DOI:
10.1039/C7FO01082F
(Paper)
Food Funct., 2018,
9, 417-425
The mechanism of reduced IgG/IgE-binding of β-lactoglobulin by pulsed electric field pretreatment combined with glycation revealed by ECD/FTICR-MS†
Received
19th July 2017
, Accepted 2nd November 2017
First published on 16th November 2017
Abstract
Bovine β-lactoglobulin (β-Lg) is a major allergen existing in milk and causes about 90% of IgE-mediated cow's milk allergies. Previous studies showed that pulsed electric field (PEF) treatment could partially unfold the protein, which may contribute to the improvement of protein glycation. In this study, the effect of PEF pretreatment combined with glycation on the IgG/IgE-binding ability and the structure of β-Lg was investigated. The result showed that PEF pretreatment combined with glycation significantly reduced the IgG and IgE binding abilities, which was attributed to the changes of secondary and tertiary structure and the increase in glycation sites and degree of substitution per peptide (DSP) value determined by electron capture dissociation Fourier transform ion cyclotron resonance mass spectrometry (ECD/FTICR-MS). Unexpectedly, glycation sites (K47, K91 and K135) added by two mannose molecules were identified in glycated β-Lg with PEF pretreatment. Moreover, the results indicated that PEF pretreatment at 25 kV cm−1 for 60 μs promoted the reduction of IgG/IgE-binding capacity by increasing the glycation degree of β-Lg, whereas single PEF treatment under the same conditions markedly enhanced the IgG/IgE-binding ability by partially unfolding the structure of β-Lg. The results suggested that ECD/FTICR-MS could help us to understand the mechanism of reduction in the IgG/IgE-binding of β-Lg by structural characterization at the molecular level. Therefore, PEF pretreatment combined with glycation may provide an alternative method for β-Lg desensitization.
1. Introduction
Cow's milk allergy (CMA), the most common food allergy in early childhood, affects between 2% and 3% of infants in developed countries.1 This limits the application of milk in the food industry although milk is an excellent nutritional and functional food resource. Beta-lactoglobulin (β-Lg), causes almost 90% of IgE-mediated CMA, and is a major allergen (constituting about 50% of whey proteins) in cow's milk.2,3 It is an 18 kDa globular protein with a sequence of 162 amino acids, in which two disulfide bonds (Cys66–Cys160 and Cys106–Cys119) stabilize a so-called-barrel (or calyx) structure that is formed by nine anti-parallel-sheets.4,5 To date, the linear and conformational epitopes (including IgG and IgE types) of β-Lg have been mainly identified.6,7 Depending on different principles, numerous processing methods, such as heating,8 high hydrostatic pressure (HHP),9 ultrasonication,10 dynamic high pressure microfluidization (DHPM),11 glycation,12 hydrolysis13 and genetic modification,14 have been conducted to alter the allergenicity of β-Lg. However, some limitations impede their practical application and a single method could not sufficiently reduce the allergenicity to a satisfactory level. Some methods, such as HHP and DHPM, could even increase the allergenicity of β-Lg.
Glycation, the early step of the Maillard reaction occurring between proteins and reducing sugars, is one of the most common and essential chemical reactions during food processing and storage. It has been considered as a relatively safe method for modifying the functional properties of proteins, such as emulsibility, antioxidant activity and allergenicity.15,16 A previous work indicated that glycation could decrease the IgE binding ability of β-Lg by masking the IgE epitopes through covalent binding with reducing sugars.16 However, satisfactory hypoallergenicity could not be achieved by single glycation due to the insufficient glycation extent.
Pulsed electric field (PEF) has been considered as an emerging and successful non-thermal technology for food processing and preservation. It processes liquid foods for a few milliseconds by using an external electrical field of high intensity (up to 40 kV cm−1).17 Compared with conventional thermal processing, it could maintain the food's quality attributes, such as sensory and nutritional properties, that may be degraded during conventional thermal processing.18 So far, PEF has been widely applied to enzyme inactivation, intracellular component extraction, pesticide degradation and food sterilization.17,19 Previous research showed that PEF could partially unfold the structure of ovalbumin (OVA) and bovine serum albumin (BSA).20 The partial unfolding of the protein structure may allow more efficient modification of the protein by glycation. Therefore, PEF pretreatment combined with glycation may provide a new method for β-Lg desensitization. However, little work has been conducted to investigate the influence of PEF pretreatment coupled with glycation on the allergenicity of β-Lg. Moreover, the mechanism of the reduction in the allergenicity of β-Lg by glycation at the molecular level remains ambiguous.
Fourier transform ion cyclotron resonance mass spectroscopy (FTICR-MS) is a high-resolution technique used to determine the composition of molecules based on accurate mass and study large macromolecules such as proteins with multiple charges, which can be produced by electrospray ionization (ESI).21 Electron capture dissociation (ECD) could preserve the specific modifications due to the low energy used, and thus is quietly useful in analyzing protein modifications, such as phosphorylation, glycation and sulfation.22 Therefore, to characterize the accurate structure of glycated β-Lg at the molecular level, ECD/FTICR-MS was employed to analyze the glycation sites and extent.
The purpose of this study was therefore to carry out a detailed interpretation of the relationship between the IgG/IgE-binding ability and structural changes of β-Lg by PEF pretreatment combined with glycation. In this work, β-Lg was pretreated with PEF followed by dry-state glycation with mannose under non-denatured conditions. The IgG and IgE binding abilities were evaluated by indirect competitive ELISA. The glycation sites and extent of glycated β-Lg were identified by ECD FTICR-MS. The secondary and tertiary structures were determined by circular dichroism (CD), ultraviolet (UV) and fluorescence spectrometry. The results of this work enhance our understanding of the mechanism of the decreased IgG/IgE-binding ability of β-Lg by PEF pretreatment combined with glycation, as well as indicating that PEF pretreatment combined with glycation may be an alternative method for β-Lg desensitization.
