DOI:
10.1039/C6SC05642C
(Edge Article)
Chem. Sci., 2017,
8, 3146-3153
A bifunctional cerium phosphate catalyst for chemoselective acetalization†
Received
23rd December 2016
, Accepted 6th February 2017
First published on 7th February 2017
Abstract
Acid–base solid catalysts synthesized with structurally controlled uniform active sites can lead to unique catalysis. In this study, a CePO4 catalyst was synthesized using a hydrothermal method and found to exhibit high catalytic performance for the chemoselective acetalization of 5-hydroxymethylfurfural with alcohols, in sharp contrast to other homogeneous and heterogeneous acid and/or base catalysts. In the presence of CePO4, various combinations of carbonyl compounds and alcohols are efficiently converted into the corresponding acetal derivatives in good to excellent yields. Mechanistic studies show that CePO4 most likely acts as a bifunctional catalyst through the interaction of uniform Lewis acid and weak base sites with 5-hydroxymethylfurfural and alcohol molecules, respectively, which results in high catalytic performance.
Introduction
Synergistic and cooperative activation by two or more catalytically active sites with acidic, basic or redox properties can allow high catalytic activity and specific selectivity.1 In particular, acid–base bifunctionality has received much attention because such concepts are widely applied to catalyses related to hydrocarbon conversion, atom-efficient functional group transformation, tandem reactions, and asymmetric syntheses.2 In the fields of heterogeneous catalysis, the acid–base properties of metal oxide-based materials have been extensively studied, and various effective simple and mixed oxide catalysts have been reported.3 However, difficulty in the construction of uniform electrically and structurally controlled acid–base sites often leads to a problem where the fine-tuning of the catalyst structure and the reactivity are restrained. While the modification of oxide surfaces with organic acids and/or bases is a powerful method,4 the susceptibility of the organic parts to oxidative/thermal degradation has limited the usefulness of such catalysts. Therefore, the design and development of new high-performance all-inorganic heterogeneous acid–base catalysts remains a strongly desired and challenging subject of research.
We have recently reported unique base catalysis using oxoanions including [WO4]2− and [PO4]3−.5 Despite their basicities being weaker than those of inorganic and organic strong bases, their specific activation of nucleophiles such as alcohols and amines results in atom-efficient reactions such as the chemical fixation of CO2, regioselective N-alkylation of indoles, and chemoselective acylation of alcohols. On the other hand, rare earth (RE) metal species act as Lewis acid catalysts for various carbon–carbon bond forming reactions through the activation of carbonyl compounds.6 Against this background, we anticipated that RE orthophosphates, REPO4, would be good candidates as bifunctional acid–base catalysts that can work in concert to promote electrophilicity and nucleophilicity in reactive partners. In this communication, we report the highly chemoselective acetalization of 5-hydroxymethylfurfural (1a), which has alcohol and aldehyde functionalities,7 with alcohols using a monoclinic CePO4 catalyst synthesized by a hydrothermal method (Fig. 1(a)). Compound 1a is a versatile carbonyl compound with sensitive functional groups. For reactions of 1a with alcohols in the presence of acid catalysts,8,9 ethers or a complex mixture of products are typically obtained due to the presence of the Brønsted acid-sensitive hydroxyl groups in 1a.8 The present system has the following significant advantages: (i) high yields and chemoselectivity toward acetals, even for substrates with hydroxyl groups, (ii) applicability to various combinations of substrates and larger-scale syntheses, and (iii) reusability as a heterogeneous catalyst system. While metal phosphates and related materials have been extensively investigated for the conversion of carbohydrates into 1a,10 acid–base catalysis over CePO4 has not been reported to date11 and the present bifunctionality can lead to the chemoselective acetalization of carbonyl compounds containing sensitive functional groups, such as 1a.
