DOI:
10.1039/C7FO01616F
(Paper)
Food Funct., 2018,
9, 440-449
Evaluation of the in vivo acute antiinflammatory response of curcumin-loaded nanoparticles
Received
16th October 2017
, Accepted 24th November 2017
First published on 27th November 2017
Abstract
Curcumin is the main curcuminoid found in turmeric rhizomes and is a strong candidate to formulate foodstuff with specific properties. Among various bioactive properties of curcumin, its antiinflammatory activity is remarkable; on the other hand, its low water solubility leads to low absorption. Thus, new formulations need to be developed to improve its efficacy, and encapsulation is a promising alternative strategy in this regard. The objective of the present study was to obtain curcumin-loaded polyvinylpyrrolidone (PVP) nanoparticles and evaluate their acute in vivo antiinflammatory activity. Nanoparticles were obtained by complexation using the solid dispersion technique, and the characterization of nanoparticles showed that curcumin and PVP formed an amorphous solid solution. Encapsulated curcumin was colloidally stable in distilled water; this was attributed to the formation of hydrogen bonds between curcumin hydroxyl and PVP carbonyl groups. Rats were treated orally with single doses of curcumin and curcumin-loaded PVP nanoparticles, and antiinflammatory activity was evaluated by an experimental model of carrageenan-induced paw edema, myeloperoxidase (MPO) activity, and microcirculation in situ. Treatment with nanoparticles at 12.5 mg kg−1 significantly reduced the intensity of edema and MPO activity, whereas pure curcumin only presented a significant effect at 400 mg kg−1. Curcumin inhibited cell migration since rolling and adherent leukocytes were significantly reduced using nanoparticles at 50 mg kg−1 and curcumin at 400 mg kg−1. Compared to free curcumin, encapsulated curcumin was effective at lower doses; this might be due to the improved water affinity and colloidal stability of curcumin nanoparticles.
Introduction
The addition of naturally occurring bioactive substances to foodstuff has attracted attention since interesting properties may be added to products with already high consumer acceptance.1 Curcumin is a promising substance because it can be readily extracted from natural sources (the rhizomes of Curcuma longa L) and also because it presents very low toxicity since clinical trials have demonstrated that curcumin is safe at the oral doses of up to 8 g day−1.2,3 Curcumin is known for its numerous biological activities such as antioxidant,4,5 antitumor,6–10 antidiabetic,11–13 and antirheumatic.14–17 It also has the potential to substitute artificial colorants in industrialized food due to its improved coloring properties.
Curcumin presents remarkable antiinflammatory activity,18,19 which is important since inflammation is involved in a number of metabolic disorders. Inflammation is a biological defense response of the organism to tissue damage, which involves characteristic clinical signs as vascular and cellular alterations, vasodilatation, greater vascular permeability, and cellular migration.20 An important step in the inflammatory response is the recruitment of leukocytes, mainly neutrophils, to the lesion site where they act as first-line defense cells from the initial phase to the resolution of the process.21,22 This stage represents a multifactorial process that involves endothelial cells, adhesion molecules, and inflammatory mediators.23,24
The manifestations of inflammatory response are modulated by several inflammatory mediators such as cytokines, arachidonic acid derivatives, and nitric oxide, among others.25 Substances that can interfere with the production or release of these inflammatory mediators are important because an exaggerated and uncontrolled inflammatory response may be detrimental to the host.26 The use of nonsteroidal and steroidal anti-inflammatory drugs has been shown to be effective in the treatment of inflammatory disorders as these drugs inhibit the synthesis or release of inflammatory mediators that are involved in the process.27 However, these drugs can cause adverse effects such as gastrointestinal discomfort, inhibition of platelet aggregation, and renal toxicity.28 Thus, the search for alternative agents, including natural products such as curcumin, that have favorable therapeutic effects but fewer and less intense adverse effects has gained attention in the treatment of inflammatory diseases.
However, the low water solubility of curcumin leads to low bioavailability that hinders its industrial application. Previous studies have shown that orally administered curcumin has low bioavailability due to its poor absorption rates, rapid hepatic and intestinal metabolism, and rapid systemic elimination, whereas its metabolites show no pharmacological activity.29–31 Various strategies, including the use of chemical stabilizers, nanoparticle administration, liposome encapsulation, associations with micelles, and phospholipid complexes, have been used to improve the solubility and bioavailability of curcumin. Curcumin nanoencapsulation usually results in increased water solubility, improved dissolution rates, controlled release in the body, and increased bioavailability.32–35
Encapsulation in biocompatible substances, such as lipids dextran, PLGA, chitosan, polyvinyl alcohol, and polyvinylpyrrolidone, has been used for curcumin and other lipophilic drugs to increase their water affinity.36–39 It has been demonstrated that particle size, surface chemistry, and physical form can influence the way nanoparticles interact with plasma proteins as well as cell membrane uptake, toxicity, and molecular response.40,41 Manju and Sreenivasan36 showed that the conjugation of hydrophobic compounds with polymers increased the biological half-life of drugs, prevented the nonspecific adsorption of proteins, and enhanced therapeutic efficacy.
Although encapsulation is a promising technology, it usually leads to additional costs and complexity in the production process of the formulated drug. The influence of the encapsulation process on drug properties deserves to be investigated, and the actual gains in drug potency must be measured. Although the benefits of encapsulation have already been demonstrated in numerous practical applications, the determination of antiinflammatory activity of curcumin solid dispersions is still a work in progress. The objective of the present study was to obtain curcumin-loaded nanoparticles via the solid dispersion method and evaluate their acute in vivo antiinflammatory activity by measuring edema development, inflammatory mediator, such as myeloperoxidase, activity, and also the recruitment of leukocytes during the inflammatory process.
Materials and methods
Material
Polyvinylpyrrolidone (PVP; 40
000 g gmol−1, Sigma-Aldrich, 99% purity) was used as an encapsulant. Curcumin (70% purity) was acquired from Sigma-Aldrich. It is worth noting that the purity percentage of curcumin has been taken into account while comparing free and encapsulated curcumin with each other and also while calculating the in vivo doses. Distilled water and ethanol (Vetec, 99.5%) were used in nanoparticle production. KBr (Sigma-Aldrich, chromatographic grade) was used for spectroscopic analyses, and λ-carrageenan (Sigma-Aldrich) was used as the phlogistic agent. Hydrogen peroxide, O-dianisidine dihydrochloride, and sodium acetate (Sigma-Aldrich) were used as reagents for the determination of myeloperoxidase (MPO) activity. Gelatine (Vetec, 100%), sodium chloride (Anidrol, 99%), potassium chloride (Anidrol, 99%), D-glucose (Synth), and chloral hydrate (Vetec, 99%) were used to evaluate leukocyte migration.