2. Materials and methods
2.1. Materials
Mannose, β-Lg, goat anti-rabbit IgG-HRP conjugate and goat anti-human IgE-HRP conjugate, Freund's complete and incomplete adjuvants, 3,3′,5,5′-tetramethylbenzidine (TMB) and 1-anilinonaphthalene-8-sulfonate (ANS) were obtained from Sigma-Aldrich (St Louis, MO, USA). Milli-Q water was used as a solvent in solution. All chemicals used were of analytical grade.
2.2. Preparation of β-Lg samples
One gram of β-Lg was dissolved in 100 mL of 0.01 mol L−1 phosphate buffer (pH 8.0). To reduce the thermal effect during processing, the electrical conductivity of the solution was less than 0.1 S m−1. Then it was treated at 25 kV cm−1 for 60 μs by using a bench scale pulse generator system with a unipolar square wave (designed by Tsinghua University, Beijing, China), in which there were two co-field flow treatment chambers (0.05 mL) with a gap distance and inner diameter of 0.4 cm. The temperature of the sample was kept below 20 °C by using a cooling coil submerged in an ice-water bath. After PEF pretreatment, the mannose was added into the sample with the mannose to β-Lg mass ratio of 2
:
1. The blended solution was lyophilized, and then incubated at 55 °C and 0.79% relative humidity for 4 h. After stopping the reaction by placing into the freezer, the remaining mannose was removed by using ultracentrifugal filters (molecular weight cut off 3000 Da). Finally, the sample was adjusted to 1 mg mL−1 and stored at 4 °C for less than 48 h. Native β-Lg was used as the blank control and was named β-Lg-N. The sample without mannose heated under the same glycation conditions was used as another control and was named β-Lg-H. The β-Lg treated at 25 kV cm−1 for 60 μs was named β-Lg-P. Native β-Lg glycated with mannose was named β-Lg-M and β-Lg pretreated at 25 kV cm−1 for 60 μs glycated with mannose was named β-Lg-PM. The PEF treatment time (t) was calculated as follows:23 | 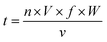 | (1) |
where n is the number of treatment chambers, V is the volume of a chamber (mL), f is the pulse repetition rate (pulses per second, Hz), W is the pulse width (μs) and v is the flow rate (mL s−1). In this study, f was 30 Hz and W was 5 μs. The flow rate was 0.25 mL s−1.
2.3. Preparation of antisera against β-Lg
Polyclonal antisera against β-Lg were produced from six Japanese male rabbits (3-month-old, about 2.0 kg), which were immunized via ear venous injection with 1 mg of native β-Lg emulsified with an equal volume of Freund's complete adjuvant for the first time, and then were re-stimulated four times at one week intervals by injection with the same amount of β-Lg emulsified with an equal volume of Freund's incomplete adjuvant. The antiserum titer was monitored by performing indirect enzyme-linked immunosorbent assay (ELISA). When the titer value was over 10
000, the rabbits were bled to obtain the antisera, which were centrifuged (4000g, 10 min, 25 °C) and stored at −80 °C until use.
Human sera were collected from eight patients with a documented clinical history and a positive skin prick test to cow's milk (details are shown in Table 1). The specific IgE levels varied from 9.58 kUA L−1 to 96.6 kUA L−1. All serum samples were provided by PlasmaLab International (Everett, WA) and stored at −80 °C. They were put together to a sera pool and the titer was monitored by indirect ELISA before use. The IgE level of the mixed sera pool of CMA patients was 53.7 kUA L−1. All experiments involving animals and human subjects were performed in compliance with the NIH guidelines and was approved by the Animal Care and Use Committee of Nanchang University [Permission No. SCXK(Gan)-2014-0005] and the Internal Ethical Committee of First Affiliated Hospital of Nanchang University. All participants provided written informed consent.
Table 1 Information of CMA patients
No. |
Gender |
Age (year) |
Clinical symptoms |
Other allergies |
IgE (kUA L−1) |
AS, asthma; HI, hives; EC, eczema; RH, rhinitis; NW, nose wheezing; CW, chest wheezing. |
1 |
Male |
5 |
AS, EC |
Egg allergy |
20.3 |
2 |
Male |
1 |
AS |
Animal allergy |
13.8 |
3 |
Female |
20 |
HI, EC, RH |
Animal allergy |
96.1 |
4 |
Female |
3 |
AS, HI |
Wheat allergy |
94.8 |
5 |
Male |
27 |
HI, NW |
Most food allergy |
9.58 |
6 |
Female |
47 |
HI, CW |
Most food allergy |
93.1 |
7 |
Male |
34 |
EC, RH |
Honey allergy |
24.7 |
8 |
Female |
2 |
AS, NW |
None |
96.6 |
2.4. Indirect competitive ELISA
According to the method of Yang et al.,24 the IgG and IgE binding abilities of β-Lg were estimated by indirect competitive ELISA with rabbit polyclonal antisera and CAM patients’ antisera, respectively. Firstly, the 96-well microplate was coated overnight at 4 °C with native β-Lg (100 μL per well, 2 μg mL−1). After washing with PBST (0.05% Tween-20 in PBS, pH 7.4), it was blocked by using pig gelatin (300 μL per well, 10 mg mL−1) for 1 h at 37 °C. Subsequently, 50 μL of either standard β-Lg (0.25, 1, 4, 16 and 32 μg mL−1) or diluted β-Lg samples and 50 μL of pooled rabbit antisera (diluted to1
:
12
800) or pooled CAM patients’ antisera (diluted to 1
:
8) were added and incubated for 30 min at 37 °C. After washing, 100 μL of goat anti-rabbit IgG-HRP conjugate or goat anti-human IgE-HRP conjugate (diluted to 1
:
5000) was added and incubated for 30 min at 37 °C. Then it was colored with 100 μL of TMB solution by incubation for 15 min at 37 °C. Finally, the reaction was stopped by adding 50 μL of 2 mol L−1 sulfuric acid and the absorbance was measured at 450 nm using an HF2000 microplate reader (Huaan Magnech, Beijing, China). The IgG/IgE-binding ability was calculated from a standard curve of β-Lg with a linear logarithmic correlation in the range of 0.25–32 μg mL−1. All analyses were carried out in triplicate, and the averaged values were finally converted to concentration equivalents in mg mL−1 by multiplying with the dilution factor.
