Regulating the stemness of mesenchymal stem cells by tuning micropattern features†
Received
23rd October 2015
, Accepted 12th November 2015
First published on 12th November 2015
Abstract
There is increasing evidence that microstructures play an important role in the maintenance of the multipotency of stem cells. However, it is not clear how micropatterns affect the stemness of stem cells. We prepared micropatterns of different sizes, shapes and aspect ratios and used them for the culture of mesenchymal stem cells (MSCs) derived from human bone marrow to investigate their influence on the stemness of MSCs at the single cell level. The percentage of cells positively stained by stem cell markers decreased with increasing spreading area and aspect ratio. However, the cellular geometry controlled by the geometrical micropatterns had no significant influence on the expression of stem cell markers. The change in the stemness of stem cells was accompanied by changes in the nuclear activity and cytoskeleton. The nuclear activity increased with increasing spreading area and aspect ratio. The actin filament structure was significantly influenced by the spreading area and aspect ratio. The cells became stiffer when they had sufficient area to spread into or when they were elongated. These results suggest that controlling the cell morphology by micropatterns may be useful in varying the stemness of MSCs. This study will contribute to the design of materials for maintaining the multipotency of stem cells, enhancing their clinical application and helping to reveal the processes taking place under conditions of stem cell quiescence in vivo.
Introduction
Pluripotent stem cells have attracted much attention in tissue engineering and regenerative medicine because of their ability to differentiate into different cell types. Mesenchymal stem cells (MSCs) are one of the most commonly used stem cells as a result of their ready availability, high expansion efficiency and multilineage differentiation.1–4 Multipotency and self-renewal are the essential characters of stem cells.5In vivo, the majority of stem cells are quiescent under homeostatic conditions, but are capable of undergoing activation when stimulated.6 The quiescent state contributes to the maintenance of the stem cell phenotype. Stem cells may lose their pluripotency during in vitro expansion culture, which limits their clinical application.7 An interest in maintaining the stemness of stem cells during expansion culture has initiated plenty of research to determine the interactions between stem cells and various biomaterials.8–13 Biomaterials can provide various physiochemical and biological cues to interact with stem cells and therefore have a significant influence on their function. For instance, micropatterns that allow the geometric and mechanical control of cell morphogenesis have been used to regulate stem cell survival, proliferation and differentiation.14 However, the influence of the spreading area, aspect ratio and geometry of cells on the stemness of stem cells remains unclear. In this study, we prepared different micropatterns to control the spreading area, geometry and aspect ratio of single stem cells and determined the influence of these physical cues on the maintenance of the stemness of MSCs.
Materials and methods
Materials
All chemicals and reagents were purchased from Sigma-Aldrich unless stated otherwise. Polystyrene tissue culture flasks and dishes were purchased from BD Falcon. MSCGM medium was purchased from Lonza. Human MSCs were obtained from Osiris Therapeutics (Columbia, MA, USA) at passage 2. BrdU labelling reagent and the secondary antibodies used for immunofluorescence staining were purchased from Invitrogen (Grand Island, NY, USA). 4′,6-Diamidino-2-phenylindole (DAPI) mounting medium was purchased from Vector Laboratories (Burlingame, CA, USA). The primary antibodies mouse anti-CD44, mouse anti-CD45, mouse anti-CD106 and mouse anti-STRO-1 were purchased from R&D Systems (Abingdon, UK). Rabbit anti-CD11b and rabbit anti-CD73 antibodies were purchased from Novus Biologicals (Littleton, CO, USA). Mouse anti-CD19 was purchased from Imgenex (San Diego, CA, USA). Mouse anti-CD105 antibodies were purchased from Exbio (Vestec, Czech Republic). Mouse anti-CD34 antibodies were purchased from Cayman Chemical (Ann Arbor, MI, USA). The secondary antibodies Alexa Fluor 488-conjugated anti-mouse IgG, Alexa Fluor 488-conjugated anti-mouse IgM and Alexa Fluor 488-conjugated anti-rabbit IgG were purchased from Invitrogen. AFM cantilevers were purchased from Bruker (Camarillo, CA, USA) and Novascan Technologies (Ames, IA, USA).
Cell purification and culture
The human MSCs were seeded onto a cell culture dish (58.9 cm2) at passage 2. Around 20 cells were added into each dish and subcultured with MSCGM medium for 3 weeks to obtain cell colonies. Crystal violet (0.3%) was used to stain the colonies formed. Colonies >4 mm in diameter were collected and subcultured in 25 cm2 tissue culture flasks for another 3 weeks to obtain a homogeneous cell mass (Fig. 1a–e). The cells were treated with a serum-free low glucose DMEM medium (starvation) for 24 h to obtain cells in a G0/G1 enriched state. The cells were subsequently collected and seeded on different micropatterns at a density of 3000 cells per cm2. The cells were then cultured with low glucose DMEM medium supplemented with 10% FBS and 1% penicillin/streptomycin. The cells attached onto the micropatterned surfaces and spread within the micropatterns to show the same geometries as the underling polystyrene micropatterns.