Nanoparticle production
Curcumin-loaded PVP nanoparticles were obtained by the solid dispersion method using a previously described method with minor modifications.42 Polyvinylpyrrolidone (300 mg) was solubilized in distilled water (75 ml) under magnetic stirring (300 rpm). Separately, curcumin (50 mg) was solubilized in ethanol (25 ml) and then quickly added to the aqueous phase. This mixture was sonicated (Fisher Scientific, 120 W, 1/8′ probe) for 3 minutes in a pulse regime (30 seconds on followed by 10 seconds off). Ethanol was then completely removed by evaporation under vacuum (Buchi, Rotavapor R-3) at 50 °C. After evaporation, the dispersion was frozen, lyophilized, and stored at 10 °C under protection from light. This procedure resulted in a mass proportion of 6
:
1 (m
:
m) for PVP and curcumin.
Determination of average particle size and polydispersity index
Aliquots were obtained immediately after sonication and then every 10 minutes during the evaporation step for a total of 60 minutes. The particle diameter distribution, average particle size, and polydispersity index (PDI; eqn (1))43 have been determined by Dynamic Light Scattering (Malvern Instruments, Zetasizer Nano S) in triplicate using the following equation: |  | (1) |
where SD is the standard deviation of the size distribution, and Dz is the average particle size.
Evaluation of the interaction between PVP and curcumin
The quantitative evaluation of the chemical interaction between polymer and curcumin was performed by ultraviolet-visible spectroscopy (OceanOptics, Red Tide USB 650 UV) according to Karavas and coworkers.44 A curcumin ethanolic solution at the same concentration used for nanoparticle production was obtained, and its absorbance was determined at 426 nm. Known amounts of PVP were then added to this solution, and absorbance was again determined. The intensity of the PVP and curcumin interaction was quantified by the interaction factor F as defined by eqn (2),44 where A and A0 are the absorbances of the PVP-curcumin nanoparticle complex and pure curcumin, respectively. This procedure was performed in triplicate. |  | (2) |
Determination of colloidally stable curcumin
Polyvinylpyrrolidone-curcumin nanoparticles were obtained by the abovementioned procedure using different amounts of PVP and a constant mass of curcumin to achieve the following PVP
:
curcumin mass proportions: 0
:
1 (pure curcumin), 2
:
1, 4
:
1, 6
:
1, 14
:
1, 16
:
1, 18
:
1, and 20
:
1. Samples of each formulation (corresponding to 50 mg curcumin) were added to distilled water (5 ml) in screw-capped tubes and brought to a thermostatic bath at 25 °C under gentle stirring under protection from light. After 48 hours, the samples were filtered (0.45 μm), frozen, and lyophilized. After lyophilization, the samples were solubilized in methanol (2 ml), and absorbance was determined by ultraviolet-visible spectroscopy at 426 nm. The concentration of curcumin was determined using a previously obtained calibration curve in triplicate. Curcumin present in the filtrate was considered as colloidally stable curcumin.
Nanoparticle characterization
Differential scanning calorimetry (DSC; PerkinElmer 4000) was used to evaluate the physical state of curcumin after the encapsulation process. Lyophilized samples (approximately 10 mg) were placed in a closed aluminum sample holder and heated from 20 °C to 390 °C at 20 °C min−1 under a nitrogen flow (50 ml min−1). The equipment was previously calibrated using indium and zinc standards. X-ray diffractograms were obtained using an X-ray diffractometer (Shimadzu model LabX XRD-6000). Samples were investigated from 2° to 60° (2θ) at 5.9° min−1 using Kα radiation at 40 kV and 53 mA. Fourier transform infrared spectroscopy (FTIR; Shimadzu Affinity-1) was performed from 4000 to 600 cm−1 at a 2 cm−1 resolution and 32 cumulative scans of KBr pellets.
Animals and treatments
Herein, male Wistar rats weighing 200–220 g were used. The animals were kept under a controlled temperature of 22 °C and a 12 hours/12 hours light/dark cycle with water and food available ad libitum. Animals were maintained in the sectoral biotery of the Department of Pharmacology and Therapeutics, State University of Maringá (UEM). The experimental protocols were approved by the Ethics Committee on Animal Experimentation of the State University of Maringá (no. 5656300316).
The treatment of the animals was performed 1 h before induction of the inflammatory response with curcumin solution, PVP-curcumin nanoparticles, and indomethacin as an antiinflammatory reference drug. Solutions were administered orally in a single dose. A group of control animals received an equivalent volume of water (vehicle) by the same route of administration.
The animals were divided into the following groups (n = 5–7): (i) carrageenan + vehicle (control group), (ii) carrageenan + 5 mg kg−1 indomethacin, (iii) carrageenan + 50 mg kg−1 curcumin, (iv) carrageenan + 200 mg kg−1 curcumin, (v) carrageenan + 400 mg kg−1 curcumin, (vi) carrageenan + 12.5 mg kg−1 PVP-curcumin nanoparticles, (vii) carrageenan + 25 mg kg−1 PVP-curcumin nanoparticles, (viii) carrageenan + 50 mg kg−1 PVP-curcumin nanoparticles, and (ix) normal group (animals that did not receive any treatment and in which no inflammatory process was induced). In the case of nanoparticles, the abovementioned concentrations are the actual curcumin concentrations in each dose taking into account the curcumin concentration in the formulation of the nanoparticles.
Induction of paw edema
Rats received an intraplantar (intradermal) injection of 100 μl of carrageenan solution (200 μg per paw) dissolved in a 0.9% saline solution in the left hind paw and the same volume of vehicle (0.9% saline solution) in the contralateral paw. The volume of both paws was determined by plethysmography at the initial time of edema induction and then 1, 2, and 4 hours later, as described by Winter, Risley, and Nuss45 with minor modifications.
The increase in paw volume (edema) was calculated by subtracting the volume of the paw that received the saline solution (control paw) from the volume of the paw that received carrageenan (eqn (3)), where Vi is the initial paw volume, and Vf is the paw volume 1, 2, and 4 hours after the carrageenan injection.
