DOI:
10.1039/C7SC03739B
(Edge Article)
Chem. Sci., 2018,
9, 1596-1603
A structurally-characterized peroxomanganese(IV) porphyrin from reversible O2 binding within a metal–organic framework†
Received
25th August 2017
, Accepted 13th December 2017
First published on 14th December 2017
Abstract
The role of peroxometal species as reactive intermediates in myriad biological processes has motivated the synthesis and study of analogous molecular model complexes. Peroxomanganese(IV) porphyrin complexes are of particular interest, owing to their potential ability to form from reversible O2 binding, yet have been exceedingly difficult to isolate and characterize in molecular form. Alternatively, immobilization of metalloporphyrin sites within a metal–organic framework (MOF) can enable the study of interactions between low-coordinate metal centers and gaseous substrates, without interference from bimolecular reactions and axial ligation by solvent molecules. Here, we employ this approach to isolate the first rigorously four-coordinate manganese(II) porphyrin complex and examine its reactivity with O2 using infrared spectroscopy, single-crystal X-ray diffraction, EPR spectroscopy, and O2 adsorption analysis. X-ray diffraction experiments reveal for the first time a peroxomanganese(IV) porphyrin species, which exhibits a side-on, η2 binding mode. Infrared and EPR spectroscopic data confirm the formulation of a peroxomanganese(IV) electronic structure, and show that O2 binding is reversible at ambient temperature, in contrast to what has been observed in molecular form. Finally, O2 gas adsorption measurements are employed to quantify the enthalpy of O2 binding as hads = −49.6(8) kJ mol−1. This enthalpy is considerably higher than in the corresponding Fe- and Co-based MOFs, and is found to increase with increasing reductive capacity of the MII/III redox couple.
Introduction
The activation of O2 by metalloproteins is central to a wide range of biological processes, including bond activation, O2 transport, metabolism, and the regulation of reactive oxygen species.1 In many of these processes, metal complexes of O22−, or peroxide, represent reactive intermediates that are generated during the catalytic cycles of enzymatic reactions.1a–e,2 For instance, C–H bond activation by heme-containing enzymes involves peroxoiron intermediates.1a–c,2a,b,d In addition, water oxidation by the oxygen-evolving complex in photosystem II2e and the degradation of superoxide ion by manganese superoxide dismutase2a are postulated to proceed through peroxomanganese intermediates. The prominence and unusual reactivity of peroxoiron and manganese species in biology has motivated efforts to synthesize and characterize synthetic molecular model complexes, largely in order to elucidate the structure, physical properties, and chemical reactivity of these intermediates.2b,3 Indeed, tremendous progress has been made in the synthesis and study of both peroxoiron and manganese complexes. For instance, a number of mononuclear heme4 and non-heme5 peroxoiron complexes have been isolated, including two examples5a,b that have been structurally characterized. In addition, several mononuclear peroxomanganese species have been isolated,6–8 with two recent structurally-characterized examples featuring MnIV.9
Despite these advances, significant challenges remain in the isolation of peroxomanganese complexes that display reversible O2 binding under ambient conditions, an important function often found in biological systems.10 Toward this end, manganese(II) porphyrin complexes present an attractive platform, owing to their ability to carry out the two-electron reductive activation of O2 (ref. 11) and thus form high-valent peroxomanganese complexes.12 To date, one crystal structure of an O2 coordinated to a manganese porphyrin has been reported. This complex, formed by addition of KO2 to (TPP)MnII (H2TPP = 5,10,15,20-tetraphenylporphyrin), was shown to feature a MnIII ion coordinated to a peroxo ligand in a side-on, η2 binding mode.7a While [(TPP)Mn(O2)]− provides an important structural example of the peroxo binding mode, the presence of MnIII renders O2 loss unfavourable, as it would necessitate the formation of a high-energy MnI species. In contrast, peroxomanganese(IV) complexes, as formed by reaction of MnII with O2, have been probed by numerous spectroscopic techniques, including EPR,12a,c,e vibrational,12l,n NMR,12k and UV/visible12a,c spectroscopies, in addition to computational methods.12d,i Although these investigations support a side-on peroxomanganese(IV), this geometry has not been confirmed by a crystal structure. Furthermore, the thermal instability of peroxomanganese(IV) species has limited their characterization and hindered a thorough investigation of their structure and properties.
A key limitation in the study of O2 binding in molecular metalloporphyrins is the propensity for these species to form oxo-bridged complexes via irreversible bimolecular condensation reactions.13 For instance, in FeII and CoII complexes, highly elaborate ligands such as the sterically protected “picket-fence” porphyrins were necessary to prevent bimolecular decomposition reactions and enable the isolation and thorough characterization of six-coordinate Fe and Co–O2 adducts, which feature axial imidazole or thiolate ligands.14 In contrast, analogous axially-ligated MnII complexes do not bind O2, which has been attributed to the preference for five-coordinate geometries in porphyrinic Mn ions.12b,g Consequently, no peroxomanganese(IV) porphyrin species have been isolated or studied under ambient conditions.
As an alternative to employing molecular systems, one can envision isolating a peroxomanganese(IV) complex within a porphyrinic metal–organic framework (MOF). Here, the porous, solid-state structure of the MOF prevents bimolecular condensation reactions and enables introduction of gas-phase substrates in the absence of exogenous solvent. Furthermore, whereas the inherent reactivity of the peroxomanganese(IV) complexes has precluded crystallographic characterization, the crystallinity of MOFs provides an ideal platform to carry out single-crystal X-ray diffraction analysis. Illustrative of this approach, we have previously shown that the porphyrinic MOF PCN-224 (ref. 15) can be employed to study low-coordinate O2 adducts16,17 and labile carbonyl complexes.18 Herein, we comprehensively examine O2 binding to MnII in PCN-224 using a host of physical methods. Taken together, these experiments unambiguously establish the presence of a side-on peroxomanganese(IV) species, and the O2 binding is shown to be reversible even at ambient temperature.