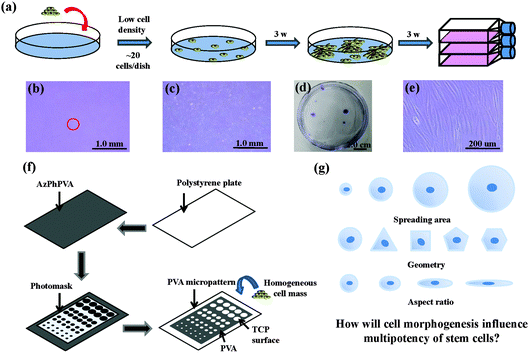 |
| Fig. 1 (a) Purification process used for the homogeneous mass of MSCs. (b) Phase-contrast micrograph of attached single cell on culture dish. (c) Phase-contrast micrograph of MSC colony after 3 weeks of culture. (d) Photograph of MSC colonies stained by crystal violet to show their size and distribution. (e) Phase-contrast micrograph of the confluent MSCs forming one MSC colony. The confluent MSCs retained their stem cell-like spindle shape. (f) Preparation of the micropatterns. (g) Schematic concept of this research. | |
Preparation and characterization of micropatterns
Photoreactive azidophenyl-derivatized poly(vinyl alcohol) (AzPhPVA) was synthesized as reported previously.15,16 The polystyrene plates were cut from the tissue culture flask and coated with 0.2 ml of 0.35 mg ml−1 AzPhPVA solution and then air-dried at room temperature in the dark. The plates were covered by photomasks with different micropatterns and irradiated with UV light (Funa-UV-linker FS-1500) at 0.25 J cm−2 at a distance of 15 cm. After irradiation, the plates were immersed in Milli-Q water and ultrasonicated to completely remove any unreacted polymer (Fig. 1f). Micropatterns of different sizes, shapes and aspect ratios were prepared to control the cell morphogenesis; this allowed us to investigate the influence of these biophysical features on the stemness of the MSCs (Fig. 1g). The surface topography of the PVA-micropatterned polystyrene plates was characterized using a MFP-3D-BIO atomic force microscope (Asylum Research, Santa Barbara, CA, USA). The micropatterns were sterilized with 70% ethanol followed by washing with aseptic water and were then used for the cell culture studies.
Immunofluorescence staining
The cells were stained for different surface markers to determine their multipotency. Cells were fixed with 4% paraformaldehyde for 10 min at room temperature and then blocked with a mixed solution of 2% BSA and 0.3 M glycine for 30 min followed by washing with PBS. The primary antibodies were diluted in 1% BSA solution at different concentrations according to the protocol. The samples were incubated with the diluted primary antibodies at room temperature for 1.5 h and washed three times with PBS. Secondary antibody labelling took place in 1% BSA solution at room temperature for 1 h. The cells were then permeabilized with 0.2% Triton X-100 in PBS solution for 2 min and stained with Alexa Fluor-594 phalloidin for 20 min. The nuclei were stained with DAPI. The percentage of cells positively stained with antibodies was counted by fluorescence microscopy. Only single cells were counted in each experiment and at least 100 single cells were analysed. Three independent experiments were performed to calculate the mean and standard deviation.
BrdU staining
After 6 h of culture of the MSCs on the micropatterns, non-adherent cells were removed by changing the medium and a low glucose DMEM medium supplemented with 10% FBS, 1% penicillin/streptomycin and 1% BrdU labelling reagent (v/v) was added. After 24 h of incubation, the cells were washed with PBS and fixed with 70% ethanol for 30 min followed by washing with PBS. Cells were denatured with 2 M HCl for 30 min and then 0.2% Triton X-100 was used to permeabilize the cells for 10 min. BSA (2%) in PBS solution was used to block the cells for 30 min. Cells were incubated with monoclonal mouse anti-BrdU primary antibody (1
:
200) at room temperature for 1.5 h and then with Alexa Fluor 488-conjugated anti-mouse IgG antibody (1
:
500) at room temperature for 1 h, followed by washing with PBS. A mounting medium with DAPI (Vector) was used to mount the samples and stain the nuclei. The percentage of stained cells incorporating BrdU was counted using fluorescence microscopy. Three independent experiments were performed to calculate the mean and standard deviation values.
Measurement of cell mechanical properties by atomic force microscopy
The cell mechanical properties were measured with a commercially available MFP-3D-Bio AFM microscope. An optical microscope was used to find the cells and control the position of the AFM tip. Silicon nitride cantilevers with a 600 nm diameter glass ball were used as the probe. Although the cantilevers had a nominal spring constant (k = 0.06 N m−1), the exact spring constant was measured before each experiment using the thermal tuning method.17 Force–volume height imaging was performed to acquire the cell height map. The scan size was set to 20 pixels × 20 lines in an 80 × 80 μm2 area. The image was recorded at an indentation velocity of 8 μm s−1 with a trigger force of 3 nN. The image acquired was used to select the region of interest in which the force curves were collected. All the force curves were obtained in the region with the highest number of cells at a loading rate of 4 μm s−1 and a trigger force of 3 nN. The samples were immersed in DMEM/HEPES serum and measured at room temperature. Live/dead staining was performed after the measurement to detect whether the cells were still alive.
