DOI:
10.1039/D2AY01977A
(Paper)
Anal. Methods, 2023,
15, 2417-2426
A sensitive enzyme-free electrochemical sensor based on a rod-shaped bimetallic MOF anchored on graphene oxide nanosheets for determination of glucose in huangshui†
Received
8th December 2022
, Accepted 22nd March 2023
First published on 15th May 2023
Abstract
In this work, we propose a bimetallic Ni–Co based MOF attached to graphene oxide (GO) by a one-step hydrothermal approach which may be employed as an electrochemical enzyme-free glucose sensor. Due to the obvious synergistic catalysis of Ni and Co, as well as the combination of NiCo-MOF and GO, NiCo-MOF/GO not only enhances energy transfer and electrocatalytic performance but also provides a larger surface area and more active sites. Electrochemical studies show that NiCo-MOF/GO exhibits outstanding electrochemical activity, with a sensitivity of 11
177 μA mM−1 cm−2 and 4492 μA mM−1 cm−2 in the linear ranges of 1–497 μM and 597–3997 μM, a detection limit of 0.23 μM, and a response time of 2 seconds. More importantly, the newly fabricated sensor is successfully applied for glucose determination in huangshui. This method provides a novel strategy for the controlled fermentation process and product quality of Chinese baijiu.
1. Introduction
Chinese baijiu has a long history and is one of the oldest alcoholic beverages.1 Chinese baijiu generates approximately 60 billion dollars in sales each year, with a consumption volume of over four million kiloliters, creating a huge revenue and causing it to take up a significant position in the national economy.2–4 Huangshui (HS) is the primary byproduct of solid-state fermentation of Chinese baijiu, and its quality assessment is critical for enhancing the fermentation technique and product control.5 In the process of alcohol fermentation, starch is converted into glucose by microbial metabolism and saccharification. Glucose flows out with the water produced by fermentation, and settles at the bottom of the pit to form HS.6,7 Glucose influences yeast development, and the amount of glucose dictates the ethanol concentration.8 It could be seen that the glucose content in HS played an important role in the fermentation state and product quality control. Real-time and rapid detection of glucose in HS is of great significance for liquor fermentation process monitoring and product quality control. Traditionally, glucose in HS is generally detected by wet chemical analysis. However, this method requires a long analysis time and is not safe.9
Electrochemical technology has the advantages of quick response, simple operation, low cost, long-term stability, and good selectivity.10–12 Because of its excellent performance, electrochemical technology has been used successfully to detect glucose in sweat,13 tears,14 saliva,15 urine,16 human serum,17 and beverages.18 At present, there is no report on electrochemical technology being used to detect glucose in HS. There are two types of electrochemical sensors for detecting glucose: enzymatic and nonenzymatic. Enzymatic sensors offer the benefits of sensitivity and selectivity, but the enzymes are expensive, have unstable activity, and need to undergo a difficult immobilization process, which prevents their use.19,20 Due to their convenient preparation, low price, and high stability non-enzymatic sensors are suitable for electrochemical sensing of glucose.21 Although precious metals and their alloys have excellent electrochemical properties, their high prices are prohibitive.22 In order to develop non-enzymatic electrochemical sensors at low cost and with high performance, non-precious metals have become the first choice. Many non-precious metals like metals or their alloys,23,24 metal oxides,25 metal phosphides,26 and metal hydroxides27 have attracted wide attention. However, their self-aggregation seriously limits their electrocatalytic activity and stability.28
In recent years, a lot of researchers have been interested in metal organic frameworks (MOF), a novel kind of porous material built from metal ions and organic ligands.29 Due to their large specific area and malleable structure and porosity, MOF have found widespread use in the fields of biosensing.30,31 Unfortunately, poor electronic conductivity leads to many active sites not participating in the reaction, which limits their catalytic performance and hinders the practical application of MOF as an electrochemical material.32 Generally, combining different metal centers in the same frame can produce new defects in the structure of MOF, and the excellent synergistic effect between different metals makes the catalytic activity of bimetallic MOF higher than that of single metal MOF.33 Among the metal sites of MOF, Ni has a wide range of sources and high electrocatalytic performance, and Co can provide more active reaction sites. Therefore, bimetallic NiCo-MOF has outstanding catalytic performance compared with single-metal Ni-based MOF and Co-based MOF.34 By introducing new metal nodes into the organic framework, the catalytic performance of MOF can be adjusted and enhanced. In addition, a typical method to increase MOF materials' conductivity is to link MOF with conductive materials.35 Examples include solvothermally synthesized Fe-MOF/reduced graphene oxide (RGO) composites, and in situ hydrothermally synthesized Co-MOF/Ti3C2Tx composites grown on Ni foam, both of which show improved electrochemical performance.36,37 Nevertheless, these materials are inconvenient to prepare, not environmentally friendly and have poor dispersibility and stability in aqueous solution. At the same time, we noticed that GO showed excellent conductivity, which could improve the electrochemical performance of GO-based hybrids.38 And GO has strong hydrophilicity and water solubility due to the existence of oxygen-containing groups.39 Therefore, due to the synergistic effect between MOF and GO, anchoring bimetallic MOF on GO may bring about more excellent and stable electrochemical performance for the manufactured biosensor.
Inspired by all of this, in this work, we have prepared a NiCo-MOF/GO hybrid composite by a one-step hydrothermal approach without any surfactant. It has excellent electrochemical performance, dispersibility, and stability. Scheme 1 depicts the synthesis of NiCo-MOF/GO nanomaterials. CV and amperometric analysis indicated the NiCo-MOF/GO/GCE showed high selectivity and sensitivity, and good long-term stability. When compared to other MOF-based non-enzymatic glucose sensors, it excels in terms of sensitivity, detection limit, and linear detection range. The most important thing is that the prepared NiCo-MOF/GO has been successfully applied to glucose detection in HS. In addition, glucose in human serum was detected. This shows that the prepared sensor has good biocompatibility and great potential for practical application.