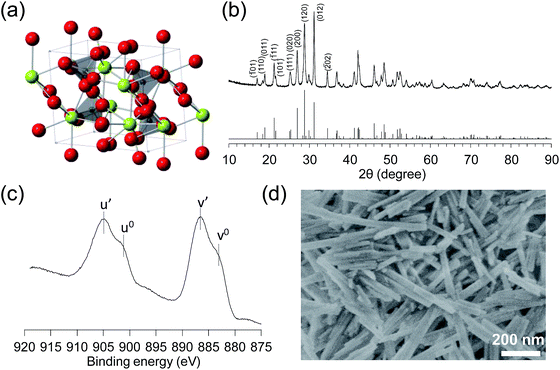 |
| Fig. 1 (a) Structure of CePO4. Green, gray, and red spheres represent Ce, P, and O atoms, respectively. (b) XRD patterns for CePO4 (upper) and monoclinic CePO4 (lower, ICSD 79748). (c) XPS Ce 3d spectrum and (d) SEM image of CePO4. | |
Results and discussion
Synthesis and characterization of CePO4
Monoclinic CePO4 was synthesized through the hydrothermal reaction of Ce(NO3)3 and (NH4)2HPO4 at 180 °C, followed by calcination at 900 °C (see details in the ESI†). Fig. 1(b) shows the powder X-ray diffraction (XRD) pattern measured for CePO4, which is in good agreement with that reported for the monoclinic CePO4 structure, in which a Ce3+ ion connects to seven tetrahedral PO43− groups [space group P21/n].12 The impurity phases of other cerium and phosphorus oxides were not observed. The infrared (IR) spectrum of CePO4 is shown in Fig. S1.† Asymmetric stretching vibrations of the PO43− groups were split into bands at 1091, 1062, 1028, 994, and 956 cm−1 due to a decrease in the symmetry of PO43− from Td to C1.13 The bands in the range of 500–700 cm−1 are assigned to the bending of the P–O links in the distorted PO43− tetrahedra, and these band positions are similar to those previously reported for monoclinic CePO4.13 The elemental analysis of bulk CePO4 using energy dispersive X-ray spectroscopy (EDX) revealed that the molar ratio of Ce
:
P is 1
:
1. The valence state of the surface Ce was investigated using X-ray photoelectron spectroscopy (XPS) (Fig. 1(c)). The Ce 3d3/2,5/2 spectra are composed of two multiplets (v and u), which correspond to the spin–orbit split 3d5/2 and 3d3/2 core holes.14 The Ce 3d spectrum of CePO4 exhibits four peaks with binding energies of 904.9, 901.0, 806.5, and 883.0 eV, which correspond to the u′, u0, v′, and v0 peaks, respectively, and are in good agreement with the reported Ce 3d spectra for Ce(III) oxides.15 The specific surface area of CePO4 calculated from a Brunauer–Emmett–Teller (BET) plot of the N2 adsorption isotherm (77 K) was up to 37 m2 g−1. Fig. 1(d) shows a scanning electron microscopy (SEM) image of CePO4 with rod-like shaped particles 100–500 nm long and 20–50 nm wide.
The acidic properties of CePO4 were evaluated using IR spectroscopy for a sample with adsorbed pyridine as a probe base (see details in the ESI†).3,10,16 Differential IR spectra of CePO4 with adsorbed pyridine are shown in Fig. 2(a) and S2.† The band at 1445 cm−1 is assigned to the pyridine species coordinated to the Lewis acid sites, while no band due to pyridinium ions bonded to the Brønsted acid sites was observed at ca. 1540 cm−1. The amount of Lewis acid sites on CePO4 was estimated from the intensity of the band at 1445 cm−1 to be 0.096 mmol g−1. The difference IR spectrum for CePO4 with adsorbed CHCl3 is shown in Fig. 2(b). The red-shift of the original C–H stretching mode of the CHCl3 molecule (from 3034 cm−1 to 3008 cm−1) indicates the presence of basic sites on the surface.16 In addition, a new broad shoulder band appeared at 1250 cm−1, which was assigned as δ(ClC–H) for the CHCl3 molecules due to the interaction of the acidic hydrogen and chlorine atoms with the basic oxygen and Lewis acid sites, respectively.16 Thus, the base sites on CePO4 could be located in close proximity to the Lewis acid sites, in agreement with the structure of CePO4.
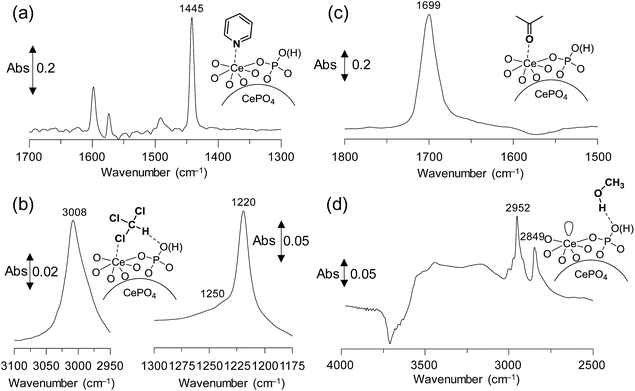 |
| Fig. 2 Difference IR spectra for (a) pyridine-, (b) chloroform-, (c) acetone-, and (d) methanol-adsorbed CePO4 at 25 °C. | |
Catalytic acetalization of 1a with methanol
The reaction of 1a with methanol was examined first in the presence of various catalysts that have been reported to be effective for acetalization,17 and the results are summarized in Table 1. The three products 5-(dimethoxymethyl)-2-furanmethanol (2a), 5-methoxymethylfurfural (3a), and 2-(dimethoxymethyl)-5-(methoxymethyl)furan (4a) were mainly formed. The reaction did not proceed in the absence of a catalyst (entry 27). Among the catalysts tested, CePO4 exhibited the highest activity for the acetalization of 1a to give 2a in 78% yield (entry 1). In this case, the selectivity toward 2a reached 96% without the formation of 3a or 4a. In the presence of homogeneous Brønsted acid