The force curves were fitted to Hertz's contact model to calculate the Young's modulus of the cells. According to the probe geometry, a parabolic model was used:
| 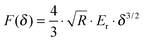 | (1) |
where
F is the loading force,
R is the radius of the tip,
Er is the reduced Young's modulus and
δ is the indentation depth. The reduced Young's modulus
Er is related to the Young's modulus of sample
Es and is given by:
| 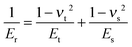 | (2) |
where
νt and
νs are the Poisson ratios of the tips and samples, respectively. As the Young's modulus of the tip material (SiO
2) is much greater than that of the living cells,
eqn (2) can be simplified to:
|  | (3) |
The Poisson ratio of the samples was assumed to be 0.5 because cells can be treated as soft incompressible materials.
18
Results
Preparation and characterization of the PVA-micropatterned polystyrene surfaces and purified cell mass
Photoreactive PVA was micropatterned on the polystyrene surface using UV photolithography. A transparent quartz slice with non-transparent micro-features at intervals was used as the photomask (Fig. 2a). Cell-adhesive polystyrene (PSt) micropatterns were surrounded by non-adhesive PVA (Fig. 2b). The thin PVA grafted to the substrate surface resisted cells from migrating and spreading across the PSt micropatterns. The height and 3D images of the micropatterns were observed by AFM in Milli-Q water in the contact mode (Fig. 2c and d and Fig. S1, ESI†). The diameters of the PSt micropatterns and the thickness of the grafted PVA layer were analysed by section analysis (Table S1, ESI†). The diameters of the PSt micropatterns were nearly the same as those of the designed photomasks, indicating the good controllability of the micropatterning method. The thickness of the grafted PVA varied from 59.66 to 67.98 nm, which was effective in constraining the cells in the PSt micropatterns. Three types of micropatterns with different spreading areas (Fig. S2, ESI†), geometries (Fig. S3, ESI†) and aspect ratios (Fig. S4, ESI†) were prepared. They were circular micropatterns with diameters of 20, 40, 60 and 80 μm and respective surface areas of 314, 1256, 2826 and 5024 μm2; the 1134 μm2 micropatterns had geometries of circles, triangles, squares, pentagons and hexagons and the 706 μm2 ellipse micropatterns had aspect ratios of 1, 1.5, 4 and 8. The micropatterns were used for the culture of MSCs to systematically compare the influence of different morphogenic cues on the functions of stem cells.
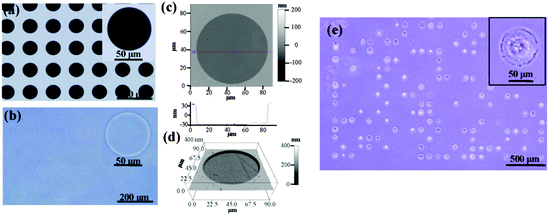 |
| Fig. 2 Characterization of the micropatterns. (a and b) Phase-contrast micrographs of the photomask and prepared micropattern. The micropattern had the same geometry as the designed photomask. (c and d) Height and 3D images of the circular micropattern with a diameter of 80 μm. (e) The size and depth of the micropattern were well controlled to support cell attachment and cell morphology confinement, as shown by the MSCs cultured on the circular micropattern with a diameter of 80 μm for 24 h. | |
MSCs derived from human bone marrow are usually primarily isolated by their tight adherence to plastic culture dishes, which will cause the initial heterogeneity.19 To obtain the homogeneous cell mass, human MSCs were purified based on clonal culture. The initial state of the purified MSCs was checked by immunofluorescence staining. The cells expressed CD73, CD105, CD44, CD106 and STRO-1 surface markers, which are commonly used to identify MSCs (Fig. S5, ESI†), but did not express CD11b, CD19, CD34 or CD45 (Fig. S6, ESI†).20–22 The purified homogeneous MSCs were cultured on the micropatterns. The MSCs attached onto the PSt micropatterns and their morphologies were controlled by the underlying polystyrene surfaces (Fig. 2e). Single MSCs arrays with different cellular sizes, geometries and aspect ratios were formed.