2.5. ECD/FTICR-MS
Before MS, β-Lg samples were digested by trypsin. Firstly, 10 μg of β-Lg samples were added into the Tris-HCl buffer (pH 7.5, 1 mol L−1) containing 6 mol L−1 guanidine, following by adding 0.25 μL of 0.5 mol L−1 DTT with incubation for 30 min at 37 °C. Then 0.5 μL of 0.5 mol L−1 iodoacetamide was added and incubated in the dark for 30 min at room temperature. After dilution (10-fold), 2 μL of 1 mg mL−1 trypsin was added and incubated overnight at 37 °C. Finally, 2 μL of 10% formic acid (FA) was added into the solution to stop digestion. After digestion, 50 μL of sample solution was injected into a BioSuite 3 μm C18 2.1 × 50 mm column (Waters, Milford, MA) for HPLC, of which the flow rate was 0.2 mL min−1. The mobile phase consisted of solvent A (0.1% FA in H2O) and solvent B (100% acetonitrile containing 0.1% FA). After desalting for 5 min with 5% B, the peptides were eluted with a gradient of 5–50% B for 40 min, 60–80% B for 3 min, and 80% B for 2 min. The effluent was infused into the Bruker 7T-SolariX FTICR-MS (Bruker Daltonics, Billerica, MA) and the peptides were fragmented by ECD, using a pulse bias of 1.0 and a pulsed length of 0.1 s, with an ionization energy of 1.0 eV. The peptides were identified according to MS data, which were processed using DataAnalysis, BioTools and SequenceEditor (Bruker Daltonics, Billerica, MA).
The glycation extent of each peptide was determined by the DSP (degree of substitution per peptide) value, which was calculated as follows:25
| 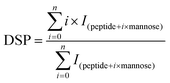 | (2) |
where
I is the sum of the intensities of the glycated peptides and
i is the number of mannose units attached to the peptide in each glycated form.
2.6. Far-UV CD spectroscopy
A MOS-450 spectropolarimeter (Bio-Logic SAS, Claix, French) was used to measure far-UV (190–240 nm) CD spectra, which were obtained at a concentration of 0.1 mg mL−1 in 0.05 mol L−1 PBS with pH 7.4 at room temperature. The bandwidth, step resolution and path length were all 1.0 mm, with a scan speed of 100 nm min−1. The data were shown as the mean residue ellipticity ([θ], deg cm2 dmol−1), and the contents of secondary structures were calculated using DichroWeb (http://dichroweb.cryst.bbk.ac.uk/html/home.shtml).
2.7. UV absorption spectroscopy
UV absorption spectra were obtained using a UV-2910 spectrophotometer (Hitachi, Tokyo, Japan). The β-Lg samples (0.5 mg mL−1) were scanned from 240 nm to 320 nm at a scan speed of 800 nm min−1.
2.8. Surface hydrophobicity
According to the method of Matulis and Lovrien,26 ANS was used as a probe to measure the surface hydrophobicity of β-Lg samples. The ANS solution (0.008 mol L−1) was added into the samples (0.25 mg mL−1, 0.5 mg mL−1 and 1.0 mg mL−1) with a volume ratio of 200
:
1. The samples were scanned from 400 nm to 600 nm at a speed of 1200 nm min−1, with an excitation at 390 nm. The surface hydrophobicity of the samples was defined as the initial slope of the fluorescence intensity versus the protein concentration plot, which was calculated by linear regression analysis.
2.9. Statistical analysis
All the experiments were carried out in triplicate and the results were presented as the mean value ± standard deviation (SD). The analysis was performed using SPSS 19.0 (SPSS Inc., Chicago, IL) and Origin-Pro 2017 (OriginLab Corp., Northampton, MA).
3. Results
3.1. IgG and IgE binding ability analysis
Fig. 1 shows the changes in the IgG and IgE binding of β-Lg samples treated under different conditions. As shown in Fig. 1A, dry-heated β-Lg (β-Lg-H) exhibited almost the same IgG binding ability as native β-Lg (β-Lg-N), whereas the PEF treated sample (β-Lg-P) had much higher (increased by about 70%) IgG binding ability than native β-Lg. Nevertheless, the glycated β-Lg without PEF pretreatment (β-Lg-M) showed around half of the IgG binding ability of native β-Lg while the glycated sample with PEF pretreatment (β-Lg-PM) displayed only about 15% of IgG-binding of native β-Lg. Interestingly, a similar trend was observed in Fig. 1B. The IgE binding ability of the dry-heated sample (β-Lg-H) was equivalent to that of the native β-Lg (1.05 mg mL−1), whereas it increased by approximately 50% after PEF treatment at 25 kV cm−1 for 60 μs. However, it reduced by nearly 35% after glycation with mannose and further decreased to almost 25% after glycation with PEF pretreatment.
