DOI:
10.1039/C7SC03232C
(Edge Article)
Chem. Sci., 2017,
8, 6777-6783
One-pot synthesis of hetero[6]rotaxane bearing three different kinds of macrocycle through a self-sorting process†
Received
25th July 2017
, Accepted 3rd August 2017
First published on 4th August 2017
Abstract
In this article, a six-component self-sorting process that involves three types of crown ether macrocycle and three types of cation guest molecule was carefully and thoroughly investigated. The six components include three kinds of crown ether, namely bis(p-phenylene-34-crown-10) (BPP34C10), dibenzo-24-crown-8 (DB24C8) and benzo-21-crown-7 (B21C7), and their corresponding cation guest molecules, namely a 4,4′-bipyridine dication (BPY2+) and dibenzylammonium (DBA) and benzylalkylammonium (BAA) ions, respectively. Based on this well-established highly selective six-component self-sorting process, a hetero[6]rotaxane bearing three different kinds of crown ether macrocycle was designed and successfully synthesized through a facile and efficient one-pot “click” stoppering strategy. Such work is proposed to be a significant advance in the construction of mechanically interlocked molecules with high structural complexity, as well as a good supplement in the areas of multi-component self-sorting and noncovalent self-assembly.
Introduction
In naturally occurring systems, the principles of reversible noncovalent self-assembly and self-sorting are widely used to construct complex architectures from several different building blocks to execute important tasks and specific functions.1 Inspired by nature, a variety of complex and well-ordered multicomponent molecular or supramolecular systems have been constructed or assembled via noncovalent synthetic strategies.2 Rotaxanes,3,4 well-known for their unique structures consisting of mechanically interlocked threads and macrocycles, have attracted considerable attention in the past few decades due to their remarkable potential to evolve into molecular switches and molecular machines.5 Towards achieving multistate and multifunctional rotaxane-based molecular systems, chemists have been putting unremitting effort into the pursuit of increasing the structural complexity of mechanically interlocked molecules6 and other chemical topological structures.7 Owing to the diversity of macrocycles and their host-guest systems, various rotaxanes bearing one or more types of macrocycle, also called hetero[n]rotaxanes,8 have been successfully designed and constructed.
There have been some elegant examples of hetero[n]rotaxanes containing two different macrocycles,9 which were constructed using efficient self-sorting and an orthogonal self-assembly synthetic approach. Schalley6a and co-workers reported the self-sorting process of two classes of macrocyclic polyether, DB24C8 and B21C7, and two sorts of ammonium cation, dibenzylammonium (DBA) and benzylalkylammonium (BAA) ions, and then used the concept of integrative self-sorting to prepare a hetero[3]rotaxane. By precisely programming the association constants and steric hindrance,6a,b,9b,c several hetero[n]rotaxanes with high structural complexity, for example, Liu’s9a twin-axle hetero[7]rotaxane, can be efficiently constructed by a facile one-pot route. Moreover, Stoddart8a–c recently presented an effective “cooperative capture” strategy to construct a series of heterorotaxanes with two different kinds of macrocyclic ring, such as cucurbiturils and cyclodextrins or cucurbiturils and pillarenes, in good yields and high stereospecificities. Goldup9d and co-workers introduced a proof-of-concept kinetic self-sorting approach for the efficient preparation of heterocircuit [3]rotaxanes. However, successful examples of heterorotaxanes bearing three or more different macrocycles have rarely been reported,9e which would provide more possibilities for functionalization10 towards more advanced and complex molecular systems. The major difficulty lies in the remarkably increased number of possible assembly modes in the presence of different kinds of macrocycle and guest molecule in a single system. The design of an ultimate specific and selective self-sorting system,11 which can be introduced as a powerful tool for the efficient construction of complex hetero[n]rotaxanes bearing different kinds of macrocycle, is of great importance and highly desirable.
In this article, we present herein an efficient six-component integrative self-sorting process among three types of crown ether macrocycle and three types of guest molecule. Based on this well-established six-component integrative self-sorting process with good selectivity, a hetero[6]rotaxane bearing three different kinds of crown ether macrocycle was designed and successfully synthesized through a facile and efficient one-pot threading-followed-by-stoppering strategy (Scheme 1). We envisage that this work will present an important advance in the design and construction of complicated mechanically interlocked structures.
