretreatment method was similar to that of the bivalve sample, and the digestion time of the fish sample was 24–48 h.
Table 1 Basic data for the analyzed bivalve samples and their corresponding levels of microplastics ingestion
Sampling area |
Species |
Number |
Shell length (cm) |
Whole body wet weight (g per individual) |
Soft tissue weight (g per individual) |
Mean microplastics per bivalve (items per individual) |
Mean microplastics per gram (items per g) |
Individual detection rate (%) |
Average ± standard deviation.
|
Qingdao |
Mytilus galloprovincialis
|
10 |
7.61 ± 0.97a |
28.62 ± 4.02 |
9.76 ± 1.91 |
1.40 ± 1.20 |
0.16 ± 0.13 |
70 |
Qingdao |
Ruditapes philippinarum
|
10 |
3.65 ± 0.15 |
7.00 ± 1.07 |
2.64 ± 0.47 |
1.90 ± 1.44 |
0.74 ± 0.54 |
90 |
Dongying |
Mytilus galloprovincialis
|
10 |
6.15 ± 0.43 |
12.21 ± 2.43 |
4.37 ± 1.05 |
1.80 ± 1.07 |
0.42 ± 0.26 |
80 |
Dongying |
Mactra veneriformis
|
10 |
3.54 ± 0.21 |
12.79 ± 3.43 |
4.75 ± 1.65 |
1.20 ± 0.97 |
0.31 ± 0.27 |
70 |
Table 2 Basic data for the analyzed fish samples and their corresponding levels of microplastics ingestion
Sampling area |
Species |
Number |
Length (cm) |
Total body wet weight (g per individual) |
Wet weight of the digestive tract (g per individual) |
Mean microplastics per fish (items per individual) |
Mean microplastics per gram (items per g) |
Individual detected rate (%) |
Average ± standard deviation.
|
Qingdao |
Pseudosciaena polyactis
|
10 |
20.25 ± 0.34a |
156.65 ± 6.97 |
4.81 ± 0.82 |
2.40 ± 0.66 |
0.51 ± 0.16 |
100 |
Qingdao |
Pleuronichthys cornutus
|
10 |
18.28 ± 0.90 |
194.00 ± 24.17 |
13.65 ± 9.71 |
3.90 ± 2.21 |
0.58 ± 0.83 |
90 |
Dongying |
Sebastes schlegeli
|
10 |
21.82 ± 0.92 |
309.38 ± 19.11 |
25.34 ± 6.20 |
2.30 ± 1.95 |
0.09 ± 0.06 |
90 |
Dongying |
Liza haematocheila
|
10 |
20.44 ± 0.82 |
106.20 ± 11.74 |
11.13 ± 1.26 |
3.70 ± 2.57 |
0.35 ± 0.27 |
90 |
Blank experiments were carried out using the same setup but without any tissue. To minimize environmental contamination, solution preparation and biological dissection were always conducted in a semi-closed space. Cotton lab coats were always worn throughout the experiment. In addition, all of the containers and conical flasks were rinsed at least three times with ultrapure water before being used. Samples and solutions were always covered with aluminium foil to avoid contamination.
2.3 Optical microscopy and spectroscopic analysis
The enriched microplastics on the membrane were observed under a stereo microscope (Nikon SMZ1270, Japan), and images (20–80× magnification) were obtained with a Nikon Ds-Ri2 digital camera. Microplastics and suspected microplastic particles were selected and identified using a PerkinElmer Spectrum Spotlight 400 micro-Fourier transform infrared spectrometer (μ-FT-IR) (PerkinElmer Inc., USA) equipped with a liquid nitrogen-cooled mercury cadmium telluride (MCT) array detector and attenuated total reflection (ATR) attachment consisting of a germanium (Ge) crystal. The spectrum range was from 4000 to 750 cm−1 with a spectral resolution of 8 cm−1. Sixteen co-scans were performed in each measurement. The scanning area ranged from 100 μm × 100 μm to 1000 μm × 1000 μm. The ATR imaging attachment was in direct contact with the microplastics on the filter membrane during data acquisition. Polymer type analysis and the characterization of functional groups were conducted according to the acquired data. All of the spectra were compared with the reference spectra from the Sadtler Library, and the characteristic peaks of the functional groups were combined to determine the polymer type. When interpreting the FTIR output, only readings with confidence levels of 70% or greater and those considered to have reliable spectrum matches (after visual inspection) were accepted.36 All confirmed polymer types are included in our results.
2.4 SEM-EDS analysis
Representative microplastics on the filter membrane were selected for SEM analysis. Microplastics were placed on double-sided adhesive tape and coated with a thin film of evaporated gold (Au) using an ion sputtering instrument (Hitachi E-1045, Japan). The morphology of the microplastics was examined under a SEM (Hitachi S-4800, Japan). The elemental composition of the microplastics surface was obtained by EDS (Horiba EX-350, Japan).