Preparation of plantar tissue
Herein, four hours after edema induction, the rats were euthanized by high doses of ketamine/xylazine, and the plantar tissue was removed and placed in Eppendorf tubes that contained 0.6 ml of phosphate-buffered saline (PBS 4 mM, pH 5.4). Samples were then homogenized in a Potter homogenizer and centrifuged at 6000g at 4 °C for 15 minutes. The supernatant was immediately separated and stored at −70 °C for later analyses.
Determination of the myeloperoxidase activity
The activity of MPO was measured in the supernatant of plantar tissue homogenates according to the technique described by Bani and coworkers46 with minor modifications. The sample (0.1 ml) and 2.9 ml of PBS (50 mM, pH 6) containing O-dianisidine hydrochloride and 0.0005% H2O2 were placed in a 96-well microplate in triplicate. The reaction was stopped using a sodium acetate solution (1.46 M, pH 3.0). Enzyme activity was determined by the end-point technique at 460 nm.
Evaluation of leukocyte migration in situ
The process of leukocyte rolling and adhesion in the endothelium was evaluated in situ in the internal spermatic fascia in rats after a carrageenan injection (100 mg) in the scrotal wall. The rats were orally pretreated with curcumin or PVP-curcumin nanoparticles 1 hour before the carrageenan injection. Then, two hours after the carrageenan injection, the animals were anesthetized with chloral hydrate (500 mg kg−1, subcutaneous) and kept on a heated plate at 37 °C with a transparent orifice that allowed tissue trans-illumination and the observation of microcirculation vessels. After positioning the animals, the spermatic fascia was kept humid and heated using a Ringer–Locke solution (pH 7.2–7.4) that contained 1% gelatin. The selected vessels were postcapillary venules (15–25 μm diameter). The number of rolling and adherent leukocytes was determined in two venules over a period of 10 minutes. Leukocytes were considered adherent when they remained adhered to the endothelium for more than 30 seconds.
Fig. 1 presents a schematic of the experimental procedure including the nanoparticle preparation, characterization, and biological analyses.
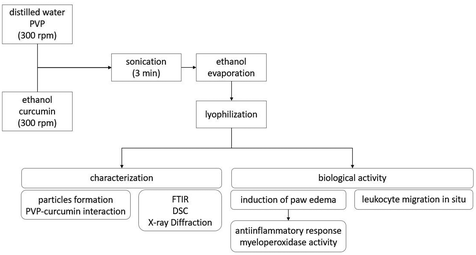 |
| Fig. 1 Schematic of the experimental procedure. | |
Statistical analysis
Results are expressed as mean ± standard error of the mean (SEM). Data were analyzed using analysis of variance (ANOVA) followed by the Tukey's test. The statistical analyses were performed using GraphPad Prism 5.0 software, and values of p < 0.05 were considered statistically significant.
Results and discussion
Nanoparticle characterization
Table 1 shows the average particle diameter (Dz) and PDI during the sonication step. Fig. 2 presents the particle size distribution immediately after sonication (Fig. 2A) and during ethanol evaporation (Fig. 2B).
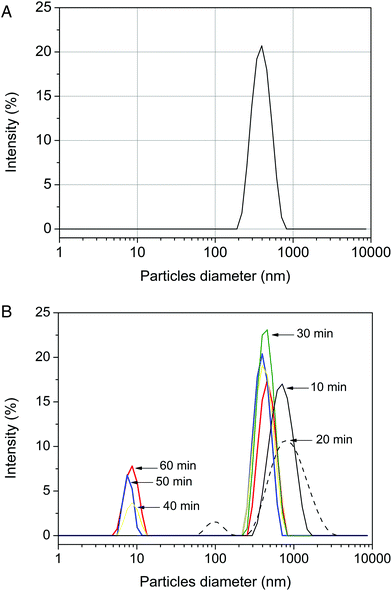 |
| Fig. 2 Particle size distribution changes during the nanoparticle production process. (a) Particle size distribution after sonication. (b) Particle size distribution during solvent evaporation. | |
Table 1 Average particle diameter (Dz) and polydispersity index (PDI) of the PVP-curcumin nanoparticles during sonication
Sonication time (min) |
D
z
(nm) |
PDI (−) |
1 |
349 ± 30 |
0.09 ± 0.04 |
2 |
372 ± 27 |
0.06 ± 0.04 |
3 |
380 ± 21 |
0.06 ± 0.02 |
PVP-curcumin nanoparticles with diameters between 200 and 700 nm were formed within the first minutes of sonication, which was in agreement with previous studies.42,47 As shown in Fig. 2A, nanoparticles with diameters around 10 nm were formed during the evaporation process probably because a small portion of curcumin remained soluble in the ethanol
:
water mixture and then precipitated in the form of nanoparticles (Fig. 2B). During sonication, there was only a small increase in the particle size; this suggested that 3 minutes of homogenization was sufficient for the formation of stable nanoparticles with a satisfactory mean diameter.
Fig. 3 shows the FTIR spectra of the PVP-curcumin nanoparticles, curcumin, and PVP. Fig. 4 shows the DSC thermograms of PVP-curcumin nanoparticles, curcumin, PVP, and the physical mixture of PVP and curcumin (manually mixed PVP and curcumin at the same concentration found in the nanoparticles). The thermal properties of curcumin, nanoparticles, and physical mixture are presented in Table 2. Fig. 5 shows the X-ray diffraction patterns of curcumin, PVP, nanoparticles, and the physical mixture.
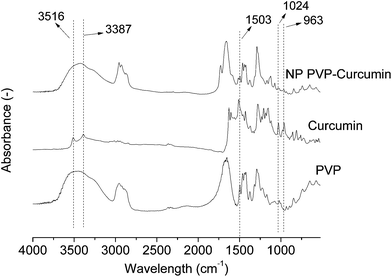 |
| Fig. 3 FTIR spectra of curcumin, PVP, and curcumin-loaded PVP nanoparticles. | |
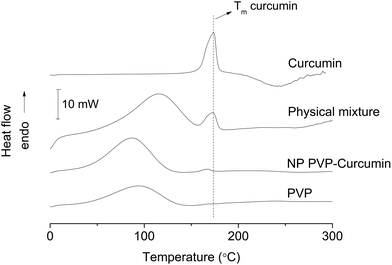 |
| Fig. 4 Differential scanning calorimetry thermograms of curcumin-loaded PVP nanoparticles, curcumin, PVP, and physical mixture of PVP and curcumin. | |
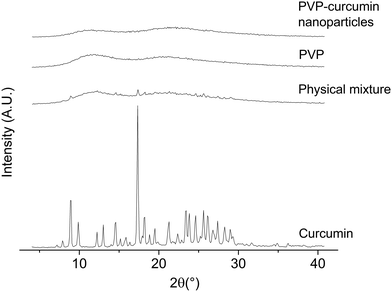 |
| Fig. 5 X-ray diffraction patterns of curcumin, PVP, physical mixture of PVP and curcumin, and curcumin-loaded PVP nanoparticles. | |
Table 2 Melting enthalpy (ΔHmcur) and melting temperature (Tmcur) of curcumin, curcumin-loaded PVP nanoparticles, and the physical mixture between PVP and curcumin
Sample |
ΔHmcur (J g−1) |
T
mcur (°C) |
Considering the mass ratio between PVP and curcumin in the nanoparticles and in the physical mixture.