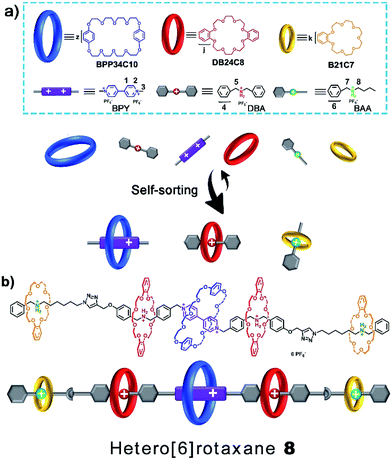 |
| Scheme 1 (a) Schematic representation of the six-component self-sorting process to predominantly form three interlocked species: BPP34C10 ⊃ BPY2+, DB24C8 ⊃ DBA and B21C7 ⊃ BAA. (b) Molecular structure and cartoon picture of the hetero[6]rotaxane 8 which has three different crown ether macrocycles, namely BPP34C10, DB24C8 and B21C7. | |
Results and discussion
Macrocyclic polyethers,12 for example BPP34C10, DB24C8 and B21C7, have been widely employed in the fabrication of mechanically interlocked molecules and supramolecular polymers due to their host–guest interactions with versatile threadlike guests, such as, 4,4′-bipyridine dications (BPY2+) and secondary DBA and BAA salts, respectively. BPP34C10, a larger macrocycle than DB24C8, could recognize viologen units by strong charge transfer and π–π stacking interactions (Ka1 = 240 M−1 in CH3CN),12e while the affinity constant between DB24C8 and a viologen unit is Ka2 = 31 M−1 in CH3CN,12f which is much smaller than that between BPP34C10 and a viologen unit. Meanwhile, the hydrogen bonding between DB24C8 and a DBA site (Ka3 = 420 M−1 in CH3CN)12g was also proven to be stronger than that between BPP34C10 and a DBA site, and the binding constant for BPP34C10 and DBA is much weaker,12h thus the elegant four-component self-sorting process between two macrocyclic rings (BPP34C10 and DB24C8) and two guest molecules (viologen and DBA) has been utilized in the orthogonal supramolecular polymerization of the two species of macrocycles and the two corresponding species of guest molecules.13 Moreover, the other four-component self-sorting process between DB24C8, B21C7, and DBA and BAA units has been demonstrated.6a Hence, herein our motivation is to integrate the above-mentioned two kinds of four-component self-sorting system into an unprecedented six-component self-sorting system based on macrocyclic polyethers, including three types of macrocycle, BPP34C10, DB24C8 and B21C7, and their three corresponding guest species, viologen, DBA and BAA units, then to utilize this unique self-sorting strategy to construct an unprecedented [6]rotaxane with three distinct macrocycles (Scheme 1).
The six-component self-sorting process was thoroughly investigated through a comparison of 1H NMR spectra of the different combinations of crown ether rings and corresponding guest molecules (Fig. 1). Firstly, BPP34C10 and BPY2+ were mixed in CD3CN in the same molar ratio, and the 1H NMR spectrum (Fig. 1a and S1 in the ESI†) clearly confirmed the formation of the pseudorotaxane BPP34C10 ⊃ BPY2+ due to strong charge transfer and π–π stacking interactions. Similarly, the host–guest interactions between DB24C8 and DBA and B21C7 and BAA were also confirmed by the comparison of different 1H NMR spectra (see Fig. S2 and S3 in the ESI†), which fully demonstrate the formation of pseudorotaxanes DB24C8 ⊃ DBA and B21C7 ⊃ BAA (Fig. 1b and c) respectively. Next, we focused on the six-component self-sorting process. Fig. 1d shows the 1H NMR spectrum of the simple mixture of the three macrocyclic crown ether rings and the three corresponding guest molecules with different sizes and shapes. Interestingly, after careful analysis, we found that the 1H NMR spectrum (Fig. 1d) of an equal molar mixture of the six components showed the same pattern as the simple spectral overlap of Fig. 1a–c, evidenced by there being no obvious changes in the chemical shifts of the protons H2–H8, which indicated that the pseudorotaxanes BPP34C10 ⊃ BPY2+, DB24C8 ⊃ DBA and B21C7 ⊃ BAA are the predominant species in this multi-component self-assembly process. This unique self-sorting process could be attributed to the following encoded structural features: (i) BPP34C10 bears a larger binding constant with the BPY2+ site compared to that of DB24C8, hence BPP34C10 binds to the BPY2+ unit prior to DB24C8;14 (ii) DB24C8 enjoys the biggest binding constant with the DBA site compared to with the BAA or BPY2+ sites;7c (iii) the binding behaviour between B21C7 and DBA is inhibited by the benzene rings of the DBA units,6a,15 leading to the specific combination with the guest BAA units. These factors synergistically resulted in a highly selective six-component self-sorting process, as shown in Scheme 1.
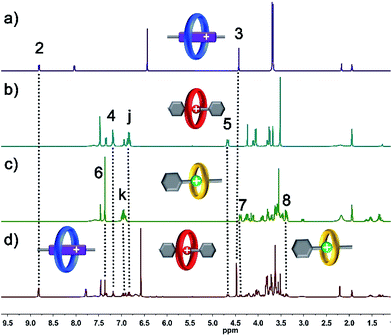 |
| Fig. 1 Partial 1H NMR spectra (400 MHz, 298 K, CD3CN) of (a) 1 : 1 mixture of BPP34C10 ⊃ BPY2+, (b) 1 : 1 mixture of DB24C8 ⊃ DBA, (c) 1 : 1 mixture of B21C7 ⊃ BAA and (d) equal molar mixture of BPP34C10, DB24C8, B21C7, BPY2+, DBA and BAA. | |
Then, we demonstrated for the first time the design and synthesis of a hetero[6]rotaxane bearing three different kinds of crown ether macrocycle via a facile and efficient one-pot strategy through a well-established self-sorting process among several types of host and guest species. As shown in Scheme 2, three macrocyclic polyethers with different cavity sizes, i.e. BPP34C10, DB24C8 and B21C7, were mixed with thread 1 and 5 according to the stoichiometric ratio in a single system. Although there were many possible assemblies among these precursors, only two types of assembly were formed in a specific and efficient self-sorting process, namely pseudo[4]rotaxane 6 consisting of BPP34C10, DB24C8 and thread 1, and [2]semi-rotaxane 7 composed of B21C7 and thread 5. Following the efficient self-sorting process which afforded pseudo[4]rotaxane 6 and [2]semi-rotaxane 7, a cascade-stopped hetero[6]rotaxane 8 could be obtained by a facile one-pot copper(I)-catalyzed alkyne–azide cycloaddition (CuAAC) “click” reaction with a moderate yield of 49%.
