DOI:
10.1039/C7MT00249A
(Paper)
Metallomics, 2018,
10, 145-153
Arsenolipid biosynthesis by the unicellular alga Dunaliella tertiolecta is influenced by As/P ratio in culture experiments†
Received
24th August 2017
, Accepted 11th December 2017
First published on 12th December 2017
Abstract
The influence of arsenate and phosphate levels in water on the formation of arsenic-containing lipids (arsenolipids) and water-soluble arsenicals by a unicellular marine alga was investigated by exposing Dunaliella tertiolecta to five regimes of arsenic and phosphate, and determining the biosynthesized organoarsenicals with HPLC/mass spectrometry. Under all conditions, the major arsenolipid produced by D. tertiolecta was the novel phytyl 5-dimethylarsinoyl-2-O-methyl-ribofuranoside (AsSugPhytol546) representing ca. 35–65% of total arsenolipids. The new compound contains a phytol aglycone and a methoxy group replacing a sugar hydroxyl – two structural features not previously observed for arsenolipids. Minor arsenolipids were several previously reported arsenosugar phospholipids (AsSugPLs, in particular AsSugPL958 and the previously unknown AsSugPL978), the relative quantities of which increased with increasing phosphate exposure, and an arsenic-containing hydrocarbon (AsHC360), which remained unaffected by the different treatments. The relative amount of total arsenolipids produced by D. tertiolecta remained remarkably constant (ca. 45% of total As) and independent of the culture conditions. In contrast, with rising As-concentrations we observed an increase of hydrophilic arsenicals, which were dominated by arsenate and arsenosugars. The results highlight a possible major difference in arsenic biochemistry between macroalgae and unicellular algae with potential implications for how various algae handle their natural arsenic exposure in the world's oceans.
Significance to metallomics
Biogeochemical pathways of arsenic compounds in marine ecosystems remain largely unknown. In particular the role of the recently discovered arsenic-containing lipids (arsenolipids) in the ocean's As-cycle is poorly understood. We conducted laboratory culture experiments with unicellular algae, which represent the base of the marine food chain, exposing them to varying arsenate/phosphate regimes and determining their arsenometallomes by HPLC/mass spectrometry. The results show the interconnection of arsenolipids and water-soluble arsenicals with environmental factors such as nutrient availability and inorganic arsenate exposure.
|
Introduction
The presence of lipid-soluble arsenicals (arsenolipids) in marine organisms was first reported in the 1920s,1 but only in the last ten years have the structures and diversity of these compounds been revealed. The various forms of arsenolipids include arsenic incorporated into fatty acids,2–4 hydrocarbons,5–7 long chain alcohols,8 glycophospholipids,9,10 and phosphatidylcholines.11 At least 70 arsenolipids have been identified so far, and the expectation is that many more await discovery. These arsenic species are of interest to human health because they are present in many common seafoods12 and some of them are cytotoxic to human cells.13,14
How arsenolipids are biosynthesized remains largely unknown, but it is likely that marine algae are the origin of these compounds. In seawater, arsenic is typically present mainly as arsenate at concentrations of 1–2 μg As L−1.15 Marine algae accumulate arsenate from seawater and metabolize it into various organoarsenic species. The uptake of arsenate is thought to take place through transport processes designed for the essential nutrient phosphate, and competitive inhibition of phosphate uptake in algae by arsenate has been reported.16 The major organoarsenic species formed by algae are usually water-soluble arsenosugars, but several studies have demonstrated that a considerable proportion of algal arsenic is lipid-soluble.17–19
To investigate metabolic pathways underlying arsenic transformations, marine unicellular algae have been cultured under various nutrient and arsenic regimes.17,20,21 In some algal species, more than half of the total arsenic was present as lipid-soluble forms, which, in the case of Dunaliella tertiolecta, is likely to have hydrolyzed to mainly glycerol- and phosphate-arsenosugars.17–19,21,22 Studies on arsenic transformation have also been performed with macro-algae. Geiszinger et al.23 showed that the brown alga Fucus serratus produced several arsenosugars when exposed to low arsenate concentrations, but in that study arsenolipids were not investigated. Petursdottir et al.24 examined the arsenolipids formed by the marine seaweed Ectocarpus cultured under environmentally relevant nutrient levels and several conditions designed to stress the organisms. Low phosphate treatments led to downregulation of phosphate arsenosugar production but resulted in an upregulation of arsenosugar phospholipid production, and only when arsenate was in excess did transformation into arsenic-containing hydrocarbons occur. It was hypothesized that low phosphate concentrations stimulated phosphate transport, and arsenate was accidentally co-transported to the mitochondria where it then led to the increased production of arsenosugar phospholipids.
Unicellular marine algae contribute strongly to global carbon fixation, accounting for more than 70% of the total primary production in marine ecosystems.25 Marine environments across the globe are steadily changing due to the effects of climate change, demographic growth and progressive industrialization. Although environmental variables (e.g. P availability) have been shown to influence concentrations and proportions of water-soluble As species in unicellular algae, the effects on lipid-soluble species are unknown.
We cultured the marine phytoplankton Dunaliella tertiolecta under five arsenate/phosphate regimes, and followed the transformation of arsenic into water- and lipid-soluble species (Fig. 1) by using HPLC coupled to elemental and molecular mass spectrometry. The results are discussed in terms of phosphate requirements and possible utilization of arsenate by the alga.