catalysts (H2SO4, p-toluenesulfonic acid (TsOH), and H3PW12O40) and Lewis acid catalysts (scandium trifluoromethanesulfonate (Sc(OTf)3) and Ce(OTf)3), only 3a and/or 4a were obtained in low to moderate yields (entries 2–6). The reaction of 1a with methanol in the presence of these homogeneous acid catalysts was carried out by reducing the amounts of the catalysts to match the surface Ce content (i.e. 9.6 μmol) with the Lewis acid sites measured using pyridine-IR (Table S1†). While the conversions using these homogeneous acid catalysts were higher than that using CePO4, 2a was not formed under the reaction conditions. In addition, no product was obtained using the homogeneous base catalysts of K3PO4, K2HPO4, and KH2PO4 (entries 7–9). Thus, homogeneous acid or base catalysts themselves are not deemed as effective for the chemoselective acetalization of 1a. Acetalization over Nb2O5 was less effective than over CePO4, and other metal oxide catalysts including SiO2, ZrO2, CeO2, Al2O3, MgO, TiO2, and SnO2 were almost inactive (entries 13–20). Typical solid acid catalysts such as sulfated zirconia, sulfonated carbon, Nafion, mordenite, and montmorillonite gave complex mixtures of 2a, 3a, and 4a (entries 21–26). The catalyst precursors of Ce(NO3)3, (NH4)2HPO4, and a mixture of Ce(NO3)3 and (NH4)2HPO4 were not effective for acetalization (entries 10–12), which indicates that CePO4 plays an important role in the acetalization reaction.18
Table 1 Effect of catalysts on the reaction of 1a with methanola

|
Entry |
Catalyst |
Conv. (%) |
Yield (%) |
2a
|
3a
|
4a
|
Reaction conditions: catalyst (0.1 g), 1a (1.0 mmol), methanol (5 mL), and reflux for 1 h. Conversion and yield were determined by GC analysis. Conversion (%) = converted 1a (mol)/initial 1a (mol) × 100. Yield (%) = product (mol)/initial 1a (mol) × 100.
Catalyst (0.43 mmol; i.e. equivalent to the Ce content in CePO4 (0.1 g)).
|
1 |
CePO4 |
81 |
78 |
<1 |
<1 |
2b |
H2SO4 |
>99 |
<1 |
24 |
2 |
3b |
TsOH |
>99 |
<1 |
54 |
2 |
4b |
H3PW12O40 |
>99 |
<1 |
4 |
<1 |
5b |
Sc(OTf)3 |
>99 |
<1 |
49 |
5 |
6b |
Ce(OTf)3 |
74 |
<1 |
27 |
<1 |
7 |
K3PO4 |
81 |
<1 |
<1 |
<1 |
8 |
K2HPO4 |
<1 |
<1 |
<1 |
<1 |
9 |
KH2PO4 |
1 |
<1 |
<1 |
<1 |
10b |
Ce(NO3)3·6H2O |
68 |
<1 |
12 |
<1 |
11b |
(NH4)2HPO4 |
43 |
<1 |
<1 |
<1 |
12b |
Ce(NO3)3·6H2O + (NH4)2HPO4 |
85 |
<1 |
<1 |
<1 |
13 |
Nb2O5 |
38 |
31 |
<1 |
<1 |
14 |
SiO2 |
12 |
1 |
<1 |
<1 |
15 |
ZrO2 |
9 |
9 |
<1 |
<1 |
16 |
CeO2 |
5 |
<1 |
<1 |
<1 |
17 |
Al2O3 |
3 |
3 |
<1 |
<1 |
18 |
MgO |
3 |
<1 |
<1 |
<1 |
19 |
TiO2 |
2 |
3 |
<1 |
<1 |
20 |
SnO2 |
2 |
1 |
<1 |
<1 |
21 |
Sulfated zirconia |
>99 |
<1 |
2 |
15 |
22 |
Sulfonated carbon |
83 |
9 |
15 |
43 |
23 |
Nafion NR-50 |
95 |
1 |
42 |
21 |
24 |
Nafion SAC-13 |
90 |
20 |
2 |
13 |
25 |
Mordenite |
86 |
39 |
9 |
43 |
26 |
Montmorillonite K10 |
85 |
55 |
4 |
23 |
27 |
Blank |
2 |
2 |
<1 |
<1 |
To verify whether the observed catalysis is derived from solid CePO4 or from leached cerium or phosphorus species, the reaction of 1a with methanol was conducted under the conditions described in entry 1 of Table 1, and CePO4 was removed from the reaction mixture by hot filtration at ca. 30% conversion of 1a (at t = 15 min). The filtrate was then heated again under the same reaction conditions. In this case, no further production of 2a was observed, as shown in Fig. 3. No leaching of cerium or phosphorus species into the filtrate was observed using inductively coupled plasma atomic emission spectroscopy (ICP-AES, with detection limits for Ce and P atoms of ca. 1 and 3 ppb, respectively). Therefore, there was no contribution to the observed catalysis from cerium or phosphorus species leached into the reaction solution, and the nature of the observed catalysis is confirmed as truly heterogeneous.19 The used CePO4 catalyst could be readily recovered from the reaction mixture by simple filtration (96% recovery). The recovered CePO4 catalyst could then be reused without a significant decrease in the yield of 2a or the selectivity: 78% yield of 2a at 81% conversion (fresh) and 78% yield of 2a at 80% conversion (reused). There was no significant difference in the XRD patterns of the fresh and reused CePO4 catalysts, which indicates the high durability of CePO4 (Fig. S3†).