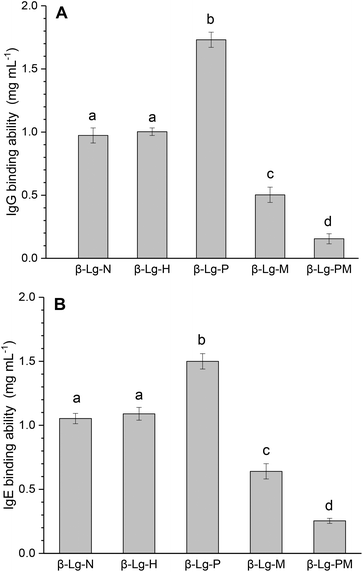 |
| Fig. 1 IgG (A) and IgE (B) binding abilities of β-Lg samples were determined by inhibition competitive ELISA. Letters (a–d) in the bars mean significantly different (p < 0.05). | |
3.2. Glycation site determination
The peptide mapping of native β-Lg by ECD/FTICR-MS is displayed in Table S1,† which showed that the sequence coverage was 98.1%. Theoretically, if a peptide is glycated with one mannose molecule, the mass will increase 162.0528 Da, reflecting in an m/z shift of 81.0264, 54.0176, 40.5132 and 32.4106, respectively, at 2, 3, 4 and 5 charges, accordingly. Likewise, glycation with two and three mannose molecules will double and triple the m/z shift, respectively. As shown in Fig. 2A, the m/z of the unglycated peptide 125–138 was 545.92953+ while the corresponding m/z of its glycated peptides were 599.94683+ and 653.96393+ with an m/z shift of 54.0173 and 108.0344, respectively, indicating that it had mono-glycated and dual-glycated peptides. Fig. 2B exhibits the ECD MS/MS spectrum of the mono-glycated peptide with an m/z of 599.94683+, which showed a series of c and z ions. The glycation site, K135, was determined by the mass difference between c10 and c11 ions, or between z3 and z5, which was the combined mass of lysine and mannose. However, according to the ECD MS/MS spectrum of this peptide with an m/z of 653.96393+ (shown in Fig. 2C), K135 reacted with two mannose molecules. It indicated that one glycation site was added by two mannose molecules. Interestingly, a similar phenomenon was observed in peptides 84–101 (shown in Fig. 3). As shown in Fig. 3A, there were three glycated types in peptides 84–101, including the mono-glycated, dual-glycated and trinal-glycated with m/z values of 564.05134+, 604.56454+ and 645.07764+, respectively. The ECD MS/MS spectra in Fig. 3B, Fig. 3C and D, respectively, determined the glycated sites K91, K91 & K100 and K100 & K91 with double mannose.
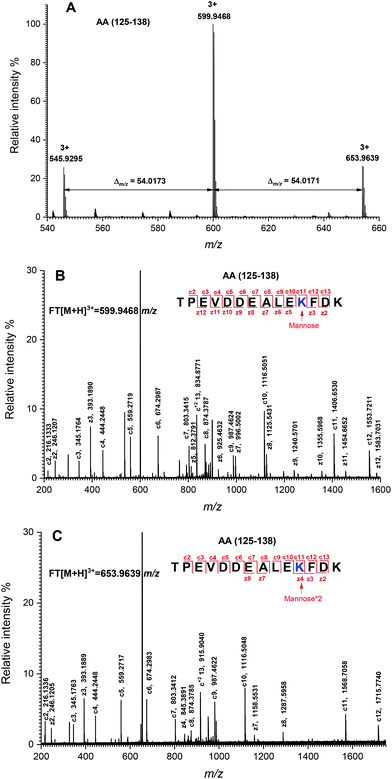 |
| Fig. 2 Determination of glycation sites in glycated peptides 125–138. (A) Mass spectrum of peptides 125–138 with one glycation site. (B) ECD MS/MS spectrum of the mono-glycated peptide with an m/z of 599.54683+. (C) ECD MS/MS spectrum of the dual-glycated peptide with an m/z of 653.96393+. The sequence of each peptide is shown in the top of the figure. The mass differences are indicated with numbers and arrows. The determined glycation sites are highlighted by blue color and indicated by an arrow with a symbol, in which “mannose” and “mannose*2” mean that the glycation site is added by one and two mannose molecules, respectively. The c and z ions are indicated by the numbers and lines. | |
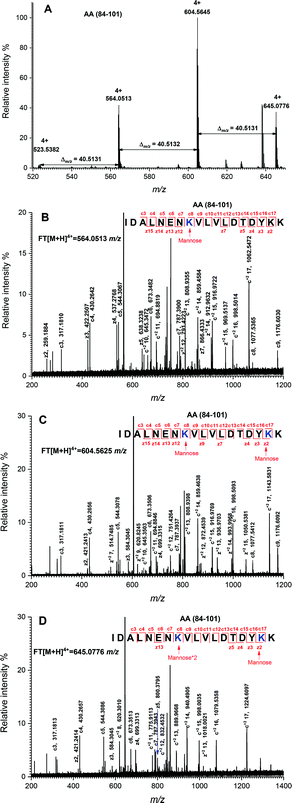 |
| Fig. 3 Determination of glycation sites in glycated peptides 84–101. (A) Mass spectrum of peptides 84–101 with multiple glycation sites. (B) ECD MS/MS spectrum of the mono-glycated peptide with an m/z of 564.05134+. (C) ECD MS/MS spectrum of the dual-glycated peptide with an m/z of 604.56454+. (D) ECD MS/MS spectrum of the trinal-glycated peptide with an m/z of 645.07764+. The sequence of each peptide is shown in the top of the figure. The mass differences are indicated with numbers and arrows. The determined glycation sites are highlighted by blue color and indicated by an arrow with a symbol, in which “mannose” and “mannose*2” mean that the glycation site is added by one and two mannose molecules, respectively. The c and z ions are indicated by the numbers and lines. | |
Likewise, other glycation sites, including L1, K8, K14, K47, K60, K69, K70, K75, K77, K83, K138 and K141, were determined by ECD MS/MS (as shown in Fig. S1†). Table 2 displays the amount of glycation sites of glycated β-Lg with and without PEF pretreatment. The glycated β-Lg without PEF pretreatment had twelve glycation sites, including K47, K60, K69, K70, K75, K77, K83, K91, K100, K135, K138 and K141. However, three additional glycation sites (L1, K8 and K14) and three glycation sites (K47, K91 and K135) added with double mannose molecules were found in glycated β-Lg with PEF pretreatment.
Table 2 The glycated peptides of β-Lg by glycation with and without PEF pretreatment
Peptide location |
m/z (glycated) |
Δm (ppm) |
Sequencea |
Glycation siteb |
C* means the alkylated cystine residue by iodoacetamide.