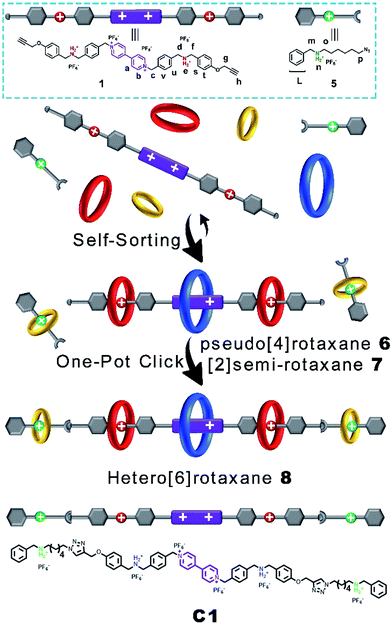 |
| Scheme 2 Schematic representation of the preparation of compound C1 from 1 and 5 and hetero[6]rotaxane 8 from a five-component modularized self-sorting process of 1 and 5 and BPP34C10, DB24C8 and B21C7. | |
The five-component self-sorting process which involves 1 and 5 and three kinds of crown ether was investigated using 1H NMR spectroscopy (Fig. 2). The 1H NMR spectrum of compound 1 in CD3CN was shown in Fig. 2a. Upon the addition of one molar equivalent of macrocycle BPP34C10, the strong host–guest interaction between BPP34C10 and the viologen unit on component 1 drove the efficient formation of the pseudo[2]rotaxane shown in Fig. 2b. Compared to the proton peaks exhibited in Fig. 2a, the aromatic protons Ha and Hb of the viologen unit in 1 were shifted upfield16 with Δδ = 0.25 ppm for Ha and Δδ = 0.05 ppm for Hb, which was attributed to the deshielding effect exerted by the macrocycle BPP34C10. No obvious change was observed for the signal peaks of the Hg, Hd, Hf and Hh protons in the DBA moieties. All of these results indicate that the BPP34C10 ring rested on the viologen sites of 1 instead of on the DBA sites.
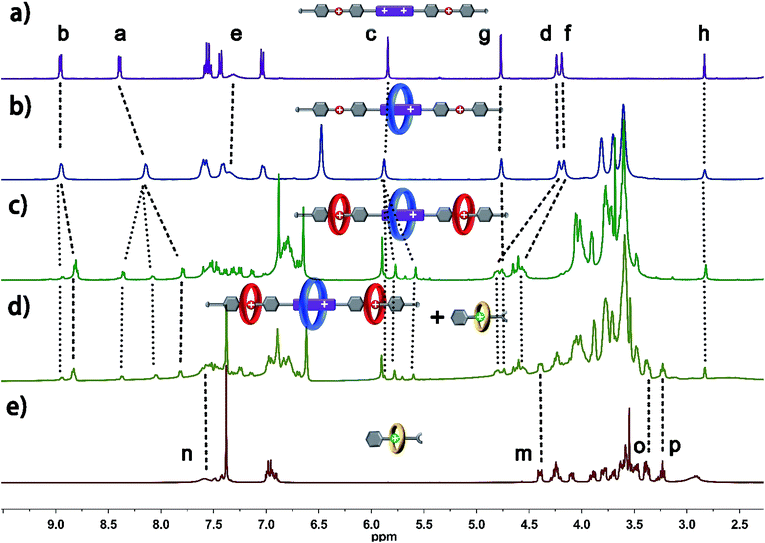 |
| Fig. 2 Partial 1H NMR spectra (400 MHz, CD3CN, 298 K) of (a) compound 1, (b) 1 : 1 mixture of 1 and BPP34C10, (c) 1 : 1 : 2 mixture of 1, BPP34C10 and DB24C8, (d) equal molar mixture of [4]pseudorotaxane 6 and [2]semi-rotaxane 7, and (e) 1 : 1 mixture of 5 and B21C7. | |
In the mixture of thread 1, BPP34C10 and DB24C8 with a molar ratio of 1
:
1
:
2, pseudo[4]rotaxane 6 was formed, as evidenced by the 1H NMR spectrum in Fig. 2c. In contrast to that shown in Fig. 2b, the Hb proton signal split into two different peaks and one of them shifted upfield with a Δδ value of 0.36 ppm. Ha split into three different peaks, one of which shifted upfield with a Δδ value of 0.20 ppm, while the other two shifted downfield with Δδ = 0.06 and 0.35 ppm respectively, due to the deshielding effect of the oxygen atoms on DB24C8 and the incomplete combination of DB24C8 and the dibenzylammonium unit.14 For the same reason, the resonance signal of Hc split into three different peaks. Since Hg and Hh did not directly interact with DB24C8, their chemical shifts were barely changed. Hd and Hf shifted downfield to Δδ values of about 0.59 and 0.43 ppm, respectively. Hd and Hf also shifted due to the deshielding effect of the oxygen atoms from DB24C8.