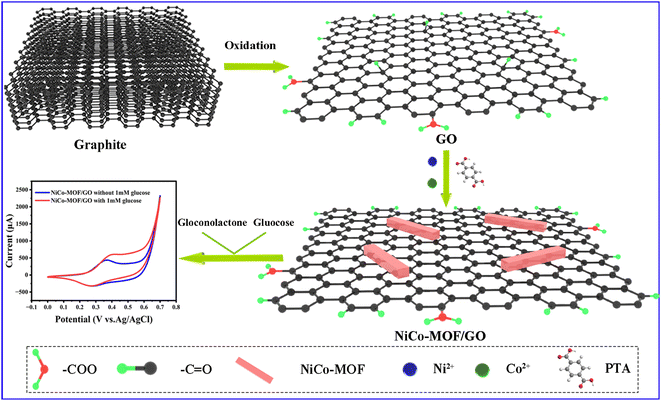 |
| Scheme 1 Illustration of the fabrication of NiCo-MOF/GO and its application in glucose determination. | |
2. Experimental section
2.1 Reagents and materials
Information on reagents and materials is presented in the ESI.†
2.2 Synthesis of NiCo-MOF
Using a simplified solvothermal route, we were able to produce NiCo-MOF nanomaterials following the established protocol with a few tweaks.40 In brief, 249.2 mg p-phthalic acid (PTA), 218.3 mg Co(NO3)2·6H2O, and 178.3 mg NiCl2·6H2O were added to a mixed solution containing N,N-dimethylformamide(DMF), ethanol and H2O (16
:
1
:
1), then ultrasonic treatment was performed to mix them evenly, and finally, they were reacted in a stainless steel autoclave lined with Teflon at 140 °C for 48 hours. After the reaction had completed and the sample had cooled, it was washed several times with DMF and ethanol. The centrifuged sample was collected and dried in a vacuum at 60 °C for 12 hours.
2.3 Synthesis of graphene oxide (GO)
A modified version of Hummers' process was used to manufacture GO.41 Briefly, potassium permanganate (KMnO4) and sulfuric acid (H2SO4) were used to oxidize graphite, and hydrogen peroxide (H2O2) and hydrochloric acid (HCl) were used to remove residual substances. And finally centrifugal drying was carried out to obtain GO powder.
2.4 Synthesis of NiCo-MOF/GO
For the production of NiCo-MOF/GO, firstly, 0.2 g GO was dissolved in a 16
:
1
:
1 solution of DMF, ethanol, and water; then, 249.2 mg of p-phthalic acid, 218.3 mg of Co(NO3)2·6H2O and 218.3 mg of NiCl2·6H2O were added and the mixture was agitated for an hour. When this step is complete, the manufacturing process is the same as in Section 2.2.
2.5 Modification of the glassy carbon electrode
Firstly, the bare GCE was polished using an aluminum oxide slurry of (0.3 μM) and (0.05 μM) on chamois leather. After that, it was cleaned using an ultrasonic washer with and without water before being air dried. The NiCo-MOF/GO composite was dissolved in DI water at a concentration of 2 mg mL−1 by using a sonicator for 2 h. Subsequently, a 5 μL dispersion of NiCo-MOF/GO was drop-cast onto the GCE's mirror-polished surface, allowed to dry at room temperature, and then put to use in electrochemical tests. In order to conduct comparative investigations, the same method was used to modify additional electrodes, including NiCo-MOF/GCE and GO/GCE.
2.6 Preparation of real samples
After preparing an electrode, HS was used to test how well it would work for detecting glucose. So, we recorded the response of current densities after dropping 10 μL of HS samples into 10 mL of 0.1 M NaOH solution and stirring the mixture continuously. After centrifuging for 10 minutes (10
000 rpm) to eliminate any leftover impurities, HS was directly diluted with 100 times 0.1 M NaOH solution for the electrochemical signal recording.
3. Results and discussion
3.1 Morphology and structural characterization of materials
Morphological studies of the synthesized samples were thoroughly investigated by field emission scanning electron microscopy (FESEM) and transmission electron microscopy (TEM). As observed in Fig. 1A and B NiCo-MOF exhibits a well-defined rod morphology, as evidenced by the smooth surface, and the rods are randomly arranged. By measurement, the average particle size of NiCo-MOF is 3.11 μm (Fig. 1B). At the same time, we can see that the rod-shaped MOF was connected. Its slight accumulation, however, prevented the exposure of more active sites. The FESEM image in Fig. 1C shows wrinkle-like graphene oxide sheets. The graphene oxide layers were slightly agglomerated. The FESEM images of the NiCo-MOF/GO composite (Fig. 1D) show that the NiCo-MOF rods were grown randomly on the surface of GO sheets and rod-shaped NiCo-MOF was unevenly formed. As for the Ni-MOF/GO composite, some rod-shaped NiCo-MOF were arranged on the surface of GO, while others were embedded in GO. The well-defined rod-shaped NiCo-MOF and its combination with GO could be validated using the TEM image of the NiCo-MOF/GO composite (Fig. S1A†). By spreading out the NiCo-MOF, additional active sites could be made available to boost glucose oxidation. And at the same time, NiCo-MOF also prevented the aggregation of GO, which was beneficial to electron transfer in the redox process. To a large extent, we believe that the better electrochemical property is a result of the interaction between NiCo-MOF and GO. Fig. 1E–H show the energy dispersive spectrometer (EDS) elemental mapping images of the NiCo-MOF/GO composite, which verified that the provided components, including C, O, Ni, and Co, were distributed uniformly over the area of interest (Fig. 1D), indicating the successful assembly of NiCo-MOF/GO.