Results and discussion
Synthesis of PCN-224MnII
The porphyrinic MOF PCN-224 was synthesized as previously described.15 Subsequent metalation of the porphyrin with MnII was carried out by heating single crystals of PCN-224 under N2 in a DMF solution containing excess MnBr2 and 2,6-lutidine, followed by evacuation at 150 °C for 12 h, to give the compound PCN-224MnII (1). Complete metalation of the porphyrin within the bulk crystalline material was confirmed by solid-state diffuse reflectance UV/visible spectroscopy, trace metals analysis and powder X-ray diffraction (see Fig. 1 and S1 and Experimental section). Furthermore, N2 adsorption data collected for a desolvated sample of 1 at 77 K provided a Brunauer–Emmett–Teller surface area of 2455 m2 g−1 (see Fig. S2†), close to the accessible surface reported for other metalated variants of PCN-224,15–17,19 thereby confirming the retention of porosity upon metalation and the successful removal of solvent molecules from the pores. While several manganese porphyrin-containing MOFs have been reported, to our knowledge,201 represents the first example of a MOF that features a four-coordinate MnII porphyrin complex.
The diffuse reflectance UV/visible spectrum obtained for an activated sample of 1 exhibits similar peak maxima to the absorption spectrum of 1 suspended in toluene, but nevertheless features key differences (see Fig. 1). Most notably, the spectrum reported for 1 in toluene displays a Soret band at 448 nm, while the spectrum for activated 1 features a Soret band at 417 nm. These differences can possibly be attributed to the rigorous four-coordinate nature of the Mn center in 1, compared to a slight distortion from local D4h symmetry at Mn in the toluene solution imposed by Mn–toluene interactions as has been observed for the molecular analogue, (TPP)Mn (see crystallography discussion below).8b,16 More specifically, these changes may be attributed to the difference in mixing of metal- and porphyrin-based π orbitals in the two complexes, as changes in the degree of mixing are known to influence the resulting adsorption spectra of metalloporphyrin complexes.21 In the case of the rigorously in-plane Mn center, the maximal overlap of Mn dxz,yz and porphyrin π* orbitals may account for the changes in the features in the electronic spectrum of 1.
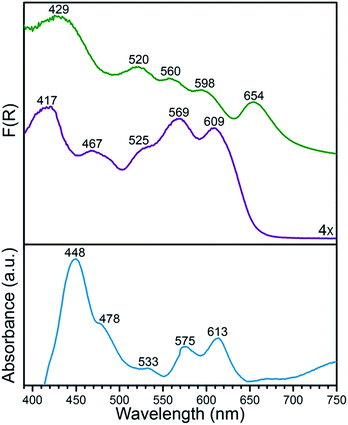 |
| Fig. 1 Solid-state diffuse reflectance UV/visible spectra for PCN-224 (green) and its MnII-metalated form 1 (purple), plotted as the Kubelka–Munk function F(R). For comparison, the absorption spectrum for a toluene suspension of 1 (blue) is shown, highlighting changes in the peak positions with and without toluene. | |
Infrared spectroscopy
As an initial investigation into the interaction between MnII and O2, a desolvated sample of 1 was exposed to ca. 1 atm of dry O2 and monitored by diffuse reflectance infrared Fourier transform spectroscopy (DRIFTS). The addition of O2 to 1 at 298 K was accompanied by the appearance of three new vibrations, situated at 801, 984, and 1017 cm−1 (see Fig. 2), indicative of conversion to a Mn–O2 complex in the compound PCN-224MnO2 (2). The vibrations at 801 and 1017 cm−1 have been previously attributed to a lowering of local symmetry from D4h to C2v at MnII upon O2 binding.12l This symmetry reduction occurs due to a displacement of Mn from the N4 plane of the porphyrin upon the addition of an axial ligand, in this case O2. Moreover, the feature at 984 cm−1 is identical within error to the formerly assigned νO–O vibration from a mixture of the molecular complex (TPP)MnII and O2, as isolated in a frozen Ar matrix at 15 K.12l Remarkably, in contrast to molecular systems, where the deoxymanganese(II) complex was only partially regenerated after purging with N2 at −78 °C, similar purging here with dry Ar gas at 25 °C gave a spectrum identical to that obtained for 1 before adding O2 (see Fig. 2).12c This process of adding then removing O2 could be cycled at least three times, underscoring the ability of the solid-state MOF to enable reversible O2 binding (see Fig. S3†).
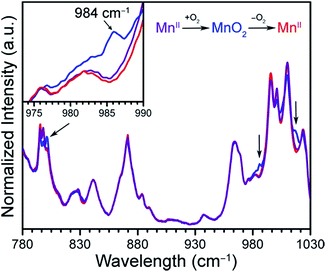 |
| Fig. 2 DRIFTS spectra for 1 at 298 K under static vacuum (purple), upon addition of O2 (blue), and after subsequent purging with Ar (red). The inset shows an expanded view of the spectra, highlighting the νO–O vibration of the dioxygen adduct in 2 at 984 cm−1. | |
The νO–O vibration in 2 at 984 cm−1 is considerably lower than those of νO–O = 1195 and 1278 cm−1 previously observed for O2 adducts of (TPP)Fe and (TPP)Co at 15 K, which have been unambiguously assigned as superoxometal(III) species.22 This contrast suggests that O2 binding in PCN-224MnII involves a two-electron transfer from MnII to O2 to give a peroxomanganese(IV) species, rather than one-electron transfer to give a superoxomanganese(III) species.12a–c,e–n Here, the weaker O–O bond in 2 relative to superoxo complexes can be primarily attributed to a doubly occupied π* orbital of an O22− ligand compared to singly occupied orbital of O2˙−. Additionally, the νO–O = 984 cm−1 in 2 is higher than those of νO–O = 806 cm−1 and 898 cm−1 previously reported for the peroxoiron(III) complex3a [(OEP)FeO2]−and the peroxotitanium(IV) complex23 (OEP)TiO2 (H2OEP = 2,3,7,8,12,13,17,18-octaethylporphyrin), which both feature side-on coordination of O22−. This difference may stem from increasing stabilization of the σ bond of O22− with increasing effective nuclear charge moving from FeIII to TiIV to MnIV. In addition, the concomitant increase in Lewis acidity across the series may serve to pull electron density out of the O22−, thereby strengthening the O–O bond by alleviating electron–electron repulsion in O22−. While the foregoing comparison of data is consistent with the presence of a side-on O22− in 2, analysis of infrared spectra alone cannot definitively confirm this assignment.