3. Results and discussion
3.1 Individual detection rate and abundance of microplastics
In our experiment, extreme precautions were taken to prevent airborne microplastics. Our results showed no microplastics on the filter of the blank samples. For statistical analysis, 10 individuals of each species were used in our study. Different levels of microplastics were present in the biological samples collected from the two sampling areas. The individual detection rate of microplastics in the bivalves varied between 70% and 90% (Table 1), whereas that in the fish varied between 90% and 100% (Table 2). Therefore, the individual detection rate of microplastics in the fish was higher than that in the bivalves.
In the bivalve samples, the number of encountered microplastics varied from 1.20 to 1.90 items per individual and from 0.16 to 0.74 items per g (wet weight) (Table 1). The average abundance of microplastics in the fish ranged from 2.30 to 3.90 items per individual and from 0.09 to 0.58 items per g (Table 2). Statistical analysis indicates that the abundance of microplastics extracted from Mytilus galloprovincialis measured in items per g was higher in the samples from Dongying than those from Qingdao (p = 0.018, T-test), while the abundance measured in items per individual did not exhibit a statistically significant variation (p = 0.47, T-test). The abundance of microplastics measured in items per individual was significantly higher in the fish than that in the bivalves (p = 0.00029, T-test), while the abundance of microplastics measured in items per g between fish and bivalve samples did not appear to have a statistically significant variation (p = 0.8, T-test).
The average abundance of microplastics was the highest in Ruditapes philippinarum collected from Qingdao either by individual (1.90 items per individual) or by weight (0.74 items per g) compared with the other bivalves. Compared with previous studies, the average number of microplastics detected in the mussels of this study (1.40 ± 1.20 items per individual and 1.80 ± 1.07 items per individual, respectively, sampled from Qingdao and Dongying) resembled values reported in the northern Ionian Sea, Greece (1.9 ± 0.2 items per individual)10 and in Giglio Island, Italy (1–2 items per individual),37 but lower than those reported in coastal waters of China (1.5 to 7.6 items per individual).20 These variations could be associated with the different environments the organisms grow in or different sampling times. In this research, Pleuronichthys cornutus (benthic fish) purchased from Qingdao contained 3.90 items of microplastics per individual (0.57 items per g), demonstrating the highest contamination levels of microplastics compared with other fish samples. The vertical distribution of the fish might affect the levels of ingested microplastics.22 This result might be related to the presence of plastic debris near the seafloor, indicating some microplastics have a tendency to sink in the aquatic environment. The average level of microplastics observed in the Pleuronichthys cornutus of this study (3.90 ± 2.21 items per individual) was higher than that reported in the Platichthys flesus of Mondego estuary, Portugal (0.18 ± 0.55 items per individual),22 indicating the location difference between the study of Bessa et al. and our study.
3.2 Shapes, colors and sizes of microplastics in the organisms
Multiple shapes of microplastics, including fibers, fragments, films and granules, were detected in the organisms (Fig. 1). Fibrous microplastics, being the most predominant ones in different organisms, accounted for over 70% of the total microplastics. More specifically, in mussels, 100% of the microplastics were categorized as fiber. The other three shapes of microplastics showed different distribution characteristics but lower abundance in the different organisms (Fig. 2(a)). In general, the detection rate of the different shapes was in the order of fiber > fragment > film > granule. The shape distribution characteristics exhibited in this study may be relevant to domestic sewage discharge, particularly coming from laundry. For example, an estimated 0 to 2 g of microfibres were lost per garment as reported by Hartline et al.,38 and Browne et al. found that as many as 1900 microfibres were lost from each piece of clothing into the environment with each wash cycle.39 This result is in agreement with the reported results from other researchers.15,23
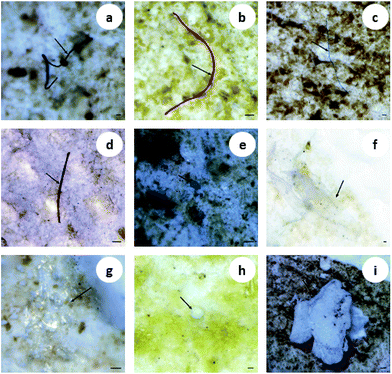 |