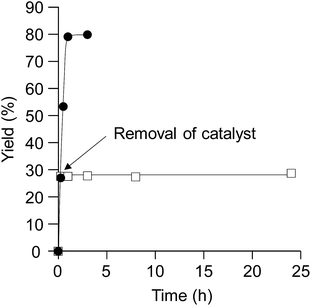 |
| Fig. 3 Effect of CePO4 removal on the acetalization of 1a with methanol. Without removal of CePO4 (●), and the arrow indicates the point of CePO4 removal (□). Reaction conditions: CePO4 (0.1 g), 1a (1.0 mmol), methanol (5 mL), and reflux. | |
Reaction mechanism for the CePO4-catalyzed acetalization
While it has been reported that other metal oxides (e.g. CeO2) can function as effective acid–base catalysts,3,17d only CePO4 exhibited high catalytic activity and chemoselectivity for acetalization. Thus, the activation mode of substrates with CePO4 and CeO2 was confirmed using IR measurements of samples with adsorbed acetone and methanol. As shown in Fig. 2(c), one strong C
O stretching band of acetone adsorbed on CePO4 was observed at a lower wavenumber (1699 cm−1) than that of acetone in the gas phase (1731 cm−1).20 In addition, the IR spectrum of acetone adsorbed on CeO2 exhibited a shoulder at 1700 cm−1 and a strong band at 1673 cm−1 due to acetone molecules coordinated to different types of Lewis acid sites, and absorptions at 1627 cm−1 and 1570–1550 cm−1 are assignable to condensed species (Fig. S4†).20 These results indicate the interaction between the carbonyl oxygen of the ketone and the uniform Lewis acid sites on CePO4 without the promotion of aldol condensation.21,22
Fig. 2(d) shows the IR spectrum of methanol adsorbed on CePO4. In the ν(O–H) region, negative OH bands were observed with the appearance of broad bands between 3000 and 3500 cm−1. In addition, the IR spectrum shows bands at 2952 and 2849 cm−1 that were assigned to asymmetric and symmetric CH3 stretching modes, respectively. The appearance of such broad bands and the band positions of ν(CH3) indicate that methanol is adsorbed molecularly on CePO4via hydrogen bonds, which is consistent with previous reports for the non-dissociative adsorption of methanol on metal oxides.23 On the other hand, the IR spectrum for methanol adsorbed on CeO2 has bands at 2911 and 2805 cm−1 that were assigned to the νas(CH3) and νs(CH3) modes of methoxide species, respectively (Fig. S4†). Therefore, CePO4 most likely acts as a bifunctional catalyst through interaction of the uniform Lewis acid sites and weak base sites with 1a and alcohol molecules, respectively, which results in highly efficient and chemoselective acetalization.24 The present acetalization of 1a with methanol possibly proceeds as follows (Fig. 4). First, the activation of both 1a and methanol by CePO4 facilitates nucleophilic attack of the OH group in methanol on the carbon atom of the carbonyl group in 1a to give the corresponding hemiacetal derivative. Further reaction of the hemiacetal with methanol then occurs, most likely with the assistance of the CePO4 catalyst, to give the corresponding acetal derivative.