Single-crystal X-ray diffraction
The stability of the Mn–O2 adduct in 2 prompted us to investigate both 1 and 2 using single-crystal X-ray diffraction analysis. The structure of 1 exhibits a four-coordinate MnII center, residing in a square planar coordination environment on a crystallographic special position of mm2 site symmetry (see Fig. 3, S3, and Table S1†). Importantly, no significant residual electron density was located in the difference Fourier map, confirming the absence of axial ligation at Mn. The Mn–N distance of 1.998(5) Å is notably shorter than those of 2.082(2)–2.085(2) Å previously reported for the toluene-solvated compound (TPP)Mn·2C7H8.12b,24 While this molecular compound features a pseudo four-coordinate MnII center, weak contacts between Mn and a toluene molecule, with a closest Mn–Ctoluene distance of 3.04 Å, lead to a 0.19 Å displacement of Mn from the N4 plane and thus slightly longer Mn–N bonds relative to 1. As such, to our knowledge, the structure of 1 provides the first example of a four-coordinate Mn porphyrin species. The isolation of a rigorously four-coordinate Mn center within a porphyrin ligand is remarkable given the relatively large ionic radius of MnII, and thus its propensity to displace out of the N4 plane12b to form five-coordinate complexes.12g Indeed, the complex in 1 demonstrates the utility of MOFs to enable isolation of reactive low-coordinate metal complexes.
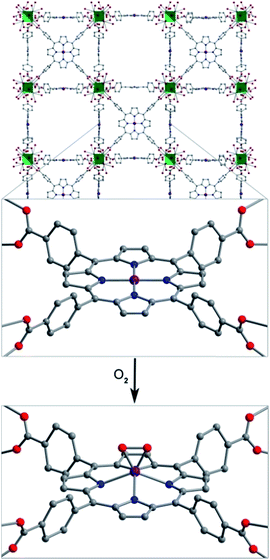 |
| Fig. 3 Reaction of 1 with O2 to form 2. Vertices of the green octahedra represent Zr atoms; purple, red, blue, and gray spheres represent Mn, O, N, and C atoms, respectively; H atoms are omitted for clarity. Selected interatomic distances (Å) for 1: Mn⋯N4 0, Mn–N 1.998(5); for 2: Mn⋯N4 0.80(2), Mn–O 1.76(3), Mn–N 2.170(9). | |
Exposure of single crystals of 1 to ca. 1 atm of dry O2 at −78 °C resulted in an immediate color change from purple to black. Subsequent X-ray diffraction analysis at 100 K revealed the formation of 2. The structure of 2 is globally quite similar to that of 1, but with key differences in the Mn coordination environment (see Fig. 3, S3, and Table S2†). Most importantly, the structure features a Mn ion that is coordinated to O2via a side-on, η2 binding mode. In addition, the Mn ion is displaced from the N4 plane by 0.80(2) Å, slightly longer than the displacement of 0.7640(4) Å observed for the peroxomanganese(III) unit in a potassium cryptate salt of [(TPP)MnO2]−.7a Likewise, the Mn–N and Mn–O distances of 2.170(9) and 1.76(3) Å in 2 are slightly shorter than the analogous mean distances of 2.184(4) and 1.895(4) Å in [(TPP)MnO2]−. While these differences are consistent with the presence of a smaller ionic radius for MnIVvs. MnIII, they should be regarded with caution owing to the positional disorder and large thermal ellipsoids of O atoms in 2 (see Fig. S4†). Finally, this crystallographic disorder also precludes a reliable determination of the O–O distance in 2. Here, this distance was fixed to a target value of 1.40 ± 0.02 Å, based on other peroxometal complexes with similar values of νO–O,9,23,25 and subsequent refinement of the structure gave a distance of 1.39(2) Å.
In the structure of 2, the Operoxo atoms are related through a crystallographic mirror plane. As such, the structure was modeled with an O2 unit bound symmetrically with respect to Mn. However, we cannot exclude the possibility of some asymmetry based on the current structure. Indeed, several previously reported crystal structures of η2-bound peroxometal complexes feature asymmetric coordination of the peroxo ligand.7,9 Nevertheless, the structure of 2 shows that the O–O bond eclipses the pyrrole-based N atoms. This conformation contrasts the results from charge iterative extended Hückel (IEH) calculations on a peroxomanganese(IV) porphyrin complex, which suggested the presence of a staggered peroxo ligand to alleviate electrostatic repulsion.12j However, the eclipsed conformation observed for 2 is consistent with those determined crystallographically for the peroxometal porphyrin complexes (OEP)TiO2,23 (TPP)Mo(O2)2,26 and [(TPP)MnO2]−.7a
Despite the large standard deviations in the structure of 2, this analysis unambiguously reveals the presence of an O2 adduct coordinated to Mn through a side-on, η2 binding mode, with the O2 eclipsing the N atoms of the porphyrin pyrroles. Moreover, the complex in 2 represents a rare example of both a structurally characterized peroxometalloporphyrin7a,23,26 and a peroxomanganese(IV) complex.9 To our knowledge, 2 provides the first crystal structure of a peroxomanganese(IV) in a porphyrinoid ligand and a rare example of a peroxomanganese(IV) in any ligand environment.9,27
Electron paramagnetic resonance (EPR) spectroscopy
To further probe the electronic structures of 1 and 2, as well as the reversibility of O2 binding, continuous wave X-band EPR spectra were collected on samples at selected temperatures between 298 K and 4.2 K (Fig. 4 and 5; see Fig. S5† for full temperature range). The spectrum obtained at 77 K for a rigorously activated sample of 1 shows a sharp axial pattern, with observed g values of g⊥ = 6 and g‖ = 2 that are characteristic of an S = 5/2 MnII ion with zero-field splitting parameters of D ≫ hν, E/D = λ = 0 (see Fig. 4, upper). In addition, the low field portion features a well-resolved six-line hyperfine splitting by the I = 5/2 55Mn nucleus, with a hyperfine constant of A⊥Mn ≈ 49 G (138 MHz).