| Fig. 1 Images of different shapes of microplastics in biological samples. The arrows indicate fibers (a–d), fragments (e and f), the film (g) and granules (h and i). Scale bar = 100 μm. | |
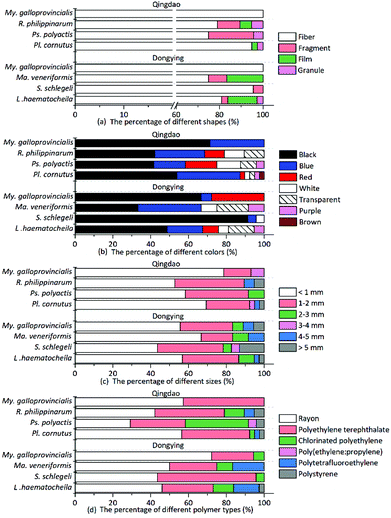 |
| Fig. 2 Percentage of the detected microplastics categorized by shape (a), color (b), size (c), and polymer type (d). | |
Microplastics detected in the organisms exhibited a variety of colors. The most prevalent colors observed in the fibers were black, blue and red (Fig. 1(a–d)). The fragments were mostly blue and transparent (Fig. 1(e and f)). The films were transparent (Fig. 1(g)), and the granules were usually white (Fig. 1(h and i)). The proportion of deep colors (black, blue, red, purple and brown) was 88.17%, larger than 11.83% of light colors (transparent and white) (Fig. 2(b)). Our results showed black to be the most common color (55.91%), followed by blue (20.97%) and red (8.06%). Similarly, Lusher et al. found black microplastics were the most abundant ones accounting for 45% of the microplastics found.40 Bellas et al. reported 51% of the particles detected in demersal fish were black microplastics.41 However, Li et al. reported the reverse result that the proportion of light colors (transparent, white, green and yellow) was significantly larger than that of deep colors (blue, brown, black and red).15
To measure the microplastics sizes, the fibrous ones were measured along their actual length, while the fragmented, granular and filmy microplastics were measured through their longest dimension. Statistically, the microplastics size ranged from 57 to 8639 μm in the examined organisms. Microplastics less than 1 mm in size were the most common ones and comprised 43–78% of the total detected microplastics. And the number of microplastics from the different organisms decreased with increasing sizes (Fig. 2(c)). This result is in accordance with previous reports.42 Smaller-sized plastic particles are more likely to be ingested by organisms and pose a higher risk.42–44 In addition, the size distribution of microplastics with different shapes showed great variations. The average size of fragmented microplastics was the largest, reaching (1.61 ± 1.59) mm; the average sizes of filmy and granular microplastics were (1.30 ± 0.83) mm and (1.27 ± 1.70) mm, respectively; and the average size of fibrous microplastics was the smallest (1.23 ± 1.45 mm). Therefore, the fibrous microplastics were the most dominant ones with the smallest average size.
3.3 Microplastics polymer types in organisms
The polymer type will dictate the transport and fate of marine debris and its affinity for other chemical pollutants.45 In the present study, a total of 186 particles were confirmed as microplastics using μ-FT-IR attached with ATR. Polymers were identified based on the matching with the spectral database and the presence of featured adsorption bands as shown in Fig. 3 and as described in Table 3. The matching degree in this study between the sample spectra and the standard spectra was greater than 89%. However, chemical weathering, natural aging, and biochemical processes affecting ingested plastics can modify their spectral features, making identification difficult.46 The spectrum of CPE differed from the standard spectrum presented in Fig. 3(b). Two bands at 2916 and 2848 cm−1 can be assigned to the CH2 stretching vibration, and two bands at 1469 and 1262 cm−1 can be assigned to the CH2 bending vibration. Additionally, the weak band at 1744 cm−1 (C
O stretching esters47) can be attributed to the presence of oxygen-containing functional groups formed during abiotic oxidation of CPE.48,49 Previous reports demonstrated that ultraviolet radiation, thermal oxidation and humidity could alter the chemical structure of microplastics through oxidation reactions.50–52 Comparing the infrared spectrum of PTFE and its standard spectrum (Fig. 3(c)), the weak band at 1027 cm−1 (phenolic band53) can be assigned to the C–O functional group.
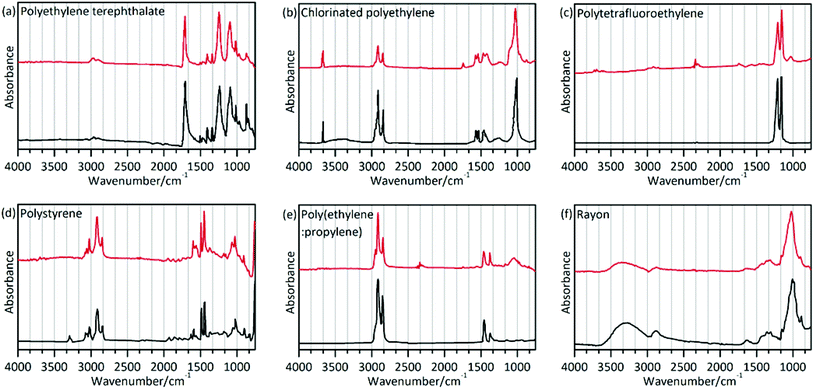 |
| Fig. 3 Examples of Fourier transform infrared spectra (top, in red) of detected microplastics and the corresponding reference spectra from the Sadtler Library (bottom, in black). The horizontal axis represents the spectral range and vertical axis represents absorbance. (a) Polyethylene terephthalate. (b) Chlorinated polyethylene. (c) Polytetrafluoroethylene. (d) Polystyrene. (e) Poly(ethylene:propylene). (f) Rayon. | |
Table 3 Infrared characteristics analysis of the analyzed microplasticsa
Sample |
Polymer type |
Match (%) |
Characteristic band (cm−1) |
Assignment |
Reference |
ν: stretching vibration; δ: bending vibration.