Double-star (**) indicates the glycation site added by two mannose molecules. The increased glycation sites and double-mannose-added glycations sites in glycated β-Lg with PEF pretreatment are displayed in bold.
|
1–14 |
583.99263+ |
−1.89 |
(−)LIVTQTMKGLDIQK(V) |
L1
|
1–14 |
638.01023+ |
−1.41 |
(−)LIVTQTMKGLDIQK(V) |
L1, K8
|
9–40 |
881.70324+ |
−2.27 |
(K)GLDIQKVAGTWYSLAMAASDISLLDAQSAPLR(V) |
K14
|
41–60 |
825.77463+ |
−0.97 |
(R)VYVEELKPTPEGDLEILLQK(W) |
K47 |
41–60 |
879.79213+ |
−1.02 |
(R)VYVEELKPTPEGDLEILLQK(W) |
**K47
|
41–70 |
774.59065+ |
−3.95 |
(R)VYVEELKPTPEGDLEILLQKWENGEC*AQKK(I) |
K60, K69 |
61–75 |
533.01804+ |
−0.23 |
(K)WENGEC*AQKKIIAEK(T) |
K69, K70 |
71–91 |
569.10985+ |
−1.06 |
(K)IIAEKTKIPAVFKIDALNENK(V) |
K75, K77, K83 |
84–101 |
604.56454+ |
−1.16 |
(K)IDALNENKVLVLDTDYKK(Y) |
K91, K100 |
84–101 |
645.07764+ |
−1.24 |
(K)IDALNENKVLVLDTDYKK(Y) |
**K91, K100 |
125–138 |
599.94683+ |
−1.00 |
(R)TPEVDDEALEKFDK(A) |
K135 |
125–138 |
653.96393+ |
−1.68 |
(R)TPEVDDEALEKFDK(A) |
**K135
|
125–141 |
568.77894+ |
−1.23 |
(R)TPEVDDEALEKFDKALK(A) |
K135, K138 |
125–141 |
609.58914+ |
−1.15 |
(R)TPEVDDEALEKFDKALK(A) |
**K135, K138 |
139–148 |
437.91813+ |
−3.89 |
(K)ALKALPMHIR(L) |
K141 |
3.3. Glycation extent analysis
The glycation extent of the protein was evaluated by the number of glycation sites and the glycation extent per glycation site. The result in Table 2 shows that PEF pretreatment increased three glycation sites (L1, K8 and K14). However, it is necessary to calculate the DSP to evaluate the glycation extent of the glycated β-Lg samples. The DSP values of all the glycated peptides produced from glycated β-Lg with and without PEF pretreatment are shown in Fig. 4. Based on the DSP value, K91 and K100 of peptides 84–101 were the most reactive mannose glycation sites in native β-Lg with a DSP value of 0.7. Nevertheless, the DSP value further increased to almost 1.0 after PEF pretreatment at 25 kV cm−1 for 60 μs. Fig. 4 displays that the DSP values were significantly increased by PEF pretreatment as a whole. Moreover, some peptides (1–14 and 9–40) were unglycated in the native form. However, they had a high DSP value (≥0.7) in the glycated sample with PEF pretreatment at 25 kV cm−1 for 60 μs.
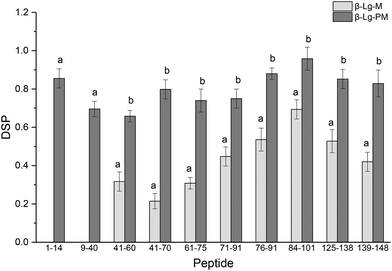 |
| Fig. 4 DSP values of glycated peptides of glycated β-Lg with and without PEF pretreatment. Means with different letters (a and b) in the bars are significantly different (p < 0.05). | |
3.4. Secondary structure analysis
The changes in the secondary structure of β-Lg under different conditions are shown in Table 3. No significant differences (p < 0.05) in the secondary structure were observed after dry-heating at 55 °C for 4 h without mannose. However, after PEF treatment at 25 kV cm−1 for 60 μs, the α-helix content of β-Lg decreased while the β-sheet content increased. Furthermore, after glycation with mannose, the α-helix and β-sheet contents of β-Lg increased from 53.0% (β-Lg) to 60.1% (β-Lg-M) while the β-turn and random coil contents decreased from 47.0% (β-Lg) to 39.9% (β-Lg-M). Moreover, PEF pretreatment induced a further increase of α-helix and β-sheet contents and an additional decrease of β-turn and random coil contents.
Table 3 The content (%) of secondary structures of β-Lg treated under different conditions
Samples |
α-Helix |
β-Sheet |
β-Turn |
Random coil |
Values followed by different letters (a–d) in the same column are significantly different (p < 0.05). |
β-Lg-N |
13.7 ± 0.5a |
39.3 ± 0.3a |
19.1 ± 0.5a |
27.9 ± 0.4a |
β-Lg-H |
13.4 ± 0.2a |
39.8 ± 0.5a |
18.7 ± 0.3a |
28.1 ± 0.7a |
β-Lg-P |
11.0 ± 0.2b |
41.7 ± 0.5b |
19.8 ± 0.2a |
27.5 ± 0.7a |
β-Lg-M |
19.3 ± 0.5c |
40.8 ± 0.8b |
16.1 ± 0.4b |
23.8 ± 0.6a |
β-Lg-PM |
20.8 ± 0.4d |
41.9 ± 0.4b |
15.0 ± 0.7b |
22.3 ± 0.3c |
3.5. Tertiary structure analysis
The changes in the UV absorption spectra of β-Lg samples under different conditions are shown in Fig. 5A. Compared to native β-Lg, the sample dry-heated without mannose had a very slight increase in the maximum UV absorbance at 280 nm, whereas β-Lg with PEF treatment at 25 kV cm−1 for 60 μs had an obvious increase from 0.47 (β-Lg) to 0.51 (β-Lg-P). Moreover, the glycated samples showed much higher maximum UV absorbance at 280 nm than native β-Lg, whereas the glycated β-Lg with PEF pretreated at 25 kV cm−1 for 60 μs had the highest value (0.58) of maximum UV absorbance at 280 nm. Fig. 5B depicts the changes in the surface hydrophobicity of β-Lg treated under different conditions. The surface hydrophobicity of β-Lg dry-heated without mannose was almost the same as native β-Lg. However, it significantly increased after PEF pretreatment at 25 kV cm−1 for 60 μs. When β-Lg was glycated with mannose, the surface hydrophobicity decreased from 1703 (β-Lg-N) to 1489 (β-Lg-M). Moreover, it further decreased to 1250 after glycation with PEF pretreatment.
