DOI:
10.1039/C3RA48018F
(Paper)
RSC Adv., 2014,
4, 15040-15047
Lanthanide-doped nanoparticles for specific recognition of toll-like receptor (TLR) in human neutrophils†
Received
27th December 2013
, Accepted 14th March 2014
First published on 17th March 2014
Abstract
KYF4:Yb,Er nanoparticles were fabricated and functionalized with streptavidin for the recognition of toll-like receptors (TLRs) on human neutrophil through biotinylated lipopolysaccharide (biotin–LPS). X-Ray diffraction, Infrared spectroscopy, Transmission Electron Microscopy and Agarose Gel Electrophoresis were used to characterize the as-prepared and functionalized nanoparticles. Confocal Scanning Laser Microscopy was applied to study the TLR specific recognition using KYF4:Yb,Er and the uptake of the nanoparticles functionalized with BSA/Dextran–streptavidin in the presence of biotin–LPS by human neutrophils under normal (37 °C) and cell stressing conditions (4 °C). Confocal microscopy studies showed that the uptake of the functionalized KYF4:Yb,Er nanoparticles occurs faster and with a higher rate at 37 °C compared to the uptake at 4 °C, indicating that the uptake mechanism is an energy dependent process. Research reported in this work provides relevant guidance for the development of lanthanide doped KYF4:Yb,Er nanoparticles as specific intracellular probes, allowing control of the nanoparticle–cell interactions by tuning the surface properties. Furthermore, our results would pave the way to the use of lanthanide-doped materials for imaging cellular processes such as uptake of nanoparticles and recognition of receptors involved in immune responses.
Introduction
Neutrophils, as an integral part of the human immune system, perform several important roles by engaging in early defense mechanisms against invasive microorganisms and pathogens. Neutrophils rapidly can be found in the infection sites, limiting their spread and subsequently allowing recruitment and activation of other immune cells. As a result, pathogens are cleared and the immune response is triggered.1,2 Moreover, neutrophils are able to discriminate between self and foreign molecules and microbial pathogens, a mechanism which relies on the evolutionary conserved family of the proteins called toll-like receptors (TLRs). TLRs are type I transmembrane proteins comprising of an amino-terminal leucine-rich repeat (LRR) domain and a carboxyl-terminal Toll-interleukin-1 receptor (TIR) domain (Scheme 1), wherein LRR domain is predominantly responsible for pathogen-associated microbial patterns (PAMP) recognition and the TIR domain is involved in intracellular signaling.3,4 In mammalians, TLRs play a critical role in the early innate and adaptive immune responses through production of proinflammatory cytokines and chemokines, upregulation of co-stimulatory molecules and activation of antigen presentation by recognizing highly conserved structural motifs known as PAMPs, which include various bacterial cell wall components such as lipopolysaccharide (LPS),5 peptidoglycan and lipopeptides, as well as flagellin, bacterial DNA and viral double-stranded RNA.6,7
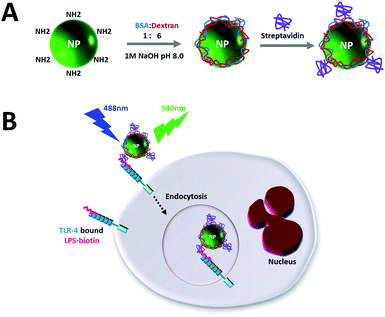 |
| Scheme 1 (A) Functionalization of UCNP (not to scale). (B) Recognition of biotin–LPS bound TLR4 and internalization of functionalized UCNP into neutrophils. | |
To date, numerous luminescent materials, including fluorescent proteins,8 organic dyes,9 and semiconductor quantum dots (QDs),10,11 have been developed as fluorescent probes for bio-imaging of TLR based on the single-photon excitation: emitting low energy fluorescence when excited by high energy light. In the last decade, rare-earth or lanthanide-doped up-conversion nanoparticles (UCNP) are emerging as a promising alternative to the conventional fluorescent labels due to their unique photo-physical properties such as converse excitation and emission profiles wherein they are excited at near infrared (NIR) wavelength of 980 nm and emit at higher energy in the visible range or at shorter NIR wavelength.12 This phenomenon allows notable tissue penetration depth (>3.2 cm), a fluorescence intensity increase of 6–8 orders of magnitude brighter than conventional fluorescence based imaging probes in two-photon processes, and use with minimal light scattering and background from the surrounding biological tissue.13,14 UCNP are being currently used in targeting and labeling of stem cells,15 tumor derived cells,16 cell surface antigens, diagnostic,17 immunohistochemistry assays,18 detection of pathogenic bacteria,19 and reporters in nucleic acid microarrays.20 Although the specific endocytic mechanism by which cells internalize nanoparticles such as UCNP is still a subject under debate, the mechanism of cellular uptake is important in the field of nanomedicine, cancer diagnostic, drug delivery and treatment. The application of UCNP nanoparticles in bioimaging represents a versatile approach. It is known that UCNP exhibit fluorescence at both the original wavelength of 980 nm, in which case they require a novel laser diode emitting at 980 nm as excitation source, and at the second-harmonic wavelength of 488 nm, respectively.21–23 The latter case implies the manipulation of UCNP on conventional imaging systems. This is attributed to the direct Er3+ excitation at the harmonic wavelength with indirect Yb3+ excitation, in contrast to the direct excitation of Yb3+ and the various up-conversion energy transfer processes at 980 nm.23 The different probability of emitting wavelength (either green 540 nm or red 660 nm) is attributed to the smaller number of photons required to fill up the energy mismatch among the energy transfer processes (Scheme SI1†).24 The use of UCNP excited at 488 nm is similar to that of organic dyes or quantum dots in terms of photoluminescent processes involved. In contrast, UCNP display exceptionally longer luminescence lifetimes, i.e. microseconds versus nanoseconds.25 This characteristic, together with the drawbacks of the organic dyes (poor photochemical stability, low quantum yield, easy deterioration in biological environments) or quantum dots (blinking, toxicity),26,27 makes UCNP excellent candidates for cell imaging and tracking of molecules. The immune system relies on a vast array of non-clonally expressed pattern recognition receptors for the detection of pathogens. Pattern recognition receptors bind conserved molecular structures shared by large groups of pathogens, termed pathogen-associated molecular patterns. The TLRs are a recently discovered family of pattern recognition receptors. Herein, we report the conjugation of UCNP (KYF4:Yb,Er) with streptavidin as the final coating element to recognize TLR in neutrophils through biotinylated lipopolysaccharide (LPS). This step is a prerequisite in elucidating unresolved questions about the detailed molecular UCNP uptake mechanism by human neutrophils.