|
Curcumin |
103.1 |
167.4 |
PVP-curcumin nanoparticles (8 : 1 m : m) |
40.2a |
172.6 |
Physical mixture |
98.0a |
172.8 |
The FTIR spectrum of curcumin indicates absorption bands relative to the stretching vibration of the curcumin hydroxyls (3516 and 3387 cm−1), which are generally used to identify these compounds. In the nanoparticles, these bands could not be visualized likely because of an attenuation effect and the presence of the bandwidth relative to adsorbed water near 3430 cm−1. Other characteristic curcumin absorption bands were found at 1024 cm−1 (C–O groups), 1503 cm−1 (C
O and C
C groups), and 963 cm−1 (aromatic C–H), which were consistent with previous studies.48–50 These bands can be seen in the spectrum of nanoparticles, although much attenuated, even considering their lower mass ratio in the sample of nanoparticles. The reduction in intensity is often considered indicative of efficient encapsulation.48,50
The DSC thermograms showed endothermic peaks at around 100 °C, which were related to the evaporation of water adsorbed on the highly hydrophilic polymer PVP. The melting of curcumin was observed at around 170 °C.48,50 The melting endothermic peak of curcumin was more attenuated in the nanoparticles than in the physical mixture despite the fact that both presented the same curcumin concentration. This is a strong evidence of efficient encapsulation of curcumin in PVP that may change its crystalline structure to form an amorphous solid solution. This was more evident while comparing the melting enthalpies and considering the sample compositions. The fusion enthalpy of the physical mixture (obtained by simple manual mixing of PVP and curcumin) and curcumin presented values that were very similar to each other. However, the melting enthalpy of the nanoparticles was lower, and this difference could be attributed to the distribution of curcumin in an amorphous form inside the nanoparticles, thus indicating its encapsulation. In fact, the melting enthalpy in the nanoparticles was 41% lower as compared to that of pure curcumin and very close to the percentage of curcumin that existed as colloidally stable nanoparticles (around 30%; Fig. 7).
Curcumin presented peaks at 8.9°, 9.7°, 12.2°, 13°, 14.6°, 17.3°, and 19.5° in the X-ray diffraction analysis, demonstrating its crystalline structure.51–54 No peaks were found in the PVP sample because this polymer was completely amorphous. Notably, curcumin peaks also appeared in the physical mixture, but characteristic curcumin peaks were not found in the nanoparticle sample; this suggested the conversion of curcumin from a highly crystalline state to an amorphous state inside the PVP matrix.
Nanoparticles in water
Fig. 6 shows the ultraviolet-visible spectra of curcumin, PVP, and the ethanolic solution that contained PVP and curcumin (10
:
1 mass proportion). Fig. 7 presents the interaction factor F for increasing PVP
:
curcumin mass proportions. Fig. 8 shows the presence of colloidally stable fractions of curcumin for different nanoparticle compositions.
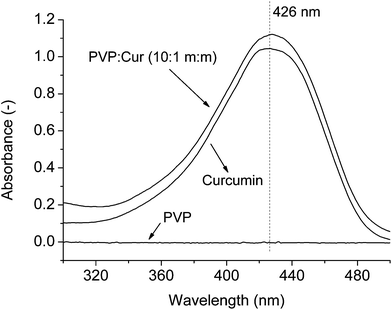 |
| Fig. 6 Ultraviolet -visible spectra of ethanolic solutions of PVP, curcumin, and PVP : curcumin (10 : 1 mass proportion). | |
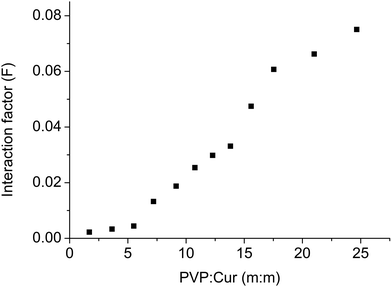 |
| Fig. 7 Interaction factor (F) for increasing PVP : curcumin mass proportions. | |
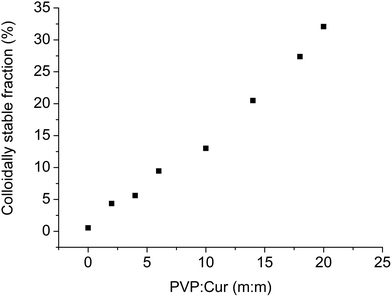 |
| Fig. 8 Percentage of colloidally stable curcumin in water for nanoparticles with different PVP : curcumin mass proportions. | |
The solvent (ethanol) was the same as that used to synthesize the nanoparticles; this meant that the obtained results corresponded to the intensity of interactions that occurred during the formation of the particles. The addition of polymer to the system increased the absorbance that was related to curcumin at 426 nm. This increase could not be due to the PVP spectrum because it presented no response at this wavelength. The increase in absorption can be attributed to the hydrogen bonds between the carbonyl group of the polymer and the hydroxyl group of curcumin. Karavas and coworkers44 observed a similar behavior in a study of PVP and felodipine interactions, in which higher concentrations of polymer in the felodipine solution presented higher absorbance at the wavelength that referred to the drug. The authors also identified the formation of hydrogen bonds between the compounds. Liu et al.25 investigated the interaction of curcumin with isolated milk protein using ultraviolet-visible and fluorescence spectroscopy, and they observed an increase in the interaction between the compounds as the amount of protein in the solution increased. The authors attributed this behavior to the formation of complexes via molecular interactions. Valero and coworkers55 evaluated the interaction between naproxen and PVP using ultraviolet-visible spectroscopy and fluorescence and found that there was an interaction ion–dipole between the compounds.
