DOI:
10.1039/D3AY00033H
(Paper)
Anal. Methods, 2023,
15, 2408-2416
Fabrication of a bi-metallic metal organic framework nanocomposite for selective and sensitive detection of triclosan†
Received
8th January 2023
, Accepted 27th March 2023
First published on 27th March 2023
Abstract
Transition metal-ion based nanocomposites are widely used owing to their ease of synthesis and cost-effectiveness in the sensor development. In this study, we have synthesized bi-metallic (iron and zinc) metal organic framework (MOF) nanorods-nanoparticles (denoted as Fe2Zn-MIL-88B) with a well-defined structure and characterized them. The bimetallic material nanocomposite (Fe2Zn-MIL-88B, nafion (Nf), and multiwalled carbon nanotube (MWCNT)) was fabricated on the electrode (glassy carbon electrode (GCE) or screen printed carbon electrode (SPCE)) surface within 10 min at room temperature. The Fe2Zn-MIL-88B/Nf/MWCNT@GCE showed an excellent electron transfer mechanism compared to a bare GCE and bare SPCE. The Fe2Zn-MIL-88B based nanocomposite electrode triggers the oxidation of the environmental carcinogenic molecule triclosan (TCS). Under optimized conditions, the sensor has a limit of detection of 0.31 nM and high selectivity to TCS in the presence of other interfering agents. The sensor has a good day-to-day TCS detection reproducibility. Fe2Zn-MIL-88B was stable even after 11 months of synthesis and detected TCS with similar sensitivity. The fabrication of the Fe2Zn-MIL-88B/Nf/MWCNT nanocomposite was successfully translated from the GCE to SPCE. TCS was detected in human plasma and commercial products such as soaps, skin care products, shampoos, and tooth pastes.
Introduction
Self-hygiene is an important part of everyday life and the current pandemic has taught the importance of it. To maintain self-hygiene, personal care products such as soaps, hand sanitizers, shampoos, and tooth pastes are used every day in every household.1 Along with other major substances, these products are also composed of few anti-bacterial, anti-fungal and anti-microbial agents as well. In particular, triclosan (TCS; 5-chloro-2-(2, 4-dichlorophenoxy) phenol) in its non-ionic form is one of the major substances used in those products to kill both Gram negative and Gram positive bacteria. However, excessive use of TCS in hygienic products can be counterproductive and has several harmful effects on human beings.2–5 Hence, monitoring TCS levels in hygienic products is of high importance.6 At present, there are multiple methods such as chromatography techniques,7–9 spectrophotometry,10 and electrochemical sensors11 for TCS detection. However, majority of these techniques require experience in handling, large amounts of reagents, and are time-consuming. Among these techniques, electrochemical sensors are easy to handle, cost effective, sensitive and selective in detection. In this regard, researchers have developed various nanomaterials using metals such as gold (Au), silver (Ag) and palladium (Pd) either individually or in combination with other materials as working electrodes.12–14 These metals are expensive compared to other metals such as iron (Fe), zinc (Zn), and nickel (Ni).
In the field of materials science, reticular chemistry has gained special attention due to its impressive properties. Metal organic frameworks (MOFs) have specific atoms of an organic molecule interlinked with metal atoms via coordination bonds. These materials possess tuneable features such as large surface area, and high porosity, and are easy building blocks in a relaxed approach.15 They are available in various formats such as nanorods,16 nanocages,17 nanoflowers,18 core–shell nanocomposites,17,19 nanoplates20,21 and nanohexagons.22 Due to their impressive unique features, there is a wide spread use of these materials in the fields of separation science, energy storage, electro catalysis, sensors and environmental applications.23–28 The most commonly used metal-ions for MOF synthesis are Fe, Cu, Co and Zr or various metal-ion nanoparticles. Although single metal-ion MOFs have displayed good stability and catalytic properties in sensing studies researchers started exploring the use of two metals in MOF synthesis.25,29 The reason for this is to further enhance the electrical conductivity, adsorption capacity, catalytic activity and selectivity or obtain luminescence properties. These properties can be achieved by fine-tuning the metal ions' molar ratio during MOF synthesis. So far MOFs (single or bimetallic) have been used for the detection of various analytes such as biomolecules,30,31 dyes,32,33 drugs,34 and metal ions.35 However, to the best of our knowledge, these materials were not explored for TCS detection. Based on the above merits of bimetallic MOFs, we for the first time have studied the potential of bimetallic MOFs for TCS detection.
Syntheses of some MOFs require multi-step processes to achieve high sensitivities.36–40 In the case of some MOFs, lack of uniform electro active surface area and agglomeration lead to their instability.41 To avoid these, a simple hydrothermal single step strategy would be the best option. In this work, we have synthesized bi-metallic (Fe and Zn) MOF nanorods-nanoparticles (Fe2Zn-MIL-88B) in a single-step process, where the metal-ions formed a coordination bond with the ligand (terephthalic acid). Combination of Fe2Zn-MIL-88B nanorods-nanoparticles with multi-walled carbon nanotubes (MWCNT) and the binder nafion (Nf) was successfully fabricated on a GCE (Fe2Zn-MIL-88B/Nf/MWCNT@GCE). The electrode has oxidized TCS at a potential of 0.62 V (vs. Ag/AgCl), confirmed using cyclic voltammetry (CV) and square wave voltammetry (SWV). In terms of limit of detection (LOD) and limit of quantification (LOQ), the performance was significantly similar to or higher than earlier reports (Au-nanoparticles,14 MWCNT,42 ZIF-11,6 and graphene quantum dots13). We have also successfully fabricated a Fe2Zn-MIL-88B/Nf/MWCNT@SCPE (SPCE: screen printed carbon electrode) strip and detected TCS in plasma and commercial products. Fig. 1 depicts the general overview of electrode fabrication and TCS sensing.