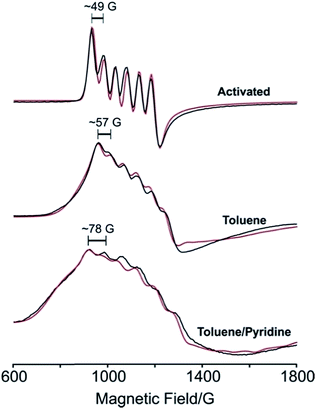 |
| Fig. 4 Experimental (black) and simulated (red) X-band EPR spectra of 1 at 77 K after activation (upper), in toluene (center), and in a mixture of toluene and pyridine (lower). | |
Addition of toluene to a crystalline sample of 1 led to a broadening of the g⊥ region of the spectrum and an increase in the hyperfine coupling (Fig. 4, middle). Accordingly, simulation of the spectrum with the program Easyspin27 show that interaction of 1 with toluene lowers the four-fold symmetry of the MnII ion, as indicated by the introduction of a non-zero rhombicity of the zero-field splitting, λ ≈ 0.027, along with an increase in hyperfine coupling to A⊥Mn ≈ 57 G (160 MHz). Addition of a three-fold molar excess of pyridine in toluene to 1 (Fig. 4, lower) further lowers the symmetry, as indicated by a further increase in the rhombicity and the hyperfine coupling to λ ≈ 0.041 and A⊥Mn ≈ 78 G (219 MHz), respectively. It appears that pyridine binding introduces a rhombicity in the hyperfine interaction as well, although precisely reproducing the poorly-resolved spectrum is not illuminating. The progressive departure from four-fold symmetry is evident from increases in λ (see Table S3†). The change in the spectrum simply upon addition of toluene solvent strongly supports the four-coordinate nature of activated 1, and the transformation to a five-coordinate complex even upon addition of toluene solvent, not merely with the strongly-coordinating pyridine. The progressive increase in hyperfine coupling in these three samples reflects an increasing departure of the MnII from the N4 plane.
Dosing 1 with ca. 1 atm of dry O2 resulted in conversion to 2, which features a rhombic spectrum at ∼7 K (see Fig. 5, lower). As has been analysed in detail for Mn(TPP)O2, O2 binding yields a complex formally described as a high-spin MnIV peroxo species, with the rhombicity of this EPR spectrum associated with orbital mixing on the MnIV.12a,c,e Following the analysis for Mn(TPP)O2, the spectrum represents an S = 3/2 spin state with axial zero-field parameter, D < 0 and |D| much greater than the microwave quantum (0.3 cm−1). In this case the spectrum comprises the overlap of two spectra, arising from transitions within each of the two doublets created by the zero-field splitting of the S = 3/2 state, and the g-values of those spectra depend only on the rhombicity parameter, λ. As with (TPP)MnO2, the spectrum in Fig. 4 corresponds well to the limit λ → 1/3, where both doublets exhibits a g-tensor with g values of [5.4–5.5, 2, 1.45].12c The feature at g = 5.4 exhibits the overlap of two 55Mn hyperfine sextet patterns, where Al = 54 G (154 MHz) for the lower doublet and Au = 89 G (251 MHz) for the upper doublet (see Fig. 5 and Table S3†).12a,c Orbital mixing on MnIV causes the difference in the observed 55Mn hyperfine splitting for the two doublets.18,28
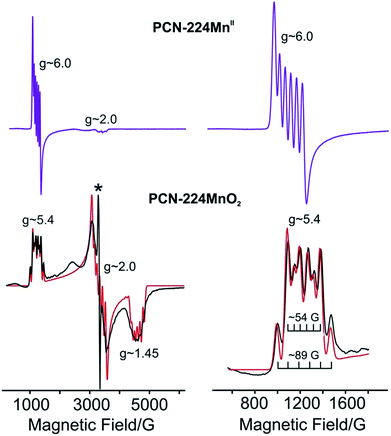 |
| Fig. 5 Left: X band EPR spectrum of 1 (purple lines) and 2 (black lines) at 4.2 K, with simulation shown in red. Right: Expanded view of the low-field portion of the EPR spectrum. Braces in the inset show the calculated hyperfine splittings of the superposed lower (Al ≈ 54 G) and upper (Au ≈ 89 G) sextets. The asterisk denotes an impurity of S = 1/2 with g = 2.00. | |
The ratio of signal intensities for the two doublets is determined by the Boltzmann populations of the two doublets at the measurement temperature, as determined by the energy difference between them, Δ = 2(D2 + 3E2)1/2 = 2|D|(1 + 3λ2)1/2. Taking into account the negative sign of D, the simulated overlapping spectra and their relative intensities at T 4.2 K are nicely reproduced using the measured hyperfine coupling and, D = −1.76 cm−1, λ = 1/3, with a corresponding zero-field energy separation of the two doublets, Δ = 4.07 cm−1. These values of D, Δ can be compared to D = −2.48 cm−1, λ ∼1/3, Δ = 5.82 cm−1 for Mn(TPP)O2.
As the temperature is increased from 4.2 K, the populations of the two doublets begin to equalize and the spectra broaden, likely in some part because of rotation of the O2 (see Fig. S5†). Finally, when 2 was purged with Ar at 298 K and evacuated for 12 h, a spectrum identical to that for 1 was obtained (see Fig. S6†). This observation corroborates the reversibility of O2 binding at Mn as revealed by DRIFTS analysis.
O2 adsorption
The reversibility of the O2 interaction enables quantitation of the O2 binding thermodynamics in PCN-224MnII through variable temperature O2 adsorption measurements. Toward this end, O2 uptake data were collected at selected temperatures between 233 and 298 K, as depicted in Fig. 6 and S7–S8. At 233 K, the O2 isotherm exhibits an initial steep uptake at low pressure. As the temperature is increased, the slope of this steep region decreases until the isotherm becomes closer to linear at 298 K. To quantify the strength of O2 binding, the isotherm data were fit to a dual-site Langmuir–Freundlich model (see Table S4†), and subsequent treatment of the variable-temperature data with the Clausius–Clapeyron equation revealed a differential enthalpy of adsorption of hads = −49.6(8) kJ mol−1 at low loading, followed by a gradual drop near 1
:
1 Mn
:
O2 to a plateau at hads = −9(2) kJ mol−1. We assign these values to O2 binding at the Mn center and physisorption to the remainder of the MOF surface, respectively.