The percentage of curcumin that presented colloidal stability in the aqueous system increased with the increasing concentration of PVP. Compared with the results shown in Fig. 7, this behavior was similar to the interaction between PVP and curcumin, and it supported the hypothesis of the formation of hydrogen bonds between the carbonyl and hydroxyl groups that resulted in an increase in the hydrophilic character of curcumin.44 Mura et al.56 evaluated the solubility of the naproxen-chitosan-PVP system and observed an increase in naproxen solubility in water; this was attributable to the formation of weak intermolecular forces between the polymer and naproxen. Sethia and Squillante57 also found a linear correlation between the solubility of carbamazepine and the PVP concentration. The same behavior was verified by Frizon et al.58 when they evaluated the solubility of loratadine through its interactions with PVP.
Carrageenan-induced paw edema in rats
Fig. 9A and B show the antiedematogenic activity of free curcumin (curcumin) and curcumin-loaded PVP nanoparticles, respectively.
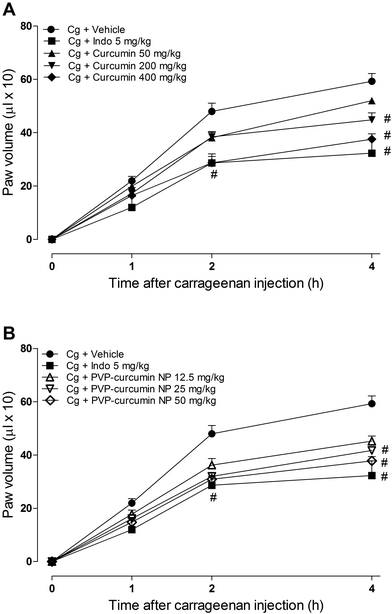 |
| Fig. 9 Effect of curcumin and curcumin-loaded PVP nanoparticles on the development of carrageenan (Cg)-induced edema (200 μg per paw) in rats. (A) Animals treated with curcumin at the doses of 50, 200, and 400 mg kg−1. (B) Animals treated with PVP-curcumin nanoparticles at the doses of 12.5, 25, and 50 mg kg−1. The data are expressed as the mean ± standard error of the mean of paw volume 1, 2, and 4 hours after the carrageenan injection. #p < 0.05, compared with the control group (ANOVA followed by Tukey's test). | |
The intraplantar injection of carrageenan induced a local inflammatory response that peaked 4 hours after the injection. This response was manifested by edema formation, an increase in cell migration, and consequently more leukocytes at the lesion site.59
Treatment with curcumin at a dose of 200 mg kg−1 significantly reduced (24.39% reduction, p < 0.05) the intensity of edema 4 hours after the carrageenan injection. Treatment with curcumin at a dose of 400 mg kg−1 significantly reduced the intensity of edema 2 and 4 hours after the carrageenan injection (36.59% and 40.11%, respectively, p < 0.05). Treatment with 50 mg kg−1 curcumin did not influence the development of the inflammatory response as compared to the case of the control group (Fig. 9A).
Treatment with curcumin-loaded PVP nanoparticles at the doses of 25 and 50 mg kg−1 significantly reduced the intensity of edema 2 and 4 hours after the carrageenan injection (43.63% and 43.65% at the 2nd hour and 34.98% and 37.78% at the 4th hour, respectively). Treatment with the nanoparticles at a dose of 12.5 mg kg−1 significantly reduced the intensity of edema only 4 hours after the carrageenan injection (23.71% reduction). Treatment with the reference antiinflammatory drug indomethacin at a dose of 5 mg kg−1 significantly reduced the inflammatory response 2 and 4 hours after the carrageenan injection (40.19%, p < 0.05, and 45.43%, p < 0.05, respectively). Treatment with a higher dose of curcumin and the 25 and 50 mg kg−1 doses of curcumin-loaded PVP nanoparticles reduced edema similar to the case of indomethacin. These data demonstrate the greater efficacy of encapsulated curcumin in reducing the intensity of paw edema as compared to that of free curcumin, in which the same inhibitory effect on the inflammatory response is achieved with doses that are 16-fold lower for encapsulated curcumin.
Carrageenan-induced paw edema involves the participation of several inflammatory mediators including histamine, bradykinin, prostaglandin, and nitric oxide.60 This model is commonly used to investigate potential antiinflammatory agents.59,61,62 Previous studies have reported that the antiinflammatory activity of curcumin may be attributable to inhibition of the expression of the enzyme cyclooxygenase 2.63–65 Recent studies showed that curcumin reduced the production of proinflammatory cytokines such as tumor necrosis factor-α, interleukin-1, interleukin-8, and nitric oxide synthase.18,66 Thus, the inhibitory effect of curcumin on carrageenan-induced paw edema, regardless of whether it is encapsulated or non-encapsulated, may be related to its action on inflammatory mediators.
Myeloperoxidase activity
The effect of curcumin and curcumin-loaded PVP nanoparticles on myeloperoxidase activity (MPO) is presented in Fig. 10.
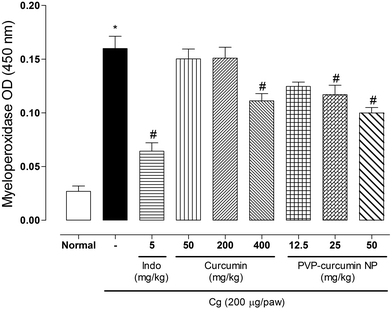 |
| Fig. 10 Effect of curcumin and curcumin-loaded PVP nanoparticles on MPO activity in plantar tissue in rats. Animals were treated with curcumin at the doses of 50, 200, and 400 mg kg−1 or PVP-curcumin nanoparticles at the doses of 12.5, 25, and 50 mg kg−1. Control rats received vehicle (water) orally 1 h before the carrageenan injection. The data are expressed as the mean ± standard error of the mean of MPO activity 4 hours after the carrageenan injection. *p < 0.05, compared with the normal group; #p < 0.05, compared with the control group (ANOVA followed by Tukey's test). | |
Myeloperoxidase is an enzyme found in intracellular neutrophil granules. Its catalytic activity provides it the ability to react with various substrates that can generate these intermediates as free radicals that can oxidize host cell structures and alter cellular responses, thus causing tissue damage.67,68 Myeloperoxidase is used as an indirect marker of leukocyte migration to the site of inflammation.
The activity of MPO in plantar tissue increased 4 hours after the carrageenan injection. Treatment with curcumin at a dose of 400 mg kg−1 and curcumin-loaded PVP nanoparticles at 25 and 50 mg kg−1 significantly reduced the MPO activity (30.63% for curcumin and 26.88% and 38.13% for nanoparticles, respectively). Treatment with curcumin at 50 and 200 mg kg−1 and nanoparticles at a dose of 12.5 mg kg−1 did not significantly alter MPO activity. Treatment with indomethacin significantly reduced the MPO activity (60% reduction, p < 0.05).
