DOI:
10.1039/C1SM06616A
(Paper)
Soft Matter, 2012,
8, 198-203
Protein detection using aqueous/LC interfaces decorated with a novel polyacrylic acid block liquid crystalline polymer†
Received
24th August 2011
, Accepted 12th October 2011
First published on 10th November 2011
Abstract
The interface between 5CB and water in a TEM grid by decoration of an amphiphilic block copolymer of PAA-b-LCP was examined for protein detection. Several proteins were detected at a few μM levels through homeotropic to planar orientational change (H–P change) of the 5CB. The H–P change became slow as the pH of water increased above the isoelectric point (pI) of the protein, and areal density of the monolayer and salt concentration increased. The TEM grid cell could detect proteins of human urine from a patient having albuminuria with a detection limit of as low as 0.032 mg mL−1.
Introduction
Proteins regulate and perform most of the biochemical processes in cells so that their detection is essential and can be applied to a variety of fields ranging from clinical diagnosis,1 environmental control2 to homeland security issues.3 Recently biosensor research has attracted considerable interest over the classical analytical methods for monitoring proteins and their interactions with other macromolecules4 due to their inherent specificity, simplicity, comparatively low cost, rapid response, and proneness to miniaturization, thereby allowing continuous monitoring. Two major strategies for protein detection, label-based and label-free, were employed in the biosensors in order to generate signals. The label-based technique requires labeling of query molecules with labels such as fluorescent dyes, radioisotopes, epitope tags, etc.5 However, label-free techniques measure an inherent property of the query itself such as mass and dielectric property so that there is no need to modify interactors.6 In the past decade, LCs have become one of the most important tools in the field of biological sensors.7–10 Some unique properties possessed by LCs include (i) high sensitivity of the orientations of the LCs to minute changes on the surface; (ii) liquid-like mobility that can amplify LC responses within tens of milliseconds; (iii) birefringence, and the orientational changes of LCs which can be observed under crossed polarizers. These enable LCs to be used for a label-free detection system with high sensitivity and without any sophisticated and expensive instrumentation.
Protein detection viaLCs on solid surfaces has been the subject of many studies.11–13 For example, Luk et al. designed a LC-based protein assay that can differentiate between random and well-defined protein orientation. Similarly, Gupta et al. studied a LC-based bioassay where nematic LCs switched from uniform to non-uniform azimuthal orientation on functionalized gold films after incubating with a protein solution.14 In another study, Deny et al. demonstrated a simple strategy to immobilize proteins on the LC surfaces with well-defined orientation and the development of a LC-based sensor for real-time and label-free detection of specific protein–protein binding events. More recently many research groups have investigated LC-based sensors that allowed real-time reporting of various biological events15,16 such as enzymatic hydrolysis of phospholipids, specific phospholipid–protein binding, and DNA hybridization.9,17–19
In this study, we utilized a functionalized interface between a nematic liquid crystal, 4-cyano-4′-pentylbiphenyl (5CB), and water as a label-free biosensor. The interface was decorated by an amphiphilic block copolymer which consists of a side-group liquid crystalline polymer block (poly(4-cyanobiphenyl-4-oxyundecylacrylate), denoted LCP) and poly(acrylic acid) (PAA) block; the PAA and LCP blocks act as recognition and transducer elements in the biosensor, respectively, and the signals are amplified by the homeotropic to planar orientational change (H–P change) of the 5CB on the octadecyltrichlorosilane (OTS)-coated glass in the transmission electron microscope (TEM) grid cell. In our previous study,20 we found that PAA-b-LCP was shown to confer pH-dependent anchoring of the director orientation of the 5CB at the water/LC interface by the conformational change of the PAA block in water translating it into rapid, reversible changes in the director field by the LCP block. The change in anchoring occurs as quickly as the buffer can be changed and is reversible, with a response that is repeatable over as many cycles as were tested. The polymer-mediated anchoring persists for a long time, indicating that the LCP block secures the self-assembled layer on the 5CB, even under conditions that cause repulsive interactions among the PAA blocks. Specifically, the water/5CB interface functionalized with PAA-b-LCP was immobilized using a TEM grid mounted in a cell (see ESI, S1 and S2†) that permitted rapid exchange of the aqueous medium, providing a versatile system for examining a variety of environmental stimuli such as change of pH, variation of ionic strength and the presence of small amounts of proteins.