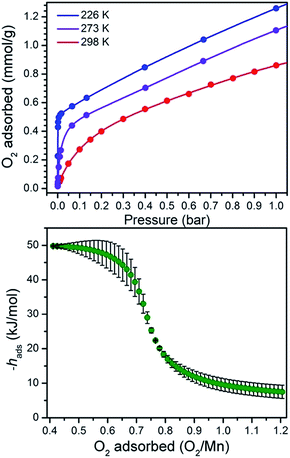 |
| Fig. 6 Upper: O2 adsorption data for 1 at 233, 273, and 298 K (blue to red gradient). Circles represent data, and solid lines correspond to fits using a dual-site Langmuir–Freundlich model. Lower: O2 differential enthalpy of adsorption curve for 1, plotted as a function of O2 adsorbed. Green circles represent data, and error bars are shown in black. | |
The binding of O2 to MnII in PCN-224MnII is significantly stronger than the analogous values measured for O2 binding at the four-coordinate metal centers in PCN-224FeII and PCN-224CoII of −34(4) and −15.2(6) kJ mol−1, respectively.16,18 As previously discussed, the difference in binding enthalpy can primarily be attributed to the difference in the redox couple at the metal center, which is associated with electron transfer upon the binding of O2.12a,29 In line with this relationship, the enthalpy of M–O2 binding from Mn to Fe to Co in PCN-224MII decreases as E1/2 (V vs. SCE) of MII/III of the analogous molecular metalloporphyins becomes less reducing (Mn: −0.230; Fe: −0.047; Co: +0.320).29 Note, however, that the binding enthalpy in Mn is further strengthened by the fact that O2 binding involves a two-electron transfer from Mn to O2.
Conclusions
The foregoing results demonstrate the ability of a MOF to enable the isolation and crystallographic characterization of a four-coordinate manganese porphyrin center and its corresponding O2 adduct. A combined array of single-crystal X-ray diffraction, solid-state infrared, and EPR analysis collectively demonstrate the O2 complex to comprise a peroxo ligand bound in a side-on, η2 mode to an S = 3/2 MnIV ion. In addition, these experiments reveal that O2 binding is reversible, even at ambient temperature, in stark contrast to behaviour observed in molecular analogues. Finally, O2 gas adsorption measurements quantify the enthalpy of O2 binding as hads = −49.6(8) kJ mol−1. This value is considerably higher than in the corresponding Fe- and Co-based MOFs, and the strength of binding is found to increase with increasing reductive capacity of the MII/III redox couple. Work is underway to carry out and study the protonation, O–O bond cleavage, and O-atom transfer ability of the peroxo ligand, with emphasis on isolating and structurally characterizing the intermediates involved in these processes.
Conflicts of interest
There are no conflicts to declare.
Acknowledgements
Research in the Harris laboratory was funded by the U. S. Army Research Office (W911NF-14-1-0168 and W911NF-15-1-0331), the American Chemical Society Petroleum Research Fund (56081-DNI3), and Northwestern University. Research in the Hoffman laboratory was funded by the National Institutes of Health National Institute of General Medical Sciences (GM 111097). A. T. G. is supported primarily by the National Science Foundation through the Graduate Research Fellowship Program. We thank Dr C. Malliakas for assistance in modeling the X-ray diffraction data, Dr L. Liu for assistance in collecting powder X-ray diffraction data, Dr A. Marts for assistance in collecting initial EPR spectra, and Mr M. S. Fataftah, Dr J. P. S. Walsh, and Dr A. Sharma for helpful discussions.
References
-
(a) M. Sono, M. P. Roach, E. D. Coulter and J. H. Dawson, Chem. Rev., 1996, 96, 2841 CrossRef CAS PubMed
;
(b) B. Meunier, S. P. de Visser and S. Shaik, Chem. Rev., 2004, 104, 3947 CrossRef CAS PubMed
;
(c) M. Costas, M. P. Mehn, M. P. Jensen and L. Que Jr, Chem. Rev., 2004, 104, 939 CrossRef CAS PubMed
;
(d) A. Decker and E. I. Solomon, Curr. Opin. Chem. Biol., 2005, 9, 152 CrossRef CAS PubMed
;
(e) M. M. Abu-Omar, A. Loaiza and N. Hontzeas, Chem. Rev., 2005, 105, 2227 CrossRef CAS PubMed
;
(f) T. L. Poulos, Chem. Rev., 2014, 114, 3919 CrossRef CAS PubMed
.
-
(a) C. Bull, E. C. Niederhoffer, T. Yoshida and J. A. Fee, J. Am. Chem. Soc., 1991, 113, 4069 CrossRef CAS
;
(b) J. A. Kovacs and L. M. Brines, Acc. Chem. Res., 2007, 40, 501 CrossRef CAS PubMed
;
(c) I. G. Denisov, P. J. Mark, T. M. Makris, S. G. Sligar and J. R. Kincaid, J. Phys. Chem. A, 2008, 112, 13172 CrossRef CAS PubMed
;
(d) W. A. Gunderson, A. I. Zatsman, J. P. Emerson, E. R. Farquhar, L. Que Jr, J. D. Lipscomb and M. P. Hendrich, J. Am. Chem. Soc., 2008, 130, 14465 CrossRef CAS PubMed
;
(e) G. Renger and T. Renger, Photosynth. Res., 2008, 98, 53 CrossRef CAS PubMed
;
(f) D. J. Vinyard, G. M. Ananyev and G. C. Dismukes, Annu. Rev. Biochem., 2013, 82, 577 CrossRef CAS PubMed
;
(g) E. I. Solomon, D. E. Heppner, E. M. Johnston, J. W. Ginsbach, J. Cirera, M. Qayyum, M. T. Kieber-Emmons, C. H. Kjaergaard, R. G. Hadt and L. Tian, Chem. Rev., 2014, 114, 3659 CrossRef CAS PubMed
;
(h) S. Sahu and D. P. Goldberg, J. Am. Chem. Soc., 2016, 138, 11410 CrossRef CAS PubMed
.
-
(a) E. McCandlish, A. R. Miksztal, M. Nappa, A. Q. Sprenger, J. S. Valentine, J. D. Stong and T. G. Spiro, J. Am. Chem. Soc., 1980, 102, 4268 CrossRef CAS
;
(b) C. H. Welborn, D. Dolphin and B. R. James, J. Am. Chem. Soc., 1981, 103, 2869 CrossRef CAS
;
(c)
C. A. Reed, in Electrochemical and Spectrochemical Studies of Biological Redox Components, ed. K. M. Kadish, American Chemical Society, Washington, D.C, 1982, vol. 201, ch. 15, pp. 333–356 Search PubMed
;
(d) K. Bajdor and K. Nakamoto, J. Am. Chem. Soc., 1984, 106, 3045 CrossRef CAS
;
(e) P. Friant, J. Goulon, J. Fischer, L. Ricard, M. Schappacher, R. Weiss and M. Momenteau, Nouv. J. Chim., 1985, 9, 33 CAS
.
