DOI:
10.1039/D3RA06976A
(Review Article)
RSC Adv., 2023,
13, 35088-35126
An overview on animal/human biomass-derived carbon dots for optical sensing and bioimaging applications†
Received
13th October 2023
, Accepted 15th November 2023
First published on 1st December 2023
Abstract
Over the past decade, carbon dots (CDs) have emerged as some of the extremely popular carbon nanostructures for diverse applications. The advantages of sustainable CDs, characterized by their exceptional photoluminescence (PL), high water solubility/dispersibility, non-toxicity, and biocompatibility, substantiate their potential for a wide range of applications in sensing and biology. Moreover, nature offers plant- and animal-derived precursors for the sustainable synthesis of CDs and their doped variants. These sources are not only readily accessible, inexpensive, and renewable but are also environmentally benign green biomass. This review article presents in detail the production of sustainable CDs from various animal and human biomass through bottom-up synthetic methods, including hydrothermal, microwave, microwave-hydrothermal, and pyrolysis methods. The resulting CDs exhibit a uniform size distribution, possibility of heteroatom doping, surface passivation, and remarkable excitation wavelength-dependent/independent emission and up-conversion PL characteristics. Consequently, these CDs have been successfully utilized in multiple applications, such as bioimaging and the detection of various analytes, including heavy metal ions. Finally, a comprehensive assessment is presented, highlighting the prospects and challenges associated with animal/human biomass-derived CDs for multifaceted applications.
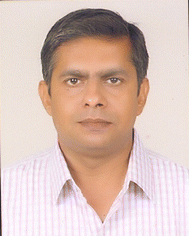 Prashant Dubey | Prashant Dubey received his PhD in Chemistry from the Indian Institute of Technology, Kanpur, India (2006) under the guidance of Professor Sabyasachi Sarkar. He also worked as a Postdoctoral Researcher with Professor C. J. Lee, School of Electrical Engineering, Korea University, South Korea. He is working as an Assistant Professor at the Centre of Material Sciences, University of Allahabad, Prayagraj, India since 03 November 2009. He has also been granted various fellowships (CSIR, DST, SSL, INSA, IASc, etc.) in his ongoing academic journey. His research interests include the development of low-dimensional nanomaterials for energy, environmental, and biological applications. |
1 Introduction
Carbon dots/quantum dots (CDs/CQDs) represent a family of zero-dimensional quasi-spherical carbon nanoparticles, which are typically less than 10 nm in size and primarily composed of a carbogenic backbone together with hydrogen (H), oxygen (O), and nitrogen (N)-containing surface functionalities.1,2 Owing to their different structures and surface chemistry, CDs can be broadly categorized as carbon nanodots (CNDs), polymer carbon dots (PCDs), and graphene quantum dots (GQDs).3,4 After the serendipitous discovery of nanosized CDs in 2004 during the purification of single-walled carbon nanotubes,5 they have attracted significant research attention in the scientific community as evidenced in Fig. 1. In 2006, Sun et al. observed bright luminescent emissions upon the surface passivation of acid treated carbon particles (obtained from laser ablation) by poly(ethylene glycol) (PEG), which, for the first time, were denoted as CDs.6 Since then, these emerging materials have become a rising star7 owing to their outstanding physiochemical properties (Fig. 2), such as multicolor photoluminescence (PL),8,9 chemical inertness,10 and resistance to photo-bleaching.11 Additionally, the aqueous/organic solvent dispersibility, high quantum yield (QY), low toxicity, good biocompatibility, and relatively inexpensive source for the low-cost, green synthesis of CDs further amplified their interest as an economical and environmentally sustainable class of fluorescent nanomaterials.7,9,12–15 Consequently, the utility of CDs have been proven for diverse applications, including solar cells,16 optoelectronic devices,17 biosensing18 and chemical/metal sensing,19,20 antibacterial agents,21 photocatalysis and electrocatalysis,22 displays,23 nanothermometers,24 drug delivery,25 energy storage,26 and live cell bioimaging27 (Fig. 2). It has been demonstrated that synthetic methods, reaction parameters, and starting precursors are highly responsible for the specific characteristics of CDs.7,28,29 Besides the conventional chemical precursors, there has been extensive research on the synthesis, properties, and applications of natural biomass/waste source-derived CDs as evidenced by the large number of review articles.30–55
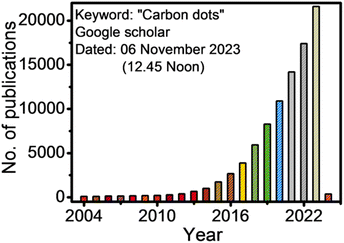 |
| Fig. 1 The number of publications according to the Google Scholar search since 2004 (keywords: carbon dots). | |
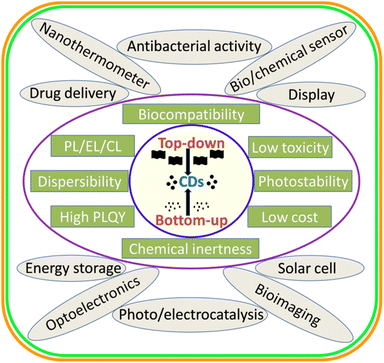 |
| Fig. 2 A schematic illustration of the broad synthetic approaches, various physiochemical properties, and applications of CDs. | |
Most of the previous review articles described the synthesis, structural/optical properties, and different applications of biogenic CDs derived from diverse natural/waste sources, such as plant components (leave, stem, root, etc.), vegetables, fruits, foods, beverages, and wastes (agricultural, industrial, food, etc.), but rarely considered animal/human-derived precursors. For instance, Sharma et al.30 discussed a variety of green source-derived CDs for sensing and bioimaging applications. Bhandari et al.32 presented the optoelectronic applications of CQDs/GQDs derived from various biomolecules including natural biomass. Meng et al.33 highlighted the current developments in biogenic CDs synthesized from diverse natural sources. Fan et al.34 focused on food waste-derived CQDs for food safety detection. Sekar et al.37 reviewed the heavy metal ion detection capability of CNDs derived from various green resources. Desmond et al.40 critically overviewed biomass-based CQDs for cancer therapy. Perumal et al.41 described the various applications of CDs derived from plant sources. The current developments in biomass-based CDs were also reviewed by Wareing et al.42 Chahal et al.43 discussed green-synthesized CDs from natural/non-toxic chemicals. Shahraki et al.46 presented the multifaceted applications of CDs particularly obtained from food waste. Ahuja et al.47 outlined the energy and bioremediation applications of CQDs obtained from biomass waste. Kaur et al.48 discussed the application of fruit waste-derived CDs in bioimaging. Manikandan et al.49 discussed the environmental applications of green CQDs derived from plant/agro-industrial waste. Xiang et al.50 critically reviewed CDs obtained from biomass/waste for biological and environmental applications. Fan et al.52 described biomass-derived CDs for sensing applications. Recently, Singh et al.54 included CDs synthesized from a variety of biomass for the detection of hazardous ions. In another recent review article, Fang et al.55 presented an overview of biomass-derived CDs, focusing on their preparation, property, and various applications. However, although there are numerous review articles related to biogenic CDs, a comprehensive overview of CDs derived from animal/human sources is limited in the literature. Natural biomass acquired from animal/human has attracted significant interest given that it is a minimal chemical intake, abundant, low-cost, and renewable green source. Moreover, the conversion of animal/human waste into value added products is an economical and sustainable approach to realize the waste-to-wealth initiative. Therefore, the assessment of animal/human biomass-derived CDs together with the recent achievements is paramount for future developments.