 |
| Fig. 1 (A and B) FESEM images of NiCo-MOF. (C) FESEM image of GO. (D) FESEM image of NiCo-MOF/GO. (E–H) EDS elemental mapping of NiCo-MOF/GO. | |
Besides, to learn more about the structure of NiCo-MOF/GO, XRD testing was performed. Fig. 2A shows the XRD pattern of GO, NiCo-MOF, and NiCo-MOF/GO. In the XRD pattern of GO, the characteristic peak at around 10.8° corresponded to the typical (001) crystal plane.42 According to the XRD pattern of NiCo-MOF, the obtained rod-shaped NiCo-MOF is isostructural with the previously reported Ni-based MOF(CCDC No. 985792).43 In this structure, PTA molecules separate two-dimensional bimetal layers formed by Co and Ni atoms arranged in octahedral coordination and connected along the [010]/[001] direction in the (200) crystallographic plane.40 Due to the fact that the NiCo-MOF/GO's diffraction pattern resembled that of NiCo-MOF, it can be concluded that the incorporation of GO does not inhibit the formation of the connection between Ni and Co ions, and organic bridges. Meanwhile, the Raman spectrum of NiCo-MOF/GO is shown in Fig. S1B.† The two peaks at 1345 and 1588 cm−1 are assigned to D and G peaks, indicating the degree of graphitization.44 Furthermore, it was confirmed that the synthesized nanomaterial was NiCo-MOF/GO.
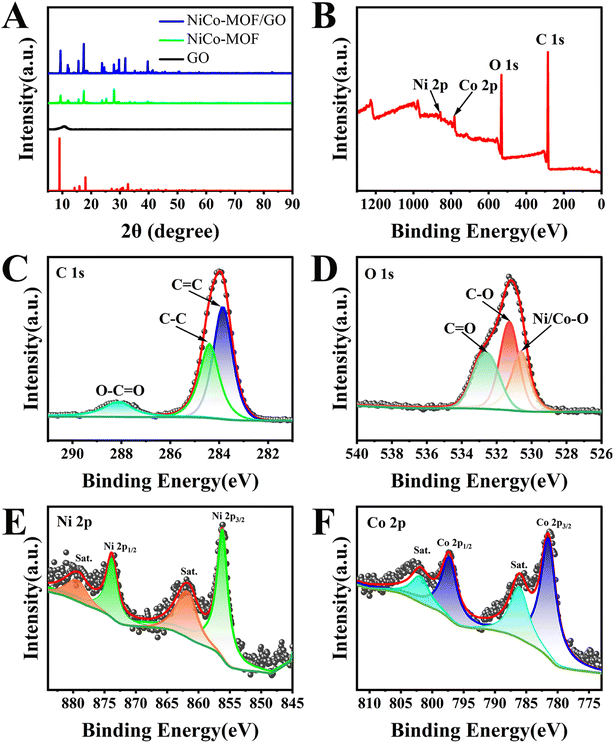 |
| Fig. 2 (A) XRD patterns of GO, NiCo-MOF/GO and NiCo-MOF/GO. XPS spectra of NiCo-MOF/GO: (B) survey spectrum; (C) high resolution C 1s spectrum; (D) high resolution O 1s spectrum; (E) high resolution Ni 2p spectrum and (F) high resolution Co 2p spectrum. | |
XPS analysis was used to identify the chemical make-up and valence state of NiCo-MOF/GO. As shown in Fig. 2B, in the survey scanning spectrum, the peaks of elements including C, O, Ni, and Co stand out clearly. It shows the results consistent with EDS. This provides additional evidence for the successful synthesis of the composite. In the C 1s spectrum (Fig. 2C), there were three kinds of C species, which were C
C located at 283.9 eV, C–C located at 284.4 eV, and O–C
O situated at 288.2 eV.45 The high-resolution O 1s spectrum of NiCo-MOF/GO is shown in Fig. 2D. The peaks at 530.6, 530.6, 531.3, and 532.6 eV are attributed to metal oxides, C–O, and C
O.46,47 The high definition of Ni 2p is depicted in Fig. 2E. The existence of Ni2+ is indicated by the binding energies of Ni 2p3/2 and Ni 2p1/2, which peak at 856.3 and 873.8 eV, respectively, and are accompanied by two vibration satellite peaks at 861.8 and 879.4 eV.48 The two primary peaks at 781.4 eV and 797.3 eV could have been attributed to Co 2p3/2 and Co 2p1/2, respectively, and the two satellite peaks at 786.2 eV and 806.1 eV suggest the presence of Co2+ in the NiCo-MOF/GO (see Fig. 2E).49,50 According to the XPS results (Fig. 2B), the chemical makeup of the NiCo-MOF/GO material includes carbon (76.43 at%), oxygen (22.11 at%), nickel (0.63 at%), and cobalt (0.82 at%). Overall, the FESEM, TEM, EDS, XRD, Raman spectrum, and XPS results indicate that the product synthesized is NiCo-MOF/GO nanomaterials.