Experimental
Synthesis of KYF4:Yb,Er–APTMS
For the synthesis of KYF4:Yb,Er, the reagents were purchased from Sigma-Aldrich Química S.L. (Spain) and used without any modification. 12 mmol KF were dissolved in 20 mL methanol, in a 50 mL round bottom flask, and heated up to 65 °C. In a separated flask, 3.89 mmol YCl3·6H2O, 0.72 mmol YbCl3·6H2O and 0.24 mmol ErCl3·6H2O were dissolved in 10 mL methanol and added dropwise to the first solution. The resulting solution was kept at 65 °C with 800 rpm stirring for 2 hours. Further, the solution was centrifuged and washed three times with methanol. For the addition of amino functional group, the method by Gorris et al. was followed28 with some modifications. 80.5 mg KYF4:Yb,Er were dissolved in 13.5 mL toluene and 403 μL of APTMS (3-aminopropyl-trimethoxysilane) were added. This was kept under N2 with stirring for 24 hours. Then, the mixture was centrifuged for 20 min at 4000 rpm and washed three times with a mixture ethanol
:
acetone (1
:
1). The pellet was dried overnight at 60 °C.
Biofunctionalization of KYF4:Yb,Er–APTMS with streptavidin
1.2 mg of KYF4:Yb,Er–APTMS were dissolved in a vial containing 1 mL Phosphate Buffer Saline (PBS). To this vial, 1 mL of glutaraldehyde (0.25 mg mL−1) was added and the mixture was stirred at 37 °C for 1 hour at 300 rpm. The resulting solution was centrifuged and the pellet washed three times with PBS. 5 mg of Bovine Serum Albumin (BSA; Sigma, Germany) was mixed with 5 mg of Dextran (Fluka, Germany) in a molar ratio of 1
:
6 in water at pH 8.0 adding 0.1 mL of NaOH 1 M in a total volume of 1 mL.29 0.5 mL of the mixture was added to 0.5 mL of glutaraldehyde-functionalized nanoparticles and stirred for 1 hour at 750 rpm. Then, the mixture was centrifuged and the BSA/Dextran-functionalized nanoparticles (BSA/Dex) were resuspended in 0.5 mL PBS. Next, 0.5 mL of 0.1, 0.5, 1.0, 2.5 and 5.0 mg mL−1 of streptavidin (Cat. no. 21135, Pierce, Thermo Scientific), respectively, were added to UCNP–BSA–Dextran samples. The mixtures were stirred for 1 hour at 750 rpm, then centrifuged and washed three times with PBS, and stored in deionized water.
Powder X-ray diffraction patterns (XRD)
Powder X-ray diffraction patterns (XRD) were measured in a Bruker AXS D8 Advance diffractometer equipped with a Cu tube, a Ge (111) incident beam monochromator, and a Vantec-1 PSD. Data were recorded in the range 5–70° 2θ with an angular step size of 0.016° and a counting time of 6 s per step.
Fourier transform infrared (FTIR) spectroscopy
Fourier transform infrared (FTIR) spectroscopy was carried out in a Bruker Optics ALPHA spectrometer equipped with an ATR Platinum Diamond unit and Shimazdu IRAffinity-1 with a Michelson interferometer. Spectra were collected in the range 400–4000 cm−1 by co-addition of 32 scans at a nominal resolution of 4 cm−1, taking the spectrum of the empty cell as the background.
Transmission electron microscopy (TEM)
Transmission Electron Microscopy (TEM) was carried out in a JEOL JEM-1011 microscope operating at 100 kV and equipped with a SIS Megaview III CCD camera. A few droplets of the sample suspended in ethanol were placed on a carbon-coated copper grids followed by evaporation at ambient conditions.
Photoluminescence (PL) spectra
Photoluminescence (PL) spectra of the KYF4:Yb,Er and KYF4:Yb,Er–APTMS nanoparticles were recorded, using a 980 nm excitation source attached to a Spectro-fluorimeter Fluorolog, from Horiba Jobin Yvon, equipped with a PMT detector.