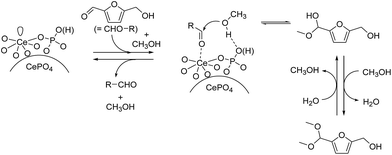 |
| Fig. 4 Proposed reaction mechanism for the CePO4-catalyzed acetalization of 1a with methanol. | |
Substrate scope for the CePO4-catalyzed acetalization
To investigate the effectiveness of the bifunctional properties of CePO4, CePO4-catalyzed acetalization was explored with various substrates. In the presence of CePO4, various combinations of carbonyl compounds and alcohols were efficiently converted into the corresponding acetal derivatives in good to excellent yields (Table 2). The acetalization of 1a with methanol and with diols such as ethylene glycol and 1,3-propanediol proceeded selectively (entries 1–3). CePO4 effectively catalyzed the acetalization of other aldehydes containing heteroatoms, such as furfural (1b) and 2-thiophenecarboxaldehyde (1c), into the corresponding dimethyl acetals, while the acetalization of 2-pyridinecarboxaldehyde (1d) did not proceed (entries 4–6). The catalytic reactivity of CePO4 in the presence of pyridine was investigated. It was confirmed that the presence of pyridine strongly inhibited the reaction of 1a with methanol (Fig. 5). The yield of 2a decreased with an increase in the small amount of pyridine added (3–12 μmol; ca. 0.3–1.3 equivalents with respect to the Lewis acid sites on CePO4). The nitrogen atom of pyridine would strongly coordinate to the cerium metal center, which would inhibit the reaction. The reactions of benzaldehydes with electron-donating and electron-withdrawing para-substituents (1e–1g) proceeded to afford the corresponding dimethyl acetals in high yields (entries 7–9). Even the bulkier aldehyde 1-naphthaldehyde (1h) could be efficiently acetalized when the reaction time was prolonged to 20 h (entry 10). The acetalization of cinnamaldehyde (1i) with methanol proceeded smoothly without influence on the C
C double bond (entry 11). Not only were aromatic and α,β-unsaturated aldehydes converted but also 3-phenylpropionaldehyde (1j) and cyclohexanecarboxaldehyde (1k) were efficiently converted into the corresponding acetals in high yields (entries 12 and 13). Furthermore, the present system could effectively catalyze aliphatic and aromatic ketones with ethylene glycol. Cyclohexanone (1l) was quantitatively converted into 2,2-pentamethylene-1,3-dioxolane (2l), and acetophenone (1m) gave its corresponding ketal (2m) in moderate yield (entries 14 and 15). Even in the presence of hydroxyl groups in the substrate (5-hydroxy-2-adamantanone (1n)), the corresponding ketal (2n) was obtained in good yield (entry 16).
Table 2 Acetalization of carbonyl compounds with alcohols catalyzed by CePO4a
Entry |
Carbonyl compound |
Alcohol |
Time (h) |
Product (yield (%)) |
Reaction conditions: CePO4 (0.1 g), 1 (1.0 mmol), alcohol (5 mL), and reflux. Yields were isolated yields.
Yield determined using nuclear magnetic resonance spectroscopy (NMR).
120 °C.
|
1 |
|
CH3OH |
1 |
|
2b |
1a
|
|
1 |
|
3b |
1a
|
|
2 |
|
4 |
|
CH3OH |
6 |
|
5 |
|
CH3OH |
6 |
|
6 |
|
CH3OH |
6 |
Not detected |
7 |
|
CH3OH |
6 |
|
8 |
|
CH3OH |
6 |
|
9 |
|
CH3OH |
6 |
|
10 |
|
CH3OH |
20 |
|
11 |
|
CH3OH |
6 |
|
12 |
|
CH3OH |
20 |
|
13 |
|
CH3OH |
20 |
|
14c |
|
|
6 |
|
15 |
|
|
6 |
|
16 |
|
CH3OH |
6 |
|
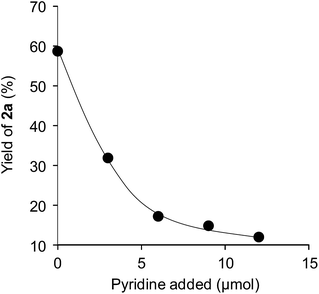 |
| Fig. 5 Yields of 2a against the amount of pyridine added. Reaction conditions: CePO4 (0.1 g), 1a (1.0 mmol), methanol (5 mL), and reflux for 30 min. | |
The present catalytic system was applicable to a gram-scale reaction of 1a (10.5 mmol scale) with methanol and 1.46 g of analytically pure 2a could be isolated (eqn (1)). In this case, the turnover number (TON) based on surface Lewis acid sites reached 177 and the corresponding turnover frequency (TOF) was 44 h−1. In addition, CePO4 efficiently catalyzed the gram-scale regioselective acetalization of acetone (1o) with glycerol into the industrially important chemical 2,2-dimethyl-1,3-dioxolan-5-ol (solketal (2o)).25,26 Solketal has been used as a highly soluble additive to increase the octane number of fuel, and as such many catalyst systems to aid its synthesis have been reported.25,26 CePO4 exhibited high regioselectivity (2o/2o′ = 98/2) and 1.29 g of analytically pure 2o was successfully isolated (eqn (2)), while the condensation of glycerol with 1o under acidic conditions sometimes affords a mixture of five- and six-membered acetals (2o and 2o′, respectively).25
|  | (1) |
| 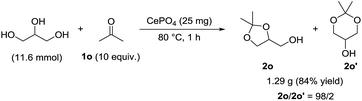 | (2) |
Conclusions
In conclusion, CePO4 efficiently catalyzes the acetalization of various aryl and aliphatic carbonyl compounds containing hydroxyl groups, C
C bonds, and heteroatoms with alcohols. This study suggests that the development of bifunctional solid catalysts with uniform active sites is of particular importance. This approach is a promising strategy for the development of highly efficient heterogeneously-catalyzed reactions through the non-dissociative activation of both electrophiles and nucleophiles under very mild conditions.