In this article, the performance of a TEM grid cell was examined for nonspecific protein detection with lysozyme, bovine serum albumin (BSA), α-lactalbumin, insulin and human urine from a patient having albuminuria. In order to optimize the TEM grid cell, we employed a Langmuir–Blodgett film fabrication method to control the areal density of the monolayer on the 5CB. We screened the effects of ionic strength and pH in water and areal density of the monolayer on the protein adsorption. The use of PAA-b-LCP is necessary because the conformational change of the PAA block of PAA-b-LCP can happen by complexation of proteins with PAA chains. It is found that PAA-b-LCP conferred pH-dependent anchoring of the director orientation of the 5CB at the LC/water interface by the conformational change of the PAA block in water changing it into rapid and reversible changes in the director field by the LCP block. This research may provide the fundamental information for designing a specific protein detection system through this convenient, label-free, and inexpensive detection method by employing selective protein detection units such as antibodies and aptamers in the PAA chains.
Experimental section
Materials
5CB (TCI, 100%), methanol (Aldrich), buffer solutions (pH = 2–12, Samchun Chemicals Korea), and dichloromethane (DCM, Aldrich) were used without further purification. Copper specimen grids (G75, with grid hole width 285 μm, pitch 340 μm, bar width 55 μm, size 3.05 mm and thickness 18 μm) were bought from Ted Pella, Inc. Lysozyme was purchased from MP Biomedicals and BSA, α-lactalbumin, and insulin were supplied from Aldrich.
PAA-b-LCP was synthesized with the same method as in our previous paper.20 The molecular weight was PAA(16k)-b-LCP(6k) with an Mw/Mn of 1.27. The chemical structure of PAA(16k)-b-LCP(6k) is shown in Scheme 1; the number average molecular weight of PAA-b-LCP was calculated from the GPC data of PtBA(27k)-b-LCP(6k) by assuming the complete removal of the protected tertiary butyl groups.
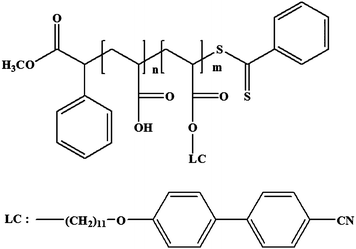 |
| Scheme 1 Chemical structure of PAA-b-LCP. | |
Preparation of the TEM grid cell and the flow chamber
A series of experiments were conducted in a home-made polydimethylsiloxane (PDMS) flow chamber designed to permit rapid and controlled exchange of the aqueous solutions (and hence, rapid and controlled changes in pH, salt concentration, amounts of proteins) at the PAA-b-LCP functionalized water/5CB interface, and to facilitate repeated displacement and introduction of fresh buffer solutions to these interfaces without requiring repeated exposure of the interface to air. The TEM grid cells and the flow chambers were prepared according to the procedures in the literature with small modifications.39,40 Fig. S1† shows the schematic of the flow chamber with a TEM grid cell in the flow chamber. Procedures of preparation of the flow chamber with a TEM grid cell are as follows. The glass microscope slides were cleaned according to published procedures and coated with OTS.41Copper specimen grids (that were cleaned sequentially in methylene chloride, ethanol, and finally, in methanol) were placed onto the surface of the small OTS-coated glass slide that was then glued onto another common slide glass with epoxy. 1 μL of 5CB was dispensed onto each grid by dropping, and the excess LC was removed by contacting a capillary tube to it. The thickness of the 5CB layer on the TEM grid was approximately 20 μm. The PAA-b-LCP solution was prepared by the following method: PAA-b-LCP was first dissolved for 2 days at 60 °C in dioxane, and then toluene was added to obtain a 0.1 wt% solution in a dioxane/toluene (6/4, v/v) mixture. A dioxane/toluene (6/4, v/v) mixture was used for a PAA-b-LCP solvent because PAA-b-LCP did not dissolve in the typical organic spreading solvents (such as pure chloroform or toluene) due to its polarity. The density of the dioxane/toluene (6/4, v/v) mixture is lower than that of water, which facilitates deposition, and the solvent mixture