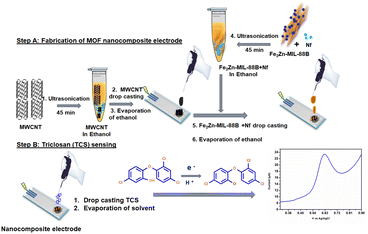 |
| Fig. 1 Schematic representation of Fe2Zn-MIL-88B/Nf/MWCNT nanocomposite fabrication on the working electrode and triclosan sensing. | |
Materials and methods
Chemicals and instrumentation
Potassium ferricyanide, nickel chloride, magnesium chloride, zinc nitrate hexahydrate, methyl salicylate, para-chlorophenol, zinc nitrate (Zn(NO3)2), potassium phosphate, 4-nitrophenol, calcium chloride, ethanol, ferric chloride hexahydrate (FeCl3·6H2O), multi-walled carbon nanotube (MWCNT), nafion (Nf) (5%), sodium hydroxide, sodium phosphate monobasic, sodium phosphate dibasic, dopamine, glucose, ascorbic acid, uric acid and terephthalic acid were purchased from Sigma Aldrich, India. An electrode polishing kit, glassy carbon electrode (GCE), Ag/AgCl electrode, platinum electrode and screen printed carbon electrode (SPCE) were purchased from Sinsil International Private Limited, India. Double distilled water was used for electrode cleaning and buffer preparation experiments. The synthesized Fe2Zn-MIL-88B was characterized using transmission electron microscopy (TEM; Tecnai, G2 20 Twin, FEI, USA), Fourier transform infrared spectroscopy (FT-IR; Shimadzu IR-Affinity 1, Shimadzu Scientific, Japan), powder XRD (D8 Advance, Bruker, Germany), field emission-scanning electron microscopy (FE-SEM; ThermoFisher Scientific FEI Quanta 250 FEG, The Netherlands) and UV-visible spectroscopy (JASCO, V-670 PC, Germany). All the electrochemical studies were performed using a CHI (1240C, USA) electrochemical workstation.
Preparation of Fe2Zn-MIL-88B
Fe2Zn-MIL-88B comprising both Fe and Zn and terephthalic acid was synthesized with slight modifications from ref. 43. Scheme 1 depicts the synthesis of Fe2Zn-MIL-88B along with its structure. FeCl3·6H2O (0.57 mmoL; 0.47 g) and Zn(NO3)2 (0.43 mmoL; 0.38 g) were mixed together with N, N-dimethyl formamide (10 mL) in a 50 mL round bottom flask. Subsequently, terephthalic acid (0.16 g) was added into the mixture, resulting in a homogeneous solution. This was followed by immediate addition of 0.2 M NaOH (2 mL) into the mixture to induce precipitation reaction. Subsequently, the mixture was stirred for another 1 h, followed by hydrothermal reaction in a 100 mL Teflon autoclave reactor at 100 °C for 15 h. After 15 h, the solution was cooled to room temperature and any unreacted components were removed using methanol (× 3 times wash) and water (× 3 times wash), followed by centrifugation at 6000 rpm at each step. Finally, the collected particles were dried in a hot air oven at 70 °C, which resulted in a dark reddish powder (0.31 g).
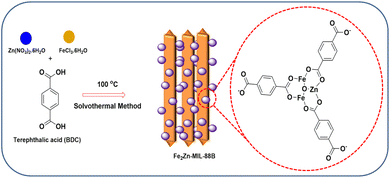 |
| Scheme 1 Schematic diagram of Fe2Zn-MIL-88B synthesis and its structure. | |
Physical characterization
Transmission electron microscopy (TEM).
TEM analysis was performed by taking 1 mg of Fe2Zn-MIL-88B in 1 mL of ethanol, followed by sonication for 10 min. Then 5 μL of this solution was coated on a copper grid around 3.05 mm. Subsequently, evaporation of ethanol solution was done, resulting in thin film formation. Finally, the sample containing grid was placed in the vacuum chamber of a TEM Tecnai, G2 20 Twin, FEI, USA, which was the instrument used to view and record the images.
Field emission-scanning electron microscopy (FE-SEM).
Fe2Zn-MIL-88B powder (1 mg) was placed on a carbon platform containing gold–palladium. Subsequently, the Fe2Zn-MIL-88B was sputter coated in a vacuum chamber for 60 s. Finally, Fe2Zn-MIL-88B was analysed in a ThermoFisher Scientific FEI Quanta 250 FEG. EDAX and mapping analysis was performed using an EDAX analyser.
Fourier transform infrared spectroscopy (FT-IR).
FT-IR studies were carried out with a Shimadzu IR-Affinity 1, Shimadzu Scientific, Japan. Prior to analysis, the sample holder was cleaned with ethanol, and dried and 2 mg of Fe2Zn-MIL-88B was placed on the sample holder. Then the holder was placed in the instrument and data were recorded in ATR mode.
X-ray diffraction (XRD).
The Fe2Zn-MIL-88B sample (5 mg) was coated as a thin film on a silicon zero balance background sample holder and placed in the instrument (D8 advance, Bruker, Germany). The applied wavelength for the analysis was Cu K- alpha radiation, 1.5406 Å, 40 kv voltage, and 30 mA current. Data were recorded using XRD Commander software.
Ultraviolet-visible spectroscopy (UV-VIS).
For UV-VIS measurements, a JASCO, V-670 PC, Germany, was used. 1 mg of Fe2Zn-MIL-88B was placed in an acetone cleaned quartz glass slide (19.8 × 1 mm). Using Spectra Manager software, the sample was analyzed from a wavelength of 200 to 800 nm.
Working electrode fabrication
Prior to fabrication, the glassy carbon electrode (GCE) was sonicated in ethanol for 1 min and cleaned using a rubbing pad with 0.5 μm alumina powder. This was followed by pre-treating the electrode in the potential range of −0.2 to 1.2 V vs. Ag/AgCl in PBS buffer (pH 7) with a scan rate at v = 50 mV s−1. Two nanomaterial solutions (A and B) were prepared separately. Solution A was composed of MWCNT (3 mg) in 500 μL ethanol. Solution B was composed of Fe2Zn-MIL-88B and Nf (450 μL of 3 mg MOF and 50 μL of 5% Nf) in 500 μL ethanol. Both solutions were sonicated for 45 min to obtain a homogeneous suspension of nanomaterials. Electrode fabrication is as follows: 5 μL of solution A was dropcast on the cleaned GCE or SPCE (screen printed carbon electrode) surface and dried at room temperature for 5 min. Subsequently, 5 μL of solution B was drop-cast and dried at room temperature for 5 min.
Cyclic voltammetry (CV) measurements
In this study, the modified glassy carbon electrode (GCE), Ag/AgCl and platinum electrodes were used as the working electrode, reference electrode and counter electrode, respectively.
The first set of experiments involved the GCE, MWCNT@GCE and Fe2Zn-MIL-88B/Nf/MWCNT@GCE as the working electrodes. The working electrode (GCE or MWCNT@GCE or Fe2Zn-MIL-88B/Nf/MWCNT@GCE) along with Ag/Agcl and platinum electrode were suspended in an electrochemical cell containing 14 mL of electrolyte solution (0.1 M PBS pH 7). Using a CHI 1240C workstation, a potential of 0 to 0.9 V vs. Ag/AgCl at 50 mV s−1 scan rate was applied to record the non-faradaic response of the prepared working electrodes.