This review explicitly aims to present an overview of CDs synthesized from animal and human biomass for sensing and bioimaging applications. Initially, the diverse synthetic strategies, formation mechanisms, and various modifications associated with CDs are outlined. Furthermore, the comprehensive correlation/development of the size, QY, and elemental compositions of CDs derived from animal/human-based precursors using various preparation methods is presented with their structural and property description/relationship. Based on the preceding knowledge, the utilization of these animal/human biomass-derived CDs for metal ion/other analyte sensing and fluorescent biomarker applications is discussed together with a brief description of their other applications. Finally, this article is concluded by highlighting the achievements of these CDs in addition to identifying the future prospects of animal/human biomass as renewable resources and attempting to address the challenges/limitations in their practical implementation.
2 Diverse strategies for the synthesis of CDs
Broadly, CDs can be synthesized either via the top-down or bottom-up approach using chemical or natural precursors as starting materials. A schematic illustration of the various top-down and bottom-up synthetic routes is shown in Fig. 3. Both synthetic strategies have their own merits/demerits and have been developed with time for the preparation of narrow-sized CDs with excellent optical properties.
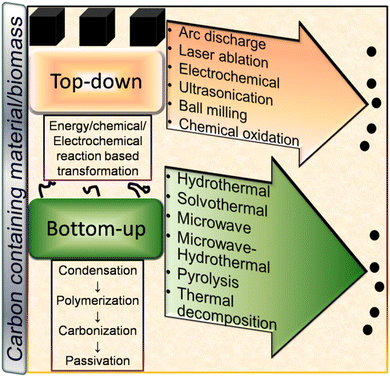 |
| Fig. 3 A schematic illustration of the various top-down and bottom-up techniques for the fabrication of CDs and their formation mechanism. | |
2.1 Top-down approach
This approach involves the breaking of bigger carbon precursors into nano-sized carbon particles. Here, the synthesis of CDs using various top-down methods is highlighted with specific examples.
2.1.1 Arc discharge method. The generation of a high-energy electric arc by placing two electrodes in close proximity under an applied potential drives the evaporation of the electrode material to produce CDs or other carbon nanostructures. Chao-Mujica et al.56 applied submerged arc discharge in water to synthesize CDs from a graphite rod. The size of the fluorescent CDs was found to be in the range of 1 to 5 nm with an appreciable QY (∼16%). It was proposed that the sublimed carbon vapor together with the exfoliated graphite/graphene fragments function as growth centers for the heterogeneous nucleation of polyhedral carbon nano-onions (CNOs) and large disordered polyhedral particles, which transformed into CDs and graphene oxide via water vapor oxidation.
2.1.2 Laser ablation method. An intense laser source (pulse) is also used to fragment micrometric carbonaceous solids into CDs.57,58 For instance, laser ablation of a carbon target (graphite) in ethylenediamine (EDA) and polyethyleneimine (PEI) resulted in the formation of crystalline CDs (1–3 nm) together with amorphous nanoparticles. Interestingly, a significant difference in the optical properties of CDs (extracted after dialysis) was observed in comparison to the remaining particles (retentate) for laser ablation conducted in EDA, indicating the presence of free fluorescent molecules. Consequently, the QY of EDA-CDs reached up to 10.4%, while PEI-CDs showed a low QY (2.5–3.3%).58
2.1.3 Electrochemical method. The electrochemical synthesis of CDs is another top-down approach, which is based on redox chemistry induced by applying a potential between two electrodes (cathode and anode).59 The first electrochemical synthesis of CDs (average diameter: 2.8 ± 0.5 nm) was reported in 2007, where multiwalled carbon nanotube (MWCNT)-coated carbon paper was used as the working electrode together with Pt-wire (counter electrode) and Ag/AgClO4 (reference electrode).60 In a recent report, Zhao et al.61 synthesized N,S-CDs through an electrochemical method for the fabrication of a flexible fluorescent film and information encryption application. An optimized potential (60 V, 3 h) was applied on two graphite electrodes (anode and cathode) together with phenylenediamine (N source) and thioacetamide (S source) to yield red/blue/green-emitting N,S-CDs. The green-emitting N,S-CDs showed a significantly higher absolute QY (12.99%) compared to their undoped counterpart (0.49%), which is ascribed to the narrowed band gap of CDs via –NH2 functionalization and increased excited-state electron transition related to the S atom of the C–S bonds.
2.1.4 Ultrasonication method. The breakdown of large carbonaceous precursors via high-energy ultrasonic waves is another simple and convenient top-down method, which has been explored for the synthesis of CDs.62 For example, the ultrasonic treatment (37 kHz) of CNOs in an H2SO4
:
HNO3 mixture (3
:
1) for 2 h followed by reflux treatment (100 °C, 2 h) resulted in the formation of green-emitting CDs (average size: 6.4 ± 1.8 nm) with excitation-wavelength independent (EWID) PL.63 Xu et al.64 synthesized biogenic CDs via the ultrasonic treatment (320 W, 80 kHz, 3 h) of kiwifruit juice in the presence of different additives such as ethanol, acetone, and EDA. The CDs obtained with acetone additive showed a smaller average diameter (4.8 nm) compared to that with ethanol (6.7 nm) and EDA (6.9 nm) additives. Although the size effect of the CDs was not very pronounced in the different additives, the absolute QY of the acetone additive-derived CDs was much higher (∼10%) compared to that of the other two CDs (∼3.7/∼2.4% for ethanol/EDA additive-derived CDs), which was explained by the occurrence of electron–hole recombination mainly from the surface trap sites.
2.1.5 Ball milling method. The breaking/milling of large-sized precursors by applying the kinetic energy of high-speed rotating balls has been considered a green mechanochemical approach for the large-scale synthesis of CDs.65 Mechanical ball milling of a mixture of cellulose and Mg with ZrO2 balls (360 rpm, 28 h) resulted in the formation of Mg-doped CDs (average diameter: 4.8 nm, QY: 9.45%), which exhibited potential as an Fe3+ sensor.66 Jeong et al.67 applied wet ball milling on spent coffee grounds to produce CDs (<2.0 nm). The surface property of the CDs was controllably tuned through the incorporation of oxygen/nitrogen-containing small molecules during the milling process, which improved the sensitivity and selectivity of the functionalized CDs for the detection of Fe3+ in aqueous medium.