On the other hand, the 1
:
1 mixture of B21C7 and component 5 in CD3CN could afford [2]semi-rotaxane 7,9b which was demonstrated by the downfield shift of the resonance peaks of Hm and Hp shown in Fig. 2e and S5 in the ESI.† It is worth mentioning that, upon mixing [2]semi-rotaxane 7 with pseudo[4]rotaxane 6 in a 1
:
1 molar ratio, no peak shift is observed in Fig. 2d, compared to either [2]semi-rotaxane 7 (Fig. 2e) or pseudo[4]rotaxane 6 (Fig. 2c). It should be noted that simultaneously mixing the five corresponding threads and macrocycles in one-pot at a molar ratio of 1
:
2
:
2
:
1
:
2 (BPP34C10
:
DB24C8
:
B21C7
:
1
:
5) in CD3CN (Fig. S6†) gave the same 1H NMR spectral pattern as that in Fig. 2d. Such a result suggests the strong independence between these two types of assembly despite the dynamic nature of pseudo[4]rotaxane 6 and [2]semi-rotaxane 7. These results indicated a highly selective self-sorting process among these five classes of component including three different kinds of macrocyclic polyether. This unique self-sorting process gave rise to the efficient and specific formation of pseudo[4]rotaxane 6 and [2]semi-rotaxane 7, which could provide an effective pre-assembly template for the one-pot facile synthesis of target hetero[6]rotaxane 8via a subsequent CuAAC stoppering reaction.
The structure of hetero[6]rotaxane 8 was confirmed by a variety of characterization tools, including 1H NMR, HRMS (Fig. S31–S35†), 1H–1H COSY (Fig. S9†) and 1H–1H NOESY (Fig. S10†). The major signal peaks in the HRMS spectrum of hetero[6]rotaxane 8 are found at m/z 1939.7592, 1244.8542, 897.3967, 688.9200, and 549.9410, which correspond to the target product after the loss of 2, 3, 4, 5 and 6 PF6− ions, i.e. [M-2PF6]2+, [M-3PF6]3+, [M-4PF6]4+, [M-5PF6]5+ and [M-6PF6]6+, respectively. The 1H–1H NOESY spectrum of hetero[6]rotaxane 8 shows cross peaks (CP1 and CP2) between the protons of the BPP34C10 ring and the protons of the BPY2+ unit (Hb and Ha). The cross peaks CP3 and CP5 suggest the correlation between the protons of the DB24C8 ring and the aromatic protons Hu and Hs. The cross peak CP4 illustrates the relationship between the protons of the B21C7 ring and the aromatic protons HL. This means that we have successfully synthesized hetero[6]rotaxane via a self-sorting strategy using a one-pot mild CuAAC “click” reaction.
In order to gain deeper insight into the self-sorting process and further verification of the formation of the interlocked molecular hetero[6]rotaxane 8, the axle compound C1 (Scheme S1†) was also synthesized. Compounds 1, 2 and Cu(CH3CN)4PF6 were mixed in CH3CN and stirred under the protection of argon at room temperature for 48 h, and the axle compound C1 was obtained in a moderate yield of 37%. Compound C1 was characterized by 1H NMR and 13C NMR spectroscopy and HRMS (Fig. S27–S30†). By comparing the 1H NMR spectra of C1 and 8, as shown in Fig. 3a and b, we can find the chemical shift changes of several peaks, which can be attributed to the fact that BPP34C10 and BPY2+, DB24C8 and DBA, and B21C7 and BAA could bind to each other via π–π stacking and hydrogen-bonding interactions. Owing to the deshielding effect of the combination of BPP34C10 and the viologen unit of BPY2+, the signals of Hb and Ha shifted upfield with Δδ = −0.14 and −0.60 ppm, respectively. Meanwhile, due to the shielding effect of BPP34C10, the proton peaks of Hc shifted upfield with a Δδ value of about −0.09 ppm. The signals of Hg shifted upfield with a Δδ value of about −0.20 ppm, due to the shielding effect of the complexation of DB24C8 and the DBA unit. The signals of Hd and Hf shifted downfield with Δδ = 0.56 and 0.35 ppm, respectively, owing to the deshielding effect of DB24C8. The signals of Hm and Ho were shifted downfield with Δδ = 0.21 and Δδ = 0.44 ppm, respectively, due to the shielding effect of the combination of B21C7 and the BAA unit. Hence the comparison of the 1H NMR spectra of C1 and 8 provided further evidence for the confirmation of the proposed structure of hetero[6]rotaxane 8.
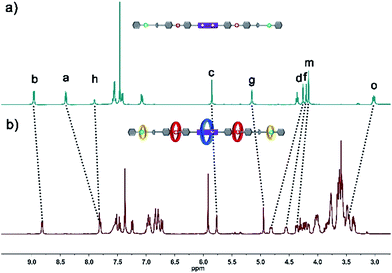 |
| Fig. 3 Partial 1H NMR spectra (400 MHz, 298 K, CD3CN) of (a) compound C1 and (b) hetero[6]rotaxane 8. | |
Experimental
General methods
1H NMR spectra were recorded at 298 K using a Brüker AV-400 spectrometer at a frequency of 400 MHz and are reported as parts per million (ppm) using CD3CN (δH 1.94 ppm) as an internal reference. 13C NMR spectra were recorded at 298 K using a Brüker AV-400 spectrometer at a frequency of 100 MHz and are reported as parts per million (ppm) using CD3CN (δH 1.94 ppm) as an internal reference. The electronic spray ionization (ESI) mass spectra were obtained using an LCT Premier XE mass spectrometer.