Acknowledgements
This work was supported in part by a Kakenhi Grant-in-Aid (No. 15K13802) from the Japan Society for the Promotion of Science (JSPS), the ALCA and CREST programs of the Japan Science and Technology Agency (JST), the Novel Cheap and Abundant Materials for Catalytic Biomass Conversion (NOVACAM) program of JST, and the European Commission Directorate-General for Research and Innovation.
Notes and references
-
Topics in Organometallics Chemistry—Bifunctional Molecular Catalysis, ed. T. Ikariya, and M. Shibasaki, Springer, Berlin, 2011 Search PubMed.
-
(a) E. Iglesia, D. G. Barton, J. A. Biscardi, M. J. L. Gines and S. L. Soled, Catal. Today, 1997, 38, 339–360 CrossRef;
(b) N. Mizuno and K. Yamaguchi, Synlett, 2010, 2365–2382 CrossRef;
(c) M. J. Climent, A. Corma, S. Iborra and M. J. Sabater, ACS Catal., 2014, 4, 870–891 CrossRef CAS;
(d) D. H. Paull, C. J. Abraham, M. T. Scerba, E. Alden-Danforth and T. Lectka, Acc. Chem. Res., 2008, 41, 655–663 CrossRef CAS PubMed;
(e) J. A. Ma and D. Cahard, Angew. Chem., Int. Ed., 2004, 43, 4566–4583 CrossRef CAS PubMed.
-
(a)
R. A. Sheldon, I. W. C. E. Arends, and U. Hanefeld, in Green Chemistry and Catalysis, ed. R. A. Sheldon, I. W. C. E. Arends and U. Hanefeld, Wiley-VCH, Weinheim, 2007, pp. 133–221 Search PubMed;
(b)
Y. Ono and H. Hattori, Solid Base Catalysis, Springer-Verlag, Heidelberg, 2011 Search PubMed;
(c)
H. Hattori, and Y. Ono, Solid Acid Catalysis: From Fundamentals to Applications, CRC Press, Stanford, 2015 Search PubMed.
-
(a) K. Motokura, M. Tada and Y. Iwasawa, Chem.–Asian J., 2008, 3, 1230–1236 CrossRef CAS PubMed;
(b) S. Shylesh and W. R. Thiel, ChemCatChem, 2011, 3, 278–287 CrossRef CAS.
-
(a) T. Kimura, K. Kamata and N. Mizuno, Angew. Chem., Int. Ed., 2012, 51, 6700–6703 CrossRef CAS PubMed;
(b) T. Kimura, H. Sunaba, K. Kamata and N. Mizuno, Inorg. Chem., 2012, 51, 13001–13008 CrossRef CAS PubMed;
(c) H. Sunaba, K. Kamata and N. Mizuno, ChemCatChem, 2014, 6, 2333–2338 CrossRef CAS;
(d) K. Sugahara, N. Satake, K. Kamata, T. Nakajima and N. Mizuno, Angew. Chem., Int. Ed., 2014, 53, 13248–13252 CrossRef CAS PubMed.
- S. Kobayashi and K. Manabe, Acc. Chem. Res., 2002, 35, 209–217 CrossRef CAS PubMed.
-
(a) A. A. Rosatella, S. P. Simeonov, R. F. M. Frade and C. A. M. Afonso, Green Chem., 2011, 13, 754–793 RSC;
(b) R. J. van Putten, J. C. van der Waal, E. de Jong, C. B. Rasrendra, H. J. Heeres and J. G. de Vries, Chem. Rev., 2013, 113, 1499–1597 CrossRef CAS PubMed.
-
1a-based ethers and acetals are important chemicals for use as biodiesel, surfactants, scents, and flavors:
(a) O. Casanova, S. Iborra and A. Corma, J. Catal., 2010, 275, 236–242 CrossRef CAS;
(b) P. Neves, M. M. Antunes, P. A. Russo, J. P. Abrantes, S. Lima, A. Fernandes, M. Pillinger, S. M. Rocha, M. F. Ribeiro and A. A. Valente, Green Chem., 2013, 15, 3367–3376 RSC;
(c) E. R. Sacia, M. Balakrishnan and A. T. Bell, J. Catal., 2014, 313, 70–79 CrossRef CAS;
(d) P. Lanzafame, K. Barbera, S. Perathoner, G. Centi, A. Aloise, M. Migliori, A. Macario, J. B. Nagy and G. Giordano, J. Catal., 2015, 330, 558–568 CrossRef CAS;
(e) W. Hao, W. Li, X. Tang, X. Zeng, Y. Sun, S. Liu and L. Lin, Green Chem., 2016, 18, 1080–1088 RSC.