Influence of cell morphology on stemness of MSCs
After the MSCs had been cultured on the micropatterns for 2 weeks, the stemness of the MSCs was determined by the expression of CD44, CD73, CD105, CD106 and STRO-1 surface markers. Each surface marker was stained and the percentage of positive stained cells was counted to quantify the influence of the micropatterns on the variation in stemness of the MSCs. The expression of CD44, CD73, CD105, CD106 and STRO-1 gradually decreased with increasing spreading area (Fig. 3). MSCs cultured on the micropatterns with different geometries expressed similar levels of CD44, CD73, CD105, CD106 and STRO-1 (Fig. 4). The expression of CD44, CD73, CD105, CD106 and STRO-1 decreased slightly with an increase in the aspect ratio. Round cells (AR = 1) had a significantly higher expression of CD44, CD73, CD105 and CD106 than cells with an aspect ratio of 8 or 4 (Fig. 5). The results indicated that the size and aspect ratio of a single cell could affect the stemness of the MSCs, whereas the shape had no influence on the stemness of the MSCs when spreading was limited. A small size and low aspect ratio were good for the maintenance of the stemness of the MSCs.
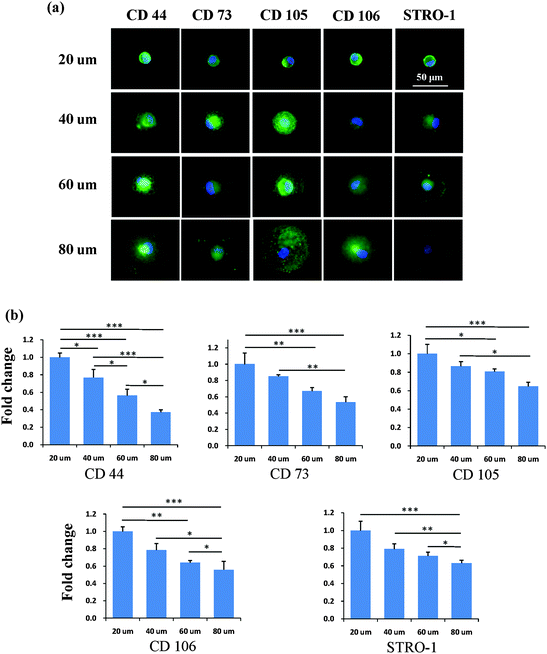 |
| Fig. 3 Influence of the spreading area on the expression of surface markers of single MSCs. (a) Representative positively stained MSCs with various spreading areas. Nuclei were stained by DPAI (blue) to distinguish single cells from multiple cells. The surface markers were stained green to quantify the percentage of positively stained cells, indicating the stemness of the MSCs. (b) Small micropatterned cells had a higher expression of CD44, CD73, CD105, CD106 and STRO-1 than large cells. In general, the number of positively stained cells decreased with increasing spreading area, indicating a loss of multipotency of MSCs. The data are presented as mean ± SD values, n > 120. *p < 0.05, **p < 0.01 and ***p < 0.001. | |
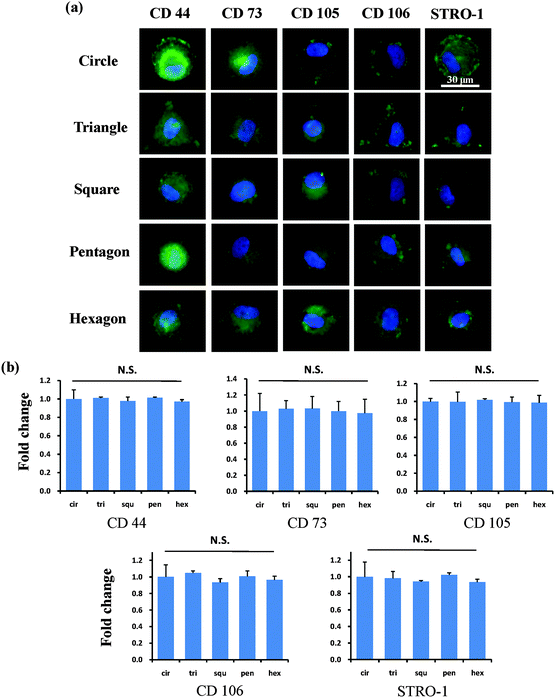 |
| Fig. 4 Influence of cell geometry on the expression of surface markers of single MSCs. (a) Representative positively stained MSCs with various geometries. Cells assembled strong stress fibres at the cell edge while the central part of the cell remained disordered. (b) There was no significant difference in the expression of surface makers among cells with different geometries. The data are presented as mean ± SD values, n > 120. N.S., no significant difference. | |
 |
| Fig. 5 Influence of aspect ratio on the expression of surface markers of single MSCs. (a) Representative positively stained MSCs with various aspect ratios. (b) An elongation in cell morphology led to pronounced nuclear deformation. Elongated cells had a lower expression of surface molecules than circular cells. The data are presented as mean ± SD values, n > 120. N.S., no significant difference, *p < 0.05, **p < 0.01, ***p < 0.001. | |
DNA synthesis of MSCs on micropatterns
We then investigated the mechanism of the stemness of MSCs tuned by the micropatterns. In vivo, stem cells are quiescent with a low activity in the nucleus and a low rate of metabolism, which gives them a superior long-term reconstitution potential.23 There is increasing evidence that stem cells in a quiescent state maintain their multipotency.24,25 Thus we investigated whether the micropatterns might also affect the stemness of the MSCs through the regulation of cell quiescence. To detect the influence of the micropatterns on cell quiescence, the cells were stained with BrdU, which reflects DNA synthesis in the cells (Fig. 6a). The results suggested that the spreading area had a significant influence on the nuclear activity. With increasing spreading area, more active nuclei were detected on the micropatterns (Fig. 6b). However, the cells cultured on micropatterns with different geometries, but the same spreading area, did not show a significant difference in BrdU staining (Fig. 6c). Elongation of cells with the same spreading area resulted in a gradual enhancement in the nuclear activity. When the aspect ratio was 8, the cells had a significantly higher nuclear activity than the round (AR = 1) or elliptical (AR = 1.5) cells (Fig. 6d). Therefore we concluded that a single MSC with a small size or low aspect ratio preferred to remain in a quiescent state with a low nuclear activity, which contributed to the maintenance of its stemness.
