DOI:
10.1039/C6QI00245E
(Research Article)
Inorg. Chem. Front., 2016,
3, 1411-1418
An anionic metal–organic framework based on angular tetracarboxylic acid and a mononuclear copper ion for selective gas adsorption†
Received
13th July 2016
, Accepted 7th September 2016
First published on 10th September 2016
Abstract
An anionic metal–organic framework [CuL]·2(CH3)2NH2·DMF (ZJNU-55, L = 5,5′-(pyrimidin-2-methyl-4,6-diyl)diisophthalate) was synthesized by a solvothermal reaction of a custom-designed angular diisophthalate and CuCl2·2H2O, and structurally characterized by single-crystal X-ray diffraction. Notably, although the ligand bears a close resemblance to the previously reported diisophthalate used to construct PCN-307, the resultant MOF's structure is totally different from that of PCN-307. The framework exhibits a mononuclear Cu(COO)4 inorganic secondary building unit, instead of the typical dinuclear Cu2(COO)4 paddlewheel unit. The combination of Cu(COO)4 with the deprotonated organic ligand results in a three-dimensional network structure featuring helical nanotube channels along the crystallographic c axis. TOPOS software analyses indicate that two alternative simplifications based on the organic linkers can produce a 4-connected binodal net with the Schläfli symbol (4·62·83)(4·64·8) or a (3,4)-connected 3-nodal network with the Schläfli symbol (6·8·9)2(6·83·92), which have been rarely observed in MOF chemistry. Furthermore, its gas adsorptive properties were systematically investigated. The activated ZJNU-55a exhibits highly selective adsorption of C2H2 and CO2 over CH4 with the adsorption selectivities of 39.2–64.9 and 13.1–29.2 at 298 K, respectively. As far as we are aware, this is a rare example of a MOF constructed from mononuclear Cu(COO)4 for selective gas adsorption. This work also demonstrates that subtle ligand modification can drastically affect the structures and properties of the resultant MOF.
1. Introduction
Metal–organic frameworks (MOFs), also known as porous coordination polymers (PCPs), are emerging as a rapidly expanding class of porous materials, which are readily constructed from the self-assembly of suitable metal ions/clusters that serve as nodes and organic ligands that act as linkers. The key advantage that MOFs have over traditional porous materials such as activated carbons and zeolites is the easy tuning of the shape, size, and chemical nature of their pores, which offers them with great promise for a broad spectrum of potential applications including but not limited to gas storage and separation,1 chemical sensing,2 drug delivery3 and heterogeneous catalysis.4 Benefiting from their wide range of applications coupled with aesthetical structure characteristics, rapid progress related to MOF chemistry has been made in the last few decades.
Since an organic ligand is one main component of MOFs, the judicious selection of the organic ligand is very critical in the construction of MOFs. Among the various organic ligands, multicarboxylate ligands, especially those containing m-benzenedicarboxylate/isophthalate moieties, are very promising because isophthalate is a very powerful subunit to construct porous MOFs.5 Recently, we became interested in the utilization of linear diisophthalate ligands to target new porous MOFs for gas storage and separation because it has been well demonstrated that a combination of linear diisophthalate ligands and dicopper paddlewheel typically yields NbO-type MOFs,6 such as the earliest reported MOF-505,7 as well as NOTT-10x series,8 which provide an excellent platform to investigate the effect of functional groups on gas adsorption properties.8a,9 Although significant progress has been made in the preparation of porous MOFs by using linear diisophthalates as organic ligands, the use of angular/nonlinear diisophthalates featuring a reduced symmetry compared to the linear diisophthalates is relatively less investigated, but appealing because novel structures might be formed from such types of ligands. For example, Zhou et al. recently used a series of angular diisophthalates bearing different functional groups to produce isostructural MOFs PCN-305–308 with a new network topology.10 To date, the number of porous MOFs based on angular diisophthalates is still vanishingly small.10,11
Based on the above considerations, by only simply substituting the central benzene ring of the recently published angular diisophthalate (5-methyl-1,3-bis(3,5-dicarboxylphenyl)benzene, Scheme 1)10 used to construct PCN-307 with the pyrimidine group, we designed and synthesized a new angular diisophthalate ligand, 5,5′-(pyrimidin-2-methyl-4,6-diyl)diisophthalic acid (Scheme 1), which contains two separated isophthalate subunits connected to central pyrimidine moieties through carbon–carbon single bonds. The introduction of nitrogen atoms is expected to enhance selective gas adsorption. Against our expectation, although the new ligand bears a close resemblance to the one used to construct PCN-307,10 the resultant MOF's structure is totally different from that of PCN-307 upon solvothermally reacting with Cu2+ ions. As a consequence, their distinct gas adsorption properties were also observed. In this work, we wish to report the solvothermal synthesis, single-crystal structure as well as gas adsorption properties of this MOF.
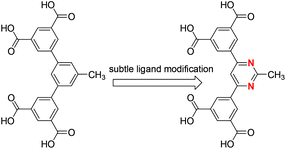 |
| Scheme 1 Chemical structure of the organic ligands employed to construct PCN-307 (left) and ZJNU-55 (right). | |
2. Experimental
2.1 Materials and methods
Dimethyl 5-(pinacolboryl)isophthalate was synthesized according to the method reported in the literature.12 All other chemicals employed were purchased from commercial suppliers and are used without further purification. 1H and 13C{1H} NMR (Nuclear Magnetic Resonance) spectra were obtained with a Bruker AV400 or AV600 NMR spectrometer that operates at 400 or 600 MHz for 1H NMR spectroscopy and 100 or 150 MHz for 13C NMR spectroscopy. The spectra were recorded in CDCl3 or DMSO-d6, and chemical shifts are reported as δ values in ppm relative to TMS (tetramethylsilane). The FTIR (Fourier transform infrared) spectra were recorded using a Nicolet 5DX FTIR spectrometer on KBr discs in the 4000–400 cm−1 region and reported in cm−1 unit. Elemental analyses for C, H and N were carried out with a Vario EL III CHNOS elemental analyser. TGA (thermogravimetric analysis) was performed with a Netzsch STA 449C thermal analyser with a heating rate of 5 K min−1 under a nitrogen atmosphere. PXRD (Powder X-ray Diffraction) measurements were carried out with a Philips PW3040/60 automated powder diffractometer using Cu-Kα radiation (λ = 1.54178 Å). The simulated PXRD pattern was calculated using Mercury 1.4.1 from the single-crystal X-ray diffraction structure. Gas sorption isotherms were measured using a Micromeritics ASAP 2020 HD88 surface-area and pore-size analyser. The temperature of 77 K was maintained using liquid nitrogen, while other temperatures were maintained using a circulating water bath. The gases used had the following purities: N2 (99.9999%), CO2 (99.999%), C2H2 (99.9%), and CH4 (99.99%).