- There are only two examples of beta zeolite-based catalysts for chemoselective acetalization of HMF with alcohols:
(a) K. S. Arias, S. I. Al-Resayes, M. J. Climent, A. Corma and S. Iborra, ChemSusChem, 2013, 6, 123–131 CrossRef CAS PubMed;
(b) O. Casanova, S. Iborra and A. Corma, J. Catal., 2009, 265, 109–116 CrossRef CAS.
-
(a) K. Nakajima, Y. Baba, R. Noma, M. Kitano, J. N. Kondo, S. Hayashi and M. Hara, J. Am. Chem. Soc., 2011, 133, 4224–4227 CrossRef CAS PubMed;
(b) A. Dutta, A. K. Patra, S. Dutta, B. Saha and A. Bhaumik, J. Mater. Chem., 2012, 22, 14094–14100 RSC;
(c) A. Dutta, D. Gupta, A. K. Patra, B. Saha and A. Bhaumik, ChemSusChem, 2014, 7, 925–933 CrossRef CAS PubMed;
(d) A. Dibenedetto, M. Aresta, L. di Bitonto and C. Pastore, ChemSusChem, 2016, 9, 118–125 CrossRef CAS PubMed.
- CePO4 catalysts have been extensively investigated for gas-phase reactions such as the selective catalytic reduction of NOx with NH3, CO oxidation, and oxidative dehydrogenation of isobutene, and their use as acid–base catalysts for liquid-phase reactions has not been reported:
(a) W. Yao, Y. Liu, X. Wang, X. Weng, H. Wang and Z. Wu, J. Phys. Chem. C, 2016, 120, 221–229 CrossRef CAS;
(b) C. Tian, S.-H. Chai, X. Zhu, Z. Wu, A. Binder, J. C. Bauer, S. Brown, M. Chi, G. M. Veith, Y. Guo and S. Dai, J. Mater. Chem., 2012, 22, 25227–25235 RSC;
(c) F. Romero-Sarria, M. I. Domínguez, M. A. Centeno and J. A. Odriozola, Appl. Catal., B, 2011, 107, 268–273 CrossRef CAS;
(d) K. Ikeue, K. Murakami, S. Hinokuma, K. Uemura, D. Zhang and M. Machida, Bull. Chem. Soc. Jpn., 2010, 83, 291–297 CrossRef CAS;
(e) Y. Takita, X. Qing, A. Takami, H. Nishiguchi and K. Nagaoka, Appl. Catal., A, 2005, 296, 63–69 CrossRef CAS;
(f) Y. Takita, K.-I. Sano, T. Muraya, H. Nishiguchi, N. Kawata, M. Ito, T. Akbay and T. Ishihara, Appl. Catal., A, 1998, 170, 23–31 CrossRef CAS.
- J. Bao, R. Yu, J. Zhang, X. Yang, D. Wang, J. Deng, J. Chen and X. Xing, CrystEngComm, 2009, 11, 1630–1634 RSC.
-
(a) K. Wang, J. Zhang, J. Wang, C. Fang, W. Yu, X. Zhao and H. Xu, J. Appl. Crystallogr., 2005, 38, 675–677 CrossRef CAS;
(b) W. Jastrzebski, M. Sitarz, M. Rokita and K. Bulat, Spectrochim. Acta, Part A, 2011, 79, 722–727 CrossRef CAS PubMed.
- E. Beche, P. Charvin, D. Perarnau, S. Abanades and G. Flamant, Surf. Interface Anal., 2008, 40, 264–267 CrossRef CAS.
-
(a) D. R. Mullins, S. H. Overbury and D. R. Huntley, Surf. Sci., 1998, 409, 307–319 CrossRef CAS;
(b) L. Qiu, F. Liu, L. Zhao, Y. Ma and J. Yao, Appl. Surf. Sci., 2006, 252, 4931–4935 CrossRef CAS;
(c) F. Larachi, J. Pierre, A. Adnot and A. Bernis, Appl. Surf. Sci., 2002, 195, 236–250 CrossRef CAS.
- T. Komanoya, K. Nakajima, M. Kitano and M. Hara, J. Phys. Chem. C, 2015, 119, 26540–26546 CAS.
-
(a) Y. Izumi, K. Urabe and M. Onaka, Microporous Mesoporous Mater., 1998, 21, 227–233 CrossRef CAS;
(b) I. Ledneczki and Á. Molnár, Synth. Commun., 2004, 34, 3683–3690 CrossRef CAS;
(c) M. Iwamoto, Y. Tanaka, N. Sawamura and S. Namba, J. Am. Chem. Soc., 2003, 125, 13032–13033 CrossRef CAS PubMed;
(d) L. Vivier and D. Duprez, ChemSusChem, 2010, 3, 654–678 CrossRef CAS PubMed;
(e) T. Sato, F. Ono, H. Takenaka, T. Fujikawa and M. Mori, Synthesis, 2009, 1318–1322 CrossRef.