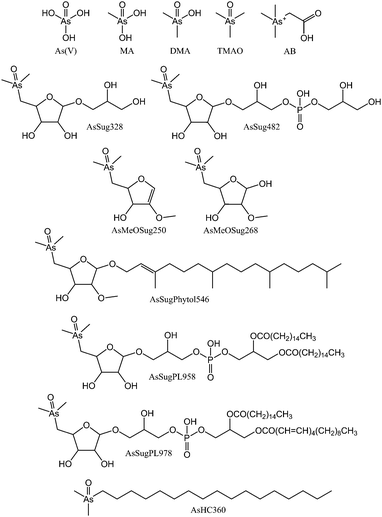 |
| Fig. 1 Arsenic species found in algae and referred to in this study (isomeric configuration of AsSugPhytol546, and double bond positions of AsSugPL978 were not determined). | |
Experimental
Chemicals and standards
Deionized water was obtained from a Milli-Q system (18.2 MΩ cm, Millipore GmbH, Vienna, Austria). Dichloromethane (≥99.9%, DCM) and ethanol (≥99.9%, EtOH) were obtained from VWR (Vienna, Austria); silica gel 60 from Merck (Buchs, Switzerland); formic acid (≥98%), aqueous ammonia (25% NH3), and ammonium formate (≥95%) from Carl Roth GmbH (Karlsruhe, Germany); acetone (≥99.8%) from Chem-Lab (Zedelgem, Belgium); and malonic acid (99%) from Sigma-Aldrich (Vienna, Austria). Saturated hydrogen sulfide solution was prepared by bubbling H2S gas through EtOH (50 mL) for 10 min. Arsenic(V) standard solution (1000 ± 5 mg As L−1) was obtained from Merck; and germanium, indium, and tellurium standard solutions (1000 ± 2 mg L−1 each) from Carl Roth. Two certified reference materials were used: NMIJ CRM 7405-a (Trace Elements and Arsenic Compounds in Seaweed – Hijiki) from the National Metrology Institute of Japan (Tsukuba, Ibaraki, Japan), and the NIST SRM 1640a (Trace Elements in Natural Water) from the National Institute of Standards and Technology (Gaithersburg, Maryland, USA). Six arsenolipids used as standards, namely AsFA362, AsFA388, AsFA418, AsHC332, AsHC360, and AsHC444 were synthesized in-house according to Taleshi et al.26 (Fig. S1, ESI†) and standard solutions of these species, each at 7.5 mg As L−1, were prepared in EtOH. Additionally, arsenolipids AsSugPL958, AsSugPL986, AsSugPL1014, AsSugPL1042, and AsSugPL1070, which are present in the NMIJ CRM 7405-a, were used as reference species (Fig. S2, ESI†). Water-soluble arsenic standards methylarsonic acid (MA), dimethylarsinic acid (DMA), arsenobetaine (AB), trimethylarsine oxide (TMAO), arsenocholine (AC), tetramethylarsonium ion (TETRA), 3-[5′-deoxy-5′-(dimethylarsinoyl)-β-ribofuranosyloxy]-2-hydroxypropylene glycol (AsSug328, glycerol arsenosugar), and 3-[5′-deoxy-5′-(dimethylarsinoyl)-β-ribofuranosyloxy]-2-hydroxypropyl-2,3-hydroxypropyl phosphate (AsSug482, phosphate arsenosugar) were prepared as previously reported.27
Instrumentation
Solvents were evaporated on a centrifugal lyophilizer (Christ RVC 2-33 CD plus, Martin Christ GmbH, Osterode am Hartz, Germany). Separation of arsenic species was carried out with an Agilent 1100 series HPLC system prior to online elemental determination with either an Agilent 7500ce (reversed-phase separation) or 7900 (cation- and anion-exchange separations) series instrument (Agilent Technologies, Waldbronn, Germany). The Agilent 7500ce instrument was equipped with an Ari Mist HP nebulizer (Burgerner, Mississauga, Canada), an ESI PC3 Peltier-cooled cyclonic spray chamber (Elemental Scientific, Omaha, USA), and Pt interface cones; the Agilent 7900 instrument was equipped with a MicroMist concentric glass nebulizer (Glass Expansion, West Melbourne, Australia) and a Peltier cooled Scott-type double-pass spray chamber with the standard Ni interface cones. High Resolution-ESMS measurements were performed on a Q-Exactive Hybrid Quadrupole-Orbitrap MS after HPLC on a Dionex Ultimate 3000 series instrument (Thermo Fisher Sci., Erlangen, Germany). The HR-ESMS was furnished with an atmospheric pressure electrospray ionization source using nitrogen as nebulizer and drying gas. Measurements were performed in positive or negative mode, with a drying gas temperature of 350 °C, spray voltage of 3.2 kV, and a resolution of 70
000 unless otherwise stated. The observed mass range was set to m/z 300–1100 with data dependent fragmentation at various normalized collision energies (NCE: 10–100) and subsequent recording of MS/MS fragment ions.
Culturing Dunaliella tertiolecta under various As/P regimes
Axenic cultures of the marine unicellular alga Dunaliella tertiolecta were obtained from CSIRO (Lucas Heights Science and Technology Centre, NSW, Australia). D. tertiolecta cultures were maintained in sterile polypropylene tubes (50 mL) containing 30 mL of autoclave-sterilized and filtered (0.2 μm) f/10 seawater culture medium.28 F/10 media was prepared under aseptic conditions in a laminar flow hood (Gelaire, BSB-12, Sydney, Australia) with the exception that phosphate concentrations were 0.85 mg L−1 (= 8950 nM P). Algal stock cultures were incubated in environmental chambers (3504 process controller, Eurotherm, Australia) with a light dark regime of 12
:
12 h, maintaining light intensity at ca. 110 μmol photons m−2 s−1 (ca. 6000 lux) and an air temperature of 25 °C during light periods and 20 °C during dark periods. Salinity was maintained at 35‰. Stock cultures were tested for microbial contamination by applying them to marine peptone yeast extract agar (PYEA) plates, which were incubated for seven days under the same conditions as the cultures and then examined for signs of microbial growth.17 Each week, D. tertiolecta stock culture (1 mL) was transferred to fresh f/10 seawater medium, prepared as described above, to maintain viable cells over time.