Leukocyte migration and consequently cell recruitment at the lesion site are important steps in the evolution of the inflammatory process, the purpose of which is to resolve inflammation. However, in the presence of an intense inflammatory response, exacerbated leukocyte migration may occur, resulting in the production/release of reactive oxygen and nitrogen species, proteolytic enzymes, chemotactic agents, and arachidonic acid metabolites that cause harmful effects on tissue.67–69 The reduction of neutrophilic infiltrate can reduce tissue damage, contributing to the antiinflammatory effect of curcumin.
Leukocyte migration in situ
Considering the abovementioned data, it is important to investigate the rolling behavior and adhesion of leukocytes in the endothelium. These parameters were evaluated in situ in the internal spermatic fascia in rats using the higher doses of curcumin and curcumin-loaded PVP nanoparticles. Fig. 11A and B present the rolling and adhesion of leukocytes in situ, respectively.
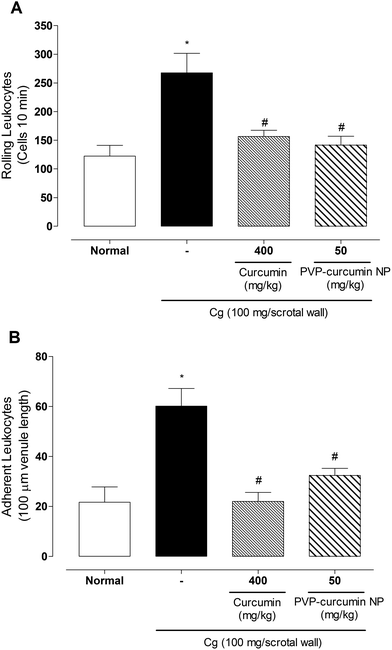 |
| Fig. 11 Leukocyte rolling (A) and adhesion (B) in microcirculation vessels in the spermatic fascia in rats, evaluated by the in situ microcirculation technique. Rats were treated orally with curcumin at a dose of 400 mg kg−1 and curcumin-loaded PVP nanoparticles at a dose of 50 mg kg−1. The data are expressed as mean ± standard error of the mean. *p < 0.05, compared with the normal group; #p < 0.05, compared with the control group (ANOVA followed by Tukey's test). | |
Treatment with both curcumin and curcumin-loaded PVP nanoparticles significantly reduced rolling leukocytes (41.46% and 47.07%, respectively) and adherent leukocytes (63.44% and 46.15%, respectively). The nanoparticles were more potent; they exerted the same effect as free curcumin, but at an eight-fold lower dose. Although the present study did not investigate the mechanism involved in the effects of curcumin and curcumin-loaded PVP nanoparticles on leukocyte migration, results suggested that curcumin and curcumin-loaded PVP nanoparticles exerted inhibitory effects on chemotactic inflammatory mediators or molecules that were involved in the migration process. Curcumin has been shown to have an inhibitory effect on cell migration due to its inhibitory activity on the enzyme lipoxygenase or reductions of the synthesis of leukotriene B470 or intercellular adhesion molecule 1 (ICAM-1).68
Conclusion
Curcumin nanoparticles were obtained using PVP as an encapsulant via the solid dispersion method. Spectroscopic evidence was obtained, showing that hydrogen bonds between these compounds played an important role in particle formation. Nanoparticles were colloidally stable in water, resulting in an increase in the water affinity of curcumin. Paw edema and myeloperoxidase activity were reduced in the animals treated with curcumin-loaded nanoparticles at lower doses than the case of free curcumin; this indicated an inhibition of vascular permeability and a reduction of leukocyte migration to the site of inflammation. Tissue analyses showed a significant reduction in rolling and adherent leukocytes. Encapsulated curcumin was more efficient than free curcumin as an inhibitor of the acute inflammatory process.
Conflicts of interest
There are no conflicts of interest to declare.
Acknowledgements
The authors thank CNPq, CAPES, and Fundação Araucária for their support.
References
- A. C. da Silva, P. D. de F. Santos, N. C. Palazzi, F. V. Leimann, R. H. B. Fuchs, L. Bracht and O. H. Gonçalves, Food Funct., 2017, 8, 1851–1858 Search PubMed.
- A.-L. Cheng, C.-H. Hsu, J.-K. Lin, M.-M. Hsu, Y.-F. Ho, T.-S. Shen, J.-Y. Ko, J.-T. Lin, B.-R. Lin, M.-S. Wu, H.-S. Yu, S.-H. Jee, G.-S. Chen, T.-M. Chen, C.-A. Chen, M.-K. Lai, Y.-S. Pu, M.-H. Pan, Y.-J. Wang, C.-C. Tsai and C.-Y. Hsieh, Anticancer Res., 2001, 21, 2895–2900 CAS.
- A. Siviero, E. Gallo, V. Maggini, L. Gori, A. Mugelli, F. Firenzuoli and A. Vannacci, J. Herb. Med., 2015, 5, 57–70 CrossRef.
- S. Jaiswal, V. Gupta, N. Siddiqi and B. Sharma, Cell. Mol. Biol., 2017, 63, 12–17 CAS.
- A. J. Akinyemi, G. Oboh, O. Ogunsuyi, A. O. Abolaji and A. Udofia, Metab. Brain Dis., 2017, 1–7 Search PubMed.
- A. B. Kunnumakkara, D. Bordoloi, C. Harsha, K. Banik, S. C. Gupta and B. B. Aggarwal, Clin. Sci., 2017, 131, 1781–1799 CrossRef CAS PubMed.
- X.-P. Wang, Q.-X. Wang, H.-P. Lin and N. Chang, Food Funct., 2017, 1, 3319–3326 Search PubMed.
- H. Zhang, Y. Zhu, X. Sun, X. He, M. Wang, Z. Wang, Q. Wang, R. Zhu and S. Wang, J. Biomed. Nanotechnol., 2016, 12, 2051–2062 CrossRef CAS.
- L. Lv, K. Qiu, X. Yu, C. Chen, F. Qin, Y. Shi, J. Ou, T. Zhang, H. Zhu, J. Wu, C. Liu and G. Li, J. Biomed. Nanotechnol., 2016, 12, 973–985 CrossRef CAS PubMed.