2.1.6 Chemical oxidation method. The breakdown/etching of a bigger carbon source via chemical oxidation is another popular and simplistic top-down approach for the synthesis of CDs. For instance, He et al.68 demonstrated a facile and low-cost acid oxidation strategy for the production of a high-yield CD-based pickling solution from various saccharides (glucose, fructose, soluble starch, and saccharose; green C source) and concentrated H2SO4 (acid oxidant). The CDs containing pickling solution were obtained in a very short time (∼3 min), which showed excellent corrosion inhibition efficiency for Q235 carbon steel. Saikia et al.69 fabricated self-doped N,S-CDs from coal feedstock using 30% hydrogen peroxide (H2O2) as an oxidant with the assistance of ultrasonication. The resulting CDs (average size: 6 nm, QY: 9.35/16.96%) were used as a plant growth promoter with low phytotoxicity on plant metabolism.
2.2 Bottom-up approach
The preparation of nano-sized CDs via the condensation of small carbon precursors can be broadly termed the ‘bottom-up’ route. Some common bottom-up methods for the synthesis of CDs include hydrothermal (HT), solvothermal (ST), microwave (MW), microwave-hydrothermal (MW-HT), pyrolysis, and thermal decomposition (TD) (Fig. 3).
2.2.1 HT method. The HT method is one of the extensively explored synthetic techniques to achieve CDs from chemical compounds70,71 and natural precursors.72,73 The reaction parameters such as temperature and reaction time have been found to be crucial to tune the size, yield and fluorescence property of the synthesized CDs. Moreover, it is an inexpensive and readily available method with broad prospects. Recently, Hou et al.73 used HT conditions (200 °C, 4 h) and an egg white precursor to synthesize CDs (2–5 nm) for the fabrication of a CDs/layered zirconium phosphate composite. Combined with the synergy of two components, the composite showed the maximum Fe3+ adsorption capacity of 93.01 mg g−1 and detection ability in real environmental samples.
2.2.2 ST method. Similar to HT, the condensation of the starting precursors can also occur in non-aqueous medium. For example, the facile ethanol-assisted ST treatment of maize starch together with Na2HPO4·12H2O, NaH2PO4·2H2O, and urea resulted in the formation of N,P-codoped CDs (average diameter: ∼2.5 nm) with a QY as high as 76.5%.74 Hao et al.75 demonstrated a one-pot ST approach to synthesize CDs using folic acid (FA; 160 °C, 1 h) as a carbon source. The synthesized CDs possessed a narrow size range (2.8–3.2 nm) together with an appreciable QY under 360 nm excitation (31.2%), which were applied to fabricate a paper-based sensor for the detection of Hg2+ and a non-toxic nanoprobe for cellular imaging.
2.2.3 MW method. An energy efficient and low time-consuming MW-assisted method has also been explored with chemical and biomass precursors to derive functional CDs.76,77 Li et al.78 applied MW treatment (3 min) to a mixture of carbonized laver (biomass) and PEG to yield blue-emitting CDs for the detection of oxytetracycline. In another recent report, citric acid (CA) and tris base were used as starting precursors to synthesize CDs via the MW method. The fluorescent CDs showed potential for the detection of Mn2+ and tartrazine and anti-counterfeiting applications.79
2.2.4 MW-HT method. HT treatment of the starting precursors under MW irradiation has also been explored as a bottom-up process in the synthesis of CDs. In contrast to the typical HT method, MW-HT treatment achieves a desired reaction temperature in a shorter time. For instance, an MW-HT method (100 °C, 5 min) was applied on empty fruit bunch biochar to produce CDs with an average diameter of ∼4.5 nm. The obtained CDs were also implemented for the fluorescence quenching-based detection of Cu2+.80
2.2.5 Pyrolysis method. The direct pyrolysis of carbonaceous precursors has been considered a simple and facile bottom-up approach to synthesize CDs. It was observed that CA can be transformed into fluorescent CDs (QY: 29%) with the optimal carbonization temperature/time (160 °C/50 min).81 The solvent-free pyrolysis of starch at 300 °C yielded narrow-sized CDs (1.3–2.3 nm) with a QY as high as 21%. The synthesized CDs were also applied for the fluorometric detection of Ru3+ in aqueous solution.82
2.2.6 TD method. TD is slightly different from the pyrolysis method in the sense that the starting precursors in liquid/semi-liquid/solution state can be carbonized at elevated temperature. For example, an aqueous extract of Miscanthus grass together with EDA was thermally decomposed at 180 °C for 4 h to produce N-CDs (average size: ∼4.6 nm, QY: 11.7%), which were also applied as a nanoprobe for Fe3+ sensing.83 In another report, tender coconut water was directly heated at 100 °C to synthesize CDs for the detection of Zn2+.84
3 Formation mechanism of CDs
The formation mechanism of CDs follows different routes in the top-down and bottom-up synthetic approaches.
3.1 Top-down pathway
In the top-down route, graphite,56,58 MWCNTs,60 and other bulk carbonaceous sources (e.g., activated carbon,85 activated carbon fiber,86 and coal feedstock69) have been utilized as the starting source, which can be broken/cleaved into tiny CDs by employing physical, electrochemical or chemical oxidation method. The breaking/etching of inherent chemical bonds associated with bulk carbon drives the formation of CDs. This bulk to nano transformation can be induced either by high energy (arc discharge, laser ablation, ultrasonication-based acoustic cavitation, etc.) or chemical/electrochemical reaction (Fig. 3). Although the physical top-down strategy is less common for biomass-derived CDs, some researchers employed the chemical oxidation-based top-down formation route and natural/waste resources such as coal feedstock,69 candle soot,87 coal tar pitch,88 and carbonized olive solid waste89 to produce surface-passivated CDs. The harsh oxidizing environment (HNO3, H2SO4/HNO3, H2O2, etc.) eventually disrupts the bonding arrangements of bulk carbon, resulting in its breakdown into CDs. He et al.90 demonstrated the one-step transformation of carbon nanotubes (CNTs) into CDs via a thiol–ene click reaction. The sp2 carbon of CNTs reacted with thiomalic acid under reflux condition to produce –COOH-functionalized CDs for bioimaging application. The formation mechanism of coal-derived N,S-CDs is based on the breaking of the organic carbon linkage (binding bridge of crystalline fragments in the microstructure of coal) via ˙OH radicals (produced from H2O2). The oxidant can reach inside the interlayers of the graphitic structure (abundantly present in coal) to facilitate the disintegration of the bridging bonds such as –CH2–CH2–, –CH2–O–, –O–, and –S–. Moreover, acoustic cavitation induced by ultrasonication favours the breaking of the bonds between the graphitic structure and polycyclic aromatic fragments. These aromatic components eventually fragment into C2 units to form N,S-CDs via polymorphic reaction.69
3.2 Bottom-up pathway
The bottom-up formation of CDs relies on the solution-phase condensation/carbonization of small carbon-containing molecules. Organic molecules (conjugated or non-conjugated) or natural biomass can be used to produce CDs via the bottom-up route.91,92 The involvement of four stages (condensation, polymerization, carbonization, and passivation) is commonly speculated in the formation of CDs from small organic precursors (Fig. 3).91,92 Initially, the starting organic molecules get condensed into a small chain-like structure, which subsequently polymerizes to form polymer-like aggregates or carbon clusters. Subsequently, the carbonization/aromatization of polymer-like aggregates generates a carbonaceous core (amorphous/graphitic) at elevated temperature, which has an outer surface containing various functional groups.91,92 The reaction temperature/time plays a crucial role in determining the sp2/sp3 domains of CDs in the carbonization step.91,92 He et al.93 described the formation mechanism of MW-synthesized N-CDs, involving inter-and/or intra-molecular dehydration of an amide-rich organic precursor, followed by polymerization and carbonization to produce a carbon core possessing oxygen-containing functional groups. It was observed that the amount of core backbone increased with an increase in temperature and time, which is ascribed to the gradual transformation of the functional groups into a carbogenic core. Cao et al.94 proposed the formation mechanism of N-CDs derived from o-phenylenediamine using the ST method. Initially, 2,3-diaminophenazine (oxidized/cyclised product of the precursor) aggregates (H-bonding and π interaction) into a polymeric cluster, which creates a core–shell type of preliminary structure in the subsequent carbonization step. With time, the shells collapse with the evolution of the graphitised core in the form of N-CDs. Recently, Mohammed et al.95 investigated the effect of reaction time on the formation mechanism of N-CDs derived from 4-aminoantipyrine under HT condition. The spectroscopic results indicated the reduction and enhancement of the peak intensities in the aromatic and aliphatic/carbonyl group region, respectively, with the extension of the reaction time, indicating the structural changes during the synthesis process. These changes are correlated with the π–π interactions (non-covalent) of the benzene rings present in the precursor molecules. Moreover, the attachment of polar functional groups to the surface of N-CDs was accompanied by the hydrolysis of the pyrazole ring present in 4-aminoantipyrine.