2.2 Single-crystal X-ray crystallography
Crystallographic data were recorded on a Bruker APEX II CCD area-detector diffractometer using Mo-Kα radiation (λ = 0.71073 Å) at T = 296(2) K. Absorption corrections by multi-scan were applied. Structures were solved by direct methods and refined by full-matrix least squares against F2 using the SHELXTL-2014 software package. All non-H atoms were refined anisotropically. Hydrogen atoms attached to carbon were placed in geometrically idealized positions and refined using a riding model. PLATON/SQUEEZE13 was employed to calculate the diffraction contribution of the disordered solvent molecules, which could not be modelled as discrete atomic sites. The structure was further refined using the data generated. Details of the crystal parameters, data collection and refinement of ZJNU-55 are summarized in Table S1.† Crystallographic data for the structures in this paper have been deposited with the Cambridge Crystallographic Data Centre, CCDC, 12 Union Road, Cambridge CB21EZ, UK. CCDC 1492066.
2.3 Synthesis and characterization of the organic ligand
Tetramethyl 5,5′-(pyrimidin-2-methyl-4,6-diyl)diisophthalate.
4,6-Dichloro-2-methylpyrimidine (0.50 g, 3.07 mmol), dimethyl 5-(pinacolboryl)isophthalate (2.45 g, 7.67 mmol), anhydrous Cs2CO3 (3.00 g, 9.20 mmol), and Pd(Ph3P)4 (0.18 g, 0.15 mmol) were added to a 250 mL flask equipped with a stirring bar and a reflux condenser. The flask was evacuated and refilled with nitrogen. The process was repeated three times. After that, dry dioxane (80 mL) was added via syringe. The resulting mixture was magnetically stirred under reflux for 48 h and allowed to cool to ambient temperature. After the solvent had evaporated, the resulting residue was extracted with CH2Cl2 three times. The organic extracts were combined, washed with brine, dried with anhydrous magnesium sulfate, vacuum filtered and concentrated under reduced pressure. The crude reaction mixture was recrystallized from toluene to give tetramethyl immediately as a white solid in 35.5% yield (0.52 g, 1.09 mmol). 1H NMR (CDCl3, 400.1 MHz) δ (ppm): 9.017 (d, J = 1.2 Hz, 4H), 8.855 (t, J = 1.2 Hz, 2H), 8.081 (s, 1H), 4.046 (s, 12H), 2.946 (s, 3H); 13C NMR (CDCl3, 150.9 MHz) δ (ppm): 169.259, 165.830, 163.281, 138.116, 132.625, 132.391, 131.540, 110.078, 52.631, 26.350.
5,5′-(Pyrimidin-2-methyl-4,6-diyl)diisophthalic acid.
Tetramethyl 5,5′-(pyrimidin-2-methyl-4,6-diyl)diisophthalate (0.52 g, 1.09 mmol) was dissolved in THF (40 mL) and MeOH (40 mL), and then 6 M NaOH aqueous solution (40 mL) was added to this solution. The resultant mixture was stirred at 323 K for 24 h, and cooled to room temperature. After the removal of the solvent under reduced pressure, the residue was dissolved in water and filtered. Upon cooling in an ice water bath, the filtrate was acidified with 6 M HCl to pH = 5. The resulting precipitation was collected by suction filtration, and dried under vacuum at 343 K to give the target compound in an almost quantitative yield. 1H NMR (DMSO-d6, 600.1 MHz) δ (ppm): 9.047 (d, J = 1.2 Hz, 4H), 8.664 (t, J = 1.2 Hz, 2H), 8.610 (s, 1H), 2.844 (s, 3H); 13C NMR (DMSO-d6, 100.6 MHz) δ (ppm): 168.404, 167.403, 163.369, 137.475, 133.825, 132.569, 131.934, 110.966, 26.576; selected FTIR (KBr, cm−1): 1714, 1606, 1581, 1539, 1444, 1228, 1111, 754, 677, 654.
2.4 Synthesis and characterization of ZJNU-55
The organic ligand (5.0 mg) and CuCl2·2H2O (10.0 mg) in a solvent mixture of N,N-dimethylformamide (DMF, 1.5 mL), MeOH (0.5 mL) and water (0.1 mL) were placed in a 10 mL vial. After the addition of 6 M HCl (50 μL), the vial was capped tightly and heated at 363 K for 60 h. After this time, blue crystals suitable for X-ray diffraction analysis were harvested in 22% yield based on the used organic ligand. The crystalline product is insoluble in the common organic solvents. Based on the single-crystal structure analysis, TGA and microanalysis, ZJNU-55 can be best formulated as [CuL]·2(CH3)2NH2·DMF. Elemental analysis for C28H33CuN5O9, calcd (%): C, 51.97; H, 5.14; N, 10.82. Found (%): C, 51.89; H, 5.21; N, 10.77. Selected FTIR (KBr, cm−1): 3421, 1622, 1574, 1419, 1363, 1246, 1107, 1022, 789, 768, 723, 660.
2.5 Fitting of pure-component isotherms
The pure component C2H2, CO2 and CH4 adsorption isotherm data measured at 278, 288 and 298 K were fitted with the singe-site Langmuir–Freundlich model
with the T-dependent parameter b
where q and qsat are the amounts adsorbed at equilibrium pressure p, and the monolayer saturation capacity in mmol g−1, b and v are Langmuir and Freundlich constants, respectively, and p is the pressure of the bulk gas in kPa. The Langmuir–Freundlich parameters are provided in Table S2. Fig. S8† provides a comparison of the experimental isotherm data for C2H2, CO2 and CH4 in ZJNU-55a with the isotherm fits.