Energy dispersive X-ray spectroscopy (EDX)
Energy Dispersive X-Ray Spectroscopy (EDX) was carried out at low voltage (20 K) in a FEI Quanta 600 microscope equipped with an EDX detector from Oxford Instruments.
Zeta potential (ζ-potential)
Zeta potential (ζ-potential) of functionalized UCNP was measured with a NanoSizer (MALVERN Nano-ZS) with a cell drive voltage of 30 V. All measurements were performed at 25 °C.
Agarose gel electrophoresis (AGE)
Gels were hand cast with agarose in the appropriate buffers. 0.5 g of agarose was briefly boiled in 100 mL of TBE (Tris-Boric-EDTA) buffer 1× (mixing 10 g of Tris–HCl, 5.5 g of boric acid and 4 mL of 0.5 M EDTA (Ethylene diamine tetraacetic acid) in a total volume of 1 L of deionized water), cooled and poured into a standard 7 cm2 gel tray. AGE was performed with the Owl Easycast B1 (Thermo Scientific) in which gels were horizontally immersed in TAE (Tris-Acetic-EDTA) (mixing 4.8 g Tris–HCl, 1.14 g acetic acid and 2 mL of 0.5 M EDTA in a total volume of 1 L deionized water) buffer 1× for electrophoresis at 90 V for 90 min. Samples were diluted in 30% glycerol. For UCNP visualization, gels were placed on a UV transilluminator (molecular imager Gel Doc XR+ (BioRad)) under UV excitation at 405 nm.
Isolation of neutrophils from human blood
Heparinized whole blood (20–30 mL) was collected from healthy human donors. After dextran sedimentation, granulocytes were isolated from the supernatant leukocyte-rich plasma by Ficoll–Hypaque gradient centrifugation and red blood cells were lysed with ammonium chloride solution.
Cellular uptake of the UCNP by neutrophils and Trypan Blue exclusion test for neutrophil viability
Neutrophils were incubated alone as a control of autofluorescence for 10 min (at 37 °C and 4 °C). For the rest of experiments, the neutrophils were incubated at 37 °C and 4 °C with 50 μL 1.2 mg mL−1 of UCNP–APMTS or UCNP–BSA/dextran–streptavidin in RPMI medium for 0, 2.5, 5 and 10 min in order to follow the uptake. In the case of the experiments for the specific recognition, neutrophils were preincubated with 0.1 mg mL−1 of biotinylated-LPS (from E. coli O111:B4, Cat. Code tlrl-bblps, InvivoGen, Toulouse, France) for 10 min at 4 °C. For all the samples, the nucleus was stained with DRAQ5 (Cat. no. DR50200, BioStatus Limited, Leicestershire, United Kingdom) dye (excitation at 633 nm and emission at 670 nm). Imaging was performed by confocal microscopy (Olympus Fluoview FV 1000) and imaging parameters were kept constant throughout the experiments. For quantitative image analysis ImageJ was used.29 Briefly, using ImageJ selection and drawing tools, a region of interest was chosen on each cell in a background corrected confocal fluorescence image. Minimum of ten cells were analyzed in each image from a set of three separate images from three biological replicates and corrected total cell fluorescence was calculated. Neutrophil viability upon their incubation with UCNPs was determined by Trypan Blue dye exclusion test based on a previously established protocol.30 Briefly, 1.2 mg mL−1 UCNP–APTMS and UCNP–APTMS–BSA/Dex–Strv were co-incubated with neutrophils in the absence and presence of biotinylated-LPS respectively for 10, 30, 60 and 180 min at 37 °C. After respective incubation time intervals neutrophils were washed and resuspended in RPMI media and were incubated with equal volume of 0.5% Trypan Blue (cat. no. L6323, Biochrom GmbH, Berlin, Germany) solution. After three minutes unstained (viable) and stained (nonviable) neutrophil count was determined using a hemacytometer.
Results and discussion
Nanoparticle characterization and functionalization
Powder XRD analysis of the as-such KYF4:Yb,Er up-conversion nanoparticles is shown in Fig. 1A. Two crystalline phases were identified: one corresponding to hexagonal KYF4 (JCPDS 27-466) and the second one to the cubic KY3F10 form (JCPDS 27-465).31 The high crystallinity of the sample is an indicative of small particles. This observation was also confirmed by the TEM measurements (Fig. 1B). The polyhedral crystals are uniform in size with very low dispersity (see inset). The average size of KYF4:Yb,Er nanoparticles as indicated by the size histogram, determined from the electron microscopy analyses, was around 12 nm (Fig. 1C).
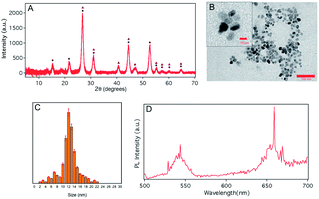 |
| Fig. 1 Nanoparticle characterization (A) XRD pattern of KYF4:Yb,Er. Symbols: KYF4 (dots), KY3F10 (triangles). (B) TEM image of KYF4:Yb,Er. (C) Size histogram of KYF4:Yb,Er. (D) Fluorescence spectrum of KYF4:Yb,Er nanoparticles; excitation under 980 nm light. | |
The PL emission under 980 nm excitation presents two peaks (Fig. 1D), one centered at 540 nm, corresponding to the 2H11/2 → 4I15/2 and 4S3/2 → 4I15/2 transitions, and another one around 660 nm, corresponding to the 4F9/2 → 4I15/2 transitions, all of them transitions of Er3+.32 These two peaks are in good agreement with the emission wavelength in the energy level diagram in the Scheme SI1.† The lambda scan of UCNP excited using 488 nm laser by confocal laser scanning microscopy (Movie in the ESI†) shows two emission peaks, i.e. 490–540 nm and 590–605 nm. In contrast to the predominant red emission at 660 nm obtained by excitation at 980 nm, excitation at the harmonic wavelength revealed a stronger green light emission, in agreement with previous studies using Yb,Er-doped nanocrystals at the same excitation wavelength.33 The reason is that the electrons reach the 4F7/2 level of Er3+ ion directly under 488 nm excitation.