Generally, biogenic CDs follow the bottom-up formation mechanism due to the abundance of organic molecules in the starting precursors. Although it is difficult to track the exact mechanism of these CDs due to the complex composition of their starting precursor, the decomposition of biomass into active components, followed by their polymerization, condensation, carbonization, and nucleation are the commonly accepted formation steps.96,97 For example, Shewanella oneidensis MR-1 bacterial cells first decomposed to macromolecules (polysaccharides/proteins/lipids, etc.), followed by hydrolysis to produce a large number of small organic molecules (saccharides/peptides/amino acids/hydrocarbon etc.). These small organic fragments get polymerized, condensed, and carbonized in the subsequent steps to form doped CDs due to nuclear burst.97
4 Modifications of CDs
The modification of CDs is vital for the improvement of physiochemical features, particularly their optical properties. Recently, Fan et al.98 reviewed the metal ion sensing application of modified CDs. The inherent modifications in CDs can be achieved either by heteroatom doping or surface functionalization.
4.1 Heteroatom doping
Various heteroatoms can be accommodated in the interior core or surface of CDs for the enhancement of their emission properties. Heteroatom-doped CDs usually possess abundant surface defects and active sites, resulting in the modification of their electronic structures via tuning of their initial band gap or formation of new energy levels.3,42 Consequently, doped CDs show better possibility for diverse applications due to the improvement in their optical characteristics and QY.3,42,98 N is one of the most common doping elements (atomic radius of C/N: 0.0914/0.092 nm), which is incorporated in CDs to improve their fluorescence properties.99 A green precursor (glucose) was HT treated in the presence of NH3 to produce N-CDs. The optimized N-CDs (N content: 10.94%) showed a significantly high PL emission with a QY of 9.6% compared to their undoped counterpart (QY: <1%), which is ascribed to the modifications in the surface/core electronic states of N-CDs. N-CDs were also used for the sensitive and selective detection of Cr6+.100 Sulfur (S) is another heteroatom that can be incorporated in CDs to enhance their optical properties. For instance, S-CDs synthesized from a mixture of ascorbic acid (AA) and thioglycolic acid (TGA) using the HT method (180 °C, 6 h) showed a QY as high as 32.07%. Moreover, they could also recognize Fe2+/Fe3+ from a real oral ferrous gluconate sample.101 N and S elements are also codoped in biogenic CDs to enhance their fluorescence property and sensing application.102 CDs doped with boron (B) and phosphorous (P) heteroatoms are also known in the literature. For example, P-doped CDs derived from a trisodium citrate–phosphoric acid (H3PO4) mixture under HT condition showed a satisfactory QY (16.1%) and Fe3+ sensing ability via the quenching of their fluorescence signal.103 Sadhanala et al.104 developed B-doped CDs through the ST treatment of catechol (C source) and naphthalene boronic acid (B source). The violet luminescent B-doped CDs (QY: 39.4%) were implemented for the sensitive/selective detection of Mg2+. The choice of the natural precursor in the synthesis of doped CDs is advantageous for the self-incorporation of heteroatoms in their structure.105 However, strategic doping can be achieved by using heteroatom-containing precursors together with the natural biomass. For example, the HT treatment of frozen tofu, EDA, and H3PO4 (210 °C, 4 h) produced N,P-codoped CDs, which were applied for sensing and bioimaging.106
Wang et al.107 firstly demonstrated the modification of CDs with metal ions (Mn2+) via the formation of Mn2+–coordination functional knots. Since then, a large number of metal ions has been used as doping agents for modifying the electronic state, charge density, and physiochemical properties of CDs.108 For example, the simple room-temperature synthesis of Si-CDs was achieved using 5-sulfosalicylic acid and 3-aminopropyl triethoxysilane (APTES), which were also used for the detection of Hg2+.109 The HT reaction between ethylenediamine tetraacetic acid disodium salt and FeCl3·6H2O yielded Fe-CDs with an Fe content as high as 13 wt%. Consequently, the Fe-CDs showed 2.6-times greater photocatalytic reduction ability (CO2 to methanol) compared to the undoped CDs.110 Zhang et al.111 used the traditional Chinese herb mulberry together with MgCl2·6H2O to synthesize Mg-CDs through the HT technique (200 °C, 10 h). The Mg-CDs were applied for in vitro osteoblastic differentiation and matrix mineralization.