The Brunauer–Emmett–Teller (BET) analysis is used to evaluate the porosity and surface area of the material, and the related result is shown in Fig. S1C and D.† The NiCo-MOF/GO composite material exhibits type IV nitrogen adsorption/desorption isotherms, indicating it is mesoporous. The specific surface area of NiCo-MOF/GO is 51.0192 m2 g−1, and the pore volume is 0.1619 cm3 g−1. The mean pore diameter was determined using the pore size distribution curve to be 12.6911 nm (Fig. S1D†). The high surface area and abundant mesoporous properties of the composites provide higher electrocatalytic activity.51
3.2 Electrochemical characterization
To characterize the electrochemical performance of NiCo-MOF/GO, the electrochemical behavior of NiCo-MOF/GO/GCE in NaOH (0.1 M) with and without glucose is studied using cyclic voltammetry (CV). In the absence of glucose, two distinct redox peaks were observed for NiCo-MOF/GCE and NiCo-MOF/GO/GCE (Fig. 3A, S2†). However, a faster electron transfer process was seen in the NiCo-MOF/GO/GCE, as evidenced by a considerably larger peak current compared to that of the NiCo-MOF/GCE. This improved electrochemical performance of NiCo-MOF/GO/GCE may be due to the incorporation of NiCo-MOF into GO. As shown in Fig. 3B, the increased Ipa observed for the NiCo-MOF/GO modified electrode over the bare, GO, and NiCO-MOF electrodes can be attributed to the improved oxidation of glucose brought about by the GO layers' greater electrical conductivity and surface area. Besides, with the support of GO sheets, NiCo-MOF nanorods were widely diffused in the NiCo/MOF/GO composite, and they also served to prevent the graphene layers from restacking. Thus, NiCo-MOF/GO has more catalytic active sites than both pure NiCo-MOF and GO. Moreover, the synergistic actions of NiCo-MOF and GO led to an increase in catalytic activity and superior conductivity in NiCo-MOF/GO.
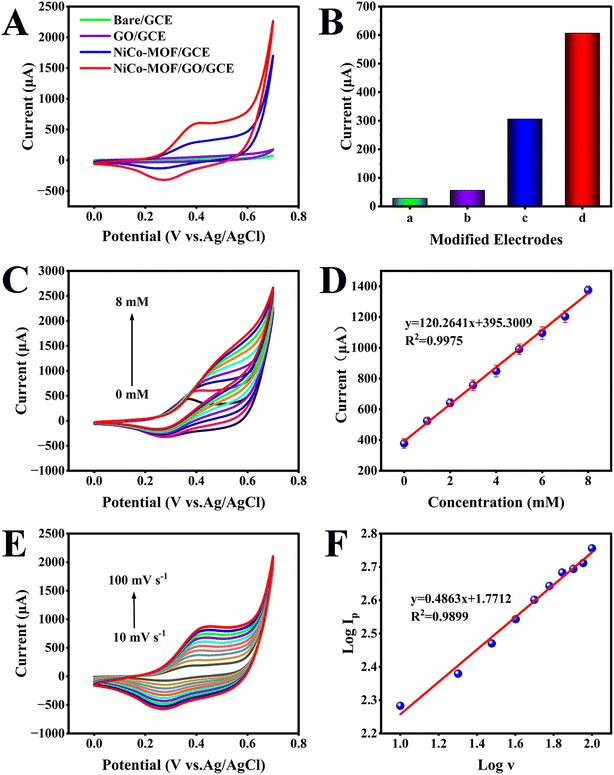 |
| Fig. 3 (A) CV curves of the bare GCE, GO/GCE, NiCo-MOF/GCE, and NiCo-MOF/GO/GCE in the presence of 1 mM glucose (solution: 0.1 M NaOH, scan rate: 50 mV s−1). (B) Bar diagram of the oxidation peak current response for glucose over different modified GCEs ((a) bare, (b) GO, (c) NiCo-MOF, (d) NiCo-MOF/GO). (C) CV curves of NiCo-MOF/GO/GCE in 0.1 M NaOH solutions containing different concentrations of glucose (0–8 mM) at a scan rate of 50 mV s−1. (D) Corresponding fitting curves of peak current vs. concentration of glucose. (E) CV curves at various scan rates of the NiCo-MOF/GO/GCE electrode. (F) Plots illustrating the anodic peak current logarithm vs. the scan rate logarithm. | |
Fig. 3C displays the CV curves of NiCo-MOF/GO/GCE in 0.1 M Na(OH) at a scan rate of 50 mV s−1 for a range of glucose concentrations (from 0 to 8 mM). As the glucose concentration rose from 0 to 8 mM, so did the magnitude of the current response. In Fig. 3D, we see that the relationship between glucose concentration and Ipa is very linear, with a coefficient of R2 = 0.9975 and a linear regression equation of Ipa(A) = 120.2641[Glu](mM) + 395.3009. It demonstrates that a broad concentration range of glucose can be oxidized on the surface of NiCo-MOF/GO. At the same time, with the anodic current to glucose increasing, the peak potential shifted slightly. According to previous reports, the reaction between NiCo-MOF/GO and glucose can be expressed by the following equations (eqn (1)–(5)):52–54
| Ni(II)/Co(II) + OH− → Ni(III)/Co(III) + e− | (1) |
| Co(III) + OH− → Co(IV) + e− | (2) |
| Ni(III) + glucose → Ni(II) + gluconolactone | (3) |
| Co(III) + glucose → Co(II) + gluconolactone | (4) |
| Co(IV) + glucose → Co(III) + gluconolactone | (5) |
As shown in eqn (1) and (2), firstly, Ni and Co are oxidized in an alkaline solution to form Ni and Co with high valence. It can be seen from eqn (3)–(5) that glucose is oxidized to gluconolactone by Ni and Co with high valence on the electrode surface. Glucose oxidation, in brief, involves the reduction of nickel(III) to nickel(II) and cobalt(IV) to cobalt(II) in a catalytic reaction. In addition, NiCo-MOF/GO/GCE CV curves were also acquired at varying scan rates (10 mV s−1–100 mV s−1) to learn more about the reaction kinetics. NiCo-MOF/GO/GCE CV curves were acquired in a 0.1 M NaOH solution (with 1.0 mM glucose) at various scan rates, as shown in Fig. 3E. Anodic peaks are found to shift to a more positive value, whereas cathodic peaks are found to shift to a more negative value, as scan rates increase, as indicated. The logarithmic value of the anode peak current is linear with the logarithmic value of the scanning rate, as illustrated in Fig. 3F. The equation of linear relationship could be expressed as log