Then they undergo a non-radiative process to reach the 2H11/2 and 4S3/2 levels, and only a few reach the 4F9/2 level at the same time. Therefore, the strong green emission corresponding to the 2H11/2, 4S3/2 → 4I15/2 transition and the weak red light corresponding to the 4F9/2 → 4I15/2 transition can be observed, respectively.34
The spectrum profile of the elemental mapping by EDX clearly shows the presence of the metals (Fig. SI1†). The detector measures the relative abundance versus their energy. The quantitative analysis gave the molecular weight of the nanoparticles, that summed up around 2000 g mol−1, with a molar ratio Yb/Er = 3.43, which is in agreement with the theoretical ratio of Yb/Er = 3. The results demonstrate that the rare earth metals (Yb,Er) were successfully incorporated during the synthesis step. The charge was determined by means of zeta potential measurements, giving a result of +14.34 meV for the KYF4:Yb,Er–APTMS nanoparticles. Z-potential results were summarized in Table SI1.†
Infrared spectra of the nanoparticles are shown in Fig. 2. After the functionalization of bare UCNP with APTMS, new bonds between the hydroxyl group from the UCNP and the Si from the APTMS are formed, corresponding to the doublet at 1000–1100 cm−1 (Si–O) and with the disappearance of the band at 3400 cm−1 indicating the conjugation of the OH. Besides the formation of the Si–O bond, new bands can be observed by IR. The NH2 terminal group of APTMS appears at 1570 cm−1 corresponding to the scissor vibration, while the bands at 1490 and 1610 cm−1 can be assigned to a symmetric deformation mode of NH3+. The propyl chain of APTMS is correlated with the stretching modes of C–H at 1380, 2850 and 2910 cm−1, as well as with the stretching modes from the C–N at 1200 cm−1.35 The next step of functionalization implies the interaction of UCNP–APTMS nanoparticles with glutaraldehyde leading to UCNP–APTMS–glutaraldehyde, forming an imine between the aldehyde from the glutaraldehyde and the free amine from the nanoparticles with the consequent apparition of a new band at 1638 cm−1. The stretching mode of free aldehyde appears at 1700 cm−1, as shown by the inset in Fig. 2. In addition, the bands from the N–H vibration and deformation decrease in intensity as the free amine reacts.36 When UCNP–APTMS–glutaraldehyde nanoparticles were incubated with BSA/Dextran, the free aldehyde groups of UCNP–APTMS–glutaraldehyde interacted with the amine groups of BSA forming another imine. In consequence, the band at 1638 cm−1 gets more intense, while the bands from the free aldehyde in the glutaraldehyde disappeared. Additional vibration bands corresponding to the stretching of the free carboxy groups of the BSA (2800–3500 cm−1), the stretching of the C
O (1717 cm−1), and the deformation vibration of the C–OH (950 cm−1) are also visible. At 1556 cm−1, a peak from the amide groups of the BSA is present. The final step of functionalization entailed the conjugation of the streptavidin to the BSA. The vibration bands of the proteins: peptide bond COOH to NH2 between streptavidin and BSA can be observed at 1740 cm−1.37 Peaks representing amide bonds of streptavidin are detected near 1500 cm−1 and 1600 cm−1. The very broad peak in the region 3100–3650 cm−1 indicates the presence of exchangeable protons from the amide peptide groups.38
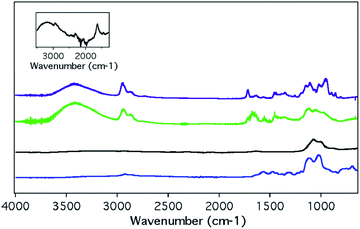 |
| Fig. 2 Infrared spectra of UCNP–APTMS (blue), UCNP–APTMS–glutaraldehyde (black), UCNP–APTMS–glutaraldehyde–BSA/Dex (green) and UCNP–APTMS–glutaraldehyde–BSA/Dex–Strv (purple), respectively. | |
Agarose gel electrophoresis was used to determine relative sizes and molecular weights of functionalized UCNP and to further investigate specific streptavidin labeling of the UNCPs. Fig. SI2† shows the AGE results carried with the materials obtained after each functionalization step. UCNP–APTMS migrated toward the cathode due to their positive charge provided by the APTMS (Fig. SI2,† lane 1). UCNP–APTMS nanoparticles functionalized with BSA (Fig. SI2,† lane 2), migrated still toward the cathode.
Functionalized with dextran (Fig. SI2,† lane 3), the nanoparticles migrated in both directions, to the cathode and anode, indicating that not all nanoparticles were functionalized. In both cases, with BSA and with dextran (Fig. SI2,† lanes 2–3, respectively), we can see fluorescence in the well, as a result of NP that remained there.