4.2 Surface functionalization
The surface of CDs contains abundant functional groups, which can be modified with functional molecules either via covalent chemistry or non-covalent interaction.112 Covalent modification involves the direct binding of additional molecules to the surface of CDs, whereas π interaction, electrostatic conjugation and van der Waals force direct non-covalent modification. The coupling of the abundant –COOH groups present on the surface of CDs with amine containing compounds via an amide linkage is one of the simplest covalent functionalization strategies to improve the fluorescence intensity and amphiphilic character of CDs.113 CDs derived from CA and diaminonaphthalene under ST condition showed an excellent QY of 70% ± 10%, which is attributed to the pronounced edge amination of the CDs to reduce the number of defect sites, therefore delaying non-radiative recombination.114 The acid-catalyzed esterification reaction between –COOH and –OH-containing compounds is another simple modification strategy. Lactose-derived CDs were modified with mercaptosuccinic acid (MSA) via the esterification protocol, which showed an improved QY (46%) in comparison to the unmodified CDs (21%). Furthermore, the MSA-CDs were also evaluated for Ag+ sensing application with good sensitivity and selectivity.115 Sulfonyl chloride compounds are attached to –NH2-containing CDs via sulfonylation reaction. For example, amino-rich CDs (chitosan derived) were coupled with 4,4′-bis(1′′,1′′,1′′,2′′,2′′,3′′,3′′-heptafluoro-4′′,6′′-hexanedion-6′′-yl)chlorosulfo-o-terphenyl-Eu3+ (BHHCT-Eu3+) via sulfonylation reaction to fabricate a ratiometric fluorescent nanoprobe for the detection of Cu2+. Interestingly, the red fluorescence of BHHCT-Eu3+ (at ∼615 nm) was quenched in the presence of Cu2+ without affecting the blue fluorescence (at ∼410 nm) arising from CDs.116 The surface of CDs can also be redesigned with the silane moiety. For example, silylation chemistry was used to covalently functionalize CDs (CA derived) with tetraethyl orthosilicate and APTES, which possessed a large number of –NH2 groups for the coupling of TGA-modified CdTe QDs. The resulting ratiometric fluorophore showed excellent Cu2+ sensing ability via quenching of the fluorescence signal originating from the CdTe QDs.117 Suzuki et al.118 grafted methyltriethoxysilane and 3-glycidyloxypropyltrimethoxysilane on –NH2-containing CDs (prepared from CA and EDA using HT method) via epoxy-amine reaction, which were used to prepare organic–inorganic hybrid films for solid-state emitting devices. In a recent report, N-aminoethyl-γ-aminopropyltrimethoxysilane-functionalized CDs were applied for corrosion inhibition.119 Modification of the surface of CDs by copolymerization is advantageous to get a high molecular weight scaffold. For instance, Li et al.120 used anionic ring-opening polymerization (monomer: glycidol) to conjugate hyperbranched polyglycerol (HPG) on the surface of –OH-containing CDs (derived from α-cyclodextrin via the HT process). Although the QY of HPG-g-CDs was estimated to be slightly lower (1.2%) than that of bare CDs (1.5%), they exhibited high water dispersibility and low cytotoxicity for cell labelling and imaging.
Besides, CDs are also modified with functional moieties via non-covalent interaction. The extended π system on the surface of CDs is advantageous to modify their surface via π–π interaction. For example, Si-CDs prepared from glycerol and APTES through the MW method were modified with dopamine (DA) via face-to-face π–π interaction. The resulting Si-CDs@DA was applied for the fabrication of an Ag+ sensor and intracellular visualization of Ag+.121 According to the surface functional groups/attached moieties, the CDs can impart surface charge (positive or negative) for the electrostatic interaction of targeting species. For instance, PEI-functionalized CDs were prepared via non-covalent adsorption/wrapping of a cationic polymer (PEI), which were further modified with FA via electrostatic interaction. The resulting FA-modified PEI-CDs were used as a fluorescent nanoprobe (turn-on, in vitro/in vivo) to target folate receptor-positive cancerous cells.122 Carrot juice-derived CDs were non-covalently modified (electrostatic interaction) with PEI and Nile blue chloride (organic dye) to assemble a two-photon fluorescent nanoprobe for the detection of Cu2+ (turn-on–off) and S2− (turn-off–on).123 The complexation/coordination strategy is also executed on CDs to attach functional species on their surface. For instance, when orange peel-derived CDs were modified with ethylenediamine tetraacetic acid (EDTA) via complexation, they showed higher sensitivity for the detection of Cr6+ compared to bare CDs due to the strong chelating ability of EDTA.124
5 Developments in the precursors and synthetic methods for animal/human biomass-derived CDs
Besides plant-derived components as a natural resource, the use of animal- and human-based biomass has attracted tremendous interest over the past one decade for the synthesis of CDs and their doped counterparts. A representative illustration of the different animal/human-derived biomass used for the synthesis of CDs is shown in Fig. 4. These types of biomass are usually referred to as ‘green precursors’, which are sustainable, inexpensive, environmentally compatible, abundant, and renewable sources of carbon together with other elements. Moreover, the preparation of applied materials from these sustainable precursors is also a waste-to-wealth approach because many of them can be recognized as discarded biowaste. Although the synthesis of CDs from biomasses can be grouped into two broad categories, namely, top-down and bottom-up approach, the bottom-up protocol is widely implemented for this purpose. In this regard, the fusion of bio-derived organic precursor molecules under HT condition is one of the highly explored techniques; however, other methods such as pyrolysis, MW, alkaline hydrolysis, ultrasonication, MW-HT, thermal annealing, roasting (R)/grilling (G)/baking (B), acid carbonization, TD, autoclave TD (AT), and condensation are also adopted in the synthesis of biogenic CDs from various animal/human biomass (Table 1). Importantly, the bottom-up approach is advantageous compared to the top-down method for the production of CDs with a high yield, narrow size, heteroatom doping, good quality, and excellent property due to its simple and cost-effective operation. The AT calcination of grass at 180 °C and subsequent separation via centrifugation were probably the pioneering method for the synthesis of biogenic CDs.125 The authors proposed that these CDs are photoluminescent polymer nano-dots because of their crystalline structure with considerably smaller lattice spacing (0.20 nm) than that of graphitic carbon (0.34 nm). This pioneer report on the use of a natural source started a new trend of utilizing green biogenic resources for the synthesis of CDs. In this section, we summarize the synthesis and structural/compositional developments of CDs obtained from different animal and human biomass in chronological order using various synthetic approaches.