Synthesis of hetero[6]rotaxane 8
Compound 1 (0.128 g, 0.10 mmol), BPP34C10 (0.057 g, 0.10 mmol), DB24C8 (0.118 g, 0.25 mmol), B21C7 (0.100 g, 0.26 mmol) and compound 5 (0.089 g, 0.23 mmol) were dissolved in CH3CN (3.0 mL) and stirred under an argon atmosphere at room temperature for 2 h. Then, Cu(CH3CN)4PF6 (0.021 g, 0.05 mmol) was added to the mixture and stirred under an argon atmosphere at room temperature for 48 h. Then, 15 mL of deionized water was added into the mixture and the mixture was extracted using CH2Cl2 (3 × 15 mL). The organic layers were collected, dried over Na2SO4 and concentrated under reduced pressure. After removal of the solvent, the crude product was purified by chromatography on a silica gel column (CH2Cl2/methanol = 30/1) to generate compound 8 (0.208 g, 49.4%) as a red solid. 1H NMR (400 MHz, CD3CN, 298 K) δ 8.82 (d, J = 6.3 Hz, 4H), 7.81 (d, J = 8.6 Hz, 6H), 7.53 (d, J = 8.0 Hz, 12H), 7.46 (d, J = 8.0 Hz, 4H), 7.37 (s, 12H), 7.25 (d, J = 8.4 Hz, 4H), 6.99–6.91 (m, 12H), 6.87–6.82 (m, 9H), 6.82–6.75 (m, 9H), 6.72 (d, J = 8.3 Hz, 4H), 5.77 (s, 4H), 4.95 (s, 4H), 4.85–4.77 (m, 4H), 4.58–4.52 (m, 4H), 4.39–4.33 (m, 4H), 4.31 (t, J = 7.0 Hz, 4H), 4.24 (d, J = 6.9 Hz, 4H), 4.21 (d, J = 4.8 Hz, 4H), 4.18–4.13 (m, 4H), 4.07–3.96 (m, 16H), 3.88–3.81 (m, 8H), 3.77 (d, J = 5.5 Hz, 16H), 3.66–3.56 (m, 66H), 3.50–3.44 (m, 16H), 3.37 (m, J = 10.5, 6.3 Hz, 8H), 1.82–1.77 (m, 4H), 1.55–1.49 (m, 4H). 13C NMR (100 MHz, CD3CN, 298 K) δ 158.6, 151.5, 147.8, 147.0, 146.6, 145.7, 145.2, 142.6, 133.8, 133.5, 132.6, 130.7, 129.8, 129.7, 129.1, 128.8, 128.4, 124.7, 123.6, 123.4, 121.4, 120.9, 120.9, 114.2, 114.1, 113.5, 112.0, 111.7, 70.8, 70.6, 70.3, 70.3, 70.0, 69.8, 69.7, 69.3, 69.2, 69.0, 68.9, 68.6, 67.9, 67.6, 67.5, 67.1, 60.9, 50.5, 50.4, 49.4, 46.6, 29.3, 29.1, 29.0, 28.6, 25.7, 25.7, 25.2. HRMS (ESI) (m/z): [M-2PF6]2+ calcd for C184H248F24N12O42P4/2: 1939.8131, found: 1939.7592; [M-3PF6]3+ calcd for C184H248F18N12O42P3/3: 1244.8872, found: 1244.8542; [M-4PF6]4+ calcd for C184H248F12N12O42P2/4: 897.4242, found: 897.3967; [M-5PF6]5+ calcd for C184H248F6N12O42P/5: 688.9464, found: 688.9200; [M-6PF6]6+ calcd for C184H248N12O42/6: 549.9612, found: 549.9410.
Synthesis of C1
Compound 1 (0.088 g, 0.07 mmol), compound 5 (0.064 g, 0.17 mmol) and Cu(CH3CN)4PF6 (0.012 g, 0.04 mmol) were dissolved in CH3CN (3.0 mL) and stirred under an argon atmosphere at room temperature for 48 h. Then, 20 mL of deionized water was added into the mixture and extracted using CH2Cl2 (3 × 20 mL). The collected organic layers were dried over Na2SO4 and concentrated under reduced pressure. After removal of the solvent, the crude product was purified by chromatography on a silica gel column (CH2Cl2/methanol = 10/1) to give compound C1 (0.052 g, 37.0%) as a yellow solid. 1H NMR (400 MHz, CD3CN, 298 K) δ 8.96 (d, J = 6.9 Hz, 4H), 8.41 (d, J = 6.9 Hz, 4H), 7.90 (s, 2H), 7.56 (q, J = 8.4 Hz, 8H), 7.46 (s, 10H), 7.42 (d, J = 8.5 Hz, 3H), 7.08 (d, J = 8.6 Hz, 4H), 5.85 (s, 4H), 5.15 (s, 3H), 4.36 (t, J = 7.1 Hz, 4H), 4.26 (s, 4H), 4.20 (s, 3H), 4.16 (s, 4H), 3.07–2.98 (m, 4H). 13C NMR (100 MHz, CD3CN, 298 K) δ 159.1, 150.1, 145.3, 133.6, 132.0, 131.6, 130.9, 130.2, 129.7, 129.4, 129.4, 128.7, 127.2, 122.3, 114.7, 63.7, 61.0, 51.2, 50.8, 50.2, 49.5, 47.4, 29.1, 25.0, 24.9, 24.9. HRMS (ESI) (m/z): [M-2PF6]2+ calcd for C72H88F24N12O2P4/2: 866.29, found: 866.26; [M-3PF6]3+ calcd for C72H88F18N12O2P3/3: 529.21, found: 529.20; [M-4PF6]4+ calcd for C72H88F12N12O2P2/4: 360.66, found: 360.66; [M-6PF6]6+ calcd for C72H88N12O2/6: 192.12, found: 192.18.