2.6 Dynamic breakthrough experiment
The dynamic breakthrough experiments were performed at 298 K and 1 atm using a lab-scale fix-bed reactor. Detailed information of the setup can be found in our previous publication.11d In a typical experiment, 260 mg of ZJNU-55 was packed into a stainless steel column (4.0 mm I.D./6.4 mm O.D. × 130 mm) with silica wool filling the void space. The sorbent was activated in situ in the column with a vacuum pump at 298 K for 48 h. A helium flow (5 mL min−1) was introduced after the activation process to purge the adsorbent. The flow of He was then turned off while a gas mixture (50
:
50 C2H2–CH4 and 50
:
50 CO2–CH4) at 5 mL min−1 was allowed to flow into the column. The effluent from the column was monitored using a mass spectrometer (HPR 20).
3. Results and discussion
3.1 Synthesis and characterization
The organic ligand was conveniently synthesized by a Pd-catalyzed Suzuki cross-coupling reaction between 4,6-dichloro-2-methylpyrimidine and dimethyl 5-(pinacolboryl)isophthalate followed by hydrolysis and acidification in a moderate yield (see the Experimental section for synthetic details). Solvothermal reaction of CuCl2·2H2O and the organic ligand in a mixed solvent of DMF, MeOH and H2O at 363 K for 60 h in the presence of HCl afforded blue needle-shaped single crystals of ZJNU-55 with the molecular formula [CuL]·2(CH3)2NH2·DMF (Fig. S1†). Its structure was determined by single-crystal X-ray diffraction and the phase purity of the bulk materials was verified by a fairly good match of the experimental powder X-ray diffraction pattern with the one simulated from the single-crystal structure data (Fig. S2†). The TGA performed under a nitrogen atmosphere from 298 to 1073 K showed a weight loss of 25.1%, which corresponds to the loss of counterions and solvent molecules (calcd 25.5%) (Fig. S3†). FTIR spectra showed that the characteristic bands corresponding to the free carboxylate groups were not observed in the region of v = 1690–1730 cm−1 (Fig. S4†), indicating full deprotonation of the organic ligands.
3.2 Single-crystal structural analysis
The single-crystal X-ray diffraction reveals that ZJNU-55 crystallizes in the chiral hexagonal space group P62 with unit cell parameters of a = b = 22.7393(15) Å, c = 10.7316(10) Å. Apart from the guest molecules, the asymmetric unit consists of one crystallographic independent Cu2+ ion and one deprotonated organic ligand. Concerning the organic ligand, the nitrogen atoms in the central pyrimidine ring did not coordinate with the Cu2+ ions, whereas four carboxylate groups were fully deprotonated and participated in coordination with Cu2+ ions. Every ligand utilizes four carboxylate groups adopting the monodentate coordination mode to link four separated Cu2+ ions (Fig. 1a). Different from what was observed in MOFs constructed from the analogous diisophthalates where the organic ligand usually adopts two different conformations, the organic ligand is incorporated into the as-synthesized ZJNU-55 in only one conformation where the dihedral angles between terminal isophthalate moieties and the central pyrimidine ring are 36.43° and 42.37°, respectively. Regarding the inorganic secondary building unit (SBU), although it is well documented that the assembly of Cu2+ ions with the ligands containing carboxylate groups usually affords a binuclear dicopper paddlewheel cluster,8b,11b,14 the inorganic SBU in this compound is unexpectedly a mononuclear Cu(COO)4 in which the Cu(II) ion is coordinated by four carboxylate oxygen atoms (O1, O3#4, O5#1 and O8#5; symmetry codes: #1: 1 − x, 1 − y, z; #4: y, −x + y, −1/3 + z; #5: −x + y, 1 − x, −2/3 + z) from four different organic ligands in a square-planar coordination geometry, which is rarely observed in copper-multicarboxylate frameworks (Fig. 1b). The Cu–Ocarboxylate distances span from 1.903 to 1.972 Å, which is comparable to those observed in other copper complexes based on the carboxylic acids.15 Notably, this type of SBU is negatively charged, which results in a negative framework that needs positive ions to balance the whole charge of the structure. The combination of Cu(COO)4 with the deprotonated organic linker generates a non-interpenetrated three-dimensional network. Along the crystallographic c axis, two different types of channels (channel A and channel B) can be observed with the dimensions of ca. 2 and 8 Å, respectively, when the van der Waals radii of the atoms are taken into account (Fig. 1c). The nitrogen atoms as well as uncoordinated carboxylate oxygen atoms point towards the channels. It is notable that channel A can be viewed as an infinite nanotube which is comprised of four left-handed helical chains with a helical pitch of 42.926 Å and two right-handed helical chains with the helical pitch of 21.463 Å, which are weaved together by sharing the metal ions (Fig. 1d). These two types of helical chains are formed by alternatively linking isophthalate moieties and Cu2+ ions. The channels are occupied by the disordered solvent molecules and dimethylammonium counterions generated via decomposition of the DMF solvent, which could not be characterized owing to the very weak diffraction intensity. PLATON calculation indicates that the effective free volume of ZJNU-55 is 47.3% of the crystal volume (2270.9 Å of the 4805.62 Å3 unit cell volume) when the solvent molecules and counter ions are not considered.
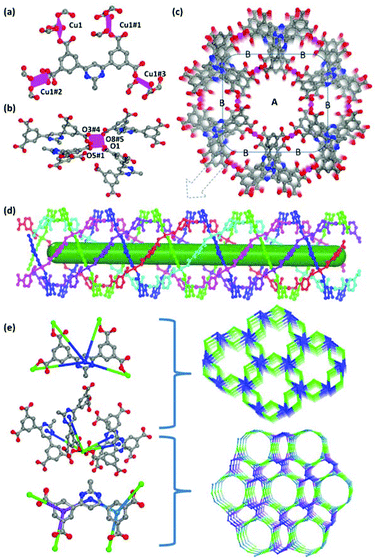 |
| Fig. 1 The coordination environment of the organic ligand (a) and the Cu(COO)4 unit (b) in ZJNU-55. Copper atoms are shown in the polyhedral model and the organic ligand was represented in the sphere and ball model. Symmetry codes: #1: 1 − x, 1 − y, z; #2: x − y, x, 1/3 + z; #3: 1 − y, 1 + x − y, 2/3 + z; #4: y, −x + y, −1/3 + z; #5: −x + y, 1 − x, −2/3 + z. (c) A three-dimensional network with two different types of channels A and B viewed along the crystallographic c axis. (d) A side view of channel A comprised of six helical chains sharing the metal ions. (e) Schematic representation of a 4-connected binodal net with the Schläfli symbol (4·62·83)(4·64·8) and a (3,4)-connected network with the Schläfli symbol (6·8·9)2(6·83·92). The hydrogen atoms are omitted for clarity. | |
From the perspective of a topological network, each Cu2+ ion links four organic ligands, and therefore can be regarded as a 4-connected node, while each organic ligand in turn connects with four Cu2+ ions and thus can also be taken as a 4-connected node. As a result, the whole framework can be rationalized as a novel 4-connected binodal net with the Schläfli symbol (4·62·83)(4·64·8) (Fig. 1e). Alternatively, if the organic ligand is treated as having a pair of three-connected nodes, the overall structure possesses a novel (3,4)-connected trinodal net with the Schläfli symbol (6·8·9)2(6·83·92), which is previously unreported.