This observation indicates they were not effectively functionalized and form large aggregates that are not able to migrate through the gel. After functionalization with a mixture of BSA/Dex (ratio 1
:
6) (Fig. SI2,† lane 4), we observed a very good functionalization and all the nanoparticles migrated towards the anode due to the negative charges provided by BSA/Dex. Further, adding streptavidin to the UCNP with BSA/Dex (Fig. SI2,† lane 5) resulted also in migration. The screening of the NP with different surface functionalization by AGE revealed UCNP–APTMS–BSA/Dex–Strv as the most effective in view of further cellular uptake experiments. Next step was to determine the optimal amount of streptavidin.
Taking this into account, different streptavidin concentrations in the range 0.1–5.0 mg mL−1 were used after BSA/Dex functionalization. Illustrated in Fig. 3, the as-such UCNP–APTMS still migrated toward the cathode due to their positive charge provided by the APTMS, but the rest of the samples migrated toward the anode due to their negative charge as a consequence of the functionalization. The higher is the amount of streptavidin, the lower is the migration of the nanoparticles due to the increase in weight. We observed that for BSA/Dex (Fig. SI2†) and BSA/Dex–Strv, due to lower concentrations of streptavidin (Fig. 3), there was a tail in the migration, indicating different net negative charge, in agreement with the results from zeta-potential measurements. However, at the highest concentration of streptavidin, all the nanoparticles were concentrated in the migrating band, as a result of their identical charge (Fig. 3, lane 6).
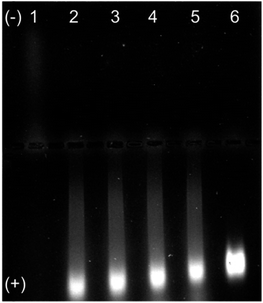 |
| Fig. 3 Electrophoresis of UCNP before and after functionalization with proteins. Lane (1) corresponds to UCNP–APTMS, and lanes 2–6 to UCNP–APTMS–BSA/Dex–Strv, where the concentration of streptavidin (Strv) is 0.1, 0.5, 1.0, 2.5 and 5.0 mg mL−1, respectively. UCNP were revealed under 405 nm excitation wavelength. | |
TLR specific recognition of UCNPs
Targeted imaging and tracking of labeled neutrophils were studied in order to elucidate whether the recognition of TLR was specific or non-specific. These cells perform multiple forms of endocytosis (see ESI†), including phagocytosis of pathogens and pinocytosis via clathrin-dependent and independent mechanisms.39 All forms of endocytosis are active transport processes. They depend on the interactions between the endoskeletal proteins, motor proteins, plasma membrane and transport vesicles.40 Endocytosis requires the movements of the cell cytoplasm to surround a given material, performing the endocytic vesicle. The movement of the cytoplasm around the extracellular molecule is dependent on changes in the cytoskeletal proteins and requires the cell energy. Thus, in extreme conditions, the cells will not perform endocytosis, as the energy will be used as primary source of cell survival. We performed experiments at different temperatures in order to compare the normal behavior of neutrophils (37 °C) and under stressing conditions (4 °C) where the neutrophils do not take up anything from the environment. Scheme 1A depicts the final step of UCNP functionalization, i.e. with streptavidin. UCNP–streptavidin recognizes the biotinylated-LPS, previously attached to the cell membrane through specific recognition by TLR (Scheme 1B). Once the complex is formed, NP uptake takes place and membrane proteins such as TLRs that have been internalized into endosomes, are expected to be recycled. Confocal images of neutrophils incubated at 4 °C with UCNP–APTMS–BSA/Dex–Strv and biotin–LPS (under 488 nm excitation) showed uptake in cells only after 5–10 min (Fig. 5). Fig. 4 shows the confocal images of the cells incubated with the nanoparticles at 37 °C over time. No autofluorescence is detected in the control (neutrophils without nanoparticles) under 488 nm excitation wavelength. A fluorescence signal is observed from 2.5 min indicating an uptake of the nanoparticles. At longer incubation times (5 and 10 min), this signal becomes brighter. There is an uptake of every type of nanoparticles independently of their surface functionalization (see Fig. SI3†). The uptake is increasing with the time and reaches a maximum at 10 min in the case of the UCNP–APTMS–BSA/Dex–Strv + biotin–LPS. Additionally, we showed using UCNP–Strv–biotin–LPS nanoparticles (UCNP–APTMS–BSA/Dex–Strv5.0–biotin–LPS0.1 prepared with 0.1 mg mL−1 of biotin–LPS added) that the uptake was lower compared with the uptake of UCNP by neutrophils incubated at 37 °C first with biotin–LPS and then with UCNP–APTMS–BSA/Dex–Strv5.0 (Fig. SI4†), indicating that the two-step procedure that we applied to bind UCNP to neutrophils is a better option with respect to TLR4 activation and LPS internalization.