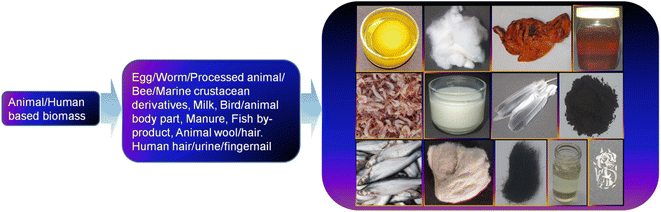 |
| Fig. 4 The representative photographs of the animal/human-based biomass applied for the synthesis of CDs. Top row (from left to right: egg white and yolk, SF, roasted chicken, and honey), middle row (from left to right: prawn, milk, pigeon feather, and cow manure), and bottom row (from left to right: fish, sheep wool, human hair, urine, and fingernails). | |
Table 1 A summary of the synthetic parameters, composition, size distribution, and QY of animal/human biomass-derived CDsa
Source |
Synthetic method |
Elemental composition (C, H, O, N, others) |
Size distribution (nm)/average size or maximum population (nm) |
QY (%) |
Ref. |
P: pyrolysis, AH: alkaline hydrolysis, U: ultrasonication, D: dialysis, TA: thermal annealing, RF: reflux, GC: gel column, EE: ethanol extraction, HPLC: high-pressure liquid chromatography, SBCW: subcritical water treatment, AC: acid carbonization, AP: amine passivation, CN: condensation, Ot.: other elements, and AFM: atomic force microscopy. From elemental analysis. From XPS analysis. From TEM/SEM EDX analysis. Absolute quantum yield. |
Animal biomass-derived CDs |
Egg white/yolk |
Plasma-induced pyrolysis |
[56.75, 6.03, 24.10, 13.12]b/[62.42, 8.74, 23.58, 5.26]b |
3.39/2.15 |
6.0/8.0 |
126 |
Egg shell membrane |
P (400 °C, 2 h); MW (5 min) |
[13.78, —, 56.86, 1.60, 27.75 (Na)]c |
2.0–12.0/5.0 |
14.0 |
127 |
Egg shell membrane |
P (300 °C, 4 h, air) |
[72.76, —, 10.58, 15.62, 1.04 (S)]c |
1.0–5.0/3.35 ± 0.5 |
8.0 |
128 |
Egg white |
HT (220 °C, 48 h) |
[46.40, 9.15, 30.39, 13.94, 0.12 (S)]b |
1.4–3.4/2.1 |
43, 61e |
129 |
Egg white (albumin) |
AH (1 M NaOH) |
[C, O, N, S]c |
—/3.2 ± 1.1 |
16.8 |
130 |
MW (700 W, 5 min) |
′′ |
—/13.4 ± 8.5 |
6.6 |
U (180 W, 5 h) |
′′ |
—/4.1 ± 2.8 |
2.3 |
Egg white |
TD (200 °C, 4 h, air) |
[C, O, N]c |
—/3.3 ± 0.4 |
43.0 |
131 |
Egg white |
MW (750 W, 25 min) |
[46.66, —, 36.79, 15.91, 0.65 (S)]c |
1.5–4.5/2.4 ± 0.6 |
— |
132 |
Egg white |
MW (1100 W, 3 min) |
[56.68, 4.57, 28.13, 9.80, 0.82 (S)]b |
—/2.98 ± 1.57 |
7.93 |
133 |
Pigeon egg white |
P (300 °C, 3 h, air) |
[68.83, —, 14.0, 9.44, 1.10 (S), 6.63 (Ot.)]c |
2.2–4.2/3.3 ± 0.5 |
17.48 |
134 |
Pigeon egg yolk |
′′ |
[78.51, —, 12.10, 5.83, 0.23 (S), 3.07 (Ot.)]c |
2.2–4.2/3.2 ± 0.5 |
16.34 |
Egg yolk oil |
TD (260 °C, 1 h); D |
[64.75, —, 10.16, 25.10 (Fe)]d |
1.0–17.0/<10.0 |
5.01 |
135 |
Egg yolk |
P (300 °C, 3 h, N2); HT (300 °C, 12 h) |
[58.76, —, 39.65, 1.59]c |
—/<10.0 |
35.0 |
136 |
Egg yolk |
P (220 °C, 24 h, air) |
[57.80, —, 29.20, 11.20]c |
1.0–6.0/2.04 |
— |
137 |
Bombyx mori silk |
HT (190 °C, 3 h) |
[—, —, —, 10.45]c |
4.0–7.0/5.0 |
13.9 |
138 |
Cocoon silk + H2O2 |
HT (260 °C, 50 min) |
[56.34, 5.63, 25.21, 12.82]b |
3.3–10.3/7.42 |
24.0 |
139 |
[53.81, —, 27.75, 18.43]c |
Silkworm chrysalis |
MW-HT (210 °C, 45 min) |
[71.32, —, 22.96, 5.72]c |
13.0–26.0/19.0 |
46.0 |
140 |
Bombyx mori silk + CA |
HT (200 °C, 3.5 h) |
[C, O, N]c |
4.0–6.0/5.6 |
61.1 |
141 |
Spider silk |
HT (180 °C, 10 h) |
[41.38, 8.65, 33.67, 16.30]b |
1.5–5.5/3.65 |
21.5e |
142 |
Silk fibroin |
MW-HT (200 °C, 20 min) |
[60.58, —, 21.72, 17.70]c |
—/6.1 ± 0.7 |
15.0 |
143 |
Silk fibroin |
HT (220 °C, 12 h) |
[59.25, 4.86, 22.68, 13.21]b |
—/7.5 |
38.0 |
144 |
Silk sericin |
MW (800 W, 2.5 min) |
[60.11, —, 35.78, 4.11]c |
2.0–7.0/4.0 |
3.60 |
145 |
Silk sericin |
P (150 °C, 2 h, air) |
[64.28, —, 21.10, 14.62]c |
4.0–6.5/5.3 ± 0.5 |
67.0 |
146 |
Silk fibroin + LMWC |
P (230 °C, 3 h, N2) |
— |
2.0–4.5/3.0 ± 1.5 |
66.0 |
147 |
Barbeque beef |
TA (5 h, Ar) |
— |
— |
40.0 |
148 |
RF (2.5 M HNO3); GC |
Hamburger |
R (220 °C, 30 min); EE & D |
[68.6, —, 17.34, 13.38, 0.56 (S), 0.12 (P)]c |
2.0–10.0/5.1 ± 4.9 |
23.25 |
149 |
Pike eel fish |
R (280 °C, 30 min); EE & D |
[66.58, —, 17.50, 15.91]c |
1.5–3.5/2.75 |
68.7 |
150 |
Pike eel fish |
R (300 °C, 30 min); EE & D |
[68.28, —, 15.33, 16.39]c |
1.75–4.25/2.75 |
80.16 |
151 |
Lamb |
B (250 °C, 30 min); EE & D |
[78.31, —, 19.70, 1.77]c |
0.8–3.8/2.0 |
10.0 |
152 |
Lamb |
B (350 °C, 30 min); EE & D |
[74.72, —, 17.11, 7.12]c |
0.6–2.4/1.69 |
45.0 |
153 |
Lamb |
B (280 °C, 45 min); EE & D |