The second set of experiments involved the use of 3 mM ferricyanide in 14 mL electrolyte solution (0.1 M PBS pH 7) and tested the redox behavior of the developed working electrodes (MWCNT@GCE or Fe2Zn-MIL-88B/Nf/MWCNT@GCE) in comparison to a blank GCE. The applied potential was from −0.1 to 0.6 V vs. Ag/AgCl at 50 mV s−1 scan rate.
The third set of experiments were carried out by varying the scan rate from 10 to 500 mV s−1 for the Fe2Zn-MIL-88B/Nf/MWCNT@GCE working electrode under the above mentioned conditions, i.e., from −0.1 to 0.6 V vs. Ag/AgCl; 14 mL electrolyte solution of 0.1 M PBS (pH 7) + 3 mM ferricyanide.
The catalytic response of all the three working electrodes (GCE or MWCNT@GCE or Fe2Zn-MIL-88B/Nf/MWCNT@GCE) to triclosan (TCS) was studied using 14 mL electrolyte solution of 0.1 M PBS pH 7. The applied potential was from 0 to 0.9 V vs. Ag/AgCl at 50 mV s−1 scan rate.
Square wave voltammetry (SWV) measurements
Using the SWV technique, the optimum pH condition, limit of detection (LOD) and selectivity of Fe2Zn-MIL-88B/Nf/MWCNT@GCE were studied at a potential from 0 to 0.9 V vs. Ag/AgCl at 50 mV s−1 scan rate.
For identifying the optimum pH condition, electrolyte solutions from pH 3 to 6 were prepared by adding 1 M orthophosphoric acid in 0.1 M PBS (pH 7) solution. Electrolyte solutions from pH 8 to 10 were prepared by adding 1 M NaOH in 0.1 M PBS (pH 7) solution.
For LOD experiment, the concentrations of TCS were varied from 0.00086 to 0.345 μM (0.86 nM to 345 nM). In the case of selectivity experiment, the concentration of TCS was fixed at 86 nM and interfering agent concentration was 60 μM. In both these cases, 14 mL of 0.1 M PBS (pH 7) was used as the electrolyte solution. The experiment was carried out at a potential from 0 to 0.9 V vs. Ag/AgCl at 50 mV s−1 scan rate.
Real sample preparation
Plasma (three donors) and commercial products (soap, shampoo, lipstick, tooth paste, and skin cream) were prepared separately in different medium. For plasma extraction, defrosted plasma was mixed with acetonitrile in 1
:
4 (v/v) ratio, vortexed for 2 min and centrifuged at 10
000 rpm for 5 min. Precipitated proteins were discarded and the clear supernatant layer was used for TCS analysis. In the case of commercial products, 0.1 g of the product was dissolved in MilliQ water according to ref. 44. Solutions (plasma and commercial samples) were directly dropcast on Fe2Zn-MIL-88B/Nf/MWCNT@GCE or Fe2Zn-MIL-88B/Nf/MWCNT@SPCE and analyzed for TCS amount in the presence of the electrolyte solution (0.1 M PBS pH 7) using the SWV technique.45
Results and discussion
Fe2Zn-MIL-88B synthesis and characterization
The synthesized Fe2Zn-MIL-88B was characterized for its structural morphology (TEM and FE-SEM), presence of functional groups and chelated metal-ions (FT-IR), diffraction patterns (XRD) and absorption spectral behaviour (UV-VIS). Fig. 2A is a TEM image of the prepared Fe2Zn-MIL-88B. The Fe2Zn-MIL-88B has highly interlinked clustered structures that are made up of a series of nanorods connected with each other via a series of nanoparticles (Fig. S1A and B†).43 Further scrutiny of the Fe2Zn-MIL-88B for elemental mapping with EDAX (in TEM) has confirmed the presence of metal ions Fe and Zn and O and C atoms as well (Fig. 2B). The surface morphology of the nanorods appears to be smooth and the FE-SEM image (Fig. 2C) has also confirmed a similar morphology for the synthesized Fe2Zn-MIL-88B. It was observed that the nanorods are 500–700 nm long with a width of 80–95 nm, while the nanostructures are globular in shape with a size of 50–100 nm. EDAX and element distribution map in FE-SEM (Fig. 2D and E) have confirmed that both Fe and Zn are present in both nanorods and nanoparticles as well. The mapped data clearly indicate that this Fe2Zn-MIL-88B (both nanorods and nanoparticles) predominantly contains Fe as compared to Zn. Similarly the high presence of both carbon and oxygen in nanorods and nanoparticles confirms that the metal-ions are chelated with BDD successfully. The predominance of Fe over other metals (Co, Ni and Mn) in MIL-88B is also reported earlier.43
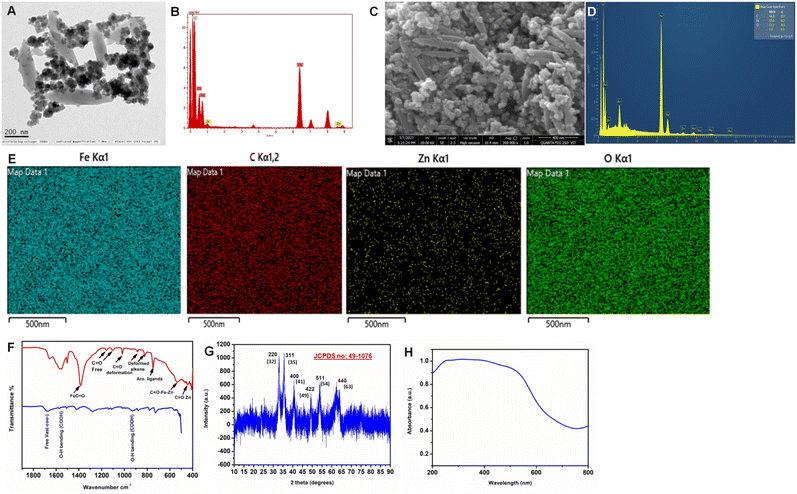 |
| Fig. 2 (A) TEM image, (B) EDAX analysis, (C) FE-SEM image, (D) EDAX analysis, (E) element mapping in FE-SEM analysis, (F) FT-IR analysis, (G) XRD pattern and (H) UV-VIS spectra of Fe2Zn-MIL-88B. | |
The FT-IR spectrum of Fe2Zn-MIL-88B explains the associated functional groups and chelated metal ions (Fig. 2F). Compared to the free ligand, i.e., terephthalic acid FT-IR spectrum, the Fe2Zn-MIL-88B spectrum is dominant with intense peaks and shifts in peak wave numbers. Formation of Fe2Zn-MIL-88B has resulted in disappearance of the typical adsorption peak of 1684 cm−1 (υas COO−) and resulted in two major (1555 cm−1 and 1380 cm−1) and two minor peaks (1660 cm−1 and 1510 cm−1) that correspond to υas COO− and υ COO−, respectively. The intense peak at 1380 cm−1 is due to the interaction of Fe with C
O groups, which indicates the dominance of Fe in Fe2ZnMIL-88B. These bands are due to the formation of coordination bonds between terephthalic acid and the two metal-ions, indicating the absence of any free ligands within its structure.43 The C–H stretching of continuous aromatic groups of the ligand was also observed at 748 cm−1. In addition, peaks at 885 and 821 cm−1 correspond to the deformed C–H alkene groups of terephthalic acid.46 In the fingerprint region, two peaks are observed at 534 and 455 cm−1. These peaks are a result of coordination bond formation between metal-ions (Fe and Zn) and the oxygen atom of carbonyl (C
O) groups of the ligand. In particular, the peak at 534 cm−1 indicates a triangular interaction between the two metal-ions and the ligand.43 The low intensity peak at 455 cm−1 is attributed to the formation of a bond between Zn and the oxygen atom of the ligand.47,48 This confirms the successful chelation of metal-ions with terephthalic acid and supports the formation of desired Fe2Zn-MIL-88B.