- Selected examples of peroxoheme species:
(a) R. A. Ghiladi, R. M. Kretzer, I. Guzei, A. L. Rheingold, Y.-M. Neuhold, K. R. Hatwell, A. D. Zuberbühler and K. D. Karlin, Inorg. Chem., 2001, 40, 5754 CrossRef CAS PubMed
;
(b) E. Kim, M. E. Helton, I. M. Wasser, K. D. Karlin, S. Lu, H. W. Huang, P. Moenne-Loccoz, C. D. Incarvito, A. L. Rheingold, M. Honecker, S. Kaderli and A. D. Zuberbuhler, Proc. Natl. Acad. Sci. U. S. A., 2003, 100, 3623 CrossRef CAS PubMed
;
(c) K. Mittra, S. Chatterjee, S. Samanta, K. Sengupta, H. Bhattacharjee and A. Dey, Chem. Commun., 2012, 48, 10535 RSC
;
(d) M. F. Sisemore, M. Selke, J. N. Burstyn and J. S. Valentine, Inorg. Chem., 1997, 36, 979 CrossRef CAS PubMed
;
(e) M. F. Sisemore, M. Selke, J. N. Burstyn and J. S. Valentine, Inorg. Chem., 1997, 36, 979 CrossRef CAS PubMed
.
- Selected examples of non-heme peroxo species:
(a) J. Cho, S. Jeon, S. A. Wilson, L. V. Liu, E. A. Kang, J. J. Braymer, M. H. Lim, B. Hedman, K. O. Hodgson, J. S. Valentine, E. I. Solomon and W. Nam, Nature, 2011, 478, 502 CrossRef CAS PubMed
;
(b) E. D. Bloch, L. J. Murray, W. L. Queen, S. Chavan, S. N. Maximoff, J. P. Bigi, R. Krishna, V. K. Peterson, F. Grandjean, G. J. Long, B. Smit, S. Bordiga, C. M. Brown and J. R. Long, J. Am. Chem. Soc., 2011, 133, 14814 CrossRef CAS PubMed
;
(c) L. V. Liu, S. Hong, J. Cho, W. Nam and E. I. Solomon, J. Am. Chem. Soc., 2013, 135, 3286 CrossRef CAS PubMed
;
(d) W. Nam, Acc. Chem. Res., 2015, 48, 2415 CrossRef CAS PubMed
.
- Selected examples of isolated peroxomanganese(III) species:
(a) D. L. Werz and J. S. Valentine, Struct. Bonding, 2000, 97, 37 CrossRef
;
(b) R. L. Shook, W. A. Gunderson, J. Greaves, J. W. Ziller, M. P. Hendrich and A. S. Borovik, J. Am. Chem. Soc., 2008, 130, 8888 CrossRef CAS PubMed
;
(c) R. A. Geiger, S. Chattopadhyay, V. W. Day and T. A. Jackson, J. Am. Chem. Soc., 2010, 132, 2821 CrossRef CAS PubMed
;
(d) R. L. Shook and A. S. Borovik, Inorg. Chem., 2010, 49, 3646 CrossRef CAS PubMed
;
(e) R. A. Geiger, S. Chattopadhyay, V. W. Day and T. A. Jackson, Dalton Trans., 2011, 40, 1707 RSC
;
(f) R. L. Shook, S. M. Peterson, J. Greaves, C. Moore, A. L. Rheingold and A. S. Borovik, J. Am. Chem. Soc., 2011, 133, 5810 CrossRef CAS PubMed
;
(g) H. So, Y. J. Park, K.-B. Cho, Y.-M. Lee, M. S. Seo, J. Cho, R. Sarangi and W. Nam, J. Am. Chem. Soc., 2014, 136, 12229 CrossRef CAS PubMed
;
(h) K. Ray, F. F. Pfaff, B. Wang and W. Nam, J. Am. Chem. Soc., 2014, 136, 13942 CrossRef CAS PubMed
;
(i) D. F. Leto and T. A. Jackson, J. Biol. Inorg Chem., 2014, 19, 1 CrossRef CAS PubMed
;
(j) H. E. Colmer, A. W. Howcroft and T. A. Jackson, Inorg. Chem., 2016, 55, 2055 CrossRef CAS PubMed
;
(k) P. Barman, P. Upadhyay, A. S. Faponle, J. Kumar, S. S. Nag, D. Kumar, C. V. Sastri and S. P. de Visser, Angew. Chem., 2016, 128, 11257 CrossRef
.
- Examples of structurally-characterized peroxomanganese(III) species:
(a) R. B. VanAtta, C. E. Strouse, L. K. Hanson and J. S. Valentine, J. Am. Chem. Soc., 1987, 109, 1425 CrossRef
;
(b) N. Kitajima, H. Komatsuzaki, S. Hikichi, M. Osawa and Y. Moro-oka, J. Am. Chem. Soc., 1994, 116, 11596 CrossRef CAS
;
(c) U. P. Singh, A. K. Sharma, S. Hikichi, H. Komatsuzaki, Y. Moro-oka and M. Akita, Inorg. Chim. Acta, 2006, 359, 4407 CrossRef CAS
;
(d) M. S. Seo, J. Y. Kim, J. Annaraj, Y. Kim, Y.-M. Lee, S.-J. Kim, J. Kim and W. Nam, Angew. Chem., Int. Ed., 2007, 46, 377 CrossRef CAS PubMed
;
(e) J. Annaraj, J. Cho, Y.-M. Lee, S. Y. Kim, R. Latifi, S. P. de Visser and W. Nam, Angew. Chem., Int. Ed., 2009, 48, 4150 CrossRef CAS PubMed
;
(f) H. Kang, J. Cho, K.-B. Cho, T. Nomura, T. Ogura and W. Nam, Chem.–Eur. J., 2013, 19, 14119 CrossRef CAS PubMed
;
(g) H. E. Colmer, R. A. Geiger, D. F. Leto, G. B. Wijeratne, V. W. Day and T. A. Jackson, Dalton Trans., 2014, 43, 17949 RSC
.