Conclusions
In conclusion, by precisely programming the association constants, steric hindrance and size-matching of different macrocyclic rings and their corresponding guest molecules, we have developed and constructed a novel six-component self-sorting system. The self-sorting strategy as a key tool ensures this highly selective self-assembly process in a multi-component system and gives rise to the formation of specific rotaxane precursors. Based on this well-organized self-sorting process, a novel hetero[6]rotaxane 8 with three different crown ether macrocycles, a BPY2+ recognition site and two secondary ammonium recognition sites was efficiently constructed via a classical CuAAC stoppering reaction. Such work has not only successfully developed a novel hetero[6]rotaxane 8, which will enrich the family of mechanically interlocked molecules, but also provides a good supplement for the self-sorting concept that can be used for the construction of complex supramolecular systems and materials with increasing complexity both in terms of structure and function.
Acknowledgements
This work was supported by NSFC/China (21672060, 21421004), the Fundamental Research Funds for the Central Universities (WJ1616011, WJ1213007, 222201717003) and the Programme of Introducing Talents of Discipline to Universities (B16017).
Notes and references
-
(a) T. Aida and K. Kinbara, Chem. Rev., 2005, 105, 1377–1400 CrossRef PubMed;
(b) J. M. Lehn, Science, 2002, 295, 2400–2403 CrossRef CAS PubMed;
(c) G. M. Whitesides and B. Grzybowski, Science, 2002, 295, 2418–2421 CrossRef CAS PubMed;
(d) J.-M. Lehn, Chem. Soc. Rev., 2007, 36, 151–160 RSC;
(e) J.-M. Lehn, Angew. Chem., Int. Ed., 2013, 52, 2836–2850 CrossRef CAS PubMed.
-
(a) Z. He, W. Jiang and C. A. Schalley, Chem. Soc. Rev., 2015, 44, 779–789 RSC;
(b) L. K. S. V. Krbek, C. A. Schalley and P. Thordarson, Chem. Soc. Rev., 2017, 46, 2622–2637 RSC;
(c) M. M. Safont-Sempere, G. Fernández and F. Würthner, Chem. Rev., 2011, 111, 5784–5814 CrossRef CAS PubMed.
-
(a)
C. J. Bruns and J. F. Stoddart, The Nature of the Mechanical Bond From Molecules to Machines, John Wiley & Sons, 2017 Search PubMed;
(b) E. R. Kay and D. A. Leigh, Angew. Chem., Int. Ed., 2015, 54, 10080–10088 CrossRef CAS PubMed;
(c) M. Xue, Y. Yang, X. Chi, X. Yan and F. Huang, Chem. Rev., 2015, 115, 7398–7501 CrossRef CAS PubMed;
(d) L. C. Gilday, S. W. Robinson, T. A. Barendt, M. J. Langton, B. R. Mullaney and P. D. Beer, Chem. Rev., 2015, 115, 7118–7195 CrossRef CAS PubMed.
-
(a)
R. M. Izatt, Macrocyclic and Supramolecular Chemistry, How Izatt-Christensen Award Winners Shaped the Field, John Wiley & Sons, 2016 Search PubMed;
(b) A. C. Fahrenbach, C. J. Bruns, H. Li, A. Trabolsi, A. Coskun and J. F. Stoddart, Acc. Chem. Res., 2014, 47, 482–493 CrossRef CAS PubMed;
(c) S. J. Loeb, Chem. Soc. Rev., 2007, 36, 226–235 RSC;
(d) J. E. M. Lewis, P. D. Beer, S. J. Loeb and S. M. Goldup, Chem. Soc. Rev., 2017, 46, 2577–3259 RSC.