3.3 Permanent porosity
Gas-adsorption measurements were performed on a Micromeritics 2020 HD88 surface-area-and-pore-size analyser. Before gas adsorption measurements, the as-synthesized sample was activated by multiple solvent exchanges with low-boiling-point dry acetone followed by evacuation under dynamic vacuum at 295 K for 24 h, generating the desolvated ZJNU-55a. No colour change after solvent removal also indicates the absence of the common dinuclear Cu(II) paddlewheel cluster in the structure, which is consistent with the above single-crystal structure analyses. PXRD analysis suggests that the activated sample is highly crystalline and retains its original structure (Fig. S2†). As shown in Fig. 2a, the activated ZJNU-55a exhibits a type-I N2 adsorption isotherm at 77 K, indicating a typical microporous material. At the maximum relative pressure, the N2 uptake capacity reaches 118 cm3 (STP) g−1, which corresponds to a pore volume of 0.1825 cm3 g−1. Deduced from nitrogen isotherm data, the Brunauer–Emmett–Teller (BET) and Langmuir surface areas are estimated to be 450.1 and 508.6 m2 g−1, respectively (Fig. S5†), which are relatively moderate compared to other reported copper-based MOFs constructed from angular diisophthalate linkers such as PCN-305–308 (1376–1927 and 2235–2929 m2 g−1 for BET and Langmuir surface areas),10PCN-124 (1372 and 2002 m2 g−1 for BET and Langmuir surface areas),11iNJU-Bai10 (2883 and 3107.9 m2 g−1 for BET and Langmuir surface areas),11h[Cu2L] (L = 1,3-bis(3,5-dicarboxylphenylethynyl)benzene, 1879 and 2489 m2 g−1 for BET and Langmuir surface areas),11j and PMOF-3 (1840 and 2020 m2 g−1 for BET and Langmuir surface areas).11k It should be mentioned that further increasing the activation temperature leads to inferior results probably due to the partial collapse of the framework (Fig. S6†), and therefore in this situation a room temperature of 295 K was employed as the optimum activation temperature.
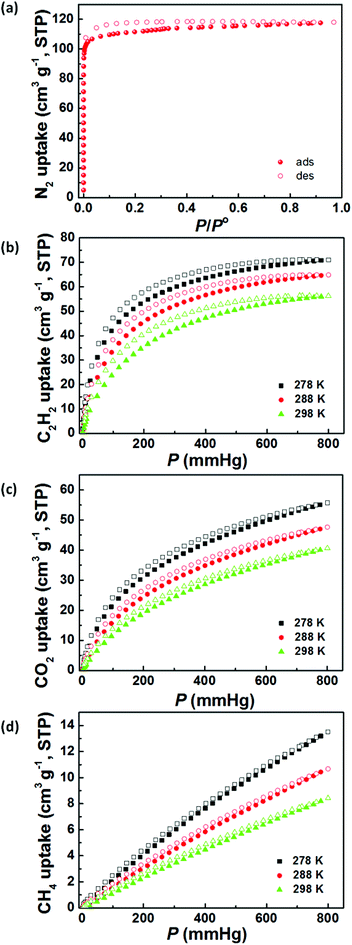 |
| Fig. 2 (a) N2 adsorption–desorption isotherm of ZJNU-55a at 77 K. Adsorption isotherms for (b) C2H2, (c) CO2 and (d) CH4 on ZJNU-55a at three different temperatures of 278 K, 288 K and 298 K. Solid and open symbols represent adsorption and desorption, respectively. | |
3.4 Selective gas adsorption
Acetylene produced by the thermal cracking of methane often contains unreacted methane, and efficient removal of CH4 from C2H2 has thus become an important issue. In addition, the separation of CO2 from CH4 is also an industrially important process for natural gas purification because the coexistence of CO2 with CH4 not only leads to pipeline corrosion but also reduces the energy content of natural gas. The conventional cryogenic distillation is very energy-intensive for the above two separation processes. A number of groups have made significant contributions towards this goal, demonstrating the advantages of MOFs for these separation processes.1i,6d,14d,16
The establishment of permanent porosity encourages us to explore its potential for C2H2/CH4 and CO2/CH4 separation. Accordingly, the pure-component C2H2, CO2 and CH4 sorption isotherms for ZJNU-55a were measured up to 1 atm at three different temperatures of 278 K, 288 K and 298 K. As shown in Fig. 2b–d, all isotherms, except the CH4 isotherm, show slightly hysteretic sorption behaviour. The isotherms are reproducible after five cycles (Fig. S7†). The most remarkable is that ZJNU-55a systematically adsorbs substantially more C2H2 and CO2 than CH4. At 298 K, for example, ZJNU-55a takes up a moderate amount of C2H2 (56.3 cm3 (STP) g−1, 66.3 mg g−1), and CO2 (40.6 cm3 (STP) g−1, 79.7 mg g−1), but basically a negligible amount of CH4 (8.4 cm3 (STP) g−1, 6.0 mg g−1) at 1 atm, indicating that ZJNU-55a is a promising material for C2H2/CH4 and CO2/CH4 separation at room temperature. The acetylene adsorption amount is higher than that for Cu2(pzdc)2(pyz), the first MOF material reported by Kitagawa and co-workers for acetylene adsorption (43 cm3 (STP) g−1 at RT and 1 atm),17 and comparable to that reported for Mn formate (51.2 cm3 (STP) g−1 at 298 K and 1 atm).16m Compared to PCN-307 (73.3 cm3 (STP) g−1 at 297 K and 1 bar),10ZJNU-55a shows a lower CO2 uptake capacity under similar conditions.