- Examples of isolated peroxomanganese(IV) species:
(a) U. Bossek, T. Weyhermueller, K. Wieghardt, B. Nuber and J. Weiss, J. Am. Chem. Soc., 1990, 112, 6387 CrossRef CAS
;
(b) S. H. Kim, H. Park, M. S. Seo, M. Kubo, T. Ogura, J. Klajn, D. T. Gryko, J. S. Valentine and W. Nam, J. Am. Chem. Soc., 2010, 132, 14030 CrossRef CAS PubMed
.
-
(a) C.-M. Lee, C.-H. Chuo, C.-H. Chen, C. C. Hu, M. H. Chiang, Y.-J. Tseng, C.-H. Hu and G.-H. Lee, Angew. Chem., Int. Ed., 2012, 124, 5523 CrossRef
;
(b) S. Hong, K. D. Sutherlin, J. Park, E. Kwon, M. A. Siegler, E. I. Solomon and W. Nam, Nat. Commun., 2014, 5, 5440 CrossRef CAS PubMed
.
-
(a) N. M. Senozan, J. Chem. Educ., 1974, 51, 503 CrossRef CAS PubMed
;
(b) E. C. Neiderhoffer, J. H. Timmons and A. E. Martell, Chem. Rev., 1984, 84, 137 CrossRef
.
-
(a) I. Tabushi and N. Koga, J. Am. Chem. Soc., 1979, 101, 6456 CrossRef CAS
;
(b) I. Tabushi and A. Yazaki, J. Am. Chem. Soc., 1981, 103, 7371 CrossRef CAS
;
(c) M. Perrée-Fauvet and A. Gaudemer, J. Chem. Soc., Chem. Commun., 1981, 874 RSC
;
(d) D. Mansuy, M. Fontecave and J.-F. Bartoli, J. Chem. Soc., Chem. Commun., 1983, 253 RSC
;
(e) M. Fontecave and D. Mansuy, Tetrahedron, 1984, 40, 4297 CrossRef CAS
;
(f) S. E. Creager, S. A. Raybuck and R. W. Murray, J. Am. Chem. Soc., 1986, 108, 4225 CrossRef CAS
;
(g) S. E. Creager and R. W. Murray, Inorg. Chem., 1987, 26, 2612 CrossRef CAS
;
(h) P. Battioni, J. F. Bartoli, P. Leduc, M. Fontecave and D. Mansuy, J. Chem. Soc., Chem. Commun., 1987, 791 RSC
;
(i) I. Tabushi, Coord. Chem. Rev., 1988, 86, 1 CrossRef CAS
;
(j) H. Sakurai, Y. Mori and M. Shibuya, Inorg. Chim. Acta, 1989, 162, 23 CrossRef CAS
;
(k) H. Nishihara, K. Pressprich, R. W. Murray and J. P. Collman, Inorg. Chem., 1990, 29, 1000 CrossRef CAS
.
-
(a) C. J. Weschler, B. M. Hoffman and F. Basolo, J. Am. Chem. Soc., 1975, 97, 5278 CrossRef CAS PubMed
;
(b) B. Gonzalez, J. Kouba, S. Yee, C. A. Reed, J. F. Kirner and W. R. Scheidt, J. Am. Chem. Soc., 1975, 97, 3247 CrossRef CAS
;
(c) B. M. Hoffman, C. J. Weschler and F. Basolo, J. Am. Chem. Soc., 1976, 98, 5473 CrossRef CAS PubMed
;
(d) A. Dedieu and M. M. Rohmer, J. Am. Chem. Soc., 1977, 99, 8050 CrossRef CAS
;
(e) B. M. Hoffman, T. Szymanski, T. G. Brown and F. Basolo, J. Am. Chem. Soc., 1978, 100, 7253 CrossRef CAS
;
(f) G. D. Lawrence and D. T. Sawyer, Coord. Chem. Rev., 1978, 27, 173 CrossRef CAS
;
(g) R. D. Jones, D. A. Summerville and F. Basolo, J. Am. Chem. Soc., 1978, 100, 4416 CrossRef CAS
;
(h) E. B. Fleischer and R. V. Ferra, J. Inorg. Biochem., 1979, 10, 91 CrossRef CAS
;
(i) W. M. Coleman and L. T. Taylor, Coord. Chem. Rev., 1980, 32, 1 CrossRef CAS
;
(j) L. K. Hanson and B. M. Hoffman, J. Am. Chem. Soc., 1980, 102, 4602 CrossRef CAS
;
(k) A. Shirazi and H. M. Goff, J. Am. Chem. Soc., 1982, 104, 6318 CrossRef CAS
;
(l) M. W. Urban, K. Nakamoto and F. Basolo, Inorg. Chem., 1982, 21, 3406 CrossRef CAS
;
(m) A. Weselucha-Birczynska, L. M. Proniewicz, K. Bajdor and K. Nakamoto, J. Raman Spectrosc., 1991, 22, 315 CrossRef CAS
;
(n) V. L. Pecoraro, M. J. Baldwin and A. Gelasco, Chem. Rev., 1994, 94, 807 CrossRef CAS
;
(o) T. S. Kurtikyan, T. H. Stepanyan, G. G. Martirosyan, R. K. Kazaryan and V. N. Madakyan, Russ. Chem. Bull., 2000, 49, 1540 CrossRef CAS
.
-
(a) A. B. Hoffman, D. M. Collins, V. W. Day, E. B. Fleischer, T. S. Strivastava and J. L. Hoard, J. Am. Chem. Soc., 1972, 94, 3620 CrossRef CAS PubMed
;
(b) D.-H. Chin, G. N. La Mar and A. L. Balch, J. Am. Chem. Soc., 1980, 102, 4344 CrossRef CAS
;
(c) G. C. Dismukes, J. E. Sheats and J. A. Smegal, J. Am. Chem. Soc., 1987, 109, 7202 CrossRef CAS
.