Ip (μA) = 0.4863 log
v + 1.7712 (R2 = 0.9899). The slope is 0.4863, which is close to 0.5 in theory. It suggests that the electrocatalytic oxidation of glucose at the NiCo-MOF/GO electrode followed the classical model of a diffusion-controlled electrochemical reaction.51,55
3.3 Electrochemical determination of glucose
Amperometry has good sensitivity and a low detection limit. Excellent stability makes it particularly suitable for long-term monitoring.56 Amperometry is chosen over other electrochemical methods such as square wave voltammetry and differential pulse voltammetry for evaluating the sensitivity and quantitative range of the manufactured sensor.57 The loading mass of the electrode material and the applied potential were related to excellent sensing performance. Therefore, before conducting the electroanalytical determination, the loading mass of NiCo-MOF/GO and applied potential was optimized. As shown in Fig. S3A,† the current response increases as the amount of NiCo-MOF/GO increases from 3 to 5 μL and decreases above 6 μL. NiCo-MOF/GO can promote electron transfer. However, when the modified material exceeds a certain value, the current response will decrease. The increase and thickness of the materials that hinder electron transfer formed on the electrodes may be the possible reasons. Fig. S3B† demonstrates that as the working voltage is increased from 0.4 V to 0.7 V, the current response of NiCo-MOF/GO to glucose rises progressively from the starting value. There is a maximum value for the electrode response current at an operating voltage of 0.6 V, and the current response signal fades between 0.60 V and 0.7 V. It is not only stable but also smooth compared with others at different potentials. Therefore, we choose the loading mass of NiCo-MOF/GO of 5 μL and +0.6 V at working potential for further studies. Fig. 4A displays the programming of the NiCo-MOF/GO/GCE under optimal conditions following several injections of glucose at concentrations ranging from 1 μM to 3.997 mM. As shown in Fig. 4C, the steady-state value of the corresponding current owing to glucose electro-oxidation is reached within 2 seconds after the injection of the glucose. One possible explanation for the lightning-fast response is that glucose molecules on the NiCo-MOF/GO/GCE surface undergo a rapid oxidation process upon contact. Furthermore, evidence that the NiCo-MOF/GO/GCE has high catalytic activity toward glucose electro-oxidation is provided by an increase in the electrocatalytic currents caused by the addition of glucose at concentrations as low as 1 μM (Fig. 4B). Fig. 4D depicts the calibration curve derived from amperometric analyses; this curve shows two linear ranges for the variation of the oxidative currents with the glucose concentration, one between 1 and 497 μM and another between 597 and 3997 μM, with the following linear regression equations describing these ranges: Ip(μA) = 790.0881 [Glu](mM) + 1.0472(R2 = 0.9945); Ip(μA) = 317.5774 [Glu](mM) + 120.2287(R2 = 0.9970). Two linear range sensitivities of 11
177 and 4492 μA mM−1 cm−2 for the modified electrode were determined. The decreased sensitivity of the sensor at higher glucose concentrations may be related to the agglomeration of gluconolactone on the electrode surface, which hinders the entry of glucose molecules and hydroxyl ions into the electrode reaction sites.58 In addition, when the concentration exceeded 3.997 mM, the calibration curve gradually deviated from the linearity, which could be attributed to the lack of enough electrocatalytic active sites on the electrode surface to react with glucose.59 According to the equation: LOD = 3σ/S (where S is the slope of the linear regression and σ is the standard deviation calculated from 10 values of the blank sample), the LOD of the NiCo-MOF/GO/GCE is calculated to be 0.23 μM (S/N = 3). As shown in Table 1, we evaluate our built sensor against the performance of previously published electrochemical glucose sensors. With a lower detection limit, higher sensitivity, and a wider linear range, our results show that our analytical parameters for NiCo-MOF/GO/GCE are superior to those of previous sensors. More electroactive sites and greater surface area of NiCo-MOF/GO nanocomposites may account for their remarkable electrocatalytic activity in the produced NiCo-MOF/GO/GCE. Consequently, this seems promising for the future of NiCo-MOF/GO nanocomposites in the development of electrochemical glucose sensors that don't rely on enzymes.