|
a |
Polyethylene terephthalate (PET) |
93 |
2965, 2898 |
ν(CH) |
61
|
1713 |
ν(C O) |
1408 |
δ(ring) |
1338 |
δ(CH) |
1242, 1097 |
ν(COC) |
1018 |
δ(CH ring) |
b |
Chlorinated polyethylene (CPE) |
89 |
2916, 2848 |
ν(CH2) |
61
|
1469, 1262 |
δ(CH2) |
c |
Polytetrafluoroethylene (PTFE) |
91 |
1208 |
ν(CF2) |
61
|
1152 |
ν(CF2) |
d |
Polystyrene (PS) |
93 |
3023 |
ν(CH ring) |
61
|
2849 |
ν(CH) |
1600, 1492 |
ν(ring) |
1451 |
δ(CH2) |
1027 |
δ(CH ring) |
752 |
δ(CH ring) |
e |
Poly(ethylene:propylene) (60% ethylene; PE + PP) |
92 |
2915, 2847 |
ν(CH2) |
61
|
1460 |
δ(CH2) |
1375 |
δ(CH3) |
f |
Rayon |
89 |
3650–3000 |
ν(OH) |
61
|
2990–2820 |
ν(CH) |
1425, 1372, 1320 |
δ(CH) |
1200–1000 |
δ(C–OH) + δ(C–C) + ν(C–O–C) + ν(C–OH) |
The spectrum of rayon (Fig. 3(f)) is similar to that of cotton or pure cellulose. This raises a question on how to differentiate rayon from natural fibers. A previous study used visual identification methods to analyze fibers,25 which might cause underestimation or overestimation of the fiber number. In the present study, chemical structure analysis was implemented to distinguish man-made fibers like rayon as described in the study by Comnea-Stancu et al.54 We also take into account the higher database search match. The results showed that spectral characteristics of the O–H stretching band of rayon were broad and featureless, while the absorption bands at 1735, 1105 and 1051 cm−1 have not been observed which may appear in several natural fiber samples. The characteristic profile of the spectra in this study is in accordance with prior reports.22
The different microplastics polymer types in different organisms are shown in Fig. 2(d). Various polymer types were validated, including rayon (48.92%), polyethylene terephthalate (PET, 33.87%), chlorinated polyethylene (CPE, 9.68%), polytetrafluoroethylene (PTFE, 4.84%), polystyrene (PS, 2.15%), and the polyethylene–polypropylene copolymer (PE + PP, 0.54%). Different shapes of microplastics were also documented, and the results indicated that fibrous microplastics were usually rayon, PET and CPE. Fragmented microplastics were usually comprised of the PE–PP copolymer and CPE. The polymers were PTFE for the films and PS for the granules.
The results showed that rayon (a semi-synthetic fiber22 and a regenerated cellulose fiber29) was the most abundant polymer type in all determined microplastics. Indeed, our results were consistent with other published studies, with rayon or a rayon-plastic polymer mix making up 55% of the analyzed particles in coastal waters near Plymouth, UK.55 Another study also reported that rayon is the most common fiber (53%) detected in the True's beaked whale (Mesoplodon mirus) stranded on the north and west coasts of Ireland.25 In addition, in the analyzed fish samples, more rayon fibers were found in the three demersal fish (average proportion: 48.61%) than in other pelagic fish (29.17%). Rayon fibers, with higher density (1.7–1.8 g cm3)56 than sea water (1.025 g cm3), are more likely to sink in the marine environment and affect demersal fish. Some possible sources of rayon can be attributed to the release of clothing, furnishing, or hygiene products from sewage.40 Given their low crystallinity and low degree of orientation, items made of rayon can disintegrate rapidly,57 hence leading to their high abundance. Rayon was described as a microplastic by some references,22,25,40,58 Therefore, we include it in our study as well. However, whether rayon should be considered as a microplastic deserves further discussion. PET made up the second largest portion of microplastics detected in the organisms. PET is one of the most common consumer plastics used and is increasingly used in the textile industry to produce clothes, nonwoven fabrics and carpets.59,60 Therefore, high amounts of microplastics like rayon and PET might come from textiles/clothing.
3.4 Surface morphological characteristics of the detected microplastics
Fibrous and filmy microplastics were selected from the soft tissue of Mytilus galloprovincialis, while granular microplastics was acquired from the digestive tract of Pseudosciaena polyactis. The surface morphologies of the representative microplastics were further observed by SEM (Fig. 4). The three shapes of microplastics exhibited different weathering morphologies. The surface of the fibers was either concave or convex, and based on the partially enlarged pictures, the surface had more scratches than pores and protrusions (Fig. 4(a–b1)). The film, with a highly deformed margin, exhibited a very rough surface (Fig. 4(c)). The foam demonstrated more roughness and a damaged surface morphology compared to new foam granules. The newly foamed granules had micro-holes with a diameter of approximately 50 μm (Fig. 4(d)), and the ones extracted from the organism sample appeared to have larger micro-holes with a diameter of approximately 300 μm (Fig. 4(e1)). The edge of the foam was damaged to a higher degree (Fig. 4(e)), and the surface exhibited delamination at higher magnification (Fig. 4(e2)). In general, the microplastics in the biological samples showed heavy weathering features of a rough surface, ragged edges and obvious pores.