- Iron:
(a) G. B. Jameson, G. A. Rodley, W. T. Robinson, R. R. Gagne, C. A. Reed and J. P. Collman, Inorg. Chem., 1978, 17, 850 CrossRef CAS
;
(b) G. B. Jameson, F. S. Molinaro, J. A. Ibers, J. P. Collman, J. I. Brauman, E. Rose and K. S. Suslick, J. Am. Chem. Soc., 1980, 102, 3224 CrossRef CAS
;
(c) M. Schnappcher, L. Ricard, J. Fischer, R. Weiss, E. Bill, R. Montiel-Montoya, H. Winkler and A. X. Trautwein, Eur. J. Biochem., 1987, 168, 419 CrossRef
;
(d) J. Li, B. C. Noll, A. G. Oliver, C. E. Schulz and W. R. Scheidt, J. Am. Chem. Soc., 2013, 135, 15627 CrossRef CAS PubMed
. Cobalt:
(e) P. Doppelt, J. Fischer, L. Ricard and R. Weiss, New J. Chem., 1987, 11, 357 CAS
;
(f) J. Li, B. C. Noll, A. G. Oliver and W. R. Scheidt, J. Am. Chem. Soc., 2012, 134, 10595 CrossRef CAS PubMed
.
- D. Feng, W.-C. Chung, Z. Wei, Z. Y. Gu, H.-L. Jiang, Y.-P. Chen, D. J. Darensbourg and H.-C. Zhou, J. Am. Chem. Soc., 2013, 135, 17105 CrossRef CAS PubMed
.
- J. S. Anderson, A. T. Gallagher, J. A. Mason and T. D. Harris, J. Am. Chem. Soc., 2014, 136, 16489 CrossRef CAS PubMed
.
- A. T. Gallagher, M. L. Kelty, J. G. Park, J. S. Anderson, J. A. Mason, J. P. S. Walsh, S. L. Collins and T. D. Harris, Inorg. Chem. Front., 2016, 3, 536 RSC
.
- A. T. Gallagher, C. D. Malliakas and T. D. Harris, Inorg. Chem., 2017, 56, 4654 CrossRef PubMed
.
- J. M. Zadrozny, A. T. Gallagher, T. D. Harris and D. E. Freedman, J. Am. Chem. Soc., 2017, 139, 7089 CrossRef CAS PubMed
.
-
(a) X.-L. Lv, K. Wang, B. Wang, J. Su, X. Zou, Y. Xie, J.-R. Li and H.-C. Zhou, J. Am. Chem. Soc., 2017, 139, 211 CrossRef CAS PubMed
;
(b) J. W. Brown, Q. T. Nguyen, T. Otto, N. N. Jarenwattananon, S. Glöggler and L.-S. Bouchard, Catal. Commun., 2015, 59, 50 CrossRef CAS
;
(c) K. S. Suslick, P. Bhyrappa, J.-H. Chou, M. E. Kosal, S. Nakagaki, D. W. Smithenry and S. R. Wilson, Acc. Chem. Res., 2005, 38, 283 CrossRef CAS PubMed
;
(d) D. H. Lee, S. Kim, M. Y. Hyun, J.-Y. Hong, S. Huh, C. Kim and S. J. Lee, Chem. Commun., 2012, 48, 5512 RSC
;
(e) Z. Guo, D. Yan, H. Wang, D. Tesfagaber, X. Li, Y. Chen, W. Huang and B. Chen, Inorg. Chem., 2015, 54, 200 CrossRef CAS PubMed
;
(f) M.-H. Xie, X.-L. Yang, Y. He, J. Zhang, B. L. Chen and C.-D. Wu, Chem.–Eur. J., 2013, 19, 14316 CrossRef CAS PubMed
;
(g) C. Zhou, T. Zhang, M.-H. Xie, J. Yan, G.-Q. Kong, X.-L. Yang, A. Ma and C.-D. Wu, Inorg. Chem., 2013, 52, 3620 CrossRef PubMed
;
(h) P. M. Barron, H.-T. Son, C. Hu and W. Choe, Cryst. Growth Des., 2009, 9, 1960 CrossRef CAS
;
(i) W. Zhang, P. Jiang, Y. Wang, J. Zhang, J. Zheng and P. Zhang, Chem. Eng. J., 2014, 257, 28 CrossRef CAS
.
-
(a) K. S. Suslick and R. A. Watson, New J. Chem., 1992, 16, 633 CAS
;
(b)
D. Dolphin, in Physical Chemistry, Academic Press, New York, 1978, vol. III, Part C Search PubMed
.
-
(a) Iron: K. Nakamoto, T. Watanabe, T. Ama and M. W. Urban, J. Am. Chem. Soc., 1982, 104, 3744 CrossRef CAS
;
(b) Cobalt: M. Kozuka and K. Nakamoto, J. Am. Chem. Soc., 1981, 103, 2162 CrossRef CAS
.
- R. Guilard, M. Fontesse and P. Fournari, J. Chem. Soc., Chem. Commun., 1976, 161 RSC
.
- J. F. Kirner, C. A. Reed and W. R. Scheidt, J. Am. Chem. Soc., 1977, 99, 1093 CrossRef PubMed
.
- J. S. Valentine, Chem. Rev., 1973, 73, 235 CrossRef CAS
; L. Vaska, Acc. Chem. Res., 1976, 9, 175 CrossRef
.
- B. Chevrier, T. Diebold and R. Weiss, Inorg. Chim. Acta, 1976, 19, 57 CrossRef
.
- A peroxo-bridged MnIV2 complex: U. Bossek, T. Weyhermueller, K. Wieghardt, B. Nuber and J. Weiss, J. Am. Chem. Soc., 1990, 112, 6387 CrossRef CAS
.
-
(a) J. M. Assour, J. Chem. Phys., 1965, 43, 2477 CrossRef CAS
;
(b) F. A. Walker, J. Am. Chem. Soc., 1970, 92, 4235 CrossRef CAS
;
(c) S. Van Doorslaer and A. Schweiger, Phys. Chem. Chem. Phys., 2001, 3, 159 RSC
.
-
(a)
D. Dolphin, Physical Chemistry, in The Porphyrins Volume V, Academic Press, New York, Part C, 1878 Search PubMed
;
(b) L. H. Vogt Jr, H. M. Faigenbaum and S. E. Wiberley, Chem. Rev., 1963, 63, 269 CrossRef
.
Footnotes |
† Electronic supplementary information (ESI) available: Additional experimental, spectroscopic, gas adsorption, and crystallographic data. Crystallographic information files for 1 and 2 can be obtained from the Cambridge Structural Database. CCDC 1570584 and 1570585. For ESI and crystallographic data in CIF or other electronic format see DOI: 10.1039/c7sc03739b |
‡ Current address: Department of Chemistry, University of Chicago, 929 E. 57th Street, Chicago, IL 60637-1454, USA. |
|
This journal is © The Royal Society of Chemistry 2018 |