The diffraction pattern of the prepared MOF (Fig. 2G) has 6 major diffraction peaks (32°, 35°, 41°, 49°, 54°, and 63°) corresponding to 220, 311, 400, 422, 511, and 440 planes, respectively. This pattern is in good agreement with JCPDS no: 49-1076 and closely matched with that of the previously reported nanorod Fe2Zn-MIL-88B.49–51 The electron and energy state of the MOF was analyzed using UV-VIS spectroscopy.50–52 The spectrum (Fig. 2H) displayed a broad absorption peak starting from the wavelength (λmax) of ∼700 nm. This broad absorption peak is due to the presence of Fe–Zn metal cluster coordination with terephthalic acid53 and is a typical absorption peak observed for some Fe2Zn-MIL-88B.52
Cyclic voltammetry (CV) response of Fe2Zn-MIL-88B/Nf/MWCNT@GCE
The prepared Fe2Zn-MIL-88B was tested for its ability to detect triclosan (TCS). For this, Fe2Zn-MIL-88B was fabricated on a glassy carbon electrode (GCE) along with MWCNT and Nf. Nf is a conducting binder and in this case is used to bind both MWCNT and Fe2Zn-MIL-88B.
Initial experiments were conducted by the cyclic voltammetry (CV) technique. Fe2Zn-MIL-88B/Nf/MWCNT@GCE, MWCNT@GCE and GCE were used as electrodes in an electrolyte solution of 0.1 M PBS buffer (pH 7) at a scan rate of 50 mV s−1 in a potential (V) range of 0 to +0.9. However, none of the electrodes displayed faradaic behavior, which could be due to less number of H+ ions (Fig. 3A) in the electrolyte solution. Importantly, Fe2Zn-MIL-88B/Nf/MWCNT@GCE has displayed high conductivity over the remaining two electrodes. Fe-MOF is electrochemically active in acidic medium with a large number of H+ ions.54 It is known that H+ ions play a vital role to obtain faradaic response, which is proton coupled electron transfer.55 For Fe2Zn-MIL-88B, to obtain faradaic response use of a good redox mediator is required. Hence, we examined the electron transfer behavior of the Fe2Zn-MIL-88B/Nf/MWCNT@GCE in the presence of a widely popular and standard redox mediator, i.e., ferricyanide, in the above mentioned electrolyte solution and compared it with the remaining two electrodes. As can be seen in Fig. 3B, presence of ferricyanide within the same electrolyte solution has produced the redox peak for Fe2Zn-MIL-88B/Nf/MWCNT@GCE. This redox peak was significantly higher than that of the remaining two electrodes (MWCNT@GCE and GCE). Fig. 3C showcases the electron transfer behavior mechanism of the Fe2Zn-MIL-88B/Nf/MWCNT@GCE at varying scan rates of 10–500 mV s−1. A gradual increase of both anodic peak current (ipa) and cathodic peak (ipc) current (Fig. S2A and B†) was observed with increasing scan rate and is in agreement with ref. 56. Similarly, the other two electrodes were also subjected to varying scan rates from 10 to 500 mV s−1. However, none of those electrodes displayed faradaic currents even with increasing scan rates (Fig. S2C and D†). All these data suggest that the prepared Fe2Zn-MIL-88B has an efficient electron transfer property.