is sufficiently hydrophobic to prevent loss during spreading. The PAA-b-LCP solution spread on the water in the bath of the Langmuir–Blodgett (LB) instrument (KSV Layer Builder, AAA 100178, KSV Instruments Ltd., Finland) and the solvent was evaporated completely for 1 h at room temperature to create a monolayer film on the water. The areal density of the monolayer was controlled by the surface pressure (SP) of the LB film. Langmuir–Schaefer transfer of the film was achieved in the following manner. The TEM grid on the OTS-coated glass (glued on the glass slide) was slowly inserted (grid side down) into the bath, coming to rest on silicon spacers (2 mm thick) attached to a glass slide, previously placed in the bath. The two glass slides spaced with silicon rubber were clipped with binding clips. Inlet and outlet ports for exchanging buffer solutions were made by needles that were punched through silicon rubber. The entire TEM grid cell and flow chamber were then flipped over and the solution was filled through an inlet syringe. The entire volume which can be occupied by water in the flow cell was 1 mL.
Analysis
The image of the TEM grid cell was observed through a polarized optical microscope (Samwon, LSP-13) equipped with crossed polarizers. The digital image and video were recorded during and after changing the solution in the flow cell and the average gray-scale intensity (GI) of the digital image was calculated using Adobe Photoshop software. A urine sample from a patient having albuminuria (supplied from Kyungpook National University Hospital) contained 1.396 mg mL−1proteins. The normal urine was also tested; normal urine should not exceed 150 mg per day of proteins and the amount of the urine for a normal person is 800 to 2000 mL per day so that the concentration of the normal urine is 0.19 to 0.08 mg mL−1.
Results and discussion
Non-specific protein detection
Protein adsorption was found to be detected through the H–P change in the TEM grid cell from our previous report with lysozyme.20Protein adsorption can occur through several mechanisms, such as electrostatic interactions, hydrogen bonding, hydrophobic interactions, etc., so that protein adsorption with other proteins having different isoelectric points (pIs) is important to figure out the role of each mechanism involved. Lysozyme, BSA, α-lactalbumin, and insulin were tested with our TEM grid cell. The pIs of lysozyme, BSA,21,22α-lactalbumin,23,24 and insulin25,26 are 11.4, 5.3, 4.2, and 5.3, respectively. The respective sizes for these proteins are 14.9, 66.4, 14.2, and 6 kilodalton (kDa). Fig. 1a shows the micrographs of the TEM grid cells after injecting lysozyme, BSA, α-lactalbumin, and insulin solutions of different concentrations at pH = 12. Denaturing of proteins can occur under a variety of physical conditions, including high temperature, very low or high pH, and very high pressure,27 but our purpose of this study is detection of the protein although it might be denatured. The H–P change happened for all studied proteins, although both proteins and deprotonated PAA bore negative net charges at pH = 12. Fig. 1b shows a model of the complexation between proteins and the PAA chains leading to the H–P change of the 5CB through conformational change of the LCP block. The conformational change of the PAA chains can happen by complexation of proteins with the PAA chains because the PAA brushes have been known to represent an exceptional surface modification useful for unspecific protein immobilization and bind proteins regardless of the sign of the protein's net charge.28,29Lysozyme was selected for a model protein for screening the effects of pH, areal density of the monolayer and salt concentration on the protein detection performance of the TEM grid cell because its isoelectric point (pI) (11.4) is in the range showing homeotropic orientation of the 5CB in the TEM grid which is a requirement for detection of the H–P change by protein adsorption through a polarized optical microscope with a cross-polar state.