- H. Xu, X. Li, H. Kong, X. Zeng, Y. Jin, X. Qi, H. Wang and W. Xie, J. Biomed. Nanotechnol., 2016, 12, 1699–1707 CrossRef CAS.
- X. Q. Ding, T. T. Gu, W. Wang, L. Song, T. Y. Chen, Q. C. Xue, F. Zhou, J. M. Li and L. D. Kong, Mol. Nutr. Food Res., 2015, 59, 2355–2370 CAS.
- L. Tian, K. Zeng, W. Shao, B. B. Yang, I. G. Fantus, J. Weng and T. Jin, J. Nutr., 2015, 145, 2300–2307 CrossRef CAS PubMed.
- G. Naijil, T. R. Anju, S. Jayanarayanan and C. S. Paulose, Nutr. Res., 2015, 35, 823–833 CrossRef CAS PubMed.
- J. W. Daily, M. Yang and S. Park, J. Med. Food, 2016, 19, 717–729 CrossRef CAS PubMed.
- W. Shang, L. J. Zhao, X. L. Dong, Z. M. Zhao, J. Li, B. B. Zhang and H. Cai, Mol. Med. Rep., 2016, 14, 3620–3626 CrossRef CAS PubMed.
- N. Nonose, J. A. Pereira, P. R. M. Machado, M. R. Rodrigues, D. T. Sato and C. A. R. Martinez, Acta Cir. Bras., 2014, 29, 727–734 CrossRef PubMed.
- A. L. Clutterbuck, D. Allaway, P. Harris and A. Mobasheri, F1000Res, 2013, 2, 147 Search PubMed.
- B. Rocha, O. Gonçalves, F. Leimann, E. Rebecca, R. Silva-Buzanello, L. Filho, P. Araújo, R. Cuman and C. Bersani-Amado, Adv. Med. Plant Res., 2014, 2, 62–73 Search PubMed.
- M. S. Baliga, N. Joseph, M. V. Venkataranganna, A. Saxena, V. Ponemone and R. Fayad, Food Funct., 2012, 3, 1109 CAS.
- D. Mesquita, J. A. P. Araújo, T. T. T. Catelan, A. W. S. de Souza, N. da Silva and L. E. C. Andrade, Sind. Reumatol., 2008, 66–81 Search PubMed.
- C. N. Serhan, S. D. Brain, C. D. Buckley, D. W. Gilroy, C. Haslett, L. A. J. O'Neill, M. Perretti, A. G. Rossi and J. L. Wallace, FASEB J., 2007, 21, 325–332 CrossRef CAS PubMed.
- H. A. S. Favacho, B. R. Oliveira, K. C. Santos, B. J. L. Medeiros, P. J. C. Sousa, F. F. Perazzo and J. C. T. Carvalho, Braz. J. Pharmacogn., 2011, 21, 105–114 CrossRef CAS.
- V. Fattori, F. A. Amaral and W. A. Verri, Pharmacol. Res., 2016, 112, 84–98 CrossRef CAS PubMed.
- R. E. Uthayashanker and M. R. Heuertz, J. Pharmacogn. Phyther., 2015, 7, 183–188 CrossRef.
- C. Y. Liu, Y. J. Chiu, C. L. Kuo, T. M. Chien, L. Y. Wu and W. H. Peng, Drug Dev. Res., 2015, 76, 176–184 CrossRef CAS PubMed.
- P. Gris, J. Gauthier, P. Cheng, D. G. Gibson, D. Gris, O. Laur, J. Pierson, S. Wentworth, A. G. Nackley, W. Maixner and L. Diatchenko, Mol. Pain, 2010, 6, 33 CrossRef PubMed.
- C. Bogdan, Nat. Immunol., 2001, 2, 907–916 CrossRef CAS PubMed.
- M. Batlouni, Arq. Bras. Cardiol., 2010, 94, 556–563 CrossRef PubMed.
- J. Shao, D. Zheng, Z. Jiang, H. Xu, Y. Hu, X. Li and X. Lu, Acta Biochim. Biophys. Sin., 2011, 43, 267–274 CrossRef CAS PubMed.
- L. Zhongfa, M. Chiu, J. Wang, W. Chen, W. Yen, P. Fan-Havard, L. D. Yee and K. K. Chan, Cancer Chemother. Pharmacol., 2012, 69, 679–689 CrossRef PubMed.
- A. N. Allam, I. A. Komeil, M. A. Fouda and O. Y. Abdallah, Int. J. Pharm., 2015, 489, 117–123 CrossRef CAS PubMed.
- M. Castillo, E. López-Tobar, S. Sanchez-Cortes, G. Flores and G. Blanch, Vib. Spectrosc., 2015, 81, 106–111 CrossRef.
- S. P. Facchi, D. B. Scariot, P. V. A. Bueno, P. R. Souza, L. C. Figueiredo, H. D. M. Follmann, C. S. Nunes, J. P. Monteiro, E. G. Bonafé, C. V. Nakamura, E. C. Muniz and A. F. Martins, Int. J. Biol. Macromol., 2016, 87, 237–245 CrossRef CAS PubMed.
- T. Esatbeyoglu, K. Ulbrich, C. Rehberg, S. Rohn and G. Rimbach, Food Funct., 2015, 6, 887–893 CAS.
- J.-R. Peng and Z.-Y. Qian, Nanomedicine, 2014, 9, 747–750 CrossRef CAS PubMed.
- S. Manju and K. Sreenivasan, J. Pharm. Sci., 2011, 100, 504–511 CrossRef CAS PubMed.
- V. P. Torchilin, T. S. Levchenko, K. R. Whiteman, A. A. Yaroslavov, A. M. Tsatsakis, A. K. Rizos, E. V. Michailova and M. I. Shtilman, Biomaterials, 2001, 22, 3035–3044 CrossRef CAS PubMed.
- Y. Cui, M. Zhang, F. Zeng, H. Jin, Q. Xu and Y. Huang, ACS Appl. Mater. Interfaces, 2016, 8, 32159–32169 CAS.
- A. P. Ranjan, A. Mukerjee, A. Gdowski, L. Helson, A. Bouchard, M. Majeed and J. K. Vishwanatha, J. Biomed. Nanotechnol., 2016, 12, 679–688 CrossRef CAS PubMed.
- K. Huynh and K. Chen, Environ. Sci. Technol., 2011, 45, 5564–5571 CrossRef CAS PubMed.
- E. Karavas, M. Georgarakis, A. Docoslis and D. Bikiaris, Int. J. Pharm., 2007, 340, 76–83 CrossRef CAS PubMed.