-
(a) D. A. Leigh, Angew. Chem., Int. Ed., 2016, 55, 14506–14508 CrossRef CAS PubMed;
(b) D. H. Qu, Q. C. Wang, Q. W. Zhang, X. Ma and H. Tian, Chem. Rev., 2015, 115, 7543–7588 CrossRef CAS PubMed;
(c) S. Silvi and A. Credi, Chem. Soc. Rev., 2015, 44, 4275–4289 RSC;
(d) G. Ragazzon, M. Baroncini, S. Silvi, M. Venturi and A. Credi, Nat. Nanotechnol., 2015, 10, 70–75 CrossRef CAS PubMed;
(e) C. Cheng, P. R. McGonigal, S. T. Schneebeli, H. Li, N. A. Vermeulen, C. Ke and J. F. Stoddart, Nat. Nanotechnol., 2015, 10, 547–553 CrossRef CAS PubMed;
(f) A. Goujon, G. Du, E. Moulin, G. Fuks, M. Maaloum, E. Buhler and N. Giuseppone, Angew. Chem., Int. Ed., 2016, 55, 703–707 CrossRef CAS PubMed;
(g) A. Goujon, G. Mariani, T. Lang, E. Moulin, M. Rawiso, E. Buhler and N. Giuseppone, J. Am. Chem. Soc., 2017, 139, 4923–4928 CrossRef CAS PubMed;
(h) D. S. Kim, J. Chang, S. Leem, J. S. Park, P. Thordarson and J. L. Sessler, J. Am. Chem. Soc., 2015, 137, 16038–16042 CrossRef CAS PubMed;
(i) L. P. Yang, F. Jia, J. S. Cui, S. B. Lu and W. Jiang, Org. Lett., 2017, 19, 2945–2948 CrossRef CAS PubMed;
(j) C. Gao, Z. L. Luan, Q. Zhang, S. Yang, S. J. Rao, D. H. Qu and H. Tian, Org. Lett., 2017, 19, 1618–1621 CrossRef CAS PubMed;
(k) Z.-Q. Cao, Y.-C. Wang, A.-H. Zou, G. London, Q. Zhang, C. Gao and D.-H. Qu, Chem. Commun., 2017, 53, 8683–8686 RSC.
-
(a) W. Jiang, H. D. F. Winkler and C. A. Schalley, J. Am. Chem. Soc., 2008, 130, 13852–13853 CrossRef CAS PubMed;
(b) W. Jiang and C. A. Schalley, Proc. Natl. Acad. Sci. U. S. A., 2009, 106, 10425–10429 CrossRef CAS PubMed;
(c) W. Jiang, A. Schafer, C. Mohr and C. A. Schalley, J. Am. Chem. Soc., 2010, 132, 2309–2320 CrossRef CAS PubMed;
(d) W. Wang, L. J. Chen, X. Q. Wang, B. Sun, X. Li, Y. Zhang, J. Shi, Y. Yu, L. Zhang, M. Liu and H. B. Yang, Proc. Natl. Acad. Sci. U. S. A., 2015, 112, 5597–5601 CrossRef CAS PubMed;
(e) C. Gao, Z. L. Luan, Q. Zhang, S. J. Rao, D. H. Qu and H. Tian, Org. Lett., 2017, 19, 3931–3934 CrossRef CAS PubMed.
-
(a) V. Marcos, A. J. Stephens, J. Jaramillo-Garcia, A. L. Nussbaumer, S. L. Woltering, A. Valero, J.-F. Lemonnier, I. J. Vitorica-Yrezabal and D. A. Leigh, Science, 2016, 352, 1555–1559 CrossRef CAS PubMed;
(b) J. J. Danon, A. Krüger, D. A. Leigh, J.-F. Lemonnier, A. J. Stephens, I. J. Vitorica-Yrezabal and S. L. Woltering, Science, 2017, 355, 159–162 CrossRef CAS PubMed;
(c) H. Li, H. Zhang, A. D. Lammer, M. Wang, X. Li, V. M. Lynch and J. L. Sessler, Nat. Chem., 2015, 7, 1003–1008 CrossRef CAS PubMed;
(d) K. S. Chichak, S. J. Cantrill, A. R. Pease, S.-H. Chiu, G. W. V. Cave, J. L. Atwood and J. F. Stoddart, Science, 2004, 304, 1308–1312 CrossRef CAS PubMed;
(e) D. B. Amabilino, P. R. Ashton, A. S. Reder, N. Specer and J. F. Stoddart, Angew. Chem., Int. Ed. Engl., 1994, 33, 1286–1290 CrossRef;
(f) J.-P. Sauvage and J. Weiss, J. Am. Chem. Soc., 1985, 107, 6108–6110 CrossRef CAS PubMed.
-
(a) C. Ke, R. A. Smaldone, T. Kikuchi, H. Li, A. P. Davis and J. F. Stoddart, Angew. Chem., Int. Ed., 2013, 52, 381–387 CrossRef CAS PubMed;
(b) C. Ke, N. L. Strutt, H. Li, X. Hou, K. J. Hartlieb, P. R. McGonigal, Z. Ma, J. Iehl, C. L. Stren, C. Cheng, Z. Zhu, N. A. Vermeulen, T. J. Meade, Y. Y. Botros and J. F. Stoddart, J. Am. Chem. Soc., 2013, 135, 17019–17030 CrossRef CAS PubMed;
(c) X. Hou, C. Ke, C. Cheng, N. Song, A. K. Blackburn, A. A. Sarjeant, Y. Y. Botros, Y.-W. Yang and J. F. Stoddart, Chem. Commun., 2014, 50, 6196–6199 RSC;
(d) E. A. Wilson, N. A. Vermeulen, P. R. McGonigal, A.-J. Avestro, A. A. Sarjeant, C. L. Stern and J. F. Stoddart, Chem. Commun., 2014, 50, 9665–9668 RSC;
(e) P.-N. Chen, C.-C. Lai and S.-H. Chiu, Org. Lett., 2011, 13, 4660–4663 CrossRef CAS PubMed.