[58.26, —, 27.82, 9.61, 4.31 (S)]c |
1.2–3.7/2.6 |
13.97 |
154 |
Duck |
G (170 °C, 60 min); EE & GC |
[70.48, —, 22.17, 6.25, 1.11 (S)]c |
0.7–2.3/1.3 |
4.4 |
155 |
Duck |
R (300 °C, 30 min); EE & D |
[64.67, —, 22.12, 12.73]c |
1.1–3.0/1.95 ± 0.41 |
38.05e |
156 |
Chicken breast |
R (230 °C, 30 min); EE & D |
[69.87, —, 19.81, 9.91]c |
0.6–2.8/1.7 ± 0.4 |
10.8 |
157 |
Chicken |
R (300 °C, 30 min); EE & D |
[67.61, —, 16.40, 15.36]c |
0.5–3.5/2.1 ± 0.6 |
17.46 |
158 |
Chicken breast |
R (200 °C & 300 °C, 30 min); EE & D |
[71.69, —, 18.84, 9.07]c & [67.15, —, 18.8, 13.29]c |
1.0–4.5/2.8 & 0.3– 2.1/1.2 |
— |
159 |
Chicken |
R (250 °C, 30 min); EE & D |
[75.70, —, —, 7.97]c |
2.0–6.0/4.7 |
12.84 |
160 |
Mackerel fish |
R (230 °C, 40 min); EE & D |
[52.70, —, 22.30, 24.10]c |
0.9–3.5/2.2 |
12.0 |
161 |
Mackerel fish |
G (230 °C, 30 min); EE & GC |
— |
1.0–4.0/2.94 ± 0.03 |
— |
162 |
Salmon fish |
R (200 °C, 50 min); EE & HPLC |
— |
1.0–4.0/2.73 ± 0.48 |
— |
163 |
Pork |
R (280 °C, 30 min); EE & D |
[C, O, N]c |
3.5–8.5/5.93 |
17.11 |
164 |
Beef |
R (280 °C, 30 min); EE & HPLC |
[68.68, —, 15.98, 10.60]c |
1.0–5.0/2.32 ± 0.71 |
— |
165 |
Honey |
HT (100 °C, 2 h) |
[44.24, —, 45.95, 9.81]c |
—/2.0 |
19.8 |
166 |
Rapeseed bee pollen |
HT (180 °C, 48 h) |
[52.30, 6.67, 32.52, 7.10]b |
0.75–2.25/1.7 |
12.8 |
167 |
Camellia bee pollen |
HT (180 °C, 24 h) |
[44.69, 6.44, 40.82, 6.67]b |
0.8–1.5/1.2 |
8.9 |
Lotus bee pollen |
′′ |
[41.30, 5.91, 46.70, 4.28]b |
0.75–1.5/1.1 |
6.1 |
Bee pollen |
HT (200 °C, 24 h) |
[C, O, N]c |
1.5–2.3/2.01 |
7.7 |
168 |
Rapeseed bee pollen |
HT (200 °C, 24 h) |
[67.30, —, 25.10, 7.60]c |
2.3–12.3/5.2 |
7.7 |
169 |
Honey |
D (48 h) |
[C, O]c |
2.3–5.4/3.2 ± 1.5 |
1.6 |
170 |
NaBH4 reduction |
4.6 |
Honey + Garlic extract + NH3 |
HT (200 °C, 6 h) |
[C, O, N, S]c |
4.0–13.0/8.29 |
4.19 |
171 |
Bee pollen (water) |
HT (180 °C, 24 h) |
[81.87, —, 13.78, 4.34]c |
1.0–5.0/2.64 |
2.15e |
172 |
Bee pollen (ethanol) |
′′ |
[67.85, —, 19.64, 12.51]c |
0.25–4.5/1.59 |
4.80e |
Shrimp egg |
P (180 °C, 25 min) |
[C, O, N]c |
0.5–6.0/3.25 |
18.5 |
173 |
Prawn shell |
RF (80 °C, 2 h); HT (200 °C, 8 h) |
[C, O, N]d |
2.0–8.0/4.0 |
9.0 |
174 |
Prawn shell |
HT (180 °C, 12 h) |
[68.50, —, 27.90, 3.60]c |
1.0–5.0/3.0 |
— |
175 |
Prawn shell + urea |
HT (150 °C, 1 h) |
— |
7.0–15.0/— |
5.84 |
176 |
Dried shrimp |
HT (170 °C, 12 h) |
[21.24, —, 48.65, 23.57, 6.22 (S), 0.32 (P)]d |
1.0–13.0/6.0 |
54.0 |
177 |
Crab shell + GdCl3 |
MW (220 °C, 10 min) |
[C, O, N, Gd]c |
—/4.0 ± 0.7 |
19.84 |
178 |
Crab shell |
U (20 kHz, 1 h) |
[C, O, N]c |
—/8.0 |
14.5 |
179 |
Crab shell |
P (210 °C, 20 min) |
[C, O, N]c |
3.0–9.0/— |
14.5 |
180 |
Crab shell |
HT (180 °C, 12 h) |
— |
—/10.0 |
— |
181 |
Shrimp shell |
HT (180 °C, 15 h) |
[C, O, N (14.40)]c |
1.5–5.5/— |
— |
182 |
Shrimp shell |
P (230 °C, 2 h, N2) |
[C, O, N, S, P]c |
3.0–5.0/— |
20.0 |
183 |
Shrimp shell |
HT (180 °C, 12 h) |
[C, O, N]c |
8.0–10.0/— |
27.14 |
184 |
Crayfish shell |
HT (200 °C, 8 h) |
[77.19, —, 13.86, 8.59, 0.36 (S)]c |
0.3–4.8/2.38 ± 0.14 |
50.2e |
185 |
Crayfish shell |
HT (200 °C, 6 h) |
[66.82, —, 20.01, 12.70, 0.47 (S)]c |
3.0–5.5/4.0 |
18.57 |
186 |
Crayfish shell |
HT (180 °C, 8 h) |
[63.0, —, 30.0, 7.0]c |
2.0–5.5/3.8 |
10.68 |
187 |
Mussel seafood |
HT (180 °C, 8 h) |
[62.15, —, 25.21, 10.43]c |
0.6–2.0/1.30 ± 0.25 |
15.2 |
188 |
Milk |
HT (180 °C, 2 h) |
[C, O, N]c |
2.0–4.0/3.0 |
12.0 |
189 |
Milk |
HT (180 °C, 8 h) |
[62.50, 8.38, 24.45, 3.92, 0.75 (S)]b |
4.6–5.3/5.0 ± 0.27 |
9.68 |
190 |
Milk + L-cysteine |
′′ |
[46.83, 8.15, 29.37, 8.22, 7.43 (S)]b |
3.78–3.98/4.0 ± 0.07 |
10.38 |
Milk + urea |
′′ |
[33.70, 7.14, 37.28, 20.88, 1.0 (S)]b |
3.15–3.35/3.0 ± 0.07 |
15.39 |
Cow milk |
HT (180 °C, 12 h) |
[92.15, —, 4.18, 3.66]c |
2.0–5.0/— |
9.6 |
191 |
Cow milk |
HT (180 °C, 12 h) |
— |
1.0–5.0/— |
— |
192 |
Milk |
MW (175 °C, 25 min) |
[50.77, 6.47, 32.38, 10.38]b |
1.5–3.0/2.3 ± 0.4 |
16.0 |
193 |
Milk |
MW (1250 W, 2 min) |
[73.30, —, 17.30, 9.30, 0.10 (P)]c |
1.0–7.0/— |
— |
194 |
Milk |
HT (180 °C, 4 h) |
[C, O, N, P]c |
—/10.0 |
10.0 |
195 |
Milk powder + FeCl3 |
HT (180 °C, 6 h) |