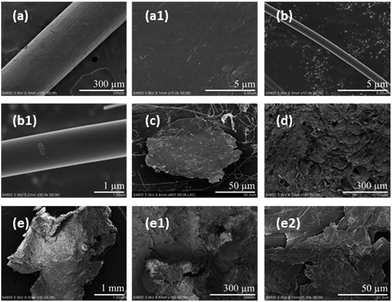 |
| Fig. 4 SEM images of the different microplastics shapes: (a–b1) fiber surface; (a1) and (b1) are the enlarged images of (a) and (b), respectively. (c) Film surface. (d) New foam granule surface. (e–e2) Foam granule surface; (e1) and (e2) are the enlarged images of (e). | |
The ability of microplastics to adsorb pollutants depends on the physicochemical properties, such as hydrophobicity and smoothness, of their surfaces.62 Rough surfaces increase the surface area of microplastics and can carry hydrophilic pollutants such as antibiotics and organophosphorus esters and phthalates.63,64 More environmentally weathered microplastics were significantly ingested by Mytilus galloprovincialis compared with virgin microplastics.65 So weathered microplastics and pollutants may have a compound toxicological effect on organisms. In addition to the variation of the surface morphology, the shape variation from fibers to irregular fragments to spheres might have potential adverse effects on organisms.66 However, only particles or spheres were used for most exposure studies, which might ignore the different shape-related toxicity of microplastics in the tested organisms. A previous study showed that beads could be egested more quickly than fibers in mussels, which might cause the long-term accumulation of fiber microplastics in the environment.6 To better understand the potential risks caused by differently shaped microplastics, a greater effort to study the impacts of multi-shape microplastics exposure on organisms is needed.
3.5 Analysis of the elemental composition signatures of the detected microplastics
SEM-EDS is most useful in providing high resolution imaging of the microplastics particle surface structures as well as elemental composition signatures. This information can be used to screen for likely microplastics and rule out non-plastics.31 Consistent with the presence of carbon and oxygen by EDS primarily, the fiber (Fig. 5(a1) and (a2)) was confirmed to be PET plastic by FT-IR analysis. Furthermore, high amounts of silicon suggested that the surface of PET fiber might have adhered silicon oxides. EDS spectra of foamed granules (Fig. 5(b1) and (b2)) displayed significant carbon peaks, which was consistent with the infrared identification of PS. SEM-EDS of the PTFE film exhibited unique characteristics of strong fluorine peaks in the EDS spectra. These characteristics were used to identify PTFE microplastics as indicated in Fig. 5(c2). A smooth, spherical morphology of a 5 μm particle was found under the SEM (Fig. 5(d1)), which was suspected to be microplastic under the stereo microscope since they have smooth surfaces. However, they can easily be ruled out by SEM-EDS, because the EDS spectrum revealed no significant carbon peak but a large peak for silicon (Fig. 5(d2)). Using SEM-EDS, as an auxiliary technology, can provide a way for data calibration between different techniques.
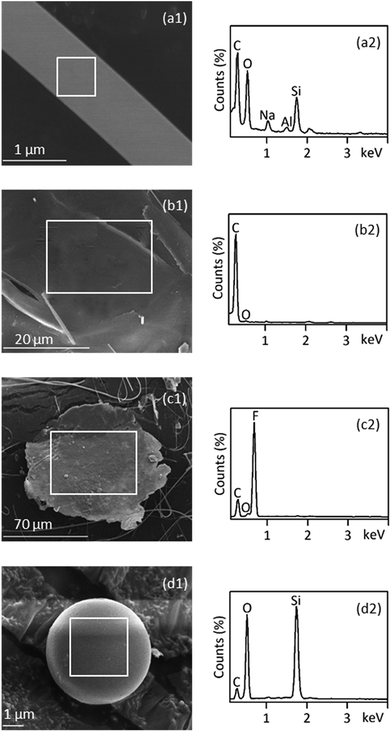 |
| Fig. 5 Identification of microplastics with SEM-EDS: (a1–d1) are SEM images, and (a2–d2) are the corresponding EDS spectra of the white boxed region. Some particles were identified as microplastics (a1–c1), and the others were identified as non-plastic (d1). | |
3.6 Assessment of multi-technology
In the present study, successive stereo microscopy and μ-ATR-FTIR are applied to all the microplastics samples. For a sample with a low database match score, preliminary visual sorting combined with SEM-EDS can more rapidly and accurately eliminate a large number of non-plastic particles and screen likely plastics given the complex matrices. SEM-EDS can compensate for the defects of an optical microscope in the magnification limit which may miss smaller microplastics and can strongly reveal certain types of fluoric microplastics such as PTFE. Using SEM-EDS prior to μ-ATR-FTIR would expedite the process of identifying microplastics, especially when the samples contain many confounding, non-plastic particles, such as silicon-containing compounds. However, it is not always convenient to conduct SEM-EDS before μ-ATR-FTIR. One can also use SEM-EDS after μ-ATR-FTIR depending on the availability of these instruments and the composition of the sample being analyzed. The multi-technique has two significant advantages: it is not necessary to repeatedly detect non-plastic particles; meanwhile, intercalibration between different methods is important to improve data accuracy especially when smaller microplastics are present in the samples. The limitation of this multi-technology is that the colors of smaller particles observed by SEM cannot be determined.