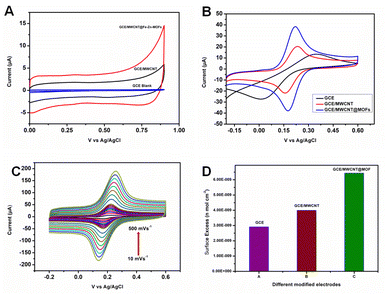 |
| Fig. 3 A) Cyclic Voltammetry (CV) of the GCE, MWCNT@GCE, and Fe2Zn-MIL-88B/Nf/MWCNT@GCE in 0.1 M PBS pH 7 at v = 50 mV s−1, (B) CV of the GCE, MWCNT@GCE, and Fe2Zn-MIL-88B/Nf/MWCNT@GCE in 0.1 M PBS pH 7 containing 3 mM [Fe(CN)6]3− at v = 50 mV s−1, (C) effect of scan rate on Fe2Zn-MIL-88B/Nf/MWCNT@GCE in 0.1 M PBS pH 7 containing 3 mM [Fe(CN)6]3− and (D) surface excess graph of the GCE, MWCNT@GCE, and Fe2Zn-MIL-88B/Nf/MWCNT@GCE. ipa values of different electrodes with TCS. | |
Additionally, the electron mediation through the surface of Fe2Zn-MIL-88B/Nf/MWCNT@GCE was observed using the surface excess (Γ) method. Γ = Q/nFA, where Q = charge, n = number of electrons, F = Faraday constant, and A = area of the electrode. The obtained data are presented in Fig. 3D using the recorded coulomb of the anodic current. The increasing order of surface excess was found to be GCE < MWCNT@GCE < Fe2Zn-MIL-88B/Nf/MWCNT@GCE, which is in accordance with ref. 57. Although the carbon material has excellent electron transfer properties, the combination of carbon and MOF along with an electron binder (nafion) has displayed enhanced electron kinetics throughout the surface with a surface excess value of 6.43 × 10−9 mol cm−2.58
Triclosan (TCS) electrochemical oxidation
The electrochemical oxidation of triclosan (TCS) was studied using the three electrodes (Fe2Zn-MIL-88B/Nf/MWCNT@GCE, MWCNT@GCE and GCE) in 0.1 M PBS buffer pH 7 (Fig. S3A†). The concentration of TCS was fixed at 604 nM. The obtained CVs are displayed in Fig. 4A. Fe2Zn-MIL-88B/Nf/MWCNT@GCE has oxidized TCS at a potential of 0.62 V with ∼19.2 μA, which was due to irreversible oxidation of the TCS benzene group. It is important to note that the obtained peak current (Ip) of TCS in the presence of MOF on the electrode surface is more than 7 fold higher than that of MWCNT@GCE and GCE, respectively. These data clearly indicate that the Fe2Zn-MIL-88B has an excellent catalytic property.14
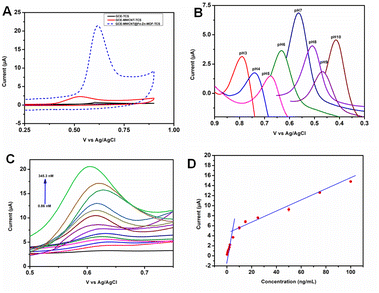 |
| Fig. 4 (A) CV curves of GCE, MWCNT@GCE, and Fe2Zn-MIL-88B/Nf/MWCNT@GCE in PBS pH 7 medium comprising TCS, (B) TCS oxidation at varying buffer pH on Fe2Zn-MIL-88B/Nf/MWCNT@GCE, (C) Square Wave Voltammetry (SWV) response of TCS oxidation with varying concentrations on Fe2Zn-MIL-88B/Nf/MWCNT@GCE in 0.1 M PBS pH 7, and (D) graph representing TCS concentration vs. current values on Fe2Zn-MIL-88B/Nf/MWCNT@GCE in 0.1 M PBS pH 7. | |
We have explored the oxidation of TCS by varying buffer pH from 3 to 10. As seen in Fig. 4B and S3B,† the oxidation ability of Fe2Zn-MIL-88B/Nf/MWCNT decreased below or above pH 7. Also a shift in the potential (v) was observed with varying pH, indicating the presence of protons in TCS oxidation. The linear fitting slope was found to be Ep = −0.053, which was close to the theoretical slope (−0.0590) derived from the Nernst equation.59 From this, we can understand that the protons and electrons are in equal number and reacted at the same time. During sensing studies, having a neutral electrolyte medium is ideal and favorable for analyte interaction with the modified surface. Based on our pH optimization experiment, the ideal pH to detect TCS is 7.
Limit of detection (LOD), limit of quantification (LOQ) and selectivity
We used square wave voltammetry (SWV) to study the analytical potential of Fe2Zn-MIL-88B/Nf/MWCNT@GCE by performing a series of experiments. The first experiment in this series was to evaluate the limit of detection (LOD) of the prepared electrode. Fig. 4C and D and S3C† depict the electrochemical oxidation of TCS and the anodic peak current in the concentration ranges of 0.00086–0.0172 μM and 0.0345–0.345 μM, respectively. With an increase of TCS concentration, the oxidation current signal has also increased gradually in a linear fashion. Also, a slight shift in the peak potential was observed, which could be due to the ease of TCS adsorption equilibrium on the electrode.14 At high TCS concentrations, the electrode surface is flooded with TCS. Hence it requires a longer time period to perform catalytic reaction. Also a possibility of electrode surface fouling could happen at high TCS concentrations. In case of low TCS concentrations, the electrode surface is not flooded with TCS. Hence the catalytic reaction of the electrode is fast and has resulted in the high sensitivity of the electrode response.14,60 The LOD and limit of quantification (LOQ) were calculated using61 (LOD = 3Sb/m and 10Sb/m, where Sb is the standard deviation value of the different concentrations and m is the analytical sensitivity obtained from the linear graph). The obtained LOD and LOQ for Fe2Zn-MIL-88B/Nf/MWCNT@GCE were found to be 0.31 nM and 2.1 nM, respectively. A comparison of our material performance with other existing materials (in terms of LOD and LOQ) is presented in Table 1. As can be seen the Fe2Zn-MIL-88B/Nf/MWCNT has displayed a significantly lower LOD for TCS than several existing sensors. The reason for this better analytical performance could be the stability of the two metal-ion (Fe and Zn) oxidation states in the single step synthesized Fe2Zn-MIL-88B as compared to other multi-step synthesized electrodes (mentioned in Table 1). The single step synthesis of Fe2Zn-MIL-88B has, therefore, enhanced the efficient catalytic conversion of TCS.62 To determine the selectivity of the Fe2Zn-MIL-88B/Nf/MWCNT@GCE sensor for TCS, different salts and chemical compounds with structural features similar to those of TCS were chosen. Based on earlier studies,6,12 the concentration of both interfering molecules and TCS was fixed as 86.3 nM.
Table 1 Detection of TCS reported in the literature versus this work
S.No |
Electrodesa |
Linear range (μM) |
LODb (nM) |
Methodc |
Ref. |
Different modified electrodes.
Obtained limit of detection.
Different voltammetry techniques.