To evaluate the gas separation ability of ZJNU-55a, the ideal adsorbed solution theory (IAST) of Myers and Prausnitz18 was employed to predict the adsorption selectivities from the experimental pure-gas adsorption isotherms. Fig. 3a and b present the predicted adsorption selectivities for equimolar C2H2/CH4 and CO2/CH4 mixtures in ZJNU-55a as a function of bulk pressures. It can be seen that the C2H2/CH4 and CO2/CH4 adsorption selectivities decrease rapidly and then remain almost constant as the pressure increases, while the C2H2/CH4 and CO2/CH4 adsorption selectivities increase with decreasing temperatures. For an equimolar C2H2/CH4 mixture, the predicted selectivities are in the range of 39.2–64.9 for a range of pressures up to 110 kPa at 298 K (Fig. 3a), which is among the highest reported for MOFs (Table S3†). For an equimolar CO2/CH4 mixture, the predicted selectivities are in the range of 13.1–29.2 for a range of pressures up to 110 kPa at 298 K (Fig. 3b), which is significantly higher than that of PCN-307 (6.3–9.0 at 273 K).10 Such high selectivities further confirm that ZJNU-55a has a considerable potential in the adsorptive separation of C2H2/CH4 and CO2/CH4 gas mixtures.
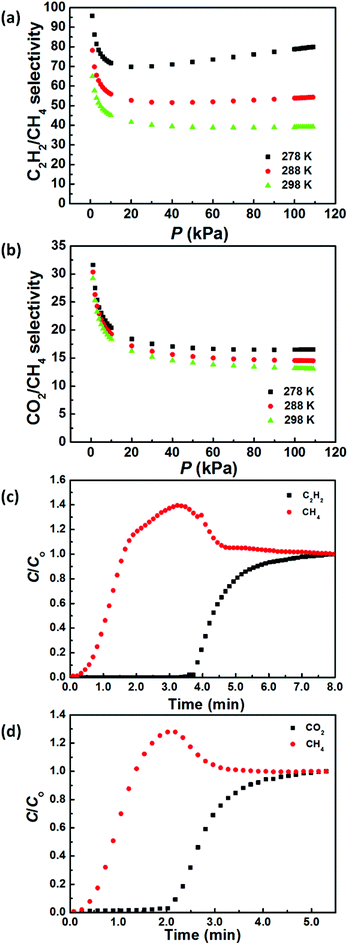 |
| Fig. 3 IAST selectivities of (a) C2H2/CH4 and (b) CO2/CH4 for equimolar binary gas mixtures in ZJNU-55a. The breakthrough curves for the equimolar C2H2/CH4 (c) and CO2/CH4 (d) gas mixtures at 298 K and 1 atm. | |
Dynamic breakthrough experiments were carried out to check the viability of using ZJNU-55a to separate equimolar C2H2/CH4 and CO2/CH4 gas mixtures. As shown in Fig. 3c and d, the separation of these two gas mixtures can be efficiently achieved. The dynamic C2H2 and CO2 uptake capacities calculated as a result of the breakthrough time were found to be 1.92 and 1.10 mmol g−1. Based on the adsorbed amount, the separation factors were calculated to be 26.4 and 7.1 for the equimolar C2H2/CH4 and CO2/CH4 gas mixtures, respectively.
To understand the affinities of these materials towards gas molecules, the coverage-dependent adsorption enthalpies were calculated using the Clausius–Clapeyron equation based on the pure-component adsorption isotherms measured at three different temperatures of 278 K, 288 K and 298 K. Fig. 4 presents the data on the isosteric heats of adsorption for C2H2, CO2 and CH4 in ZJNU-55a as a function of component loadings. At low coverage, the isosteric heats of adsorption are 42.40 ± 0.95, 35.44 ± 0.14 and 25.21 ± 0.99 kJ mol−1 for C2H2, CO2 and CH4, respectively. The Qst of CO2 is even higher than 22.836 kJ mol−1 observed for PCN-307.10 Moreover, the isosteric adsorption heat follows the trend: C2H2 > CO2 > CH4. As demonstrated in the MOF literature,16l the higher adsorption heats for CO2 and C2H2 might to be due to the ionic nature of the framework and the basic sites such as pyrimidine nitrogen and uncoordinated carboxylate oxygen atoms in the pore surface enforcing their stronger interactions with C2H2 and CO2 molecules, thus being responsible for the highly selective separation of C2H2 and CO2 over CH4.
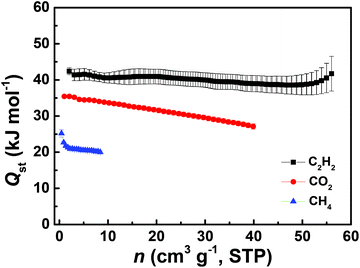 |
| Fig. 4 Dependence of the enthalpies of adsorption for C2H2, CO2 and CH4 with coverage. | |
4. Conclusions
In conclusion, a chiral anionic MOF with a novel topology was solvothermally synthesized from an angular diisophthalate ligand and CuCl2·2H2O. Although the ligand bears a close resemblance to the previously reported diisophthalate used to construct PCN-307, the resultant MOF's structure is totally different. The MOF is composed of a mononuclear Cu(COO)4 inorganic SBU, instead of a typical dinuclear Cu2(COO)4 paddlewheel unit, which is connected with the deprotonated organic ligand to form a novel network structure. More importantly, although the inorganic SBU is mononuclear Cu(COO)4, the framework after desolvation exhibits permanent porosity which has been established by various gas adsorption isotherms, showing the highly selectively adsorptive separation of C2H2 and CO2 over CH4. This is a rare example of a MOF constructed from mononuclear Cu(COO)4 for selective gas adsorption. This work also demonstrates that subtle ligand modification, in our case only simply substituting the central benzene ring with the pyrimidine group, can drastically impact the structures and gas adsorption properties of the resultant MOFs.