Batch cultures were prepared by transferring the stock culture (30 mL) to sterilized glass flasks (5 L) containing 3 L of sterile 0.2 μm filtered f/10 seawater media. Three phosphate treatments were used in the experiment: (i) no added phosphate, <0.05 mg phosphate L−1 (ii) low – 0.1 mg phosphate L−1 (ca. 1050 nM P), and (iii) high – 0.85 mg phosphate L−1 (ca. 8950 nM). After preparation of the media, a portion (20 mL) of each batch culture was tested for microbial contamination as described earlier, and to determine cell growth over time. Batches were incubated for one week under the conditions described above; each day, the culture flasks were shaken to ensure mixing, and placed in the environmental chambers in a randomized design. After 72 hours, filtered (0.2 μm) arsenate stock solutions (0.5 mL of either 0.4 or 1.2 mM) were added to the phosphate-containing batches (ii and iii) giving final concentrations of 5.0 μg As L−1 (ca. 67 nM As) or 15 μg As L−1 (ca. 200 nM As) in the culture media. In total, five sets of As/P conditions for culturing the alga, each replicated seven times (n = 7), were utilized in the experiments (Table 1). After seven days of incubation, D. tertiolecta cells were harvested by centrifugation at 4500 g at 20 °C for 10 min (Eppendorf centrifuge 5804, Hamburg, Germany), and algal tissues were freeze-dried (Labconco 77540 12L, Kansas City, KS, USA) and stored at 4 °C before analysis.
Table 1 Arsenate/phosphate culturing conditions for Dunaliella tertiolecta (As/P added to f/10 media28). Phosphate was added at t = 0 hours; arsenate at = 72 h (n = 7)
Regime |
Arsenate (nM) |
Phosphate (nM) |
No added As/P |
<20 |
<500 |
Low As/low P |
67 |
1050 |
High As/low P |
200 |
1050 |
Low As/high P |
67 |
8950 |
High As/high P |
200 |
8950 |
Determination of cell growth at various As/P regimes
The growth of D. tertiolecta was evaluated by applying a portion of the culture (10 μL) onto a haemocytometer slide and counting cells with a phase contrast microscope (Olympus model CHS, Tokyo, Japan) as described by Foster et al.17
Sample extraction procedure
The following procedures were carried out with the harvested freeze-dried D. tertiolecta cells cultured under five As/P regimes (each n = 7) and CRM 7405-a (n = 4) using 10 mg of dry sample weighed (to a precision of 0.01 mg) directly into polypropylene tubes (15 mL; Greiner Bio-One, Kremsmuenster, Austria).
Water-soluble arsenicals.
The freeze-dried algal sample (10 mg) was extracted with water (2.5 mL) for 1 hour on a rotary cross at room temperature. Then the mixture was centrifuged (10 min, 2000g) and the supernatant was transferred to a new tube. This process was repeated with the wet pellet; the two supernatants were combined, evaporated to dryness (10 mbar, 25 °C), and stored (−20 °C). The residue was re-dissolved in water (200 μL) prior to measurement by HPLC/mass spectrometry.
Arsenolipids.
The sample preparation was based on the method for determining arsenolipids in macroalgae.29 The freeze-dried algal sample was extracted with DCM/MeOH (2 + 1, v/v; 3 mL) for 1 hour on a rotary cross at room temperature. The mixture was centrifuged (10 min, 2000g) and a portion of the liquid phase (2.7 mL) was transferred directly onto a silica column (glass Pasteur pipette, 230 × 5 mm, filled to a height of 4 cm with silica gel), conditioned with MeOH/acetone (1 + 1, v/v, containing 1% v/v formic acid, 3 mL). The column was washed with the same mixture (3 mL) and then with pure MeOH (2 mL). Finally arsenolipids were eluted with MeOH containing 1% v/w NH3 (8 mL); H2S (in EtOH, 100 μL) was added to the alkaline fraction before it was evaporated to dryness (10 mbar, 25 °C), and the residue stored at −20 °C. The residue was re-dissolved in EtOH/water (1 + 1, v/v; 200 μL) prior to measurement.
Determination of water-soluble and lipid-soluble arsenic species
Water-soluble arsenic species were determined by a combination of cation- and anion-exchange HPLC coupled to ICPMS or HR-ESMS. Separation of cationic arsenicals was carried out on either an Agilent IonoSpher 5C (4.6 × 100 mm, 5 μm particle size) or a ZORBAX 300-SCX (4.6 × 150 mm, 5 μm particle size) cation-exchange column using aqueous pyridine buffer (10 mM, pH 2.6) or aqueous ammonium formate buffer (20 mM, pH 2.6) including 3% MeOH (v/v), respectively. Arsenical anions were separated on a Hamilton PRP-X100 (4.6 × 150 mm, 5 μm particle size) HPLC column. Elution was carried with an aqueous malonate buffer (5 mM, pH 5.6). All ion-exchange HPLC was carried out at 30 °C with an injection volume of 20 μL and at a flow rate of 0.8 mL min−1.
ICPMS signals were monitored at m/z 75 (75As and 40Ar35Cl), m/z 77 (40Ar37Cl, as a check for possible chloride interferences), and 53 (40Ar13C, monitoring carbon content in the plasma) at dwell times of 300, 100, and 50 ms, respectively. Carbon enhancement of arsenic signals during ICPMS measurements was effected by addition of 12% v/v optional gas comprising 1% v/v CO2 in argon. Internal standards were recorded at m/z 74 (74Ge) and m/z 125 (125Te) at dwell times of 100 ms. ESMS data were recorded in positive and negative scan mode with data dependent MS/MS recording at m/z 300–1100. Normalized collision energy was set to 50 instrument units for MS/MS measurements unless otherwise stated.