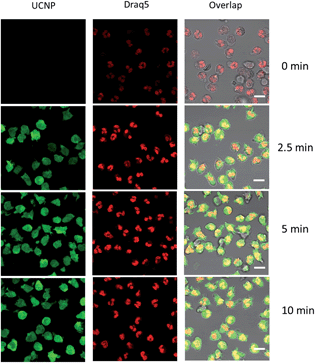 |
| Fig. 4 Confocal images of neutrophils incubated at 37 °C first with biotin–LPS and then with UCNP–APTMS–BSA/Dex–Strv5.0 under 488 nm excitation. From left to right, the images correspond to nanoparticles (green), Draq5 (red) and overlap (green and red), respectively. Incubation times (in minutes) are on the right hand side. Scale bars correspond to 10 μm. | |
To prove that the biotin–streptavidin interaction plays a vital role in the nanoparticle recognition by TLR4 receptors and their efficient internalization, we incubated the neutrophils directly with UCNP–APTMS–BSA/Dex–Strv5.0 without a previous incubation of the cells with biotin–LPS. The results are shown in the ESI (Fig. SI5†). Comparing these results with those reported in Fig. 4 in the main manuscript, a higher uptake can be observed when the cells were previously treated with biotin–LPS (Fig. 4), followed by incubation with the UCNP–APTMS–BSA/Dex–Strv5.0. It is therefore clear that the union biotin–streptavidin plays a vital role in the nanoparticle recognition by TLR4 receptors and their efficient internalization. Confocal images of neutrophils incubated at 4 °C with UCNP–APTMS–BSA/Dex–Strv and biotin–LPS (under 488 nm excitation) showed uptake in cells only after 5–10 min (Fig. 5). The fact that neutrophils uptake only the UCNP–APTMS–BSA/Dex–Strv nanoparticles in the presence of biotin–LPS for the specific recognition by TLR (see Fig. SI6† and Fig. 6), allows us to conclude that there is a specific recognition by TLR due to the formation of streptavidin–biotin complex. Higher rate of uptake at 37 °C and a low uptake at 4 °C shows that the internalization of the particles is an energy dependent process41 and it also depends on the charged surface of the NP.42 This is in good agreement with previously reported results, which demonstrated the effective internalization of UCNP coated with cationic polymers via energy dependent endocytosis.43,44 In the latter cases, the studies were performed with HeLa cell lines, differently from our investigation carried out with primary human neutrophils. Cell lines are immortal and might differ with respect to the primary cells in their nanoparticle uptake characteristics. In line with this, several researchers demonstrated that negatively charged nanoparticles were successfully internalized by neutrophils, while the cationic nanomaterials were trapped into extracellular structures,42 in contrast with the results reported with the HeLa cell lines. Additionally, primary human blood phagocytes (i.e. macrophages, monocytes and neutrophil granulocytes) show comparable phagocytic activity in vivo and in vitro.45 Therefore, neutrophils are ideal in vitro test platform for evaluation of the biological fate and consequences of nanoparticles in the human body. Moreover, endocytosis in neutrophils is a complex process involving fluid phase endocytosis and macropinocytosis39,46 and there will be uptake of NPs, even when no receptors are targeted, as seen in Fig. SI3.† We next investigated the viability of neutrophils coincubated with nonfunctionalized and functionalized UCNP at 37 °C in the absence and presence of biotin–LPS at 10, 30, 60 and 180 min by using Trypan Blue dye exclusion assay. We observed no significant decrease in neutrophil viability up to three hours of coincubation between control neutrophils and those incubated with UCNPs (Fig. SI7†). The results from Trypan Blue dye exclusion viability assay indicate that neutrophil plasma membrane integrity is not compromised by prolonged incubation with UCNPs.
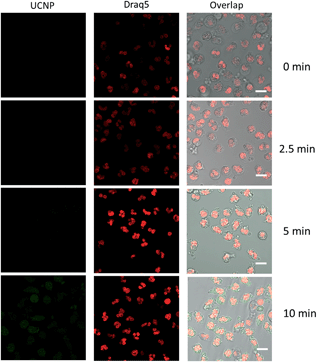 |
| Fig. 5 Confocal images of neutrophils incubated at 4 °C first with biotin–LPS and then with UCNP–APTMS–BSA/Dex–Strv5.0 under 488 nm excitation. From left to right, the images correspond to nanoparticles (green), Draq5 (red) and overlap (green and red), respectively. Incubation times (in minutes) are on the right hand side. Scale bars correspond to 10 μm. | |
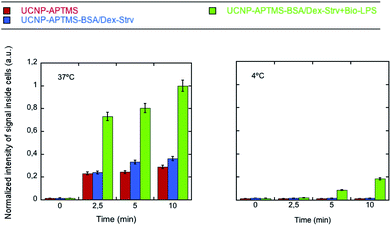 |
| Fig. 6 Normalized fluorescence intensity of nanoparticles signal inside the cells from the grey scale at different times, at 37 °C and 4 °C, respectively. Legend: UCNP–APTMS (red), UCNP–APTMS–BSA/Dex–Strv5.0 (blue), UCNP–APTMS–BSA/Dex–Strv 5.0 + Bio–LPS (green). | |
Conclusions
We reported the fabrication of up-conversion nanoparticles (UCNP) KYF4:Yb,Er and their functionalization with BSA/Dex–Strv for the specific recognition by toll-like receptors (TLRs) in the presence of biotin–LPS. Using highly fluorescent UCNP, we have monitored the uptake of functionalized UCNP in human neutrophils under normal (37 °C) and stressing conditions (4 °C). Uptake of the UCNP–APTMS–BSA/Dex–Strv nanoparticles in the presence of biotin–LPS was proven to be faster and higher at 37 °C compared to the uptake at 4 °C. These results indicate that an energy-dependent uptake process is the principal mechanism responsible for the UCNP internalization into human PMNs. Besides, negatively charged UCNP are required for cell uptake studies with PMNs in order to avoid extracellular nanoparticle trapping. Excitation of the UCNP at the second-harmonic wavelength at 488 nm allows introducing new non-conventional detection imaging nanotools on commercially available imaging equipment.