Acknowledgements
We gratefully acknowledge the financial support of the Natural Science Foundation of China (no. 21301156), the Qianjiang talents project in Zhejiang province, China (ZC304015017), and the Natural Science Foundation of Zhejiang province, China (LR16B010001).
Notes and references
-
(a) A. Schoedel, Z. Ji and O. M. Yaghi, Nat. Energy, 2016, 1, 16034 CrossRef;
(b) Q. Wang, J. Bai, Z. Lu, Y. Pan and X. You, Chem. Commun., 2016, 52, 443–452 RSC;
(c) Z. R. Herm, E. D. Bloch and J. R. Long, Chem. Mater., 2014, 26, 323–338 CrossRef CAS;
(d) Y. He, W. Zhou, G. Qian and B. Chen, Chem. Soc. Rev., 2014, 43, 5657–5678 RSC;
(e) Y. Yan, S. Yang, A. J. Blake and M. Schröder, Acc. Chem. Res., 2014, 47, 296–307 CrossRef CAS PubMed;
(f) Y. He, W. Zhou, T. Yildirim and B. Chen, Energy Environ. Sci., 2013, 6, 2735–2744 RSC;
(g) Y. He, W. Zhou, R. Krishna and B. Chen, Chem. Commun., 2012, 48, 11813–11831 RSC;
(h) J.-R. Li, J. Sculley and H.-C. Zhou, Chem. Rev., 2012, 112, 869–932 CrossRef CAS PubMed;
(i) K. Sumida, D. L. Rogow, J. A. Mason, T. M. McDonald, E. D. Bloch, Z. R. Herm, T.-H. Bae and J. R. Long, Chem. Rev., 2012, 112, 724–781 CrossRef CAS PubMed;
(j) H. Wu, Q. Gong, D. H. Olson and J. Li, Chem. Rev., 2012, 112, 836–868 CrossRef CAS PubMed;
(k) M. P. Suh, H. J. Park, T. K. Prasad and D.-W. Lim, Chem. Rev., 2012, 112, 782–835 CrossRef CAS PubMed;
(l) Y. He, R. Krishna and B. Chen, Energy Environ. Sci., 2012, 5, 9107–9120 RSC;
(m) S. Ma and H.-C. Zhou, Chem. Commun., 2010, 46, 44–53 RSC.
-
(a) Y. Cui, Y. Yue, G. Qian and B. Chen, Chem. Rev., 2012, 112, 1126–1162 CrossRef CAS PubMed;
(b) L. E. Kreno, K. Leong, O. K. Farha, M. Allendorf, R. P. V. Duyne and J. T. Hupp, Chem. Rev., 2012, 112, 1105–1125 CrossRef CAS PubMed.
- P. Horcajada, R. Gref, T. Baati, P. K. Allan, G. Maurin, P. Couvreur, G. Férey, R. E. Morris and C. Serre, Chem. Rev., 2012, 112, 1232–1268 CrossRef CAS PubMed.
-
(a) M. Zhao, S. Ou and C.-D. Wu, Acc. Chem. Res., 2014, 47, 1199–1207 CrossRef CAS PubMed;
(b) M. Yoon, R. Srirambalaji and K. Kim, Chem. Rev., 2012, 112, 1196–1231 CrossRef CAS PubMed;
(c) Y. Liu, W. Xuan and Y. Cui, Adv. Mater., 2010, 22, 4112–4135 CrossRef CAS PubMed;
(d) A. Corma, H. García and F. X. L. I. Xamena, Chem. Rev., 2010, 110, 4606–4655 CrossRef CAS PubMed;
(e) J. Lee, O. K. Farha, J. Roberts, K. A. Scheidt, S. T. Nguyen and J. T. Hupp, Chem. Soc. Rev., 2009, 38, 1450–1459 RSC;
(f) L. Ma, C. Abney and W. Lin, Chem. Soc. Rev., 2009, 38, 1248–1256 RSC.
- Y. He, B. Li, M. O'Keeffe and B. Chen, Chem. Soc. Rev., 2014, 43, 5618–5656 RSC.
-
(a) D. De, T. K. Pal, S. Neogi, S. Senthilkumar, D. Das, S. S. Gupta and P. K. Bharadwaj, Chem. – Eur. J., 2016, 22, 3387–3396 CrossRef CAS PubMed;
(b) M. Zhang, B. Li, Y. Li, Q. Wang, W. Zhang, B. Chen, S. Li, Y. Pan, X. You and J. Bai, Chem. Commun., 2016, 52, 7241–7244 RSC;
(c) C. Song, H. Liu, J. Jiao, D. Bai, W. Zhou, T. Yildirim and Y. He, Dalton Trans., 2016, 45, 7559–7562 RSC;
(d) Y. Yan, M. Juríček, F.-X. Coudert, N. A. Vermeulen, S. Grunder, A. Dailly, W. Lewis, A. J. Blake, J. F. Stoddart and M. Schröder, J. Am. Chem. Soc., 2016, 138, 3371–3381 CrossRef CAS PubMed;
(e) X. Liu, Z. Xiao, J. Xu, W. Xu, P. Sang, L. Zhao, H. Zhu, D. Sun and W. Guo, J. Mater. Chem. A, 2016, 4, 13844–13851 RSC;
(f) C. Song, J. Hu, Y. Ling, Y. Feng, D.-L. Chen and Y. He, Dalton Trans., 2015, 44, 14823–14829 RSC;
(g) N. H. Alsmail, M. Suyetin, Y. Yan, R. Cabot, C. P. Krap, J. Lü, T. L. Easun, E. Bichoutskaia, W. Lewis, A. J. Blake and M. Schröder, Chem. – Eur. J., 2014, 20, 7317–7324 CrossRef CAS PubMed;
(h) R.-R. Cheng, S.-X. Shao, H.-H. Wu, Y.-F. Niu, J. Han and X.-L. Zhao, Inorg. Chem. Commun., 2014, 46, 226–228 CrossRef CAS;
(i) C. Wang, L. Li, S.-F. Tang and X. Zhao, ACS Appl. Mater. Interfaces, 2014, 6, 16932–16940 CrossRef CAS PubMed;
(j) Q. Zhang, J. Yu, J. Cai, R. Song, Y. Cui, Y. Yang, B. Chen and G. Qian, Chem. Commun., 2014, 50, 14455–14458 RSC;
(k) Y. He, S. Xiang, Z. Zhang, S. Xiong, C. Wu, W. Zhou, T. Yildirim, R. Krishna and B. Chen, J. Mater. Chem. A, 2013, 1, 2543–2551 RSC;
(l) X. Rao, J. Cai, J. Yu, Y. He, C. Wu, W. Zhou, T. Yildirim, B. Chen and G. Qian, Chem. Commun., 2013, 49, 6719–6721 RSC;
(m) R. D. Kennedy, V. Krungleviciute, D. J. Clingerman, J. E. Mondloch, Y. Peng, C. E. Wilmer, A. A. Sarjeant, R. Q. Snurr, J. T. Hupp, T. Yildirim, O. K. Farha and C. A. Mirkin, Chem. Mater., 2013, 25, 3539–3543 CrossRef CAS;
(n) S. Ma, D. Sun, J. M. Simmons, C. D. Collier, D. Yuan and H.-C. Zhou, J. Am. Chem. Soc., 2008, 130, 1012–1016 CrossRef CAS PubMed;
(o) Y.-G. Lee, H. R. Moon, Y. E. Cheon and M. P. Suh, Angew. Chem., Int. Ed., 2008, 47, 7741–7745 CrossRef CAS PubMed.