- F. L. Yen, T. H. Wu, C. W. Tzeng, L. T. Lin and C. C. Lin, J. Agric. Food Chem., 2010, 58, 7376–7382 CrossRef CAS PubMed.
- V. A. Hackley and J. D. Clogston, NIST Spec. Publ. 1200-6, 2011, 35–52 CAS.
- E. Karavas, G. Ktistis, A. Xenakis and E. Georgarakis, Eur. J. Pharm. Biopharm., 2006, 63, 103–114 CrossRef CAS PubMed.
- C. Winter, E. Risley and G. Nuss, Proc. Soc. Exp. Biol. Med., 1962, 111, 544–547 CrossRef CAS PubMed.
- D. Bani, E. Masini, M. G. Bello, M. Bigazzi and T. B. Sacchi, Am. J. Pathol., 1998, 152, 1367–1376 CAS.
- W. Khan and V. Rathod, Chem. Eng. Process. Process Intensif., 2014, 80, 1–10 CrossRef CAS.
- M. Yallapu, B. Gupta, M. Jaggi and S. Chauhan, J. Colloid Interface Sci., 2010, 351, 19–29 CrossRef CAS PubMed.
- E. I. Paramera, S. J. Konteles and V. T. Karathanos, Food Chem., 2011, 125, 892–902 CrossRef CAS.
- R. A. Silva-Buzanello, M. F. De Souza, D. A. De Oliveira, E. Bona, F. V. Leimann, L. C. Filho, P. Henrique, H. De Araújo, S. Regina, S. Ferreira and O. H. Gonçalves, Polímeros, 2016, 26, 207–214 CrossRef.
- M. K. Jeengar, S. Shrivastava, K. Nair, S. R. Singareddy, U. K. Putcha, M. V. N. K. Talluri, V. G. M. Naidu and R. Sistla, Inflammation, 2014, 37, 2139–2155 CrossRef CAS PubMed.
- C. S. Mangolim, C. Moriwaki, A. C. Nogueira, F. Sato, M. L. Baesso, A. M. Neto and G. Matioli, Food Chem., 2014, 153, 361–370 CrossRef CAS PubMed.
- J. R. Villalobos-Hernández and C. C. Müller-Goymann, Eur. J. Pharm. Biopharm., 2005, 60, 113–122 CrossRef PubMed.
- M. C. Silva, T. B. Fideles and M. V. L. Fook, Rev. Eletrônica Mater. e Process., 2015, 1, 21–28 Search PubMed.
- M. Valero, B. Pérez-Revuelta and L. Rodríguez, Int. J. Pharm., 2003, 253, 97–110 CrossRef CAS PubMed.
- P. Mura, N. Zerrouk, N. Menninia, F. Maestrellia and C. Chemtob, Eur. J. Pharm. Sci., 2003, 19, 67–75 CrossRef CAS PubMed.
- S. Sethia and E. Squillante, Int. J. Pharm., 2004, 272, 1–10 CrossRef CAS PubMed.
- F. Frizon, J. Eloy, C. Donaduzzi, M. Mitsui and J. Marchetti, Powder Technol., 2013, 235, 523–539 CrossRef.
- R. M. Martinez, D. T. Longhi-Balbinot, A. C. Zarpelon, L. Staurengo-Ferrari, M. M. Baracat, S. R. Georgetti, R. C. Sassonia, W. A. Verri and R. Casagrande, Arch. Pharmacal Res., 2015, 38, 494–504 CrossRef CAS PubMed.
- T. V. De Brito, R. D. S. Prudêncio, A. B. Sales, F. D. C. Vieira Júnior, S. J. N. Candeira, Á. X. Franco, K. S. Aragão, R. D. A. Ribeiro, M. H. L. Ponte De Souza, L. D. S. Chaves, A. L. P. Freitas, J. V. R. Medeiros and A. L. Dos Reis Barbosa, J. Pharm. Pharmacol., 2013, 65, 724–733 CrossRef PubMed.
- M. M. Sitônio, C. H. R. Carvalho, I. A. Campos, J. B. N. F. Silva, M. C. A. Lima, A. J. S. Góes, M. B. S. Maia, P. J. R. Neto and T. G. Silva, Inflammation Res., 2013, 62, 107–113 CrossRef PubMed.
- J. M. Dias, T. V. de Brito, D. de Aguiar Magalhães, P. W. da Silva Santos, J. A. Batista, E. G. do Nascimento Dias, H. de Barros Fernandes, S. R. B. Damasceno, R. O. Silva, K. S. Aragão, M. H. L. P. Souza, J. V. R. Medeiros and A. L. R. Barbosa, Inflammation, 2014, 37, 1826–1836 CrossRef CAS PubMed.
- A. Goel, C. R. Boland and D. P. Chauhan, Cancer Lett., 2001, 172, 111–118 CrossRef CAS PubMed.
- S. Lev-Ari, L. Strier, D. Kazanov, O. Elkayam, D. Lichtenberg, D. Caspi and N. Arber, Rheumatology, 2006, 45, 171–177 CrossRef CAS PubMed.
- B. B. Aggarwal and K. B. Harikumar, Int. J. Biochem. Cell Biol., 2009, 41, 40–59 CrossRef CAS PubMed.
- S. Singh, K. W. Basniwal and H. S. Buttar, J. Agric. Food Chem., 2011, 59, 2056–2061 CrossRef PubMed.
- S. J. Klebanoff, J. Leukocyte Biol., 2005, 77, 598–625 CrossRef CAS PubMed.
- D. Thong-Ngam, S. Choochuai, S. Patumraj, M. Chayanupatkul and N. Klaikeaw, World J. Gastroenterol., 2012, 18, 1479–1484 CrossRef CAS PubMed.
- V. Fattori, F. A. Amaral and W. A. Verri, Pharmacol. Res., 2016, 112, 84–98 CrossRef CAS PubMed.
-
M.-T. Huang, F. M. Robertson, T. Lysz, T. Ferraro, Z. Y. Wang, C. A. Georgiadis, J. D. Laskin and A. H. Conney, in Phenolic Compounds in Food and Their Effects on Health II: Antioxidants and Cancer Prevention, ed. M. T. Huang, C. T. Ho and C. Y. Lee, American Chemical Society, 1992, vol. 27, pp. 338–349 Search PubMed.
|
This journal is © The Royal Society of Chemistry 2018 |
Click here to see how this site uses Cookies. View our privacy policy here.