- The acetalization of 1a with methanol in the presence of CePO4 was carried out under the same reaction conditions as those for beta-zeolite reported in ref. 9. The 2a yield was 6% and lower than that of calcined and dehydrated Al-beta zeolite (ca. 600 m2 g−1, Si/Al = 12.5), whereas the reactivity per unit surface area was comparable to that of zeolite.
- R. A. Sheldon, M. Wallau, I. W. C. E. Arends and U. Schuchardt, Acc. Chem. Res., 1998, 31, 485–493 CrossRef CAS.
-
(a) M. I. Zaki, M. A. Hasan, F. A. Al-Sagheer and L. Pasupulety, Langmuir, 2000, 16, 430–436 CrossRef CAS;
(b) M. I. Zaki, M. A. Hasan and L. Pasupulety, Langmuir, 2001, 17, 768–774 CrossRef CAS.
- The amount of Ce cations exposed on the surface was estimated assuming that the (011) plane is a possible surface structure because of its stability.22 The amount of surface Ce cations was estimated to be 3.1 nm−2. This value was almost comparable to that (1.6 nm−2) calculated from the BET surface area of CePO4 (37 m2 g−1) and the surface Ce cations with Lewis acid sites measured using pyridine-IR (0.096 mmol g−1). These results also support the idea that uniform Lewis acid sites exist on CePO4.
- Y. Zhang and H. Guan, J. Cryst. Growth, 2003, 256, 156–161 CrossRef CAS.
-
(a) Z. Martinez-Ramirez, G. A. Flores-Escamilla, G. S. Berumen-España, S. A. Jimenez-Lam, B. E. Handy, M. G. Cardenas-Galindo, A. G. Sarmiento-Lopez and J. C. Fierro-Gonzalez, Appl. Catal., A, 2015, 502, 254–261 CrossRef CAS;
(b) Z. Wu, M. Li, D. R. Mullins and S. H. Overbury, ACS Catal., 2012, 2, 2224–2234 CrossRef CAS.
- The C
O stretching band (1699 cm−1) of acetone adsorbed on CePO4 was observed at a higher wavenumber than that on CeO2 (a strong band at 1673 cm−1), indicating the lower Lewis acid strength of CePO4. In addition, the chloroform- and methanol-adsorbed IR measurements also indicate the lower basicity of CePO4. Therefore, not only the presence of moderate Lewis acid sites but also the weakening of the basicity by replacement of the strong basic sites of CeO2 using PO4 would suppress side reactions such as aldol condensation, resulting in the present high chemoselectivity.
-
(a) M. S. Khayoon and B. H. Hameed, Appl. Catal., A, 2013, 464–465, 191–199 CrossRef CAS;
(b) T. E. Davies, S. A. Kondrat, E. Nowicka, J. J. Graham, D. C. Apperley, S. H. Taylor and A. E. Graham, ACS Sustainable Chem. Eng., 2016, 4, 835–843 CrossRef CAS;
(c) G. S. Nair, E. Adrijanto, A. Alsalme, I. V. Kozhevnikov, D. J. Cooke, D. R. Brown and N. R. Shiju, Catal. Sci. Technol., 2012, 2, 1173–1179 RSC;
(d) S. Zhang, Z. Zhao and Y. Ao, Appl. Catal., A, 2015, 496, 32–39 CrossRef CAS.
-
(a) T. E. Souza, M. F. Portilho, P. M. T. G. Souza, P. P. Souza and L. C. A. Oliveira, ChemCatChem, 2014, 6, 2961–2969 CrossRef CAS;
(b) K. N. Tayade, M. Mishra, M. K. and R. S. Somani, Catal. Sci. Technol., 2015, 5, 2427–2440 RSC;
(c) T. Mitsudome, T. Matsuno, S. Sueoka, T. Mizugaki, K. Jitsukawa and K. Kaneda, Heterocycles, 2012, 84, 371–376 CrossRef CAS;
(d) C. Crotti, E. Farnetti and N. Guidolin, Green Chem., 2010, 12, 2225–2231 RSC;
(e) L. Li, T. I. Koranyi, B. F. Sels and P. P. Pescarmona, Green Chem., 2012, 14, 1611–1619 RSC;
(f) A. W. Pierpont, E. R. Batista, R. L. Martin, W. Chen, J. K. Kim, C. B. Hoyt, J. C. Gordon, R. Michalczyk, L. A. Silks and R. Wu, ACS Catal., 2015, 5, 1013–1019 CrossRef CAS.
Footnote |
† Electronic supplementary information (ESI) available. See DOI: 10.1039/c6sc05642c |
|
This journal is © The Royal Society of Chemistry 2017 |
Click here to see how this site uses Cookies. View our privacy policy here.