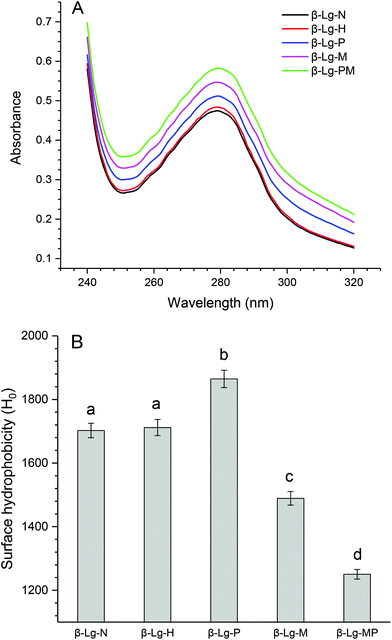 |
| Fig. 5 The changes in the UV absorption (A) and surface hydrophobicity (B) of β-Lg samples. Means with different letters (a and b) in the bars are significantly different (p < 0.05). | |
4. Discussion
Glycation, which extensively occurs during food storage and processing, has been used to reduce the allergenicity of proteins by modifying the allergenic epitopes via a covalent bond between proteins and reducing sugars. However, the reduction extent of allergenicity is insufficient due to the limited glycation degree. Previous research indicated that PEF could make proteins, such as OVA, BSA and lysozyme, partially unfolded,17,20,27 which may be conducive to the improvement of glycation. Therefore, the effect of PEF pretreatment combined with glycation on the IgG/IgE-binding ability and the structure of β-Lg was investigated in this work. The results showed that PEF pretreatment combined with glycation significantly reduced the IgG and IgE binding abilities, which were closely related to the structural changes of β-Lg. We hypothesized that the masking of linear epitopes caused by the modification of the acid amino sequence was the dominant mechanism for the decreased IgG- and IgE-binding ability of β-Lg, and the destruction of conformational epitopes that resulted from structural changes was the subordinate reason for the reduction.
To provide further insight into the mechanism of reduced IgG/IgE-binding ability by PEF pretreatment combined with glycation, ECD/FTICR-MS was applied to characterize the structural changes at the molecular level. As we know, the main glycation sites in proteins are lysine, arginine, and the N-terminus. There are nineteen potential glycation sites in β-Lg, including one N-terminal Leu, fifteen Lys and three Arg. Prior to PEF pretreatment, twelve glycation sites were identified, while the number of glycation sites was increased to fifteen after pretreatment (shown in Fig. 6). The result conforms with the findings from a previous study,28 in which the glycation sites (L1, K8 and K14) were not observed in the glycated native β-Lg. Moreover, the glycation extent of β-Lg was overall improved by PEF pretreatment (shown in Fig. 4). Typically, β-Lg exists as a dimer through non-covalent (such as hydrophobic and electrostatic interactions) in the native state, making some glycation sites hardly modified by mannose.29 PEF treatment partially unfolded the structure of β-Lg by destroying these interactions via continuous electric fields, leading to the exposure of some glycation sites and finally improving the glycation. To date, the linear IgG and IgE epitopes of β-Lg have been identified by different methods.6,7,30–33 It is summarized that the linear IgG and IgE epitopes spread through the whole amino acid sequence of β-Lg. To ensure the universality of IgG and IgE epitopes, in this work, the pooled antisera were obtained from six rabbits and eight CAM patients, of which the patients’ age and allergy symptoms are different (shown in Table 1). The modification of glycation sites resulted in the masking of linear IgG and IgE epitopes via covalent attachment,34 making them unrecognized by IgG and IgE, and finally reducing the IgG and IgE binding abilities of β-Lg. Thus, PEF pretreatment combined with glycation unambiguously decreased the IgG/IgE-binding ability of β-Lg by masking the IgG and IgE epitopes through covalent binding with mannose, and PEF pretreatment promoted the reduction of IgG and IgE binding abilities by improving the glycation degree.
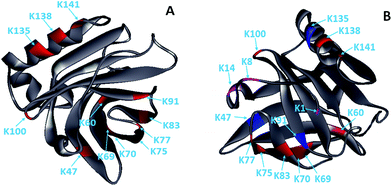 |
| Fig. 6 Solid ribbon diagram of the glycated β-Lg (PDB 1BSQ). (A) The glycated β-Lg without PEF pretreatment. (B) The glycated β-Lg with PEF pretreatment. The glycation sites are colored as follows: grey, framework of β-Lg; red, glycation sites of the native β-Lg; magenta, additional glycation sites of the β-Lg after PEF treated at 25 kV cm−1 for 60 μs; blue, the glycation sites had two mannose molecules. | |
Unexpectedly, three glycation sites (K47, K91 and K135) added by two mannose molecules were observed in the glycated β-Lg after PEF pretreatment. Maillard-type glycation could produce the reactive intermediates,35 which may react with mannose under alkaline conditions. PEF treatment could change the electrostatic environment of protein molecules,27 which may make it conducive to the production of reactive intermediates during the Maillard reaction. The addition of two mannose molecules in one glycation site caused steric hindrance that made IgG and IgE more difficult to recognize their linear epitopes of β-Lg. Therefore, the IgG/IgE-binding capacity of β-Lg was further dramatically reduced by PEF pretreatment combined with glycation.