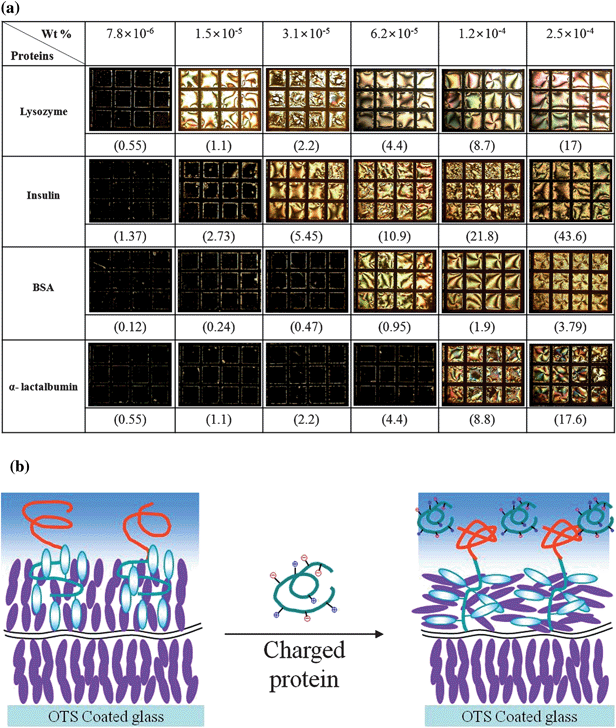 |
| Fig. 1 (a) The optical micrographs of the TEM grid cells under a polarized optical microscope with a cross-polar state for lysozyme, insulin, BSA, and α-lactalbumin of different concentrations; the numbers in parentheses indicate μM; (b) a model of the complexation between proteins and the PAA chains leading to the conformational change of the LCP block. | |
pH effect
Fig. 2 shows the change of the average gray-scale intensity (GI) after injecting a 1 mg mL−1 (70 μM) lysozyme solution in the TEM grid cell at pH = 10, 11, and 12 with the addition of 1 M NaCl. We only tested these pH regions due to the limitation for obtaining the homeotropic orientation of 5CB on the OTS-coated glass in the TEM grid at pH ≤ 9 as discussed in our previous paper.20 By injecting the lysozyme solution into the TEM grid cell, the GI increased step-wise regardless of the tested pHs (10, 11, and 12) indicating that the H–P change happened due to complexation between the deprotonated PAA chains and lysozyme which could shrink the PAA chains so that the H–P change occurred in a manner similar to that reported in our previous report for the H–P change occurring at low pHs without proteins. However, the speed of the H–P change was dependent on the pH. Lysozyme is known to have a pI of 11.4 at which the predominant ionic form of the lysozyme has a net charge of zero. The lysozyme bears a positive net charge below the pI (pH = 10 and 11) so that the electrostatic interactions between the protonated PAA chains and lysozyme accelerate the rate of the adsorption. However, the speed of the pH change became slower above the pI (pH = 12) at which lysozyme bears a negative net charge so that the electrostatic repulsions between the protonated PAA chains and lysozyme caused slow complexation. A number of forces involved in the complexation include electrostatic interaction, hydrogen bonding, hydrophobic interaction, conformation entropy gain, etc.30–34 Although electrostatic interactions were depressed at pH = 12, other interactions were still possible so that the complexation could happen at pH = 12 with reduced adsorption rates.