The integrative in vitro approach proposed herein using the innovative UCNP imaging combined with the primary human blood neutrophils testing platform could be used in vivo to identify/evaluate the biological fate and consequences of nanoparticles in the human body. In addition, the applicability of these functionalized UCNP could be extended to other types of cells and to the recognition of other receptors involved in immune responses and to specific recognition of anti-cancer drugs since the mechanism of cellular uptake is important in the field of nanomedicine, cancer diagnosis, drug delivery and treatment.
Acknowledgements
We thank the Department of Transfusion Medicine, Universitätsmedizin Greifswald for providing the neutrophils. This work was financially supported by the German Ministry of Education and Research (BMBF) within the project FKZ 03Z2CN11 and the Spanish MICINN project CTQ2010-18859, ICIQ, ICREA and ERCstg Polydot.
Notes and references
- S. Yamashiro, H. Kamohara, J. M. Wang, D. Yang, W. H. Gong and T. Yoshimura, J. Leukocyte Biol., 2001, 69, 698–704 CAS
. - L. C. Parker, M. K. B. Whyte, S. K. Dower and I. Sabroe, J. Leukocyte Biol., 2005, 77, 886–892 CrossRef CAS PubMed
. - Z. Shi, Z. Cai, S. Wen, C. Chen, C. Gendron, A. Sanchez, K. Patterson, S. Fu, J. Yang, D. Wildman, R. H. Finnell and D. Zhang, J. Biol. Chem., 2009, 284, 20540–20547 CrossRef CAS PubMed
. - K. Takeda, T. Kaisho and S. Akira, Annu. Rev. Immunol., 2003, 21, 335–376 CrossRef CAS PubMed
. - A. M. Hajjar, R. K. Ernst, J. H. Tsai, C. B. Wilson and S. I. Miller, Nat. Immunol., 2002, 3, 354–359 CrossRef CAS PubMed
. - K. Takeda and S. Akira, J. Dermatol. Sci., 2004, 34, 73–82 CrossRef CAS PubMed
. - D. M. Underhill and A. Ozinsky, Curr. Opin. Immunol., 2002, 14, 103–110 CrossRef CAS PubMed
. - G. Horvath, S. Young and E. Latz, Methods Mol. Biol., 2009, 517, 33–54 CAS
. - E. Latz, A. Visintin, E. Lien, K. A. Fitzgerald, B. G. Monks, E. A. Kurt-Jones, D. T. Golenbock and T. Espevik, J. Biol. Chem., 2002, 277, 47834–47843 CrossRef CAS PubMed
. - M. A. Dobrovolskaia and S. E. McNeil, Nat. Nanotechnol., 2007, 2, 469–478 CrossRef CAS PubMed
. - R. Singh and J. W. Lillard Jr, Exp. Mol. Pathol., 2009, 86, 215–223 CrossRef CAS PubMed
. - F. Auzel, Chem. Rev., 2003, 104, 139–174 CrossRef PubMed
. - J. V. Frangioni, Curr. Opin. Chem. Biol., 2003, 7, 626–634 CrossRef CAS PubMed
. - S. A. Hilderbrand and R. Weissleder, Curr. Opin. Chem. Biol., 2010, 14, 71–79 CrossRef CAS PubMed
. - L. Zhao, A. Kutikov, J. Shen, C. Duan, J. Song and G. Han, Theranostics, 2013, 3, 249–257 CrossRef CAS PubMed
. - X. Yang, Q. Xiao, C. Niu, N. Jin, J. Ouyang, X. Xiao and D. He, J. Mater. Chem. B, 2013, 1, 2757–2763 RSC
. - H. J. M. A. A. Zijlmans, J. Bonnet, J. Burton, K. Kardos, T. Vail, R. S. Niedbala and H. J. Tanke, Anal. Biochem., 1999, 267, 30–36 CrossRef CAS PubMed
. - P. L. Corstjens, S. Li, M. Zuiderwijk, K. Kardos, W. R. Abrams, R. S. Niedbala and H. J. Tanke, IEE Proc.: Nanobiotechnol., 2005, 152, 64–72 CrossRef CAS PubMed
. - R. S. Niedbala, H. Feindt, K. Kardos, T. Vail, J. Burton, B. Bielska, S. Li, D. Milunic, P. Bourdelle and R. Vallejo, Anal. Biochem., 2001, 293, 22–30 CrossRef CAS PubMed
. - F. van de Rijke, H. Zijlmans, S. Li, T. Vail, A. K. Raap, R. S. Niedbala and H. J. Tanke, Nat. Biotechnol., 2001, 19, 273–276 CrossRef CAS PubMed
. - F. Song, G. Zhang, M. Shang, H. Tan, J. Yang and F. Meng, Appl. Phys. Lett., 2001, 79, 1748–1750 CrossRef CAS
. - A. M. Tkachuk, S. E. Ivanova, M. F. Joubert, Y. Guyot, L. I. Isaenko and V. P. Gapontsev, J. Lumin., 2007, 125, 271–278 CrossRef CAS