|
1 |
DDT/Au-ErGO/GCE |
0.0005–20.0 |
0.253 |
DPV |
14
|
2 |
AgNPs/C3N4NTs@GQD/ILS/GCE |
0.000001–0.01 |
0.002 |
DPV |
13
|
3 |
PDDA-Gr/PdNPs/GCE |
0.009–20.0 |
3.5 |
DPV |
12
|
4 |
ZIF-11/RHAC/GCE |
0.1–8 |
76 |
DPV |
6
|
5 |
N-HC/Nafion/GCE |
0.09–20 |
30 |
DPV |
11
|
6 |
2-Hp-βCD |
0.001–10 |
9.86 |
— |
63
|
7 |
MWCNT/GCE |
0.172–6 |
57 |
DPV |
42
|
8 |
CNTs/Fe3O4@PPy/Pd |
0.0021–0.27 |
1.4 |
DPV |
64
|
9 |
CMK-3/CH/SPCE |
0.00276–0.138 |
0.82 |
SWV |
65
|
10
|
Fe
2
Zn-MIL-88B/Nf/MWCNT@GCE
|
0.00086-0.172 and 0.0345-0.345
|
0.31
|
SWV
|
This work
|
For selectivity studies, lower concentration of the target analyte in the presence of high concentration of interfering agents is a better choice. This is to prove the selective ability of the sensor. SWV experiment was conducted by preparing a series of binary mixtures of TCS and each interfering molecule individually. Selectivity evaluation was done by detecting the changes occurring at the maximum oxidation peak current at 0.62 V in 0.1 M PBS pH 7. Fig. 5A, S3D, and E† depict the observed changes in anodic current values (ipa in μA) for each tested binary mixture and SWV graphs, respectively. The observed average current value was 7.3 μA in the presence of interfering agents (IZn+, Ica+, Img+, INO3−, INi+, IMS, IPCP, INp, IPo4−, IAA, IDA, IGlu, and IUA). This value is significantly closer to the anodic current value of TCS, i.e., 7.5 μA. These data clearly indicate that Fe2Zn-MIL-88B/Nf/MWCNT@GCE has high selectivity to TCS in the presence of interfering substances with close structural similarity or salts. Electrode-to-electrode TCS sensing ability of Fe2Zn-MIL-88B/Nf/MWCNT on the GCE was tested by fabricating 7 independent electrodes. The obtained data indicate high TCS sensing reproducibility with a relative standard deviation of 0.31% (Fig. S4A†) and importantly with no significant change in the peak current value. Also, the stability of the Fe2Zn-MIL-88B was tested by storing the synthesized powder at room temperature for an 11 months period of time. The electrode prepared after 11 months has displayed similar oxidation potential for TCS to the electrode prepared during the 1st month of its synthesis (Fig. S4B†). This indicates that Fe2Zn-MIL-88B was stable even after 11 months of storage at room temperature.
Real sample testing
The application of Fe2Zn-MIL-88B/Nf/MWCNT@GCE was examined using human plasma and other commercial products such as soap, skin cream, etc. Extraction of the plasma sample and commercial products is explained in Section 2.4. Detection of TCS was performed using SWV and under optimized conditions. The obtained electrochemical oxidation SWV of all the samples is presented in Fig. S5A and B.† The overall extracted data from these graphs are presented in Fig. 5B. As can be seen, TCS was detected in all commercial samples and in the case of soap a very high amount of TCS was noticed. Importantly, all the three tested plasma samples were found to have TCS. To confirm that the obtained oxidation peak current is due to TCS, we have spiked the sample with two different TCS concentrations. As can be seen in Fig. 5C, with increasing TCS concentration the oxidation peak current also increased significantly at the TCS potential (0.62 V). This indicates that we have detected TCS only in the case of plasma (without spiking). We hypothesize that the daily use of hygiene items such as skin cream, soap, tooth paste, etc. would have resulted in slow penetration of TCS through the skin and into blood.
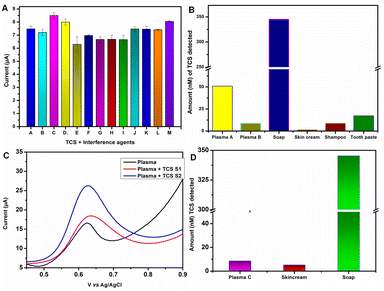 |
| Fig. 5 A) Peak current values of TCS in the presence of interfering agents: A) TCS, B) methyl salicylate + TCS, C) 4-nitrophenol + TCS, D) para-chlorophenol + TCS, E) calcium chloride + TCS, F) zinc chloride + TCS, G) magnesium chloride + TCS, H) nickel chloride + TCS, I) potassium phosphate + TCS, J) ascorbic acid + TCS, K) dopamine + TCS, L) glucose + TCS, and M) uric acid + TCS; B) amount of TCS detected in different samples using Fe2Zn-MIL-88B/Nf/MWCNT@GCE, C) SWV response of peak current values of TCS detected in plasma (before and after spiking with TCS; S1: 86.3 nM spiked and S2: 172.5 nM spiked) and D) amount of TCS detected in different samples using Fe2Zn-MIL-88B/Nf/MWCNT@SPCE. | |
Fabricating the MOF on a screen printed carbon electrode and testing
Based on the need to have an on-site detection strip, we explored the potential to translate the developed electrode (MOF/Nf/MWCNT) from the GCE to a commercially available screen printed carbon electrode (SPCE) strip. Under similar electrode fabrication and detection conditions, the MOF/Nf/MWCNT@SPCE detected TCS successfully in the tested samples (plasma, soap and skin cream; Fig. 5D). The detected TCS values are lower than those of Fe2Zn-MIL-88B/Nf/MWCNT@GCE. This result indicates that we need to optimize the electrode fabrication on the SPCE strip to achieve similar TCS concentrations. However, the positive outcome is that the Fe2Zn-MIL-88B/Nf/MWCNT@SPCE could detect TCS in biological and non-biological samples.
Conclusion
We have successfully synthesized iron–zinc based bimetallic nanorod shaped metal organic frameworks. A combination of Fe2Zn-MIL-88B with Nf and MWCNT on a glassy carbon electrode produced a well-defined and excellent oxidation current signal at 0.62 V in neutral electrolyte solution. This Fe2Zn-MIL-88B nanocomposite sensor has displayed excellent LOD and LOQ for TCS compared to majority of existing sensors and good selectivity in the presence of interfering agents. Presence of TCS in the nM range was observed in tested human plasma and commercial products. Under similar fabrication conditions, the nanocomposite was successfully translated from the GCE to the SPCE strip. We believe this work has opened doors for further exploring the potential of MOFs as materials for sensor application. With slight modifications in the fabrication approach, they can be used on strips as well.
Author contributions
Lokesh Kumar S: investigation, methodology, writing – original draft. Kishore K. R. Tetala: conceptualization, resources, funding acquisition, supervision, data curation, writing – review & editing.
Conflicts of interest
There are no conflicts to declare.
Acknowledgements
Dr Kishore K. R. Tetala acknowledges DBT (Department of Biotechnology, Government of India; BT/PR29096/PFN/20/1371/2018) for funding to establish electrochemical workstation platform. The authors thank FIST-DST, VIT for UV-VIS, FT-IR, TEM, FE-SEM and XRD analysis.