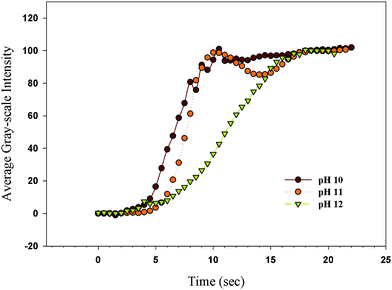 |
| Fig. 2 The change of the GI after injecting a 1 mg mL−1lysozyme solution into the TEM grid cell at pH = 10, 11, and 12 with addition of 1 M NaCl; the monolayer was made at SP = 30 mN m−1; the original movies can be found in the ESI, S4(a–c)†. | |
Effect of ionic strength
Fig. 3 shows the change of the GI at pH = 12 after injecting a 1 mg mL−1lysozyme solution into the TEM grid cell with different NaCl concentrations. The PAA-b-LCP monolayer on the 5CB in the TEM grid cell was made at SP = 30 mN m−1. The solution in the TEM grid cell was initially without protein. The salt concentration of the TEM grid cell before injecting the lysozyme solution was kept the same as the controlled salt concentration of the lysozyme solution. The GI increased regardless of the tested salt concentrations (0.1, 0.5, and 1 M). However, the speed of the H–P change (caused by the lysozyme adsorption) was dependent on the salt concentration in the TEM grid cell; the speed of the H–P change decreased as the salt concentration increased. The dependence of the speed of the adsorption on the salt concentration might be related to the protein resistance that can be controlled by the ionic strength of the protein solution.35 The electrostatic repulsive forces between the deprotonated PAA chains and lysozyme at pH = 12 would be reduced as ionic strength increases due to an increased direct screening of electrostatic interactions between the proteins and the PAA chains by an increase of the counterions at high salt concentrations. However, we found the opposite results. Wittemann et al. also found that the steric repulsion between protein and the spherical polyelectrolyte brushes becomes operative and no adsorption takes place at high salt concentrations.36 The particles bound huge amounts of different proteins at low ionic strength and were essentially protein resistant at an elevated ionic strength of 100 mM in their results. It has been postulated that the binding of the protein molecules to the PAA brush particles was driven by a release of counterions by adsorption of proteins, which increases the entropy of the system. At high salt concentration, the ionic strength in the brush is almost the same as that in water so that a release of counterions by protein adsorption could not be expected. In this study, we found that the speed of the adsorption was reduced as the salt concentration increased but the adsorption was detected at a relatively high ionic concentration (1 M) which is in the region of protein resistance in Wittemann's study. This result also indicates that our system might be more sensitive to detection of the adsorption of the protein even at the high ionic concentrations.
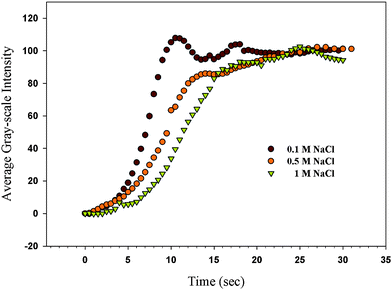 |
| Fig. 3 The change of the GI at pH = 12 after injecting a 1 mg mL−1lysozyme solution into the TEM grid cell with different NaCl concentrations; the PAA-b-LCP monolayer on the 5CB in the TEM grid cell was decorated at SP = 30 mN m−1; the original movies can be found in the ESI, S5(a–c)†. | |
Effect of surface pressure
In order to study the effect of areal density of the monolayer on the speed of the H–P change by PAA and lysozyme complex formation, we recorded movies after injecting the 1 mg mL−1lysozyme solution into the TEM grid cell at pH = 12 with the PAA-b-LCP monolayers made at different SPs with addition of 1 M NaCl. Fig. 4 shows the GI change after injecting lysozyme solution at different SPs. The areal density of the monolayer of the block copolymer on the 5CB increases as the SP value increases. The H–P change started at 210 seconds at SP = 40 mN m−1 and it did not happen at SP ≥ 45 mN m−1 under our experimental conditions. The variations in the structure between different types of proteins are too great to allow interpretations, but the use of protein mutants affords new opportunities to elucidate even finer details of the adsorption process. Minimal changes in amino acid composition can change adsorption behavior significantly. Large lysozyme might be hard to penetrate into the dense PAA brush so that the H–P change through complex formation between PAA and protein might be difficult for the dense PAA brush. The dense brush might have a more condensed interface between water and brush so that the adsorption might increase as the areal density increased if the immobilization of protein at the PAA brush happened at the interface rather than in the brush. However, no adsorption occurred at higher SPs (≥45 mN m−1) indicating that the immobilized proteins were entrapped within the PAA brush. This result strongly suggests that the adsorption of protein was strongly dependent on the areal density of the PAA brush. Wittemann and Ballauff also observed the protein adsorption in the PAA brush with spherical PAA brush particles.37