. - J. Hoang, R. N. Schwartz, K. L. Wang and J. P. Chang, J. Appl. Phys., 2012, 112, 063117 CrossRef
. - H.-T. Wong, H. L. W. Chan and J. Hao, Opt. Express, 2010, 18, 6123–6130 CrossRef CAS PubMed
. - F. Leblond, S. C. Davis, P. A. Valdes and B. W. Pogue, J. Photochem. Photobiol., B, 2010, 98, 77–94 CrossRef CAS PubMed
. - M. C. Mancini, B. A. Kairdolf, A. M. Smith and S. Nie, J. Am. Chem. Soc., 2008, 130, 10836–10837 CrossRef CAS PubMed
. - U. Resch-Genger, M. Grabolle, S. Cavaliere-Jaricot, R. Nitschke and T. Nann, Nat. Methods, 2008, 5, 763–775 CrossRef CAS PubMed
. - H. H. Gorris, R. Ali, S. M. Saleh and O. S. Wolfbeis, Adv. Mater., 2011, 23, 1652–1655 CrossRef CAS PubMed
. - C. A. Schneider, W. S. Rasband and K. W. Eliceiri, Nat. Methods, 2012, 9, 671–675 CrossRef CAS PubMed
. - W. Strober, Curr. Protoc. Immunol., 2001, 21, A.3B.1–A.3B.2 Search PubMed
. - H. Schäfer, P. Ptacek, O. Zerzouf and M. Haase, Adv. Funct. Mater., 2008, 18, 2913–2918 CrossRef
. - F. Wang and X. Liu, Chem. Soc. Rev., 2009, 38, 976–989 RSC
. - E. D. L. Rosa-Cruz, L. A. Diaz-Torres, R. A. Rodriguez-Rojas, M. A. Meneses-Nava, O. Barbosa-Garcia and P. Salas, Appl. Phys. Lett., 2003, 83, 4903–4905 CrossRef
. - Q. Y. Zhang and X. Y. Huang, Prog. Mater. Sci., 2010, 55, 353–427 CrossRef CAS
. - R. M. Pasternack, S. Rivillon Amy and Y. J. Chabal, Langmuir, 2008, 24, 12963–12971 CrossRef CAS PubMed
. - P. H. Pandya, R. V. Jasra, B. L. Newalkar and P. N. Bhatt, Microporous Mesoporous Mater., 2005, 77, 67–77 CrossRef CAS
. - M. S. Celej, G. G. Montich and G. D. Fidelio, Protein Sci., 2003, 12, 1496–1506 CrossRef PubMed
. - G. Gaur, D. S. Koktysh and S. M. Weiss, Adv. Funct. Mater., 2013, 23, 3604–3614 CrossRef CAS
. - S. M. Uriarte, N. R. Jog, G. C. Luerman, S. Bhimani, R. A. Ward and K. R. McLeish, Am. J. Physiol., 2009, 296, C857–C867 CrossRef CAS PubMed
. - F. Paradisi, C. D'Onofrio, G. Pepe, A. Cifarelli and D. Piccolo, Int. J. Clin. Lab. Res., 1979, 9, 47–60 CAS
. - P. A. Detmers, N. Thieblemont, T. Vasselon, R. Pironkova, D. S. Miller and S. D. Wright, J. Immunol., 1996, 157, 5589–5596 CAS
. - M. Bartneck, H. A. Keul, G. Zwadlo-Klarwasser and J. Groll, Nano Lett., 2010, 10, 59–63 CrossRef CAS PubMed
. - J. Jin, Y. J. Gu, C. W. Man, J. Cheng, Z. Xu, Y. Zhang, H. Wang, V. H. Lee, S. H. Cheng and W. T. Wong, ACS Nano, 2011, 5, 7838–7847 CrossRef CAS PubMed
. - P. A. Ma, H. Xiao, X. Li, C. Li, Y. Dai, Z. Cheng, X. Jing and J. Lin, Adv. Mater., 2013, 25, 4898–4905 CrossRef CAS PubMed
. - E. Schwarzer, H. Kuhn, E. Valente and P. Arese, Blood, 2003, 101, 722–728 CrossRef CAS PubMed
. - F. S. Lamb, J. S. Hook, B. M. Hilkin, J. N. Huber, A. P. Volk and J. G. Moreland, J. Biol. Chem., 2012, 287, 12395–12404 CrossRef CAS PubMed
.
Footnotes |
† Electronic supplementary information (ESI) available: Energy transfer processes of Er3+ and Yb3+ at different excitation wavelengths; zeta-potential data from the UCNP after each functionalization step; elemental mapping with EDX of KYF4:Yb,Er; electrophoresis of UCNP before and after functionalization; mechanisms of nanoparticles cellular uptake; confocal images of neutrophils incubated at 37 °C first with biotin–LPS and then with UCNP–APTMS–BSA/Dex–Strv5.0 under 488 nm excitation; confocal images of neutrophils incubated at 4 °C first with biotin–LPS and then with UCNP–APTMS–BSA/Dex–Strv5.0 under 488 nm excitation; lambda scan of UCNP excited using 488 nm laser by confocal laser scanning microscopy (movie in the ESI). See DOI: 10.1039/c3ra48018f |
‡ These authors contributed equally to this work. |
|
This journal is © The Royal Society of Chemistry 2014 |
Click here to see how this site uses Cookies. View our privacy policy here.