References
- R. Giné-Garriga, A. Delepiere, R. Ward, J. Alvarez-Sala, I. Alvarez-Murillo, V. Mariezcurrena, H. G. Sandberg, P. Saikia, P. Avello, K. Thakar, E. Ibrahim, A. Nouvellon, O. El Hattab, G. Hutton and A. Jiménez, Sci. Total Environ., 2021, 795, 148789 CrossRef PubMed.
- L. M. Weatherly and J. A. Gosse, J. Toxicol. Environ. Health, Part B, 2017, 20, 447–469 CAS.
- G. Bedoux, B. Roig, O. Thomas, V. Dupont and B. Le Bot, Environ. Sci. Pollut. Res. Int., 2012, 19, 1044–1065 CrossRef CAS PubMed.
- R. D. Jones, H. B. Jampani, J. L. Newman and A. S. Lee, Am. J. Infect. Control, 2000, 28, 184–196 CrossRef CAS PubMed.
- A. B. Dann and A. Hontela, J. Appl. Toxicol., 2011, 31, 285–311 CrossRef CAS PubMed.
- N. D. Luyen, T. T. T. Toan, H. T. Trang, V. T. Nguyen, L. V. T. Son, T. S. Thanh, N. M. Thanh, P. T. Quy and D. Q. Khieu, J. Nanomater., 2021, 2021, 8486962 Search PubMed.
- M. Cobo-Golpe, J. García-Martín, M. Ramil, R. Cela and I. Rodríguez, Anal. Bioanal. Chem., 2021, 413, 6355–6364 CrossRef CAS PubMed.
- H. C. Chen and J. W. Chang, Toxics, 2022, 10, 1–21 CAS.
- M. Ma, Y. Wei, H. Wei, X. Liu and H. Liu, RSC Adv., 2021, 11, 28632–28642 RSC.
- S. Gopalakrishnan, R. Ghosh, T. Renganathan and S. Pushpavanam, Spectrochim. Acta, Part A, 2021, 254, 119623 CrossRef CAS PubMed.
- X. Huang, A. Jiang, X. Jin, H. Yu, Y. Zhang, S. Xia, Z. Liu, G. Zhang and D. D. Dionysiou, J. Environ. Chem. Eng., 2021, 9, 106022 CrossRef CAS.
- T. Wu, T. Li, Z. Liu, Y. Guo and C. Dong, Talanta, 2017, 164, 556–562 CrossRef CAS PubMed.
- O. Akyıldırım, Bull. Mater. Sci., 2020, 43, 195 CrossRef.
- H. Zhou and Q. Lu, Microchem. J., 2022, 175, 107144 CrossRef CAS.
- C. Guo, F. Duan, S. Zhang, L. He, M. Wang, J. Chen, J. Zhang, Q. Jia, Z. Zhang and M. Du, J. Mater. Chem., 2022, 10, 475–507 RSC.
- S. Choi, T. Kim, H. Ji, H. J. Lee and M. Oh, J. Am. Chem. Soc., 2016, 138, 14434–14440 CrossRef CAS PubMed.
- Y. Wang, K. Kretschmer, J. Zhang, A. K. Mondal, X. Guo and G. Wang, RSC Adv., 2016, 6, 57098–57102 RSC.
- W. Sun, X. Tang, Q. Yang, Y. Xu, F. Wu, S. Guo, Y. Zhang, M. Wu and Y. Wang, Adv. Mater., 2019, 31, e1903176 CrossRef PubMed.
- S. Wu, G. Zhuang, J. Wei, Z. Zhuang and Y. Yu, J. Mater. Chem., 2018, 6, 18234–18241 RSC.
- M. Zhao, J. Chen, B. Chen, X. Zhang, Z. Shi, Z. Liu, Q. Ma, Y. Peng, C. Tan, X. J. Wu and H. Zhang, J. Am. Chem. Soc., 2020, 142, 8953–8961 CrossRef CAS PubMed.
- C. Li, X. Zhang, S. Wen, R. Xiang, Y. Han, W. Tang, T. Yue and Z. Li, J. Hazard. Mater., 2020, 395, 122615 CrossRef CAS PubMed.
- D. Sun and D. P. Kim, ACS Appl. Mater. Interfaces, 2020, 12, 20589–20595 CrossRef CAS PubMed.
- C. Li, X. Sun, Y. Yao and G. Hong, Mater. Today Nano, 2021, 13, 100105 CrossRef CAS.
- S. H. Yuan, A. P. Isfahani, T. Yamamoto, A. Muchtar, C. Y. Wu, G. Huang, Y. C. You, E. Sivaniah, B. K. Chang and B. Ghalei, Small Methods, 2020, 4, 2000021 CrossRef CAS.
- M. Chen, P. Zhu, B. Xu, R. Zhao, S. Qiao, X. Chen, R. Tang, D. Wu, L. Song, S. Wang, Y. Xia and X. Wang, J. Anal. Toxicol., 2012, 36, 608–615 CrossRef CAS PubMed.
- W. Qiu, F. Gao, N. Yano, Y. Kataoka, M. Handa, W. Yang, H. Tanaka and Q. Wang, Anal. Chem., 2020, 92, 11332–11340 CrossRef CAS PubMed.
- F. Gao, Y. Chu, Y. Ai, W. Yang, Z. Lin and Q. Wang, Chin. Chem. Lett., 2021, 32, 2192–2196 CrossRef CAS.
- Y. Ling, Y.-R. Chu, F. Gao, Q. Y. Feng, H. Q. Xie, Y. Shao and Q. X. Wang, J. Phys. Chem. C, 2022, 126, 9416–9424 CrossRef CAS.
- S. Liu, Y. Qiu, Y. Liu, W. Zhang, Z. Dai, D. Srivastava, A. Kumar, Y. Pan and J. Liu, New J. Chem., 2022, 46, 13818–13837 RSC.
- W. Pan, Z. Zheng, X. Wu, J. Gao, Y. Liu, Q. Yuan and W. Gan, Microchem. J., 2021, 170, 106652 CrossRef CAS.
- L. Gao and J. Zheng, Microchem. J., 2021, 171, 106782 CrossRef CAS.
- S. Feng, W. Ding, Y. Zhang, J. Wu, Z. Zou, T. Wu and Q. Tang, J. Solid State Chem., 2021, 303, 122508 CrossRef CAS.
- R. Darabi, M. Shabani-Nooshabadi, H. Karimi-Maleh and A. Gholami, Food Chem., 2022, 368, 130811 CrossRef CAS PubMed.
- L. Guo, L. Hao, Y. Zhang, X. Yang, Q. Wang, Z. Wang and C. Wang, Talanta, 2021, 228, 122228 CrossRef CAS PubMed.