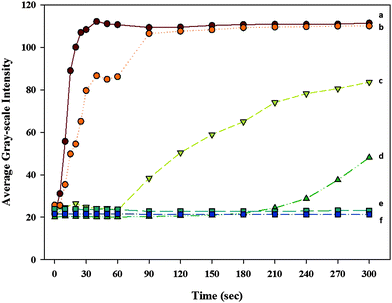 |
| Fig. 4 The GI change after injecting the 1 mg mL−1lysozyme solution into the TEM grid cell which is made with the PAA-b-LCP decoration on the 5CB at SP = (a) 30, (b) 35, (c) 38, (d) 40, (e) 45 and (f) 50 mN m−1 in the TEM grid cell at pH = 12 with addition of 1 M NaCl; the TEM micrographs for this figure can be found in the ESI, S3†. | |
Application to detection of albuminuria
One of the applications for this system is a sensor for detection of protein in the urine of patients having albuminuria. The two proteins of primary interest in urine are albumin and globulin. Albumin has a molecular weight of approximately 66 while globulin has a molecular weight of approximately 150 kDa. Due to the “small” size of the albumin, it is more readily filtered by the kidney into the filtrate. Therefore, albumin is the most common type of protein found in urine. Excessive albumin in urine is a condition called albuminuria.38 A human urine sample from a patient having albuminuria was supplied from Kyungpook National University Hospital and contained 1.396 mg mL−1proteins. A normal urine sample was obtained from a person without albuminuria. Fig. 5 shows the optical micrographs of the TEM grid cells observed under a polarized optical microscope with a cross-polar state after injecting human urine into the TEM grid cell. The PAA-b-LCP monolayer was made at the pH = 12 and SP = 30 mN m−1 with the addition of 1 M NaCl. The initial homeotropic orientation (Fig. 5a) was maintained after injecting the normal urine (Fig. 5f) although the H–P change occurred when a urine sample from a patient having albuminuria was injected (Fig. 5b–e). The urine sample from a patient having albuminuria was diluted with distilled water until the homeotropic orientation was maintained. The H–P change was observed until the concentration of the protein was 0.032 mg mL−1. The protein concentration was measured by KNU hospital with the turbidimetric method using a Roche/Hitachi Modular P analyzer (Roche Diagnostics, Indianapolis, IN). The H–P change did not happen when the concentration was 0.016 mg mL−1.
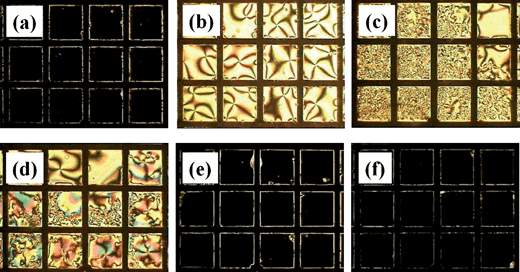 |
| Fig. 5 The optical micrographs of the TEM grid cells with the PAA-b-LCP monolayer on the 5CB observed under a polarized optical microscope with a cross-polar state after injecting human urine into the TEM grid cell; the TEM grid cell was made at pH = 12 and SP = 30 mN m−1 with the addition of 1 M NaCl; (a) initial state without injecting human urine; (b–e) urine samples from a patient having albuminuria at different concentrations; (f) normal urine sample; the urine samples were diluted with distilled water (b) ×10 (0.1396 mg mL−1), (c) ×20 (0.0698 mg mL−1), (d) ×40 (0.0349 mg mL−1), (e) ×80 (0.0174 mg mL−1), and (f) ×10 for change of pH to 12; a urine sample from a patient having albuminuria (supplied from Kyungpook National University Hospital) contained 1.396 mg mL−1proteins and a normal urine sample was obtained from a person without albuminuria. | |
Conclusions
In summary, this work has determined fast, reversible, and durable switching between homeotropic and planar anchoring at the water/5CB interface in response to environmental stimuli mediated by an appropriately designed PAA-b-LCP which was examined for protein adsorption. This interface was found to be sensitive to protein adsorption and detects the small amount of protein (a few μM levels) regardless of the state of net charge through the H–P change. The effects of pH, areal density of the monolayer and salt concentration on the protein detection performance of the TEM grid cell were examined with a model protein of lysozyme. The H–P change became slower as the pH of the water increased above the pI of lysozyme (11.4), the areal density of the block copolymer monolayer on the 5CB increased, and the salt concentration increased. The TEM grid cell performance was examined with human urine from a patient having albuminuria. The lower detection limit for proteins in human urine was as low as 0.032 mg mL−1 under our experimental conditions. We demonstrated the sensitivity of our TEM grid cell by measuring adsorption of proteins in this article and are exploring its use as a biosensor by employing selective protein detection units such as antibodies and aptamers in the PAA chains.