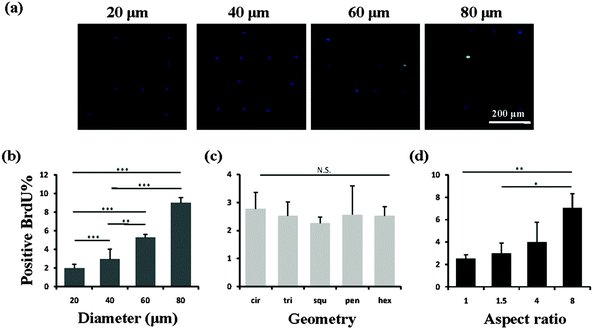 |
| Fig. 6 DNA synthesis activity of MSCs evaluated by BrdU staining. (a) Staining images of cell nuclei cultured on circular micropatterns with a diameter of 20, 40, 60 and 80 μm. Quiescent nuclei were stained by DAPI (blue) and activated nuclei were stained by anti-BrdU (green). (b) The nuclear activity increased with increasing spreading area. (c) Cells with different geometries had similar nuclear activity. (d) More positively stained nuclei were found in elongated cells. The data are presented as mean ± SD values, n > 120. N.S., no significant difference, *p < 0.05, **p < 0.01, ***p < 0.001. | |
Influence of cytoskeleton on cell mechanics
We then studied how the micropatterns modulated the nuclear activity. Previous studies have shown that cells respond to biophysical stimuli through the reorganization of their cytoskeleton.26 F-actin filaments can bind to the nuclear envelope, anchoring proteins and generating a force to the nucleus that influences its state.27 We thought that the micropatterns should regulate the cytoskeleton and influence the mechanical state of the cell, which could activate or passivate the nuclear activity and finally determine the stemness of the MSCs. To confirm this hypothesis, we first investigated the architecture of the cytoskeleton in single MSC arrays on the micropatterns from their F-actin staining images. The F-actin structure of single MSCs was significantly influenced by the spreading area (Fig. 7a). Circular cells with a large spreading area assembled their actin filaments in both the radial and concentric directions of the circle. With a decrease in the spreading area, the radial filaments gradually disappeared and the concentric filaments only assembled at the cell periphery. MSCs cultured on micropatterns with different geometries showed similar actin organization (Fig. 7b). The micropatterned cells predominately assembled their actin filaments at the periphery of the cells and stress fibres formed stretched along the edges of the micropatterns; no ordered filament structure was found at the central region of the cells. The aspect ratio had no significant effect on the F-actin structures. Unlike in circular cells, the actin filaments in elongated cells were parallel along the long axis of the cell and spanned over the nucleus (Fig. 7c). Not only the cytoskeleton, but also the nuclear geometry was elongated dramatically with an increase in the aspect ratio and was oriented towards the direction of long cell axis.
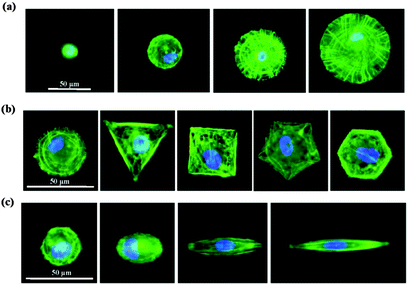 |
| Fig. 7 F-actin staining of MSCs cultured on micropatterns with various (a) spreading areas, (b) geometries and (c) aspect ratios. MSCs cultured on circular micropatterns with large spreading areas showed a highly ordered actin network. With decreasing spreading area, the actin filaments were weakened and became randomly oriented (a). Cells with different geometries formed strong stress fibres at the cell edge, while disrupted actin assembly was observed at the cell centre (b). With increasing aspect ratio, the MSCs formed straight stress fibres along the long axis of the cells (c). | |
The mechanical properties of the cells, which depend on the structure of the cytoskeleton, were then measured by AFM nanoindentation. The AFM measurement of each cell was finished within 1 h to guarantee cell viability and all the cells attached on the micropatterns were alive after the measurement. The obtained force curves were used to calculate the Young's modulus of cells according to Hertz's model. The final Young's modulus was determined by fitting the Gaussian function to the histogram created from all the collected data (Fig. 8). The centre of the fitting curve represented the average value of the Young's modulus and the half-width at half-height was the standard deviation. The histogram became wider with an increase in the spreading area, but showed a similar shape for cells with various geometries and aspect ratios. Table 1 gives the Young's modulus obtained for the MSCs. The results indicated that Young's modulus of the MSCs was regulated by their F-actin structure, which assembled according to the micropatterns. MSCs with large spreading areas formed more stress fibres, which assembled in the radial and concentric directions of the circle. The highly ordered actin structure resulted in a higher elasticity of the cells. With decreasing spreading area, the cells became soft. Cells with different geometries but with the same spreading area showed similar values for the Young's modulus. The parallel stress fibres formed in elongated cells also increased the Young's modulus of the MSCs.