- B. Chen, N. W. Ockwig, A. R. Millward, D. S. Contreras and O. M. Yaghi, Angew. Chem., Int. Ed., 2005, 44, 4745–4749 CrossRef CAS PubMed.
-
(a) X. Lin, I. Telepeni, A. J. Blake, A. Dailly, C. M. Brown, J. M. Simmons, M. Zoppi, G. S. Walker, K. M. Thomas, T. J. Mays, P. Hubberstey, N. R. Champness and M. Schröder, J. Am. Chem. Soc., 2009, 131, 2159–2171 CrossRef CAS PubMed;
(b) X. Lin, J. Jia, X. Zhao, K. M. Thomas, A. J. Blake, G. S. Walker, N. R. Champness, P. Hubberstey and M. Schröder, Angew. Chem., Int. Ed., 2006, 45, 7358–7364 CrossRef CAS PubMed.
-
(a) C. Song, Y. Ling, L. Jin, M. Zhang, D.-L. Chen and Y. He, Dalton Trans., 2016, 45, 190–197 RSC;
(b) C. Song, J. Jiao, Q. Lin, H. Liu and Y. He, Dalton Trans., 2016, 45, 4563–4569 RSC;
(c) C. Song, J. Hu, Y. Ling, Y. Feng, R. Krishna, D.-L. Chen and Y. He, J. Mater. Chem. A, 2015, 3, 19417–19426 RSC.
- Y. Liu, J.-R. Li, W. M. Verdegaal, T.-F. Liu and H.-C. Zhou, Chem. – Eur. J., 2013, 19, 5637–5643 CrossRef CAS PubMed.
-
(a) Z. Lu, J. Bai, C. Hang, F. Meng, W. Liu, Y. Pan and X. You, Chem. – Eur. J., 2016, 22, 6277–6285 CrossRef CAS PubMed;
(b) B. Liu, S. Yao, C. Shi, G. Li, Q. Huo and Y. Liu, Chem. Commun., 2016, 52, 3223–3226 RSC;
(c) T. K. Pal, D. De, S. Senthilkumar, S. Neogi and P. K. Bharadwaj, Inorg. Chem., 2016, 55, 7835–7842 CrossRef CAS PubMed;
(d) J. Jiao, L. Dou, H. Liu, F. Chen, D. Bai, Y. Feng, S. Xiong, D.-L. Chen and Y. He, Dalton Trans., 2016, 45, 13373–13382 RSC;
(e) T. K. Pal, D. De, S. Neogi, P. Pachfule, S. Senthilkumar, Q. Xu and P. K. Bharadwaj, Chem. – Eur. J., 2015, 21, 19064–19070 CrossRef CAS PubMed;
(f) B. Zheng, X. Lin, Z. Wang, R. Yun, Y. Fan, M. Ding, X. Hua and P. Yi, CrystEngComm, 2014, 16, 9586–9589 RSC;
(g) Y. Xie, H. Yang, Z. U. Wang, Y. Liu, H.-C. Zhou and J.-R. Li, Chem. Commun., 2014, 50, 563–565 RSC;
(h) Z. Lu, L. Du, K. Tang and J. Bai, Cryst. Growth Des., 2013, 13, 2252–2255 CrossRef CAS;
(i) J. Park, J.-R. Li, Y.-P. Chen, J. Yu, A. A. Yakovenko, Z. U. Wang, L.-B. Sun, P. B. Balbuena and H.-C. Zhou, Chem. Commun., 2012, 48, 9995–9997 RSC;
(j) P. Zhang, B. Li, Y. Zhao, X. Meng and T. Zhang, Chem. Commun., 2011, 47, 7722–7724 RSC;
(k) X. Liu, M. Park, S. Hong, M. Oh, J. W. Yoon, J.-S. Chang and M. S. Lah, Inorg. Chem., 2009, 48, 11507–11509 CrossRef CAS PubMed.
- C. Song, Y. Ling, Y. Feng, W. Zhou, T. Yildirim and Y. He, Chem. Commun., 2015, 51, 8508–8511 RSC.
-
(a) A. L. Spek, Acta Crystallogr., Sect. D: Biol. Crystallogr., 2009, 65, 148–155 CrossRef CAS PubMed;
(b) A. L. Spek, Acta Crystallogr., Sect. C: Cryst. Struct. Commun., 2015, 71, 9–18 CrossRef CAS PubMed.