Acknowledgements
This work was supported by the National Research Foundation of Korea Grant (2010-0014822) funded by the South Korean Government. We also appreciate Dr Sun-Hee Park in Kyungpook National University Hospital, Daegu, South Korea for supplying the urine samples.
References
- S. Ciordia, V. De Los Rios and J. P. Albar, Clin. Transl. Oncol., 2006, 8, 566–580 CrossRef CAS.
- B. W. Clark, T. A. Phillips and J. R. Coats, J. Agric. Food Chem., 2005, 53, 4643–4653 CrossRef CAS.
- P. E. Andreotti, G. V. Ludwig, A. H. Peruski, J. J. Tuite, S. S. Morse and L. F. Peruski, BioTechniques, 2003, 35, 850–859 CAS.
- L. Murphy, Curr. Opin. Chem. Biol., 2006, 10, 177–184 CrossRef CAS.
- V. Espina, E. C. Woodhouse, J. Wulfkuhle, H. D. Asmussen, E. F. Petricoin and L. A. Liotta, J. Immunol. Methods, 2004, 290, 121–133 CrossRef CAS.
- P. Y. Li, B. Lin, J. Gerstenmaier and B. T. Cinningham, Sens. Actuators, B, 2004, 99, 6–13 CrossRef.
- Y. Y. Luk, M. L. Tingey, K. A. Dickson, R. T. Raines and N. L. Abbott, J. Am. Chem. Soc., 2004, 126, 9024–9032 CrossRef CAS.
- D. Hartono, X. Y. Bi, K. L. Yang and L. Y. L. Yung, Adv. Funct. Mater., 2008, 18, 2938–2945 CrossRef CAS.
- A. D. Price and D. K. Schwartz, J. Am. Chem. Soc., 2008, 130, 8188–8194 CrossRef CAS.
- S. J. Woltman, G. D. Jay and G. P. Crawford, Nat. Mater., 2007, 6, 929–938 CrossRef CAS.
- T. Govindaraju, P. J. Bertics, R. T. Raines and N. L. Abbott, J. Am. Chem. Soc., 2007, 129, 11223–11231 CrossRef CAS.
- Y. Y. Luk, M. L. Tingey, D. J. Hall, B. A. Israel, C. J. Murphy, P. J. Bertics and N. L. Abbott, Langmuir, 2003, 19, 1671–1680 CrossRef CAS.
- C. Y. Xue, S. A. Khan and K. L. Yang, Adv. Mater., 2008, 20, 198–202 Search PubMed.
- V. K. Gupta, J. J. Skaife, T. B. Dubrovsky and N. L. Abbott, Science, 1998, 279, 2077–2080 CrossRef CAS.
- D. Hartono, C. Y. Xue, K. L. Yang and L. Y. L. Yung, Adv. Funct. Mater., 2009, 19, 3574–3579 CrossRef CAS.
- A. R. Wise, J. A. Nye and J. T. Groves, ChemPhysChem, 2008, 9, 1688–1692 CrossRef CAS.
- J. M. Brake and N. L. Abbott, Langmuir, 2007, 23, 8497–8507 CrossRef CAS.
- J. M. Brake, M. K. Daschner, Y. Y. Luk and N. L. Abbott, Science, 2003, 302, 2094–2098 CrossRef CAS.