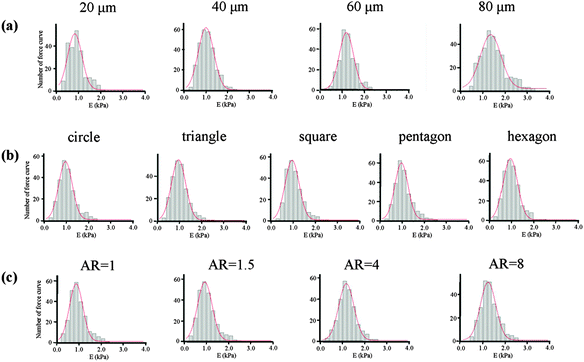 |
| Fig. 8 Histograms of the value of Young's modulus with Gaussian fittings obtained for MSCs cultured on micropatterns with various (a) spreading areas, (b) geometries and (c) aspect ratios. The data were obtained at 200 nm indentation depths. Bin size 0.2 kPa (n > 200). | |
Table 1 Young's modulus of living MSCs cultured on different micropatterns
Size (μm) |
E (kPa) |
Shape |
E (kPa) |
Aspect ratio |
E (kPa) |
20 |
0.82 ± 0.65 |
Circle |
0.96 ± 0.61 |
1 |
0.89 ± 0.60 |
40 |
0.99 ± 0.71 |
Triangle |
0.97 ± 0.64 |
1.5 |
0.93 ± 0.65 |
60 |
1.22 ± 0.65 |
Square |
0.95 ± 0.63 |
4 |
1.16 ± 0.68 |
80 |
1.38 ± 0.94 |
Pentagon |
0.97 ± 0.59 |
8 |
1.23 ± 0.69 |
|
|
Hexagon |
0.96 ± 0.64 |
|
|
Discussion
The maintenance of the multipotency of stem cells is of increasing interest and various methods have been used to investigate the self-renewal of stem cells. It has been reported that the culture of MSCs on a hard unpatterned surface for a prolonged period of time would cause irreversible effects by activating YAP and RUNX2 in the nucleus.28 The mechanical dosing effects remind us that it is difficult to preserve the multipotency of stem cells if only conventional tissue culture plates are used. In this study, micropatterns of different sizes, geometries and aspect ratios were used to culture MSCs at the single cell level to investigate how these physical cues affected the stemness of stem cells and changed the cytoskeleton. After two weeks of culture on the micropatterns, the expression of CD44, CD73, CD105, CD106 and STRO-1 decreased with increasing spreading area and aspect ratio of the MSCs, although it remained at a similar level in cells of different geometries.
The nuclear activity of the micropatterned cells increased with increasing spreading area. It is agreed that the spreading area is a crucial parameter in the nuclear deformation process and can enhance cell proliferation. An increase in spreading area leads to an enlargement of the nucleus, which activates DNA synthesis.29 At the same time, the cells need to maintain an intact actin cytoskeleton, which increases cell contraction at the critical time point in the late G1 phase of the cell cycle as it enters the S phase.30 The ordered actin structure observed in large cells could fulfil this requirement. While being cultured on micropatterns with different aspect ratios, the elongated cells assembled their parallel actin filaments throughout the cells. The perinuclear actin filaments formed a cap, which has been reported to stimulate cell proliferation.31–33 However, the non-elongated cells only assembled their actin filaments at the periphery of the cell. There were only a few actin filaments in the perinuclear space. Therefore no nuclear deformation was observed. The MSCs on the micropatterns with different geometries had a limited spreading area. The cells exhibited a similar disrupted actin structure at the perinuclear region, although they formed intensive stress fibres at the cell edge. These results indicate that the cytoskeletal structure might be an important factor in the regulation of cell quiescence, which contributes to maintaining the stem cell phenotype.