Separation of arsenolipid species was carried out by reversed-phase HPLC using a ZORBAX Eclipse XDB-C8 column (4.6 × 150 mm, 5 μm particle size). Elution was performed with water containing 1% v/v formic acid (mobile phase A) and EtOH containing 1% v/v formic acid (mobile phase B) under gradient elution conditions: 0–30 min, 50–100% B; 30–35 min, 100% B; 35–35.1 min, 100–50% B; and 35.1–42 min, 50% B. Flow rate was 0.8 mL min−1, column temperature was set to 30 °C, and injection volume was 40 μL for ICPMS and 10 μL for HR-ESMS. For ICPMS detection, the HPLC effluent was split whereby 20% was transported to the ICPMS and 80% to waste (to maintain a stable plasma by reducing the organic solvent load) using a passive splitter (Analytical Scientific Instruments, Richmond, USA) and post-splitter support of the outflow with water containing 1% v/v formic acid and 10 μg L−1 Ge and Te (0.8 mL min−1) through a T-piece. Carbon compensation30 was performed by constant introduction of an aqueous EtOH solution (4 + 1, v/v) directly into the spray chamber delivered with an ISIS pump (0.02 rpm) to ensure constant carbon content reaching the plasma. Lipid extracts were measured on an Agilent 7500ce instrument. For HR-ESMS detection, the HPLC effluent was directly introduced into the ion source without splitting.
A summary of the techniques and parameters is given in Table S1 (ESI†). Data evaluation of ICPMS measurements was carried out with chromatographic software MassHunter B.01.01 from Agilent Technologies (Waldbronn, Germany), and HR-ESMS data were evaluated with chromatographic software Xcalibur 3.0.63 from Thermo Scientific (San Jose, USA). Quantification was based on peak areas and external calibration with standards of arsenate and arsenobetaine for water-soluble arsenic species, and standards of AsHC332, AsHC360, and AsHC444 for lipid-soluble arsenicals.
Statistical analysis
A one-way ANOVA with least significant difference post-hoc test was performed to test differences between the means of treatments. Significance levels tested were: * p < 0.05; ** p < 0.01; or *** p < 0.001.
Results and discussion
Cell growth at various As/P regimes
Dunaliella tertiolecta, cultured under five As/P regimes, showed increased biomass when higher amounts of phosphate were present (Table 2), a result consistent with findings from a previous study.17 Higher amounts of arsenate (up to 200 nM, 15 μg As L−1), however, had no apparent influence on biomass.
Table 2
Dunaliella tertiolecta biomass and cell counts for the different As/P batch culture regimes (total volume of each culture medium was 3 L; means ± standard deviation; n = 4; cell growth was not measured in the remaining three replicates). Statistically significant differences from control (no added As or P) are depicted as * p < 0.05; ** p < 0.01; or *** p < 0.001
Regime |
Cells × 104 [mL−1] |
Biomass [mg] |
Day 7 |
Day 7 |
No added As or P |
17 ± 4 |
40 ± 5 |
Low As/low P |
35 ± 7 |
70 ± 10** |
High As/low P |
37 ± 5 |
70 ± 10** |
Low As/high P |
57 ± 10 |
95 ± 5*** |
High As/high P |
60 ± 9 |
95 ± 5*** |
As transformation under various As/P regimes
Extraction efficiency and arsenic distribution.
The total arsenic content in D. tertiolecta showed a trend towards higher As-concentrations at elevated arsenate exposure, but the As-concentrations were not greatly influenced by phosphate exposure (Fig. 2). The total extractable arsenic (hydrophilic plus lipophilic) followed this same trend, but it was more marked. Thus, when expressed as a percentage of total arsenic, the extractable arsenic increased from 65% (no added As or P) to 84% (low As/low P) to 96% (high As/low P) (Fig. 2). There were differences, however, between the hydrophilic and lipophilic extractions. With increasing arsenate exposure, water-soluble arsenic concentrations in D. tertiolecta increased from 2 to 10 μg As g−1, whereas added phosphate did not greatly influence this value. The percentage of water-soluble arsenic also increased (from 23 to 35 to 58% of total As) with increasing arsenate exposure, whereas different phosphate concentrations had no effect on this percentage. Both arsenate and phosphate had a marked effect on the type of water-soluble arsenic species produced by the alga (see below). The lipid As concentrations in D. tertiolecta showed a clear positive correlation with arsenate availability increasing from 4.0 to 6.3 to 8.1 μg As g−1 dry alga mass for cultures exposed to increasing concentrations of added arsenate. The phosphate concentration in the media had no significant effect on the production of arsenolipids by D. tertiolecta. The percentage contribution of lipid As to the total As content, however, remained remarkably constant at 46 ± 2% (w/w) over the five treatment regimes (Fig. 2).
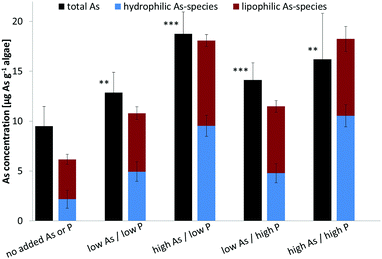 |
| Fig. 2 Total arsenic concentration and sum of extracted water-/lipid-soluble arsenicals in Dunaliella tertiolecta cultured under various As/P regimes (harvested after seven days; means ± standard deviation, n = 7). Statistically significant differences from control (no added As or P) are depicted as * p < 0.05; ** p < 0.01; or *** p < 0.001. | |
Water-soluble arsenicals in D. tertiolecta extracts.
A total of ten water-soluble arsenic species were detected in the unicellular algal cultures: inorganic As(V), dimethylarsinic acid DMA, arsenosugars AsSug328, AsSug482 and AsMeOSug250, one unknown arsenic species, and traces (≤0.1 μg As g−1 dry mass) of MA, TMAO, AB, and AsMeOSug268 (Fig. 3 and Table S2, ESI†). The two S-containing arsenosugars commonly found in macroalgae31 were not produced by D. tertiolecta under our culturing conditions.