Furthermore, to comprehensively understand the mechanism of decrease in the IgG and IgE binding of β-Lg by PEF pretreatment combined with glycation, the conventional spectrometry was used to measure the structural changes at the macro level. Glycation could make the conformation of proteins more stable at the expense of β-turn or unordered structures.36 Accordingly, the content of α-helix and β-sheet structures was increased while that of β-turn and unordered structures was decreased. Besides this, glycation could cause exposure of Trp15 and lead to an obvious increase of UV absorbance at 280 nm. Meanwhile, it could mask some hydrophobic groups by amino acid residue modification through the covalent attachment of mannose37,38 and cause a significant decrease of surface hydrophobicity. The reduction in the surface hydrophobicity resulted in the harder binding of IgG or IgE with the protein because the hydrophobicity interaction is the main action force that maintains the binding.39 However, no shift of maximum UV absorbance was observed, suggesting that glycation partially unfolded the β-Lg structure and made aromatic amino acid (mainly Trp) residues exposed on the surface of the β-Lg molecule. The partial unfolding of the β-Lg structure may result in the damage of conformational IgG and IgE epitopes and lead to the reduction of IgG and IgE binding of β-Lg. PEF intensified the changes in the secondary and tertiary structures of β-Lg by improving glycation and further decreased the IgG/IgE-binding ability. Therefore, the destruction of conformational epitopes caused by PEF pretreatment combined with glycation was another reason for the reduction of IgG/IgE-binding of β-Lg, and declined surface hydrophobicity also contributed to the decrease.
In addition, the results also indicated that dry-heating at 55 °C for 4 h had an inconspicuous influence on the IgG/IgE-binding capacity, which resulted from the insignificant changes in the secondary and tertiary structures of β-Lg. It may be caused by the movement limitation of β-Lg molecules due to the low water activity. Similar results were found in a previous study,40 in which the OVA almost retained the secondary and tertiary structures after dry-heating at 80 °C for 7 days. However, PEF treatment at 25 kV cm−1 for 60 μs obviously increased the IgG- and IgE-binding ability of β-Lg. PEF made the structure of β-Lg partially unfolded, reflecting in the decrease in α-helix content and the increase in β-sheet content, UV absorbance intensity and surface hydrophobicity. The result conformed with the findings from some research studies,23,41 wherein they showed that PEF treatment decreased the α-helix structure and increased the β-sheet structure, UV absorbance and ANS-fluorescence intensity of peroxidase and lysozyme. The reduction of the α-helix structure may be attributed to the susceptible influence by a high electric field due to its dipole moment.42 The partial unfolding caused by PEF treatment may result in the exposure of linear and conformational IgG/IgE epitopes originally buried in molecules. Although the conformational epitopes may be destroyed by the partial unfolding, the exposure of linear and conformational IgG/IgE epitopes was more than the destruction of conformational epitopes. Thus, dry-heating at 55 °C for 4 h hardly changed the IgG- and IgE-binding ability of β-Lg, whereas PEF treatment at 25 kV cm−1 for 60 μs markedly enhanced them.
In conclusion, this work provided a comprehensive understanding of the mechanism of reduction in the IgG/IgE-binding ability of β-Lg by PEF pretreatment combined with glycation. It was shown that PEF pretreatment combined with glycation significantly decreased the IgG and IgE binding of β-Lg. Moreover, PEF pretreatment promoted the reduction of IgG and IgE binding abilities by improving glycation. In addition, the results indicated that PEF treatment at 25 kV cm−1 for 60 μs dramatically increased the IgG/IgE-binding capacity by partially unfolding the structure of β-Lg. The result also demonstrated that ECD/FTICR-MS is a powerful tool for analyzing the protein modifications at the molecular level. Therefore, PEF pretreatment combined with glycation may provide an alternative method for β-Lg hyposensitization. However, in the future, other experiments in vivo, such as double-blind placebo-controlled (DBPC) trials should be used to further prove the reduced allergenicity by PEF pretreatment combined with glycation.
Conflicts of interest
There are no conflicts to declare.
Acknowledgements
The authors gratefully acknowledge the financial support of the National Natural Science Foundation of China (NSFC) (No. 21706111), Excellent Youth Foundation of Jiangxi Province (No. 20162BCB23017) and China Agriculture Research System (No. CARS-45).
References
- C. Lifschitz and H. Szajewska, Eur. J. Pediatr., 2015, 174, 141–150 CrossRef CAS PubMed.
- N. O'Riordan, M. Kane, L. Joshi and R. M. Hickey, Glycobiology, 2014, 24, 220–236 CrossRef PubMed.
- L. P. C. Shek, L. Bardina, R. Castro, H. A. Sampson and K. Beyer, Allergy, 2005, 60, 912–919 CrossRef CAS PubMed.
- M. Z. Papiz, L. Sawyer, E. E. Eliopoulos, A. C. T. North, J. B. C. Findlay, R. Sivaprasadarao, T. A. Jones, M. E. Newcomer and P. J. Kraulis, Nature, 1986, 324, 383–385 CrossRef CAS PubMed.
- F. Fogolari, L. Ragona, L. Zetta, S. Romagnoli, K. G. De Kruif and H. Molinari, FEBS Lett., 1998, 436, 149–154 CrossRef CAS PubMed.
- S. Benedé, I. López-Expósito, G. Giménez, G. Grishina, L. Bardina, H. A. Sampson, R. López-Fandiño and E. Molina, Food Res. Int., 2014, 62, 1127–1133 CrossRef.
- K. M. Järvinen, P. Chatchatee, L. Bardina, K. Beyer and H. A. Sampson, Int. Arch. Allergy Immunol., 2001, 126, 111–118 CrossRef.
- K. A. Bloom, F. R. Huang, R. Bencharitiwong, L. Bardina, A. Ross, H. A. Sampson and A. Nowak-Węgrzyn, Pediatr. Allergy Immunol., 2014, 25, 740–746 CrossRef PubMed.
- X. Meng, Y. Bai, J. Gao, X. Li and H. Chen, Food Chem., 2017, 219, 290–296 CrossRef CAS PubMed.
- D. Stanic-Vucinic, M. Stojadinovic, M. Atanaskovic-Markovic, J. Ognjenovic, H. Grönlund, M. van Hage, R. Lantto, A. I. Sancho and T. C. Velickovic, Mol. Nutr. Food Res., 2012, 56, 1894–1905 CAS.
- J. Z. Zhong, W. Liu, C. M. Liu, Q. H. Wang, T. Li, Z. C. Tu, S. J. Luo, X. F. Cai and Y. J. Xu, J. Dairy Sci., 2012, 95, 4237–4245 CrossRef CAS PubMed.
- J. Zhong, Y. Tu, W. Liu, S. Luo and C. Liu, Food Chem., 2015, 188, 658–663 CrossRef CAS PubMed.