The cellular tension, which depends on the cytoskeletal organization, is also important for the maintenance of the multipotency of stem cells. It has previously been reported that round ESCs showed a higher expression of Oct4 and nanog than flattened cells as a result of weak membrane–cytoskeleton linkages.34 A limited spreading area was shown to be beneficial for the maintenance of the undifferentiated state of the ESCs.35 Human induced pluripotent stem cells with weak stress fibres were Oct3/4 positive, whereas those that formed pronounced stress fibres became Oct3/4 negative.36 For the MSCs, undifferentiated cells were found to have a low contractility compared with osteogenic differentiated cells, indicating that a low cytoskeletal tension was required to maintain the multipotency.37 In this study, the elasticity of MSCs cultured on the micropatterns increased with the cell size, which was in good agreement with previous work.28 Similarly, the parallel stress fibres formed in elongated cells enhanced the elasticity of the MSCs. MSCs with different geometries showed a disrupted actin structure at the cell centre, which led to a low elasticity. Combined with the staining results, the high elasticity of the MSCs was always accompanied by the low expression of surface molecules, suggesting a partial loss of multipotency.
Conclusions
Cell morphogenesis was well controlled by the PSt micropatterns of different sizes, geometries and aspect ratios. The MSCs on the micropatterns showed a different level of expression of stem cell surface markers, accompanied by different nuclear activities, cytoskeletal structures and nanomechanical properties. The micropatterns should directly affect the cytoskeletal structure. The resulting cytoskeletal structure could determine the nanomechanics, nuclear activity and stemness of the MSCs. A large spreading area and high aspect ratio produced a stressed state in the cells, with active nuclear synthesis, and therefore resulted in a low expression of stem cell surface markers. When the spreading area was limited, changes in the cell geometries did not influence the cell elasticity and nuclear activity. An ordered cytoskeletal structure resulted in high cell elasticity and nuclear activity and decreased the expression of surface markers, indicating a partial loss of multipotency. The MSCs with a disrupted cytoskeletal structure showed low nanomechanical properties and were retained in a quiescent state, which promoted the stem cell phenotype.
Acknowledgements
This work was supported by the World Premier International Research Center Initiative on Materials Nanoarchitectonics and JSPS KAKENHI Grant Numbers 24300177 and 15J01781 (Grant-in-Aid for Scientific Research (B)).
References
- F. P. Barry and J. M. Murphy, Int. J. Biochem. Cell Biol., 2004, 36, 568–584 CrossRef CAS PubMed.
- M. F. Pittenger, Science, 1999, 284, 143–147 CrossRef CAS PubMed.
- R. McBeath, D. M. Pirone, C. M. Nelson, K. Bhadriraju and C. S. Chen, Dev. Cell, 2004, 6, 483–495 CrossRef CAS PubMed.
- M. J. Dalby, N. Gadegaard, R. Tare, A. Andar, M. O. Riehle, P. Herzyk, C. D. W. Wilkinson and R. O. C. Oreffo, Nat. Mater., 2007, 6, 997–1003 CrossRef CAS PubMed.
- M. P. Lutolf, P. M. Gilbert and H. M. Blau, Nature, 2009, 462, 433–441 CrossRef CAS PubMed.
- Y. Kunisaki, I. Bruns, C. Scheiermann, J. Ahmed, S. Pinho, D. Zhang, T. Mizoguchi, Q. Wei, D. Lucas, K. Ito, J. C. Mar, A. Bergman and P. S. Frenette, Nature, 2013, 502, 637–643 CrossRef CAS PubMed.
- M. M. Bonab, K. Alimoghaddam, F. Talebian, S. H. Ghaffari, A. Ghavamzadeh and B. Nikbin, BMC Cell Biol., 2006, 7, 14–21 CrossRef PubMed.
- R. J. McMurray, N. Gadegaard, P. M. Tsimbouri, K. V. Burgess, L. E. McNamara, R. Tare, K. Murawski, E. Kingham, R. O. C. Oreffo and M. J. Dalby, Nat. Mater., 2011, 10, 637–644 CrossRef CAS PubMed.
- K. C. Rustad, V. W. Wong, M. Sorkin, J. P. Glotzbach, M. R. Major, J. Rajadas, M. T. Longaker and G. C. Gurtner, Biomaterials, 2012, 33, 80–90 CrossRef CAS PubMed.
- S. Gerecht, J. Burdick, L. S. Ferreira, S. Townsend, R. Langer and G. Vunjak-Novakovic, Proc. Natl. Acad. Sci. U. S. A., 2007, 104, 11298–11303 CrossRef CAS PubMed.
- P. M. Gilbert, K. L. Havenstrite, K. E. Magnusson, A. Sacco, N. A. Leonardi, P. Kraft, N. K. Nguyen, S. Thrun, M. P. Lutolf and H. M. Blau, Science, 2010, 329, 1078–1081 CrossRef CAS PubMed.
- J. H. Wen, L. G. Vincent, A. Fuhrmann, Y. S. Choi, K. C. Hribar, H. Taylor-Weiner, S. Chen and A. J. Engler, Nat. Mater., 2014, 13, 979–987 CrossRef CAS PubMed.