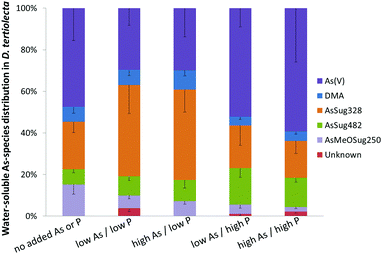 |
| Fig. 3 Relative distribution of major water-soluble arsenic species in Dunaliella tertiolecta cultured under various As/P regimes after seven days (mean values ± standard deviations of n = 7); MA, TMAO, AB, and AsMeOSug268 (not shown) were present in traces (≤0.1 μg As g−1 dry alga). | |
Arsenate was the major species when cultures were grown under background (no added As or P) and both high P regimes, representing about half of the total water-soluble As. At low P regimes, however, the proportion of arsenate was lower even though arsenate was added to the cultures. Under low P conditions, the glycerol arsenosugar AsSug328 was the major species suggesting that the alga could efficiently biosynthesize this arsenical at the tested exposure levels. The phosphate arsenosugar AsSug482 was also an abundant species but it had a pattern contrary to that of AsSug328 by showing higher amounts at the higher P conditions. The simple arsenical DMA and an unknown arsenic species were lesser constituents in D. tertiolecta, and their accumulation pattern was similar to that of AsSug328. The 2-O-methyl riboside AsMeOSug250 followed the pattern of the lipid-soluble AsSugPhytol546 (see below) but at ca. 10 fold lower concentrations and is likely either a degradation product, or a precursor of it.
Our results are substantially in agreement with previous studies17–19,22 and support the view that phosphate concentration influences arsenic uptake and speciation of arsenicals in water-soluble cell fractions of D. tertiolecta. We note, however, that the earlier study of Andreae and Klumpp32 with four species of marine unicellular algae indicated that phosphate concentrations did not influence the algae's cellular arsenic concentrations.
Arsenolipids in D. tertiolecta extracts.
Typical HPLC/ICPMS chromatograms of the various arsenolipids produced by D. tertiolecta are shown in Fig. 4; the quantitative and qualitative data appear in Tables S2 and S3 (ESI†), and an overview of these data showing relative distribution of the arsenolipids is presented in Fig. 5. The major species (33–67% of total arsenolipids) was a new type of arsenolipid recently identified as a phytyl 5-dimethylarsinoyl-2-O-methyl-riboside (AsSugPhytol546).33
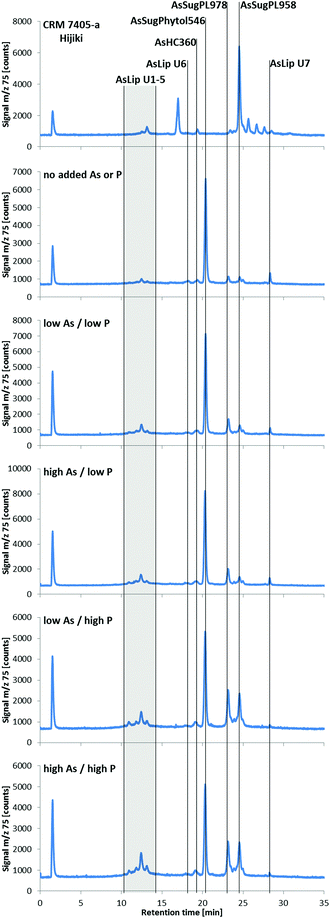 |
| Fig. 4 RP-HPLC-ICPMS chromatograms of lipid-soluble arsenic species in Dunaliella tertiolecta cultured under various As/P regimes after seven days (the CRM 7405-a Hijiki is also shown as a comparison). Samples were prepared following the method of Glabonjat et al.;29 the arsenicals were chromatographed as their thioxo derivatives. ZORBAX Eclipse XDB-C8 (4.6 × 150 mm, 5 μm); mobile phase, water/EtOH gradient (50–100% EtOH, incl. 0.1% formic acid); flow rate, 0.8 mL min−1; column temperature, 30 °C; injection volume, 40 μL. Representative chromatograms from each treatment are shown; each treatment had seven replicates. | |
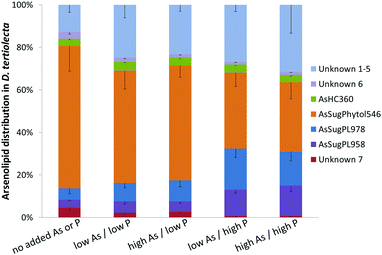 |
| Fig. 5 Relative distribution of lipid-soluble arsenic species in Dunaliella tertiolecta cultured under various As/P regimes after seven days (mean values ± standard deviations, n = 7). The small quantities of Unknowns 1–5, Unknown 6 and Unknown 7 precluded their identification. | |
Three known arsenolipids, namely AsHC360, AsSugPL958, and AsSugPL978 were also significant metabolites of D. tertiolecta accounting for 3–4%, 4–14%, and 5–18%, respectively, of the arsenolipids present, while smaller amounts of other arsenolipids were also present but could not be identified.
Although AsSugPhytol546 was the major arsenolipid under all culture conditions, it was, relative to the other arsenolipids, most dominant under background regimes. As a consequence of this behavior, the concentration of AsSugPhytol546, when going from low As to high As exposures, showed only modest (ca. 40%; p < 0.001) or no increases at low and high P regimes, respectively (Table S2, ESI†). The arsenic hydrocarbon AsHC360 showed similar behavior with P having no apparent effect on its biosynthesis by Dunaliella. In contrast, the arsenic phospholipid AsSugPL958 recorded almost 3-fold increases (p < 0.001) in concentration when going from low to high P exposure (for both low and high As regimes). The second arsenic phospholipid, AsSugPL978, behaved similarly with a 2.7-fold increase (p < 0.001) in concentration when going from low to high P exposure in the low As regimes, although the effect was much less marked (1.5-fold; p < 0.05) for the high As regimes.