- D. Hartono, W. J. Qin, K. L. Yang and L. Y. L. Yung, Biomaterials, 2009, 30, 843–849 CrossRef CAS.
- D. Y. Lee, J. M. Seo, W. Khan, J. A. Kornfield, Z. Kurji and S. Y. Park, Soft Matter, 2010, 6, 1964–1970 RSC.
- D. C. Carter and J. X. Ho, Adv. Protein Chem., 1994, 45, 153–203 CrossRef CAS.
- C. La Rosa, D. Milardi, S. Fasone and D. Grasso, Thermochim. Acta, 1994, 235, 231–237 CrossRef CAS.
- C. Bramaud, P. Aimar and G. Daufin, Biotechnol. Bioeng., 1995, 47, 121–130 CrossRef CAS.
- F. Galisteo and W. Norde, Colloids Surf., B, 1995, 4, 375–387 CrossRef CAS.
- O. Wintersteiner and H. Abramson, J. Biol. Chem., 1933, 99, 741–753 CAS.
- D. N. Brems, P. L. Brown, L. A. Heckenlaible and B. H. Frank, Biochemistry, 1990, 29, 9289–9293 CrossRef CAS.
- A. M. Klibanov, Science, 1983, 219, 722–727 CAS.
- K. Anikin, C. Rocker, A. Wittemann, J. Wiedenmann, M. Ballauff and G. U. Nienhaus, J. Phys. Chem. B, 2005, 109, 5418–5420 CrossRef CAS.
- O. Hollmann and C. Czeslik, Langmuir, 2006, 22, 3300–3305 CrossRef CAS.
- X. L. Kong, L. C. L. Huang, C. M. Hsu, W. H. Chen, C. C. Han and H. C. Chang, Anal. Chem., 2005, 77, 259–265 CrossRef CAS.
- J. Y. Yoon, H. Y. Park, J. H. Kim and W. S. Kim, J. Colloid Interface Sci., 1996, 177, 613–620 CrossRef CAS.
- M. J. Malmsten, J. Colloid Interface Sci., 1998, 207, 186–199 CrossRef CAS.
- C. A. Haynes, E. Sliwinsky and W. Norde, J. Colloid Interface Sci., 1994, 164, 394–399 CrossRef CAS.
- K. Rezwan, L. P. Meier and J. L. Gauckler, Biomaterials, 2005, 26, 4351–4357 CrossRef CAS.
- C. Czeslik, R. Jansen, M. Ballauff, A. Wittemann, C. A. Royer, E. Gratton and T. Hazlett, Phys. Rev. E: Stat., Nonlinear, Soft Matter Phys., 2004, 69, 021401 CrossRef CAS.
- A. Wittemann, B. Haupt and M. Ballauff, Phys. Chem. Chem. Phys., 2003, 5, 1671–1677 RSC.
- A. Wittemann and M. Ballauff, Anal. Chem., 2004, 76, 2813–2819 CrossRef CAS.
- N. Stankeviciute, S. Sabah, A. Singh, M. Shaykh, A. A. Bakir, J. A. Arruda and G. Dunea, Am. J. Nephrol., 1998, 18, 285–290 CrossRef CAS.
- J. M. Brake, M. K. Daschner and N. L. Abbott, Langmuir, 2005, 21, 2218–2228 CrossRef CAS.
- M. I. Kinsinger, M. E. Buck, F. Campos, D. M. Lynn and N. L. Abbott, Langmuir, 2008, 24, 13231–13236 CrossRef CAS.
-
J. Y. Yang, K. Mathauer and C. W. Frank, in Microchemistry, ed. H. Masuhara, F. C. De Schryver, N. Kitamura and N. Tamai, North-Holland, Amsterdam, 1994, p. 441 Search PubMed.
Footnote |
† Electronic supplementary information (ESI) available. See DOI: 10.1039/c1sm06616a |
|
This journal is © The Royal Society of Chemistry 2012 |
Click here to see how this site uses Cookies. View our privacy policy here.