4. Conclusions
Multiple microscopic techniques and micro-analytical methods were implemented in this work to identify the abundance, polymer type, surface morphology, and surface element characteristics of microplastics in marine organisms. The abundance of microplastics in items per individual was significantly higher in the fish than that in bivalves at the same nutritional level. Four shapes of microplastics, including fibers, fragments, granules and films, were detected in the samples. Fibrous microplastics were the dominant ones in different organisms. Microplastics with sizes below 1 mm accounted for the largest portion, and the amount of microplastics decreased dramatically with the increasing particle size. Rayon and PET were commonly found with rayon being the most frequently detected polymer type in the organisms. Some spectral change of the detected microplastics may happen due to abiotic oxidation. The different shapes of microplastics exhibited different weathering surface morphologies. SEM-EDS technology was implemented to provide high resolution images of microplastics surface structures and to provide a way for data calibration in microplastics identification. The combination of multiple analytical techniques provides an accurate approach for microplastics identification and characterization.
Conflicts of interest
There are no conflicts of interests to declare.
Acknowledgements
This work was supported by the Basic Scientific Fund for National Public Research Institutes of China (No. 2016Q02/2017Y03), Qingdao National Laboratory for Marine Science and Technology (QNLM2016ORP0403), the Research Cooperation and Exchange of Marine Litter and Microplastics, China (No. QY0518011), the Second Sino-German Cooperation in Marine Sciences (No. QY0518016), and the Special Operational Funding Project of the State Oceanic Administration-Marine Environmental Monitoring/Assessment and Capacity Upgrading (No. BJ1318004).
Notes and references
- K. L. Law and R. C. Thompson, Science, 2014, 345, 144–145 CrossRef CAS PubMed.
- X. Sun, Q. Li, M. Zhu, J. Liang, S. Zheng and Y. Zhao, Mar. Pollut. Bull., 2017, 115, 217–224 CrossRef CAS PubMed.
- H. S. Auta, C. U. Emenike and S. H. Fauziah, Environ. Int., 2017, 102, 165–176 CrossRef CAS.
- D. K. A. Barnes, F. Galgani, R. C. Thompson and M. Barlaz, Philos. Trans. R. Soc., B, 2009, 364, 1985–1998 CrossRef CAS.
- A. Cozar, F. Echevarria, J. I. Gonzalez-Gordillo, X. Irigoien, B. Ubeda, S. Hernandez-Leon, A. T. Palma, S. Navarro, J. Garcia-de-Lomas, A. Ruiz, M. L. Fernandez-de-Puelles and C. M. Duarte, Proc. Natl. Acad. Sci. U. S. A., 2014, 111, 10239–10244 CrossRef CAS.
- X. Qu, L. Su, H. Li, M. Liang and H. Shi, Sci. Total Environ., 2018, 621, 679–686 CrossRef CAS.
- M. Cole, P. Lindeque, C. Halsband and T. S. Galloway, Mar. Pollut. Bull., 2011, 62, 2588–2597 CrossRef CAS.
- O. M. Lonnstedt and P. Eklov, Science, 2016, 352, 1213–1216 CrossRef.
- S. L. Wright and F. J. Kelly, Environ. Sci. Technol., 2017, 51, 6634–6647 CrossRef CAS.
- N. Digka, C. Tsangaris, M. Torre, A. Anastasopoulou and C. Zeri, Mar. Pollut. Bull., 2018, 135, 30–40 CrossRef CAS PubMed.
- A. Bakir, S. J. Rowland and R. C. Thompson, Environ. Pollut., 2014, 185, 16–23 CrossRef CAS.
- C. M. Rochman, E. Hoh, T. Kurobe and S. J. Teh, Sci. Rep., 2013, 3, 3263 CrossRef.
- P. Kolandhasamy, L. Su, J. Li, X. Qu, K. Jabeen and H. Shi, Sci. Total Environ., 2018, 610–611, 635–640 CrossRef CAS.
- L. Van Cauwenberghe and C. R. Janssen, Environ. Pollut., 2014, 193, 65–70 CrossRef CAS.
- H. Li, L. Ma, L. Lin, Z. Ni, X. Xu, H. Shi, Y. Yan, G. Zheng and D. Rittschof, Environ. Pollut., 2018, 236, 619–625 CrossRef CAS.