An unexpected result from the D. tertiolecta culture experiments was the discovery of the new type of arsenolipid AsSugPhytol546, an arsenic-containing 2-O-methyl riboside bound to phytol. The observation that AsSugPhytol546 was particularly dominant under the culture regime with no added arsenate and phosphate suggests that this compound might also be a major arsenolipid in natural unicellular algal assemblages. The arsenic species has several novel structural features that have already been briefly discussed;33 a potential biosynthetic pathway is depicted in Fig. 6. Of particular relevance here is the incorporation of the phytol side chain; phytol provides the lipid anchor for chlorophyll-a enabling it to secure its place in the lipid membrane of chloroplasts,34 and possibly it serves a similar role for this major algal arsenic species as well. The AsSugPhytol546 accounted for 2.2 to 4.9 μg As g−1 dry alga mass and represented up to 67% (w/w) of all extracted arsenolipids. Despite several studies with macroalgae, the new arsenolipid AsSugPhytol546 has never been reported as a macroalgal metabolite, suggesting that it may be unique to unicellular algae. It is worth noting, however, that non-arsenic lipids based on ether linkages (a distinguishing feature of AsSugPhytol546) are more typically found in Archaea where the greater stability of the ether bond over an ester bond is thought to be beneficial for organisms adapted to harsher environments.
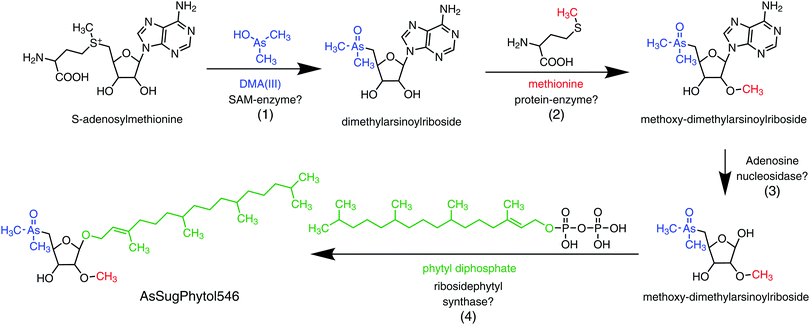 |
| Fig. 6 Possible pathway for the biosynthesis of AsSugPhytol546 in the marine unicellular alga Dunaliella tertiolecta: (1) substitution of methionine by DMA(III) in S-adenosylmethionine;40,41 (2) O-methylation in C2-position of the riboside by methionine;42,43 (3) hydrolytic removal of adenine;42,43 (4) condensation of 2-O-methylribose with phytyl diphosphate to the final arsenolipid (AsSugPhytol546).44,45 | |
The various arsenolipids produced by D. tertiolecta possibly reflect the needs of the organism regarding P and As in natural environments. The arsenic species, e.g. AsSugPhytol546, produced by the alga when no As or P is added might be those of some use to the alga. As we go to higher As/P regimes, other arsenolipids start to increase relative to AsSugPhytol546 (Fig. 7 and Table S2, ESI†), but perhaps these arsenic species are simply excess to the alga's biochemical requirements. If, on the other hand, all the arsenic species had increased at a similar rate with increasing As/P exposure, it might suggest that As was just attaching itself to whichever algal metabolite was available, with no biochemical involvement of the alga. An alternative, and less speculative, explanation could be that increasing As/P regimes lead to different amounts of the various metabolites, to which arsenic then attaches itself indiscriminately. Future research with mixed algal cultures and analysis of oceanic phytoplankton for arsenic species may shed light on the varying ways algae handle arsenic and the possible biological significance.
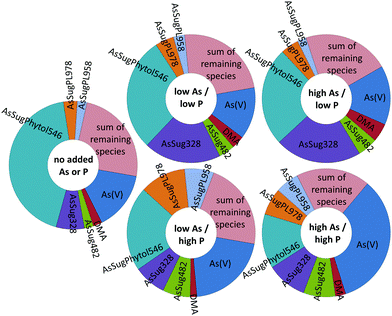 |
| Fig. 7 Relative abundance of the seven main arsenic species present in Dunaliella tertiolecta cultures at various As/P regimes (trace-minor As-species are depicted as the ‘sum of remaining species’). | |
An important aspect of our laboratory study was that the aqueous concentrations of phosphate and arsenate to which D. tertiolecta was exposed did not greatly exceed ambient environmental levels. Our maximum exposure levels were 8950 nM phosphate and 200 nM arsenate compared with typical oceanic phosphate and arsenate levels of 50–3000 nM and 10–40 nM, respectively. In contrast, past research on arsenic metabolites in marine unicellular algae was often conducted at very high As-concentrations, sometimes exceeding natural environmental levels by a factor of 1000–1
000
000.20,35–38 Furthermore, such studies endeavored to maximize algal growth by using culture media containing high concentrations of nutrients greatly exceeding natural levels.17,18,36–38 These chosen culture regimes can substantially influence the type and concentration of arsenic species produced by the algae.39 Thus, culture conditions that are too far removed from natural environmental conditions may be misleading to our understanding of the biogeochemical cycling of arsenic in the ocean.
Culture experiments performed under environmentally unrealistic conditions might also confound interpretation of studies investigating competitive uptake of P and As. Although it is often stated that arsenate enters algae through the phosphate uptake pathway, some studies have indicated separate uptake pathways for these two similar oxyanions.32 Further studies under environmentally relevant As/P levels are called for to explain these discordant observations.
In summary, we have shown that culture conditions influence the arsenic species produced by the marine unicellular alga Dunaliella tertiolecta, and we have identified patterns of accumulation depending on availability of arsenic and phosphate in the culture media. Further studies on the hierarchy and order of biosynthesis of the various arsenic species produced by D. tertiolecta may shed light on their relative importance. Such information might be revealed from time-resolved uptake experiments in combination with the measurement techniques described here, and we are currently pursuing this line of research.
Conflicts of interest
There are no conflicts to declare.
Acknowledgements
This research was supported by the Austrian Science Fund (FWF) project numbers 23761-N17 and I2412-B21. We also thank the EcoChemistry & Toxicology Lab in Canberra and NAWI Graz is thanked for supporting the Graz Central Lab – Environmental Metabolomics.
References
- E. Sadolin, Investigations into the occurrence of arsenic in the organism of fish, Biochem. Z., 1928, 201, 323–331 CAS.