-
(a) P.-Z. Li, X.-J. Wang, J. Liu, J. S. Lim, R. Zou and Y. Zhao, J. Am. Chem. Soc., 2016, 138, 2142–2145 CrossRef CAS PubMed;
(b) R. Luebke, Ł. J. Weseliński, Y. Belmabkhout, Z. Chen, Ł. Wojtas and M. Eddaoudi, Cryst. Growth Des., 2014, 14, 414–418 CrossRef CAS;
(c) K. Liu, B. Li, Y. Li, X. Li, F. Yang, G. Zeng, Y. Peng, Z. Zhang, G. Li, Z. Shi, S. Feng and D. Song, Chem. Commun., 2014, 50, 5031–5033 RSC;
(d) Y. He, Z. Guo, S. Xiang, Z. Zhang, W. Zhou, F. R. Fronczek, S. Parkin, S. T. Hyde, M. O'Keeffe and B. Chen, Inorg. Chem., 2013, 52, 11580–11584 CrossRef CAS PubMed;
(e) O. K. Farha, I. Eryazici, N. C. Jeong, B. G. Hauser, C. E. Wilmer, A. A. Sarjeant, R. Q. Snurr, S. T. Nguyen, A. Ö. Yazaydın and J. T. Hupp, J. Am. Chem. Soc., 2012, 134, 15016–15021 CrossRef CAS PubMed;
(f) U. Stoeck, S. Krause, V. Bon, I. Senkovska and S. Kaskel, Chem. Commun., 2012, 48, 10841–10843 RSC;
(g) Y. He, Z. Zhang, S. Xiang, H. Wu, F. R. Fronczek, W. Zhou, R. Krishna, M. O'Keeffe and B. Chen, Chem. – Eur. J., 2012, 18, 1901–1904 CrossRef CAS PubMed;
(h) X.-S. Wang, L. Meng, Q. Cheng, C. Kim, L. Wojtas, M. Chrzanowski, Y.-S. Chen, X. P. Zhang and S. Ma, J. Am. Chem. Soc., 2011, 133, 16322–16325 CrossRef CAS PubMed;
(i) L. Ma, A. Jin, Z. Xie and W. Lin, Angew. Chem., Int. Ed., 2009, 48, 9905–9908 CrossRef CAS PubMed;
(j) S. S.-Y. Chui, S. M.-F. Lo, J. P. H. Charmant, A. G. Orpen and I. D. Williams, Science, 1999, 283, 1148–1150 CrossRef CAS PubMed.
- A. G. Wong-Foy, O. Lebel and A. J. Matzger, J. Am. Chem. Soc., 2007, 129, 15740–15741 CrossRef CAS PubMed.
-
(a) L. Li, X. Wang, J. Liang, Y. Huang, H. Li, Z. Lin and R. Cao, ACS Appl. Mater. Interfaces, 2016, 8, 9777–9781 CrossRef CAS PubMed;
(b) H.-R. Fu, F. Wang and J. Zhang, Dalton Trans., 2015, 44, 2893–2896 RSC;
(c) Q.-G. Zhai, N. Bai, S. N. Li, X. Bu and P. Feng, Inorg. Chem., 2015, 54, 9862–9868 CrossRef CAS PubMed;
(d) K. Liu, D. Ma, B. Li, Y. Li, K. Yao, Z. Zhang, Y. Han and Z. Shi, J. Mater. Chem. A, 2014, 2, 15823–15828 RSC;
(e) Y. He, C. Song, Y. Ling, C. Wu, R. Krishna and B. Chen, APL Mater., 2014, 2, 124102 CrossRef;
(f) H. Xu, Y. He, Z. Zhang, S. Xiang, J. Cai, Y. Cui, Y. Yang, G. Qian and B. Chen, J. Mater. Chem. A, 2013, 1, 77–81 RSC;
(g) Z. Zhang, S. Xiang, K. Hong, C. D. Madhab, H. D. Arman, M. Garcia, J. U. Mondal, K. M. Thomas and B. Chen, Inorg. Chem., 2012, 51, 4947–4953 CrossRef CAS PubMed;
(h) Y. He, Z. Zhang, S. Xiang, F. R. Fronczek, R. Krishna and B. Chen, Chem. – Eur. J., 2012, 18, 613–619 CrossRef CAS PubMed;
(i) Z. Chen, S. Xiang, H. D. Arman, J. U. Mondal, P. Li, D. Zhao and B. Chen, Inorg. Chem., 2011, 50, 3442–3446 CrossRef CAS PubMed;
(j) M. C. Das, H. Xu, S. Xiang, Z. Zhang, H. D. Arman, G. Qian and B. Chen, Chem. – Eur. J., 2011, 17, 7817–7822 CrossRef CAS PubMed;
(k) Z. Chen, S. Xiang, H. D. Arman, P. Li, S. Tidrow, D. Zhao and B. Chen, Eur. J. Inorg. Chem., 2010, 3745–3749 CrossRef CAS;
(l) E. Q. Procopio, F. Linares, C. Montoro, V. Colombo, A. Maspero, E. Barea and J. A. R. Navarro, Angew. Chem., Int. Ed., 2010, 49, 7308–7311 CrossRef PubMed;
(m) D. G. Samsonenko, H. Kim, Y. Sun, G.-H. Kim, H.-S. Lee and K. Kim, Chem. – Asian J., 2007, 2, 484–488 CrossRef CAS PubMed.
- R. Matsuda, R. Kitaura, S. Kitagawa, Y. Kubota, R. V. Belosludov, T. C. Kobayashi, H. Sakamoto, T. Chiba, M. Takata, Y. Kawazoe and Y. Mita, Nature, 2005, 436, 238–241 CrossRef CAS PubMed.
- A. L. Myers and J. M. Prausnitz, AIChE J., 1965, 11, 121–127 CrossRef CAS.
Footnote |
† Electronic supplementary information (ESI) available: Photograph of the crystals of ZJNU-55 (Fig. S1), PXRD (Fig. S2), TGA (Fig. S3), FTIR (Fig. S4), BET and Langmuir plots (Fig. S5), N2 adsorption isotherms at 77 K of ZJNU-55 activated at different temperatures (Fig. S6), five cycles of C2H2 adsorption at 298 K (Fig. S7), comparison of the pure-component isotherm data with the fitted isotherms (Fig. S8), isosteric plots for C2H2, CO2 and CH4 adsorption (Fig. S9), 1H and 13C NMR spectra (Fig. S10), crystal data and structure refinement for ZJNU-55 (Table S1), Langmuir–Freundlich parameters for adsorption of C2H2, CO2, and CH4 in ZJNU-55a (Table S2). CCDC 1492066. For ESI and crystallographic data in CIF or other electronic format see DOI: 10.1039/c6qi00245e |
|
This journal is © the Partner Organisations 2016 |