- M. Cole, H. Webb, P. K. Lindeque, E. S. Fileman, C. Halsband and T. S. Galloway, Sci. Rep., 2014, 4, 4528 CrossRef.
- J. P. G. L. Frias, V. Otero and P. Sobral, Mar. Environ. Res., 2014, 95, 89–95 CrossRef CAS.
- S. L. Wright, D. Rowe, R. C. Thompson and T. S. Galloway, Curr. Biol., 2013, 23, 1031–1033 CrossRef.
- J. Li, C. Green, A. Reynolds, H. Shi and J. M. Rotchell, Environ. Pollut., 2018, 241, 35–44 CrossRef CAS.
- J. Li, X. Qu, L. Su, W. Zhang, D. Yang, P. Kolandhasamy, D. Li and H. Shi, Environ. Pollut., 2016, 214, 177–184 CrossRef CAS.
- J. Li, D. Yang, L. Li, K. Jabeen and H. Shi, Environ. Pollut., 2015, 207, 190–195 CrossRef CAS.
- F. Bessa, P. Barria, J. M. Neto, J. Frias, V. Otero, P. Sobral and J. C. Marques, Mar. Pollut. Bull., 2018, 128, 575–584 CrossRef CAS.
- M. Compa, A. Ventero, M. Iglesias and S. Deudero, Mar. Pollut. Bull., 2018, 128, 89–96 CrossRef CAS.
- M. Codina-García, T. Militão, J. Moreno and J. González-Solís, Mar. Pollut. Bull., 2013, 77, 220–226 CrossRef.
- A. L. Lusher, G. Hernandez-Milian, J. O'Brien, S. Berrow, I. O'Connor and R. Officer, Environ. Pollut., 2015, 199, 185–191 CrossRef CAS.
- M. C. Fossi, L. Marsili, M. Baini, M. Giannetti, D. Coppola, C. Guerranti, I. Caliani, R. Minutoli, G. Lauriano, M. G. Finoia, F. Rubegni, S. Panigada, M. Bérubé, J. Urbán Ramírez and C. Panti, Environ. Pollut., 2016, 209, 68–78 CrossRef CAS PubMed.
- A. Mathalon and P. Hill, Mar. Pollut. Bull., 2014, 81, 69–79 CrossRef CAS.
- A. I. Catarino, V. Macchia, W. G. Sanderson, R. C. Thompson and T. B. Henry, Environ. Pollut., 2018, 237, 675 CrossRef CAS PubMed.
- E. Hermsen, R. Pompe, E. Besseling and A. A. Koelmans, Mar. Pollut. Bull., 2017, 122, 253–258 CrossRef CAS PubMed.
- T. M. Karlsson, A. D. Vethaak, B. C. Almroth, F. Ariese, M. van Velzen, M. Hassellöv and H. A. Leslie, Mar. Pollut. Bull., 2017, 122, 403–408 CrossRef CAS PubMed.
- Z. M. Wang, J. Wagner, S. Ghosal, G. Bedi and S. Wall, Sci. Total Environ., 2017, 603–604, 616–626 CrossRef CAS.
- A. Käppler, M. Fischer, B. M. Scholz-Böttcher, S. Oberbeckmann, M. Labrenz, D. Fischer, K. Eichhorn and B. Voit, Anal. Bioanal. Chem., 2018, 410, 5313–5327 CrossRef.
- W. J. Shim, S. H. Hong and S. E. Eo, Anal. Methods, 2017, 9, 1384–1391 RSC.
- Y. K. Song, S. H. Hong, M. Jang, G. M. Han, M. Rani, J. Lee and W. J. Shim, Mar. Pollut. Bull., 2015, 93, 202–209 CrossRef CAS.
- J. Ding, J. Li, C. Sun, C. He, F. Jiang, F. Gao and L. Zheng, Chin. J. Anal. Chem., 2018, 46, 690–697 CAS.
- S. E. Nelms, T. S. Galloway, B. J. Godley, D. S. Jarvis and P. K. Lindeque, Environ. Pollut., 2018, 238, 999–1007 CrossRef CAS.
- C. G. Avio, L. R. Cardelli, S. Gorbi, D. Pellegrini and F. Regoli, Environ. Pollut., 2017, 227, 207–214 CrossRef CAS.
- N. L. Hartline, N. J. Bruce, S. N. Karba, E. O. Ruff, S. U. Sonar and P. A. Holden, Environ. Sci. Technol., 2016, 50, 11532–11538 CrossRef CAS.
- M. A. Browne, P. Crump, S. J. Niven, E. Teuten, A. Tonkin, T. Galloway and R. Thompson, Environ. Sci. Technol., 2011, 45, 9175–9179 CrossRef CAS.
- A. L. Lusher, M. McHugh and R. C. Thompson, Mar. Pollut. Bull., 2013, 67, 94–99 CrossRef CAS.
- J. Bellas, J. Martínez-Armental, A. Martínez-Cámara, V. Besada and C. Martínez-Gómez, Mar. Pollut. Bull., 2016, 109, 55–60 CrossRef CAS.