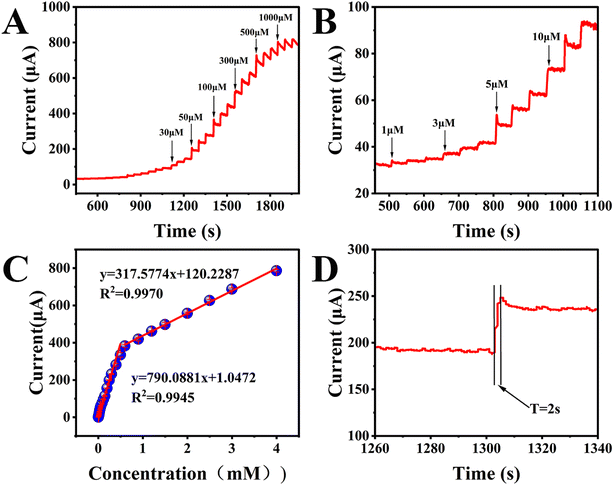 |
| Fig. 4 (A) Amperometric responses of the NiCo-MOF/GO/GCE to the continual addition of glucose at 0.6 V. (B) Magnification of the program of low glucose concentrations. (C) Corresponding linear calibration curve of A. (D) Response time of the modified GCE. | |
Table 1 Detection of glucose in HS samples using the Ni/Co-MOF/GCE
Samples |
Added (μM) |
Found (μM) |
Mean recovery (%) |
RSD (%, n = 3) |
HS |
0 |
1.46 |
0 |
0 |
100 |
104.68 |
104.68 |
1.18 |
500 |
482.10 |
96.42 |
4.89 |
1000 |
981.22 |
98.12 |
2.58 |
3.4 Selectivity, reproducibility, and stability
For the further practical application of glucose sensors, selectivity, reproducibility, and stability are very important. In order to further explore the performance of our sensor, the selectivity, repeatability, and stability were evaluated. NiCo-MOF/GO/GCE selectivity was measured using an amperometric technique, and the species potassium chloride (KCl), sodium chloride (NaCl), ascorbic acid (AA), uric acid (UA), ethanol, sucrose (Suc), fructose (Fru), lactose (Lac) were used as biological interferences. As shown in Fig. 5A, we can see that the addition of glucose resulted in pronounced current responses while the interfering species elicited barely perceptible changes. As can be seen in the bar chart of Fig. 5B, the response current of interfering compounds does not surpass 7.58 percent of the glucose response current, thus illustrating a good selectivity of NiCo-MOF/GO/FCE for the glucose detection. The current response of five individually adjusted NiCo-MOF/GO electrodes to 500 μM was measured to examine the electrode's repeatability; the results are depicted in Fig. 5C. The result of one-way ANOVA shows that the overall mean is not significantly different at the level of 0.05 and a computed relative standard deviation (RSD) of 1.6% demonstrates the NiCo-MOF/GO electrode's high degree of repeatability. In addition, a single suggested modified electrode was used to test the NiCo-MOF/GO electrode's long-term stability in response to glucose (500 μM) over 28 days (Fig. 5D). The outstanding stability of our NiCo-MOF/GO sensor was demonstrated by the fact that the NiCo-MOF/GO electrode maintained 91.74% of the original current value.
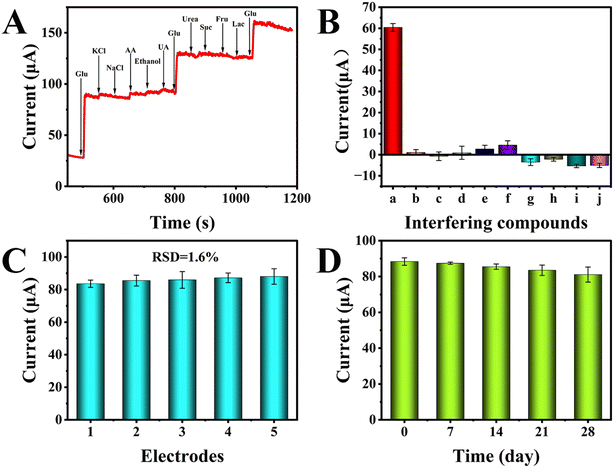 |
| Fig. 5 (A) Current response of NiCo-MOF/GO/GCE in 0.1 M NaOH with addition of 500 μM glucose, 50 μM KCl, 50 μM NaCl, 50 μM AA, 150 μM ethanol, 50 μM UA, 50 μM urea, 50 μM Suc, 50 μM Fru, and 50 μM Lac. (B) The corresponding bar chart of current response versus interfering molecules ((a) glucose, (b) KCl, (c) NaCl, (d) AA, (e) ethanol, (f) UA, (g) urea, (h) Suc, (i) Fru, (j) Lac). (C) Current response to 500 μM glucose for NiCo-MOF/GO/GCE GCE versus different electrodes. (D) Variation of the current response to 500 μM glucose for NiCo-MOF/GO/GCE GCE versus storage time. | |
3.5 Real sample detection
The described sensor was utilized to detect the concentration of glucose in HS samples in order to evaluate its applicability as a reliable sensor for the measurement of the glucose level in genuine samples. In this respect, the method of standard addition was used to measure the glucose level in these samples. In order to measure the effects of different concentrations of glucose on HS, standard solutions of 0, 100, 500, and 1000 μM were added to an HS alkaline solution (10 μL samples in 10 mL 0.1 M NaOH) and the resulting amperometric responses were recorded. The recoveries of the spiked samples in this investigation ranged from 96.42 to 104.68 percent, and the RSD was smaller than 4.89 percent, as shown in Table 1. These results validated the NiCo-MOF/GO/GCE as an enzyme-free sensor for reliable and precise glucose detection in actual samples.
4. Conclusions
In conclusion, the conductive NiCo-MOF/GO has been successfully compounded via a simple one-step hydrothermal method free of polymer binders, and it has also been shown to be an effective sensor for glucose electrocatalytic oxidation. With the synergistic effect of NiCo-MOF and GO, the sensor has excellent catalytic performance, conductivity, and water solubility. With a low LOD of 0.23 μM (S/N = 3), a rapid reaction time of 2 s and high sensitivity (11
177 and 4492 μA mM−1 cm−2), the NiCo-MOF/GO/GCE displays outstanding catalytic activity toward glucose. Additionally, recoveries for glucose sensing in HS from the prepared NiCo-MOF/GO/GCE range from 96.42 percent to 104.68 percent (RSD 4.89%). The results contribute to NiCo-MOF/GO/GCE as a practical sensing platform that may be used to determine glucose in HS. At the same time, this study provides a promising strategy for preparing other metal–organic framework-based composite materials.