- A. Rumpler, J. S. Edmonds, M. Katsu, K. B. Jensen, W. Goessler, G. Raber, H. Gunnlaugsdottir and K. A. Francesconi, Arsenic-containing long-chain fatty acids in cod-liver oil: A result of biosynthetic infidelity?, Angew. Chem., Int. Ed., 2008, 47, 2665–2667 CrossRef CAS PubMed.
- S. Lischka, U. Arroyo-Abad, J. Mattusch, A. Kuehn and C. Piechotta, The high diversity of arsenolipids in herring fillet (Clupea harengus), Talanta, 2013, 110, 144–152 CrossRef CAS PubMed.
- V. Sele, J. J. Sloth, B. Holmelid, S. Valdersnes, K. Skov and H. Amlund, Arsenic-containing fatty acids and hydrocarbons in marine oils – determination using reversed-phase HPLC–ICP-MS and HPLC–qTOF-MS, Talanta, 2014, 121, 89–96 CrossRef CAS PubMed.
- M. S. Taleshi, K. B. Jensen, G. Raber, J. S. Edmonds, H. Gunnlaugsdottir and K. A. Francesconi, Arsenic-containing hydrocarbons: natural compounds in oil from the fish capelin, Mallotus villosusw, Chem. Commun., 2008, 4706–4707 RSC.
- U. Arroyo-Abad, J. Mattusch, S. Mothes, M. Moeder, R. Wennrich, M. P. Elizalde-González and F.-M. Matysik, Detection of arsenic-containing hydrocarbons in canned cod liver tissue, Talanta, 2010, 82, 38–43 CrossRef CAS PubMed.
- V. Sele, H. Amlund, M. H. G. Berntssen, J. A. Berntsen, K. Skov and J. J. Sloth, Detection of arsenic-containing hydrocarbons in a range of commercial fish oils by GC-ICPMS analysis, Anal. Bioanal. Chem., 2013, 405, 5179–5190 CrossRef CAS PubMed.
- K. O. Amayo, A. Raab, E. M. Krupp, H. Gunnlaugsdottir and J. Feldmann, Novel identification of arsenolipids using chemical derivatizations in conjunction with RP-HPLC-ICPMS/ESMS, Anal. Chem., 2013, 85, 9321–9327 CrossRef CAS PubMed.
- S. García-Salgado, G. Raber, R. Raml, C. Magnes and K. A. Francesconi, Arsenosugar phospholipids and arsenic hydrocarbons in two species of brown macroalgae, Environ. Chem., 2012, 9, 63–66 CrossRef.
- A. Raab, C. Newcombe, D. Pitton, R. Ebel and J. Feldmann, Comprehensive analysis of lipophilic arsenic species in a brown alga (Saccharina latissima), Anal. Chem., 2013, 85, 2817–2824 CrossRef CAS PubMed.
- S. A. Viczek, K. B. Jensen and K. A. Francesconi, Arsenic-containing phosphatidylcholines: A new group of arsenolipids discovered in herring caviar, Angew. Chem., Int. Ed., 2016, 55, 5259–5262 CrossRef CAS PubMed.
- V. Taylor, B. Goodale, A. Raab, T. Schwerdtle, K. Reimer, S. Conklin, M. R. Karagas and K. A. Francesconi, Human exposure to organic arsenic species from seafood, Sci. Total Environ., 2016, 580, 266–282 CrossRef PubMed.
- S. Meyer, M. Matissek, S. M. Mueller, M. S. Taleshi, F. Ebert, K. A. Francesconi and T. Schwerdtle,
In vitro toxicological characterisation of three arsenic-containing hydrocarbons, Metallomics, 2014, 6, 1023–1033 RSC.
- S. Meyer, G. Raber, F. Ebert, L. Leffers, S. M. Mueller, M. S. Taleshi, K. A. Francesconi and T. Schwerdtle,
In vitro toxicological characterisation of arsenic-containing fatty acids and three of their metabolites, Toxicol. Res., 2015, 4, 1289–1296 RSC.
- M. O. Andreae, Distribution and speciation of arsenic in natural waters and some marine algae, Deep-Sea Res., 1978, 25, 391–402 CAS.
- J. J. Blum, Phosphate uptake by phosphate-starved Euglena, J. Gen. Physiol., 1966, 49, 1125–1137 CrossRef CAS PubMed.
- S. D. Foster, D. Thomson and W.
A. Maher, Uptake and metabolism of arsenate by anexic (sic) cultures of the microalgae Dunaliella tertiolecta and Phaeodactylum tricornutum, Mar. Chem., 2008, 108, 172–183 CrossRef CAS.
- E. Duncan, S. Foster and W. Maher, Uptake and metabolism of arsenate, methylarsonate and arsenobetaine by axenic cultures of the phytoplankton Dunaliella tertiolecta, Bot. Mar., 2010, 53, 377–386 CAS.
- E. G. Duncan, W. A. Maher, S. D. Foster and F. Krikowa, Influence of culture regime on arsenic cycling by the marine phytoplankton Dunaliella tertiolecta and Thalassiosira pseudonana, Environ. Chem., 2013, 10, 91–101 CrossRef CAS.
- S.-Y. Zhang, G.-X. Sun, X.-X. Yin, C. Rensing and Y.-G. Zhu, Biomethylation and volatilization of arsenic by the marine microalgae Ostreococcus tauri, Chemosphere, 2013, 93, 47–53 CrossRef CAS PubMed.
- E. G. Duncan, W. A. Maher, S. D. Foster, K. M. Mikac and F. Krikowa, The influence of bacteria on the arsenic species produced by laboratory cultures of the marine phytoplankton Dunaliella tertiolecta, J. Appl. Phycol., 2014, 26, 2129–2134 CrossRef CAS.
- E. G. Duncan, W. A. Maher, S. D. Foster and F. Krikowa, The influence of arsenate and phosphate exposure on arsenic uptake, metabolism and species formation in the marine phytoplankton Dunaliella tertiolecta, Mar. Chem., 2013, 157, 78–85 CrossRef CAS.