- P. Y. Wang, H. H. Lee, A. Higuchi, Q. D. Ling, H. R. Lin, H. F. Li, S. S. Kumar, Y. Chang, A. A. Alarfai, M. A. Munusamy, D. C. Chen, S. T. Hsu, H. C. Wang, H. Y. Hsiao and G. J. Wu, J. Mater. Chem. B, 2015, 3, 3858–3869 RSC.
- M. Thery, J. Cell Sci., 2010, 123, 4201–4213 CrossRef CAS PubMed.
- X. Wang, W. Song, N. Kawazoe and G. Chen, J. Biomed. Mater. Res., Part A, 2013, 101A, 3388–3395 CrossRef CAS PubMed.
- X. Wang, W. Song, N. Kawazoe and G. Chen, Soft Matter, 2013, 9, 4160–4166 RSC.
- B. Ohler, Rev. Sci. Instrum., 2007, 78, 063701 CrossRef PubMed.
- K. D. Costa, Dis. Markers, 2004, 19, 139–154 CrossRef.
- S. Zhang, Z. Jia, J. Ge, L. Gong, Y. Ma, T. Li, J. Guo, P. Chen, Q. Hu, P. Zhang, Y. Liu, Z. Li, K. Ma, L. Li and C. Zhou, Cell Transplant., 2005, 14, 787–798 Search PubMed.
- M. Dominici, K. Le Blanc, I. Mueller, I. Slaper-Cortenbach, F. Marini, D. Krause, R. Deans, A. Keating, D. Prockop and E. Horwitz, Cytotherapy, 2006, 8, 315–317 CrossRef CAS PubMed.
- M. K. Majumdar, M. Keane-Moore, D. Buyaner, W. B. Hardy, M. A. Moorman, K. R. McIntosh and J. D. Moska, J. Biomed. Sci., 2003, 10, 228–241 CrossRef CAS PubMed.
- D. Docheva, F. Haasters and M. Schieker, Curr. Rheumatol. Rev., 2008, 4, 155–160 CrossRef CAS.
- L. Li and H. Clevers, Science, 2010, 327, 542–545 CrossRef CAS PubMed.
- M. Osawa, G. Egawa, S. S. Mak, M. Moriyama, R. Freter, S. Yonetani, F. Beermann and S. I. Nishikawa, Development, 2005, 132, 5589–5599 CrossRef CAS PubMed.
- J. P. Winer, P. Jammey, M. E. McCormick and M. Funaki, Tissue Eng., Part A, 2009, 15, 147–154 CrossRef CAS PubMed.
- A. J. Engler, S. Sen, H. L. Sweeney and D. E. Discher, Cell, 2006, 126, 677–689 CrossRef CAS PubMed.
- K. N. Dahl, A. J. S. Ribeiro and J. Lammerding, Circ. Res., 2008, 102, 1307–1318 CrossRef CAS PubMed.
- C. Yang, M. W. Tibbitt, L. Basta and K. S. Anseth, Nat. Mater., 2014, 13, 645–652 CrossRef CAS PubMed.
- P. Roca-Cusachs, J. Alcaraz, R. Sunyer, J. Samitier, R. Farré and D. Navajas, Biophys. J., 2008, 94, 4984–4995 CrossRef CAS PubMed.
- A. Mammoto, S. Huang, K. Moore, P. Oh and D. E. Ingber, J. Biol. Chem., 2004, 279, 26323–26330 CrossRef CAS PubMed.
- S. B. Khatau, C. M. Hale, P. J. Stewart-Hutchinson, M. S. Patel, C. L. Stewart, P. C. Searson, D. Hodzic and D. Wirtz, Proc. Natl. Acad. Sci. U. S. A., 2009, 106, 19017–19022 CrossRef CAS PubMed.
- Q. Li, A. Kumar, E. Makhija and G. V. Shivashankar, Biomaterials, 2014, 35, 961–969 CrossRef CAS PubMed.
- K. Kurpinski, J. Chui, C. Hash and S. Li, Proc. Natl. Acad. Sci. U. S. A., 2006, 103, 16095–16100 CrossRef CAS PubMed.
- F. Veraitch, Cell Health Cytoskelet., 2011, 3, 23–34 CrossRef.
- D. Bae, S. H. Moon, B. G. Park, S. J. Park, T. Jung, J. S. Kim, K. B. Lee and H. M. Chung, Biomaterials, 2014, 35, 916–928 CrossRef CAS PubMed.
- M. H. Kim and M. Kino-Oka, Biomaterials, 2014, 35, 5670–5678 CrossRef CAS PubMed.
- P. M. Tsimbouri, R. J. McMurray, K. V. Burgess, E. V. Alakpa, P. M. Reynolds, K. Murawski, E. Kingham, R. O. Oreffo, N. Gadegaard and M. J. Dalby, ACS Nano, 2012, 6, 10239–10249 CrossRef CAS PubMed.
Footnote |
† Electronic supplementary information (ESI) available. See DOI: 10.1039/c5tb02215k |
|
This journal is © The Royal Society of Chemistry 2016 |