- Y. Zhang, H. Zheng, P. Zhang, X. Zheng and Q. Zuo, J. Hazard. Mater., 2021, 408, 124917 CrossRef CAS PubMed.
- C. Gu, C. Guo, Z. Li, M. Wang, N. Zhou, L. He, Z. Zhang and M. Du, Biosens. Bioelectron., 2019, 134, 8–15 CrossRef CAS PubMed.
- W. Liang, M. Gao, Y. Li, Y. Tong and B.-C. Ye, Talanta, 2021, 225, 122042 CrossRef CAS PubMed.
- C. Chai, J. Gao, G. Zhao, L. Li, Y. Tang, C. Wu and C. Wan, Anal. Chim. Acta, 2021, 1152, 338242 CrossRef CAS PubMed.
- L. Gao and J. Zheng, Microchem. J., 2021, 171, 106782 CrossRef CAS.
- K. V. Kavya, D. Muthu, D. Pattappan, S. Vargheese, N. Gokila, M. S. Sivaramkumar, R. T. Rajendra Kumar and Y. Haldorai, Mater. Lett., 2022, 306, 130926 CrossRef CAS.
- M. Ahmadi, S. M. Ayyoubzadeh, F. Ghorbani-Bidkorbeh, S. Shahhosseini, S. Dadashzadeh, E. Asadian, M. Mosayebnia and S. Siavashy, Heliyon, 2021, 7, e06914 CrossRef CAS PubMed.
- J. Yang, P. Wang, X. Zhang and K. Wu, J. Agric. Food Chem., 2009, 57, 9403–9407 CrossRef CAS PubMed.
- C. Wu, X. Zhang, H. Li, Z. Xia, S. Yu, S. Wang and G. Sun, Chin. J. Catal., 2021, 42, 637–647 CrossRef CAS.
- X. Wang, M. Gao, J. Gao, X. Wang, M. Ma and H. Wang, J. Chromatogr. B: Anal. Technol. Biomed. Life Sci., 2018, 1092, 19–28 CrossRef CAS PubMed.
- M. J. Chen, Y. T. Liu, C. W. Lin, V. K. Ponnusamy and J. F. Jen, Anal. Chim. Acta, 2013, 767, 81–87 CrossRef CAS PubMed.
- P. Zierdt, T. Theumer, G. Kulkarni, V. Däumlich, J. Klehm, U. Hirsch and A. Weber, Sustainable Mater.Technol., 2015, 6, 6–14 CrossRef CAS.
- K. Handore, S. Bhavsar, A. Horne, P. Chhattise, K. Mohite, J. Ambekar, N. Pande and V. Chabukswar, J. Macromol. Sci. Part A Pure Appl. Chem., 2014, 51, 941 CrossRef CAS.
- K. Sowri Babu, A. Ramachandra Reddy, C. Sujatha, K. Venugopal Reddy and A. N. Mallika, J. Adv. Ceram., 2013, 2, 260–265 CrossRef CAS.
- D. T. C. Nguyen, H. T. N. Le, T. S. Do, V. T. Pham, D. Lam Tran, V. T. T. Ho, T. V. Tran, D. C. Nguyen, T. D. Nguyen, L. G. Bach, H. K. P. Ha and V. T. Doan, J. Chem., 2019, 2019, 5602957 Search PubMed.
- M. del Rio, J. C. Grimalt Escarabajal, G. Turnes Palomino and C. Palomino Cabello, Chem. Eng. J., 2022, 428, 131147 CrossRef CAS.
- L. Zheng, S. Yu, X. Lu, W. Fan, B. Chi, Y. Ye, X. Shi, J. Zeng, X. Li and S. Liao, ACS Appl. Mater. Interfaces, 2020, 12, 13878–13887 CrossRef CAS PubMed.
- H. Lv, L. Ma, P. Zeng, D. Ke and T. Peng, J. Mater. Chem., 2010, 20, 3665–3672 RSC.
- I. Chi-Durán, J. Enríquez, C. Manquian, K. Wrighton-Araneda, W. Cañon-Mancisidor, D. Venegas-Yazigi, F. Herrera and D. P. Singh, ACS Omega, 2018, 3, 801–807 CrossRef PubMed.
- R. Nivetha, K. Gothandapani, V. Raghavan, G. Jacob, R. Sellappan, P. Bhardwaj, P. Sudhagar, A. Kannan, S. Jeong and A. Grace, ACS Omega, 2020, 5, 18941–18949 CrossRef CAS PubMed.
- R. Tyburski, T. Liu, S. D. Glover and L. Hammarström, J. Am. Chem. Soc., 2021, 143, 560–576 CrossRef CAS PubMed.
- T. Sarkar, H. B. Bohidar and P. R. Solanki, Int. J. Biol. Macromol., 2018, 109, 687–697 CrossRef CAS PubMed.
- C. Bermúdez-Salguero and J. Gracia-Fadrique, J. Phys. Chem. B, 2015, 119, 5598–5608 CrossRef PubMed.
- J. Krejci, Z. Sajdlova, V. Nedela, E. Flodrova, R. Sejnohova, H. Vranova and R. Plicka, J. Electrochem. Soc., 2014, 161, B147–B150 CrossRef CAS.
- R. Porada, K. Fendrych and B. Baś, Microchim. Acta, 2021, 188, 323 CrossRef CAS PubMed.
- X. X. Dong, M. Y. Li, N. N. Feng, Y. M. Sun, C. Yang and Z. l. Xu, RSC Adv., 2015, 5, 86485–86489 RSC.
- G. L. Long and J. D. Winefordner, Anal. Chem., 1983, 55, 712A–724A CrossRef CAS.
- D. Bara, E. G. Meekel, I. Pakamorė, C. Wilson, S. Ling and R. S. Forgan, Mater. Horiz., 2021, 8, 3377–3386 RSC.
- N. Safwat, A. M. Mahmoud, M. F. Abdel-Ghany and M. F. Ayad, Environ. Sci. Processes Impacts, 2021, 23, 457–466 RSC.
- J. Zheng, M. Zhang, Y. Ling, J. Xu, S. Hu, T. Hayat, N. S. Alharbi and F. Yang, J. Electroanal. Chem., 2018, 818, 97–105 CrossRef CAS.
- M. Regiart, J. L. Magallanes, D. Barrera, J. Villarroel-Rocha, K. Sapag, J. Raba and F. A. Bertolino, Sens. Actuators, B, 2016, 232, 765–772 CrossRef CAS.
|
This journal is © The Royal Society of Chemistry 2023 |