- L. Su, H. Cai, P. Kolandhasamy, C. Wu, C. M. Rochman and H. Shi, Environ. Pollut., 2018, 234, 347–355 CrossRef CAS.
- E. Bergami, E. Bocci, M. L. Vannuccini, M. Monopoli, A. Salvati, K. A. Dawson and I. Corsi, Ecotoxicol. Environ. Saf., 2016, 123, 18–25 CrossRef CAS PubMed.
- E. Besseling, B. Wang, M. Lürling and A. A. Koelmans, Environ. Sci. Technol., 2014, 48, 12336–12343 CrossRef CAS PubMed.
- M. R. Jung, F. D. Horgen, S. V. Orski, C. V. Rodriguez, K. L. Beers, G. H. Balazs, T. T. Jones, T. M. Work, K. C. Brignac, S. Royer, K. D. Hyrenbach, B. A. Jensen and J. M. Lynch, Mar. Pollut. Bull., 2018, 127, 704–716 CrossRef CAS PubMed.
- M. Mecozzi, M. Pietroletti and Y. B. Monakhova, Mar. Pollut. Bull., 2016, 106, 155–161 CrossRef CAS PubMed.
- M. Gardette, A. Perthue, J. Gardette, T. Janecska, E. Földes, B. Pukánszky and S. Therias, Polym. Degrad. Stab., 2013, 98, 2383–2390 CrossRef CAS.
- B. Gewert, M. M. Plassmann and M. MacLeod, Environ. Sci.: Processes Impacts, 2015, 17, 1513–1521 RSC.
- T. D. S. E. Pegado, K. Schmid, K. O. Winemiller, D. Chelazzi, A. Cincinelli, L. Dei and T. Giarrizzo, Mar. Pollut. Bull., 2018, 133, 814–821 CrossRef CAS.
- S. Veerasingam, M. Saha, V. Suneel, P. Vethamony, A. C. Rodrigues, S. Bhattacharyya and B. G. Naik, Chemosphere, 2016, 159, 496–505 CrossRef CAS.
- P. L. Corcoran, M. C. Biesinger and M. Grifi, Mar. Pollut. Bull., 2009, 58, 80–84 CrossRef CAS.
- J. Brandon, M. Goldstein and M. D. Ohman, Mar. Pollut. Bull., 2016, 110, 299 CrossRef CAS.
- H. S. Auta, C. U. Emenike and S. H. Fauziah, Environ. Pollut., 2017, 231, 1552–1559 CrossRef CAS PubMed.
- I. R. Comnea-Stancu, K. Wieland, G. Ramer, A. Schwaighofer and B. Lendl, Appl. Spectrosc., 2017, 71, 939–950 CrossRef CAS PubMed.
- M. Steer, M. Cole, R. C. Thompson and P. K. Lindeque, Environ. Pollut., 2017, 226, 250–259 CrossRef CAS PubMed.
- L. Li, M. Li, H. Deng, L. Cai, H. Cai, B. Yan, J. Hu and H. Shi, Sci. Total Environ., 2018, 639, 367–373 CrossRef CAS.
- C. H. Park, Y. K. Kang and S. S. Im, J. Appl. Polym. Sci., 2010, 94, 248–253 CrossRef.
- D. Neves, P. Sobral, J. L. Ferreira and T. Pereira, Mar. Pollut. Bull., 2015, 101, 119–126 CrossRef CAS.
- M. Lehtiniemi, S. Hartikainen, P. Näkki, J. Engström-Öst, A. Koistinen and O. Setälä, Food Webs, 2018, 17, e97 CrossRef.
- J. Zhao, W. Ran, J. Teng, Y. Liu, H. Liu, X. Yin, R. Cao and Q. Wang, Sci. Total Environ., 2018, 640–641, 637–645 CrossRef CAS.
-
D. O. Hummel, Atlas of Plastics Additives: Analysis by Spectrometric Methods, Springer-Verlag, 2002, ISBN: 3-540-42414-8 Search PubMed.
- O. G. Apul and T. Karanfil, Water Res., 2015, 68, 34–55 CrossRef CAS PubMed.
- K. Ashton, L. Holmes and A. Turner, Mar. Pollut. Bull., 2010, 60, 2050–2055 CrossRef CAS PubMed.
- Q. Zhou, H. Zhang, C. Fu, Y. Zhou, Z. Dai, Y. Li, C. Tu and Y. Luo, Geoderma, 2018, 322, 201–208 CrossRef CAS.
- I. L. N. Bråte, M. Blázquez, S. J. Brooks and K. V. Thomas, Sci. Total Environ., 2018, 626, 1310–1318 CrossRef PubMed.
- S. L. Wright, R. C. Thompson and T. S. Galloway, Environ. Pollut., 2013, 178, 483–492 CrossRef CAS PubMed.
|
This journal is © The Royal Society of Chemistry 2019 |
Click here to see how this site uses Cookies. View our privacy policy here.