Conflicts of interest
There are no conflicts to declare.
Acknowledgements
This work was supported by the National Natural Science Foundation of China (No. 31171684) and Wuliangye Group research project (CXY2020ZR004).
References
- W. Tu, X. Cao, J. Cheng, L. Li, T. Zhang, Q. Wu, P. Xiang, C. Shen and Q. Li, Front.Microbiol., 2022, 13, 919044 CrossRef PubMed
.
- M. Xu, Y. Yu, H. Ramaswamy and S. Zhu, Sci. Rep., 2017, 7, 1–9 CrossRef PubMed
.
- F. Yao, B. Yi, C. Shen, F. Tao, Y. Liu, Z. Lin and P. Xu, Sci. Rep., 2015, 5, 9553 CrossRef CAS PubMed
.
- J. Hong, D. Zhao and B. Sun, Food Rev. Int., 2021, 1–27 CrossRef
.
- J. Huo, J. Wu, B. Sun, M. Zhao, W. Sun, J. Sun and M. Huang, Int. J. Biol. Macromol., 2020, 161, 406–416 CrossRef CAS PubMed
.
- Z. Gao, Z. Wu and W. Zhang, Foods, 2020, 9, 372 CrossRef CAS PubMed
.
- H. Liu and B. Sun, J. Agric. Food Chem., 2018, 66, 5425–5432 CrossRef CAS PubMed
.
- A. Samphao, P. Butmee, P. Saejueng, C. Pukahuta, Ľ. Švorc and K. Kalcher, J. Electroanal. Chem., 2018, 816, 179–188 CrossRef CAS
.
- L. Qi, T. Xianguo, Z. Guiyu, L. Lin, Z. Xuemei and L. Wenbin, J. Food Saf. Qual., 2022, 13, 6026–6031 Search PubMed
.
- X. Chen, D. Liu, G. Cao, Y. Tang and C. Wu, ACS Appl. Mater. Interfaces, 2019, 11, 9374–9384 CrossRef CAS PubMed
.
- Y. Zhao, X. Zheng, Q. Wang, T. Zhe, Y. Bai, T. Bu, M. Zhang and L. Wang, Food Chem., 2020, 333, 127495 CrossRef CAS PubMed
.
- F. Sun, X. Wang, Z. You, H. Xia, S. Wang, C. Jia, Y. Zhou and J. Zhang, J. Mater. Sci. Technol., 2022, 123, 113–122 CrossRef
.
- P.-H. Lin, S.-C. Sheu, C.-W. Chen, S.-C. Huang and B.-R. Li, Talanta, 2022, 241, 123187 CrossRef CAS PubMed
.
- H. Lee, Y. J. Hong, S. Baik, T. Hyeon and D. H. Kim, Adv. Healthcare Mater., 2018, 7, 1701150 CrossRef PubMed
.
- P.-H. Lin, W.-L. Chang, S.-C. Sheu and B.-R. Li, Iscience, 2020, 23, 101658 CrossRef PubMed
.
- V. E. Coyle, A. E. Kandjani, M. R. Field, P. Hartley, M. Chen, Y. M. Sabri and S. K. Bhargava, Biosens. Bioelectron., 2019, 141, 111479 CrossRef CAS PubMed
.
- Y. Shu, Y. Yan, J. Chen, Q. Xu, H. Pang and X. Hu, ACS Appl. Mater. Interfaces, 2017, 9, 22342–22349 CrossRef CAS PubMed
.
- Z. Xu, Q. Wang, H. Zhangsun, S. Zhao, Y. Zhao and L. Wang, Food Chem., 2021, 349, 129202 CrossRef CAS PubMed
.
- H. Zhu, L. Li, W. Zhou, Z. Shao and X. Chen, J. Mater. Chem. B, 2016, 4, 7333–7349 RSC
.
- T. Zhu, Y. Zhang, L. Luo and X. Zhao, ACS Appl. Mater. Interfaces, 2019, 11, 10856–10861 CrossRef CAS PubMed
.
- A. Muthumariappan, K. Sakthivel, S.-M. Chen, T.-W. Chen, G. Mani and B.-S. Lou, Sens. Actuators, B, 2018, 266, 655–663 CrossRef CAS
.
- D.-W. Hwang, S. Lee, M. Seo and T. D. Chung, Anal. Chim. Acta, 2018, 1033, 1–34 CrossRef CAS PubMed
.
- S. Nantaphol, T. Watanabe, N. Nomura, W. Siangproh, O. Chailapakul and Y. Einaga, Biosens. Bioelectron., 2017, 98, 76–82 CrossRef CAS PubMed
.
- M. Zhang, Y. Liu, J. Wang and J. Tang, Microchim. Acta, 2019, 186, 1–8 CrossRef PubMed
.
- S. Yang, G. Li, D. Wang, Z. Qiao and L. Qu, Sens. Actuators, B, 2017, 238, 588–595 CrossRef CAS
.
- L. Xie, A. M. Asiri and X. Sun, Sens. Actuators, B, 2017, 244, 11–16 CrossRef CAS
.
- X. Wu, F. Li, C. Zhao and X. Qian, Sens. Actuators, B, 2018, 274, 163–171 CrossRef CAS
.
- Y. Fu, H. Y. Yu, C. Jiang, T. H. Zhang, R. Zhan, X. Li, J. F. Li, J. H. Tian and R. Yang, Adv. Funct. Mater., 2018, 28, 1705094 CrossRef
.
- H.-S. Wang, Coord. Chem. Rev., 2017, 349, 139–155 CrossRef CAS
.
- S. Shahrokhian and S. Ranjbar, Analyst, 2018, 143, 3191–3201 RSC
.