- A. Geiszinger, W. Goessler, S. N. Pedersen and K. A. Francesconi, Arsenic biotransformation by the brown macroalga Fucus serratus, Environ. Toxicol. Chem., 2001, 20, 2255–2262 CrossRef CAS PubMed.
- A. H. Petursdottir, K. Fletcher, H. Gunnlaugsdottir, E. Krupp, F. C. Kuepper and J. Feldmann, Environmental effects on arsenosugars and arsenolipids in Ectocarpus (Phaeophyta), Environ. Chem., 2015, 13, 21–33 CrossRef.
- J. A. Downing, Marine nitrogen: Phosphorus stoichiometry and the global N:P cycle, Biogeochemistry, 1997, 37, 237–252 CrossRef.
- M. S. Taleshi, R. K. Seidler-Egdal, K. B. Jensen, T. Schwerdtle and K. A. Francesconi, Synthesis and characterization of arsenolipids: naturally occurring arsenic compounds in fish and algae, Organometallics, 2014, 33, 1397–1403 CrossRef CAS PubMed.
- K. A. Francesconi, D. A. Hunter, B. Bachmann, G. Raber and W. Goessler, Uptake and transformation of arsenosugars in the shrimp Crangon crangon, Appl. Organomet. Chem., 1999, 13, 669–679 CrossRef CAS.
- R. R. L. Guillard and J. H. Ryther, Studies of marine planktonic diatoms: I. Cyclotella nana hustedt, and Detonula confervacea (cleve) Gran, Can. J. Microbiol., 1962, 8, 229–239 CrossRef CAS PubMed.
- R. A. Glabonjat, G. Raber, K. B. Jensen, J. Ehgartner and K. A. Francesconi, Quantification of arsenolipids in the certified reference material NMIJ 7405-a (Hijiki) using HPLC/mass spectrometry after chemical derivatization, Anal. Chem., 2014, 86, 10282–10287 CrossRef CAS PubMed.
- G. Raber, R. Raml, W. Goessler and K. A. Francesconi, Quantitative speciation of arsenic compounds when using organic solvent gradients in HPLC-ICPMS, J. Anal. At. Spectrom., 2010, 25, 570–576 RSC.
- K. A. Francesconi and J. S. Edmonds, Arsenic and marine organisms, Adv. Inorg. Chem., 1996, 44, 147–189 CrossRef.
- M. O. Andreae and D. Klumpp, Biosynthesis and release of organoarsenic compounds by marine algae, Environ. Sci. Technol., 1979, 13, 738–741 CrossRef CAS.
- R. A. Glabonjat, G. Raber, K. B. Jensen, N. Guttenberger, K. Zangger and K. A. Francesconi, A 2-O-methylriboside unknown outside the RNA world contains arsenic, Angew. Chem., Int. Ed., 2017, 56, 11963–11965 CrossRef CAS PubMed.
- J. M. Anderson, Possible location of chlorophyll within chloroplast membranes, Nature, 1975, 253, 536–537 CrossRef CAS.
- W. R. Cullen, L. G. Harrison, H. Li and G. Hewitt, Bioaccumulation and excretion of arsenic compounds by a marine unicellular alga, Polyphysa peniculus, Appl. Organomet. Chem., 1994, 8, 313–324 CrossRef CAS.
- O. Takimura, H. Fuse, K. Murakami, K. Kamimura and Y. Yamaoka, Uptake and reduction of arsenate by Dunaliella sp, Appl. Organomet. Chem., 1996, 10, 753–756 CrossRef CAS.
- T. Kaise, S. Fujiwara, M. Tsuzuki, T. Sakurai, T. Saitoh and C. Mastubara, Accumulation of arsenic in a unicellular alga Chlamydomonas reinhardtii, Appl. Organomet. Chem., 1999, 13, 107–111 CrossRef CAS.
- Y. Yamaoka, O. Takimura, H. Fuse and K. Murakami, Effect of glutathione on arsenic accumulation by Dunaliella salina, Appl. Organomet. Chem., 1999, 13, 89–94 CrossRef CAS.
- E. G. Duncan, W. A. Maher and S. D. Foster, Contribution of arsenic species in unicellular algae to the cycling of arsenic in marine ecosystems, Environ. Sci. Technol., 2015, 49, 33–50 CrossRef CAS PubMed.
- J. S. Edmonds and K. A. Francesconi, Transformations of arsenic in the marine environment, Experientia, 1987, 5, 553–557 CrossRef.
- X.-M. Xue, Y. Jun, G. Raber, K. A. Francesconi, G. Li, H. Gao, Y. Yan, C. Rensing and Y.-G. Zhu, Arsenic methyltransferase is involved in arsenosugar biosynthesis by providing DMA, Environ. Sci. Technol., 2017, 51, 1224–1230 CrossRef CAS PubMed.
- A. Poole, D. Penny and B.-M. Sjöberg, Methyl-RNA: An evolutionary bridge between RNA and DNA?, Chem. Biol., 2000, 7, R207–R216 CrossRef CAS PubMed.
- M. Hengesbach and H. Schwalbe, Structural basis for regulation of ribosomal RNA 2'-O-methylation, Angew. Chem., Int. Ed., 2014, 53, 1742–1744 CrossRef CAS PubMed.
- F. Bouvier, A. Rahier and B. Camara, Biogenesis, molecular regulation and function of plant isoprenoids, Prog. Lipid Res., 2005, 44, 357–429 CrossRef CAS PubMed.
- S. Jain, A. Caforio and A. J. M. Driessen, Biosynthesis of archaeal membrane ether lipids, Front. Microbiol., 2014, 5, 641 Search PubMed.
Footnote |
† Electronic supplementary information (ESI) available. See DOI: 10.1039/c7mt00249a |
|
This journal is © The Royal Society of Chemistry 2018 |