- I. López-Expósito, R. Chicón, J. Belloque, R. López-Fandiño and M. C. Berin, J. Dairy Sci., 2012, 95, 541–548 CrossRef PubMed.
- A. Taheri-Kafrani, N. Tavakkoli Koupaie and T. Haertlé, J. Biotechnol., 2015, 212, 181–188 CrossRef CAS PubMed.
- D. Stanic-Vucinic, I. Prodic, D. Apostolovic, M. Nikolic and T. Cirkovic Velickovic, Food Chem., 2013, 138, 590–599 CrossRef CAS PubMed.
- A. Taheri-Kafrani, J.-C. Gaudin, H. Rabesona, C. Nioi, D. Agarwal, M. Drouet, J.-M. Chobert, A.-K. Bordbar and T. Haertle, J. Agric. Food Chem., 2009, 57, 4974–4982 CrossRef CAS PubMed.
- K. Yogesh, J. Food Sci. Technol., 2016, 53, 934–945 CrossRef CAS PubMed.
- X. Tao, J. Chen, L. Li, L. Zhao, M. Zhang and A. Sun, Int. J. Food Prop., 2015, 18, 1416–1427 CrossRef CAS.
- M. J. Rodriguez-Roque, B. de Ancos, R. Sanchez-Vega, C. Sanchez-Moreno, M. P. Cano, P. Elez-Martinez and O. Martin-Belloso, Food Funct., 2016, 7, 380–389 CAS.
- W. Zhao and R. J. Yang, Food Bioprocess Technol., 2012, 5, 1706–1714 CrossRef CAS.
- A. G. Marshall, C. L. Hendrickson and G. S. Jackson, Mass Spectrom. Rev., 1998, 17, 1–35 CrossRef CAS PubMed.
- C. E. Bobst and I. A. Kaltashov, Anal. Chem., 2014, 86, 5225–5231 CrossRef CAS PubMed.
- W. Zhao, R. Yang, R. Lu, Y. Tang and W. Zhang, J. Agric. Food Chem., 2007, 55, 9850–9858 CrossRef CAS PubMed.
- W.-H. Yang, Z.-C. Tu, H. Wang, X. Li and M. Tian, J. Sci. Food Agric., 2017, 97, 2714–2720 CrossRef CAS PubMed.
- X. Q. Huang, Z. C. Tu, H. Wang, Q. T. Zhang, Y. Shi and H. Xiao, J. Agric. Food Chem., 2013, 61, 2253–2262 CrossRef CAS PubMed.
- D. Matulis and R. Lovrien, Biophys. J., 1998, 74, 422–429 CrossRef CAS PubMed.
- W. Zhao, Y. Tang, L. Lu, X. Chen and C. Li, Food Bioprocess Technol., 2014, 7, 114–125 CrossRef CAS.
- Y. Chen, Z. Tu, H. Wang, Q. Zhang, L. Zhang, X. Sha, T. Huang, D. Ma, J. Pang and P. Yang, J. Agric. Food Chem., 2017, 65, 6179–6187 CrossRef CAS PubMed.
- A. Barbiroli, F. Bonomi, P. Ferranti, D. Fessas, A. Nasi, P. Rasmussen and S. Iametti, J. Agric. Food Chem., 2011, 59, 5729–5737 CrossRef CAS PubMed.
- X. Li, S. Yuan, S. He, J. Gao and H. Chen, J. Sci. Food Agric., 2015, 95, 2916–2923 CrossRef CAS PubMed.
- G. Ball, M. J. Shelton, B. J. Walsh, D. J. Hill, C. S. Hosking and M. E. H. Howden, Clin. Exp. Allergy, 1994, 24, 758–764 CrossRef CAS PubMed.
- I. Sélo, G. Clément, H. Bernard, J.-M. Chatel, C. Créminon, G. Peltre and J. M. Wal, Clin. Exp. Allergy, 1999, 29, 1055–1063 CrossRef.
- I. Sélo, L. Négroni, C. Créminon, M. Yvon, G. Peltre and J. M. Wal, Int. Arch. Allergy Immunol., 1998, 117, 20–28 CrossRef.
- X. J. Ma, H. B. Chen, J. Y. Gao, C. Q. Hu and X. Li, Food Addit. Contam., Part A: Chem., Anal., Control, Exposure Risk Assess., 2013, 30, 1684–1692 CrossRef CAS PubMed.
- M. A. Glomb and V. M. Monnier, J. Biol. Chem., 1995, 270, 10017–10026 CrossRef CAS PubMed.
- T. Aoki, Y. Hiidome, Y. Sugimoto, H. R. Ibrahim and Y. Kato, Food Res. Int., 2001, 34, 127–132 CrossRef CAS.
- Y. Chen, Z. Tu, H. Wang, L. Zhang, X. Sha, J. Pang, P. Yang, G. Liu and W. Yang, Food Res. Int., 2016, 89(Part 1), 882–888 CrossRef CAS PubMed.
- J. Zhong, Y. Tu, W. Liu, Y. Xu, C. Liu and R. Dun, J. Dairy Sci., 2014, 97, 4695–4702 CrossRef CAS PubMed.
- J. J. Gooding, C. Wasiowych, D. Barnett, D. B. Hibbert, J. N. Barisci and G. G. Wallace, Biosens. Bioelectron., 2004, 20, 260–268 CrossRef CAS PubMed.
- N. Matsudomi, H. Takahashi and T. Miyata, Food Res. Int., 2001, 34, 229–235 CrossRef CAS.
- K. Zhong, J. Wu, Z. Wang, F. Chen, X. Liao, X. Hu and Z. Zhang, Food Chem., 2007, 100, 115–123 CrossRef CAS.
- A. Budi, F. S. Legge, H. Treutlein and I. Yarovsky, J. Phys. Chem. B, 2007, 111, 5748–5756 CrossRef CAS PubMed.
Footnote |
† Electronic supplementary information (ESI) available. See DOI: 10.1039/c7fo01082f |
|
This journal is © The Royal Society of Chemistry 2018 |