- J. Calbo, M. J. Golomb and A. Walsh, J. Mater. Chem. A, 2019, 7, 16571–16597 RSC
.
- Z. Wei, W. Zhu, Y. Li, Y. Ma, J. Wang, N. Hu, Y. Suo and J. Wang, Inorg. Chem., 2018, 57, 8422–8428 CrossRef CAS PubMed
.
- M. Ezzati, S. Shahrokhian and H. Hosseini, ACS Sustainable Chem. Eng., 2020, 8, 14340–14352 CrossRef CAS
.
- X. Zha, W. Yang, L. Shi, Y. Li, Q. Zeng, J. Xu and Y. Yang, ACS Appl. Mater. Interfaces, 2022, 14, 37843–37852 CrossRef CAS PubMed
.
- M. Ko, L. Mendecki and K. A. Mirica, Chem. Commun., 2018, 54, 7873–7891 RSC
.
- Y. Jin, C. Zhao, Z. Sun, Y. Lin, L. Chen, D. Wang and C. Shen, RSC Adv., 2016, 6, 30763–30768 RSC
.
- R. Ramachandran, K. Rajavel, W. Xuan, D. Lin and F. Wang, Ceram. Int., 2018, 44, 14425–14431 CrossRef CAS
.
- Q.-Q. Zhu, H.-W. Zhang, R. Yuan and H. He, J. Mater. Chem. C, 2020, 8, 15823–15829 RSC
.
- J. Fu, X. Wang, T. Wang, J. Zhang, S. Guo, S. Wu and F. Zhu, ACS Appl. Mater. Interfaces, 2019, 11, 33238–33244 CrossRef CAS PubMed
.
- S. Zhao, Y. Wang, J. Dong, C.-T. He, H. Yin, P. An, K. Zhao, X. Zhang, C. Gao and L. Zhang, Nat. Energy, 2016, 1, 1–10 Search PubMed
.
- W. S. Hummers Jr and R. E. Offeman, J. Am. Chem. Soc., 1958, 80, 1339 CrossRef
.
- H. Karimi-Maleh, M. Shafieizadeh, M. A. Taher, F. Opoku, E. M. Kiarii, P. P. Govender, S. Ranjbari, M. Rezapour and Y. Orooji, J. Mol. Liq., 2020, 298, 112040 CrossRef CAS
.
- A. Mesbah, P. Rabu, R. Sibille, S. Lebègue, T. Mazet, B. Malaman and M. François, Inorg. Chem., 2014, 53, 872–881 CrossRef CAS PubMed
.
- C. Shi, H. Cao, S. Li, L. Guo, Y. Wang and J. Yang, J. Energy Storage, 2022, 54, 105270 CrossRef
.
- J. Jin, Y. Zheng, S.-Z. Huang, P.-P. Sun, N. Srikanth, L. B. Kong, Q. Yan and K. Zhou, J. Mater. Chem. A, 2019, 7, 783–790 RSC
.
- B. Geng, F. Yan, X. Zhang, Y. He, C. Zhu, S. L. Chou, X. Zhang and Y. Chen, Adv. Mater., 2021, 33, 2106781 CrossRef CAS PubMed
.
- H. Rong, T. Chen, R. Shi, Y. Zhang and Z. Wang, ACS Omega, 2018, 3, 5634–5642 CrossRef CAS PubMed
.
- Q. Gan, B. Liu, K. Zhao, Z. He and S. Liu, Electrochim. Acta, 2018, 279, 152–160 CrossRef CAS
.
- Z. Li, P. Li, X. Meng, Z. Lin and R. Wang, Adv. Mater., 2021, 33, 2102338 CrossRef CAS PubMed
.
- S. H. Kazemi, B. Hosseinzadeh, H. Kazemi, M. A. Kiani and S. Hajati, ACS Appl. Mater. Interfaces, 2018, 10, 23063–23073 CrossRef CAS PubMed
.
- L. Naderi, S. Shahrokhian, M. K. Amini and M. Hafezi Kahnamouei, ACS Appl. Nano Mater., 2023, 6, 2755–2769 CrossRef CAS
.
- H. Wei, Q. Xue, A. Li, T. Wan, Y. Huang, D. Cui, D. Pan, B. Dong, R. Wei and N. Naik, Sens. Actuators, B, 2021, 337, 129687 CrossRef CAS
.
- Z. Zhao, Y. Sun, J. Song, Y. Li, Y. Xie, H. Cui, W. Gong, J. Hu and Y. Chen, Sens. Actuators, B, 2021, 326, 128811 CrossRef CAS
.
- Y. Miao, L. Ouyang, S. Zhou, L. Xu, Z. Yang, M. Xiao and R. Ouyang, Biosens. Bioelectron., 2014, 53, 428–439 CrossRef CAS PubMed
.
- U. T. Yilmaz, D. Uzun and H. Yilmaz, Microchem. J., 2015, 122, 159–163 CrossRef CAS
.
- S. Radhakrishnan, S. Lakshmy, S. Santhosh, N. Kalarikkal, B. Chakraborty and C. S. Rout, Biosensors, 2022, 12, 467 CrossRef CAS PubMed
.
- M. Baghayeri, E. N. Zare and M. M. Lakouraj, Sens. Actuators, B, 2014, 202, 1200–1208 CrossRef CAS
.
- A. Sun, J. Zheng and Q. Sheng, Electrochim. Acta, 2012, 65, 64–69 CrossRef CAS
.
- Y. Lin, B. Yuan and C. Wang, Adv. Energy Mater., 2018, 8, 1800584 CrossRef
.
|
This journal is © The Royal Society of Chemistry 2023 |
Click here to see how this site uses Cookies. View our privacy policy here.