-
(a) Z. J. Zhang, H. Y. Zhang, H. Wang and Y. Liu, Angew. Chem., Int. Ed., 2011, 50, 10834–10838 CrossRef CAS PubMed;
(b) X. Fu, Q. Zhang, S.-J. Rao, D.-H. Qu and H. Tian, Chem. Sci., 2016, 7, 1696–1701 RSC;
(c) Q.-F. Luo, L. Zhu, S.-J. Rao, H. Li, Q. Miao and D.-H. Qu, J. Org. Chem., 2015, 80, 4704–4709 CrossRef CAS PubMed;
(d) E. A. Neal and S. M. Goldup, Angew. Chem., Int. Ed., 2016, 55, 12488–12493 CrossRef CAS PubMed;
(e) J. E. M. Lewis, J. Winn, L. Cera and S. M. Goldup, J. Am. Chem. Soc., 2016, 138, 16329–16336 CrossRef CAS PubMed.
-
(a) P. Waeles, B. Riss-Yaw and F. Coutrot, Chem.–Eur. J., 2016, 22, 6837–6845 CrossRef CAS PubMed;
(b) Z. Meng and C. F. Chen, Chem. Commun., 2015, 51, 8241–8244 RSC;
(c) B. Lewandowski, G. D. Bo, J. W. Ward, M. Papmeyer, S. Kuschel, M. J. Aldegunde, P. M. E. Gramlich, D. Heckmann, S. M. Goldup, D. M. D’Souza, A. E. Fernandes and D. A. Leigh, Science, 2013, 339, 189–193 CrossRef CAS PubMed.
-
(a) W. Jiang, D. Sattler, K. Rissanen and C. A. Schalley, Org. Lett., 2011, 13, 4502–4505 CrossRef CAS PubMed;
(b) W. Jiang and C. A. Schalley, J. Mass Spectrom., 2010, 45, 788–798 CrossRef CAS PubMed;
(c) W. Wang, Y. Zhang, B. Sun, L. J. Chen, X. D. Xu, M. Wang, X. Li, Y. Yu, W. Jiang and H. B. Yang, Chem. Sci., 2014, 5, 4554–4560 RSC.
-
(a) Z. Liu, S. K. M. Nalluri and J. F. Stoddart, Chem. Soc. Rev., 2017, 46, 2459–2478 RSC;
(b) P. L. Anelli, P. R. Ashton, R. Ballardini, V. Balzani, M. Delgado, M. T. Gandolfi, T. T. Goodnow, A. E. Kaifer, D. Philp, M. Pietraszkiewicz, L. Prodi, M. V. Reddington, A. M. Z. Slawin, N. Spencer, J. F. Stoddart, C. Vicent and D. J. Williams, J. Am. Chem. Soc., 1992, 114, 193–218 CrossRef CAS;
(c) J. Wu, K. C. F. Leung, D. Benitez, J. Y. Han, S. J. Cantrill, L. Fang and J. F. Stoddart, Angew. Chem., Int. Ed., 2008, 47, 7470–7474 CrossRef CAS PubMed;
(d) Z. Li, G. Liu, W. Xue, D. Wu, Y. W. Yang, J. Wu, S. H. Liu, J. Yoon and J. Yin, J. Org. Chem., 2013, 78, 11560–11570 CrossRef CAS PubMed;
(e) P. R. Ashton, S. E. Boyd, A. Brindle, S. J. Langford, S. Menzer, L. Perez-Garcia, J. A. Preece, F. M. Raymo, N. Spencer, J. F. Stoddart, A. J. P. White and D. J. Williams, New J. Chem., 1999, 23, 587–602 RSC;
(f) M. Hmadeh, L. Fang, A. Trabolsi, M. Elhabiri, A.-M. Albrecht-Gary and J. F. Stoddart, J. Mater. Chem., 2010, 20, 3422–3430 RSC;
(g) P. R. Ashton, P. J. Campbell, E. J. T. Chrystal, P. T. Glink, S. Menzer, D. Philp, N. Spencer, J. F. Stoddart, P. A. Tasker and D. J. Williams, Angew. Chem., Int. Ed. Engl., 1995, 34, 1865–1869 CrossRef CAS;
(h) P. R. Ashton, P. T. Glink, M.-V. Martinez-Diaz, J. F. Stoddart, A. J. P. Whitr and D. J. Williams, Angew. Chem., Int. Ed. Engl., 1996, 35, 1930–1933 CrossRef CAS.
- F. Wang, C. Han, C. He, Q. Zhou, J. Zhang, C. Wang, N. Li and F. Huang, J. Am. Chem. Soc., 2008, 130, 11254–11255 CrossRef CAS PubMed.
- P. R. Ashton, E. J. T. Chrystal, P. T. Glink, S. Menzer, C. Schiavo, N. Spencer, J. F. Stoddart, P. A. Tasker, A. J. P. Whitr and D. J. Williams, Chem.–Eur. J., 1996, 2, 709–728 CrossRef CAS.
- C. Zhang, S. Li, J. Zhang, K. Zhu, N. Li and F. Huang, Org. Lett., 2007, 9, 5553–5556 CrossRef CAS PubMed.
- F. Wang, B. Zheng, K. Zhu, Q. Zhou, C. Zhai, S. Li, N. Li and F. Huang, Chem. Commun., 2009, 45, 4375–4377 RSC.
Footnote |
† Electronic supplementary information (ESI) available. See DOI: 10.1039/c7sc03232c |
|
This journal is © The Royal Society of Chemistry 2017 |