[60.28, —, 32.76, 6.05, 0.91 (Fe)]c |
2.0–4.5/2.9 |
8.73 |
196 |
Cow milk |
HT (180 °C, 8 h) |
— |
1.0–3.0/2.0 |
— |
197 |
Cow milk |
HT (180 °C, 2 h) |
[67.36, —, 22.73, 9.91]c |
4.5–11.5/7.0 |
38.0 |
198 |
Cow milk |
HT (180 °C, 4 h) |
[61.12, —, 31.49, 6.06]c |
1.0–2.0/1.6 ± 0.4 |
59.47 |
199 |
Milk powder + methionine |
HT (220 °C, 3 h) |
[C, O, N, S]c |
—/3.4 |
32.7 |
200 |
Pasteurized milk |
HT (170 °C, 12 h) |
— |
1.75–4.25/2.5 ± 1.0 |
5.7 |
201 |
Expired milk |
SBCW (180 °C, 1.2 MPa, 2 h) |
[70.11, —, 24.12, 5.77]c |
1.1–2.7/2.0 |
8.64 |
202 |
Denatured milk |
HT (120 °C, 3 h) |
— |
1.0–5.0/2.0 |
— |
203 |
Denatured sour milk |
HT (160 °C, 3 h) |
[76.16, —, 17.52, 6.32]c |
2.0–4.0/— |
13.0 |
204 |
Goose feather |
MW-HT (180 °C, 40 min) |
[43.50, 5.57, 34.70, 14.40, 1.83 (S)]b |
19.0–23.0/21.5 |
17.1 |
205 |
[48.40, —, 33.30, 16.30, 1.90 (S)]c |
Pigeon feather |
P (300 °C, 3 h, air) |
[74.78, —, 12.76, 11.30, 1.16 (S)]c |
2.8–4.3/3.8 ± 0.5 |
24.87 |
134 |
Chicken feather |
HT (180 °C, 18 h) |
[62.90, —, 35.80, 1.30]d |
—/35.0 |
— |
206 |
Carbonized hen feather + Zn salt |
P (350 °C, 3.5 h, air); MW (900 W, 6 min) |
[66.13, —, 26.16, 5.33, 2.37 (Zn)]c |
2.0–5.5/4.09 |
10.34 |
207 |
Carbonized hen feather + Mg salt |
′′ |
[53.0, —, 34.0, 9.0, 4.0 (Mg)]c |
1.5–5.5/3.4 |
9.23 |
Pig skin |
HT (250 °C, 2 h) |
[19.31 : 6.02 : 1.0 (C : O : N atomic ratio)]c |
3.5–7.0/5.58 ± 0.21 |
24.1 |
208 |
Pig skin |
P (300 °C, 2 h, N2); MW (400 W, 4 min) |
[82.93, —, 13.52, 2.43, 1.12 (Ot.)]c |
3.0–9.0/5.78 |
51.35 |
209 |
Pork |
HT (200 °C, 10 h) |
[C, O, N]c |
2.1–4.9/3.5 |
17.3 |
210 |
Pig skin collagen |
HT (240 °C, 3 h) |
[61.85, —, 21.75, 15.66]c |
0.8–1.8/1.25 ± 0.21 |
15.0 |
211 |
Pork rib bone |
P (700 °C, 5 h); AC; HT (200 °C, 10 h) |
[C, O, N, S, 5.35 (Ca)]c |
—/4.2 ± 1.2 |
— |
212 |
Pork liver |
HT (180 °C, 5 h) |
[64.06, —, 21.05, 14.88]c |
1.3–4.5/3.2 |
11.74 |
213 |
Chicken drumstick |
HT (190 °C, 5 h) |
[C, O, N]c |
2.0–9.0/5.0 |
32.86 |
214 |
Bovine bone |
P (700 °C, 5 h); AC; HT (200 °C, 10 h) |
[23.81, —, 53.28, 6.29, 1.54 (S), 15.09 (Ca)]c |
—/6.6 |
8.4 |
215 |
Pork bone |
′′ |
[28.59, —, 49.08, 7.19, 1.18 (S), 13.96 (Ca)]c |
—/6.7 |
7.3 |
Sheep bone |
′′ |
[27.35, —, 51.57, 4.65, 1.73 (S), 14.70 (Ca)]c |
—/9.5 |
8.0 |
GGEC |
HT (240 °C, 8 h) |
[64.86, —, 27.90, 7.24]c |
0.98–5.6/2.46 |
19.45 |
216 |
Chicken cartilage |
HT (200 °C, 8 h) |
[C, O, N]c |
3.5–11.5/7.6 |
10.3e |
217 |
Chicken bone |
HT (180 °C, 4 h) |
[75.34, —, 19.49, —]c |
1.8–4.6/3.2 ± 0.2 |
— |
218 |
Cow manure |
P (300 °C, 3 h, air); RF (5 M HNO3, 72 h); AP |
— |
2.3–6.8/4.8 |
65 |
219 |
Cow manure |
P (300 °C, 3 h, air); RF (5 M HNO3, 72 h); AP & 4-FPBA modification |
[48.0, —, 39.10, 4.11, 3.45 (S), 5.42 (B)]c |
2.0–6.0/4.2 ± 0.032 |
— |
220 |
Pigeon manure |
P (300 °C, 3 h, air) |
[41.96, —, 29.71, 12.17, 1.26 (S), 14.9 (Ot.)]c |
3.2–5.2/4.2 ± 0.5 |
33.5 |
134 |
Pigeon manure |
HT (150 °C, 6 h) |
[50.55, —, 37.81, 11.64]c |
12.0–20.0/15.65 |
25.92 |
221 |
Fish scale (grass carp) |
HT (200 °C, 24 h) |
[60.35, —, 25.05, 14.60]c |
1.0–4.0/— |
17.08 |
222 |
Fish scale (grass carp) |
HT (200 °C, 20 h) |
[65.20, —, 21.90, 12.90]c |
4.0–9.0/— |
9.0 |
223 |
Fish scale (grass carp) |
MW-HT (200 °C, 2 h) |
[C, O, N, S]c |
1.4–3.4/2.6 ± 0.8 |
19.92 |
224 |
Fish scale (crucian carp) |
HT (200 °C, 20 h) |
[70.0, —, 16.0, 14.0]c |
5.0–10.0/— |
6.9 |
225 |
Fish scale (Lethrinus lentjan) |
HT (280 °C, 3 h) |
— |
3.0–15.0/— |
— |
226 |
Fish scale (Ctenopharyngodon idella) |
Water extraction, 1 h |
[64.87, —, 24.16, 4.54, 1.33 (S), 1.16 (P), 1.64 (Ca)]c |
30.0–130.0/61.0 ± 3.6; 2.5–7.5/4.23 ± 0.13 |
15.6 |
227 |
Fish scale (Dicentrarchus labrax) |
HT (200 °C, 24 h) |
[66.0, —, 26.0, 8.0]c |
—/10.0 (AFM) |
6.0 |
228 |
Fish scale (Dicentrarchus labrax) |
HT (200 °C, 24 h) |
— |
—/10.0 |
— |
229 |
Fish scale (Labeo rohita) |
HT (180 °C, 7 h) |
— |
4.0–5.0/— |
— |
230 |
Fish scale (silver carp) |
HT (200 °C, 4 h) |
[65.80, —, 21.10, 13.10]c |
1.7–6.5/4.02 ± 0.89 |
6.04 |
231 |
MW (400 W, 1.5 min) |
[59.40, —, 24.10, 16.50]c |
2.4–7.2/4.23 ± 1.03 |
5.10 |
Shark cartilage (chondroitin sulphate) |
HT (240 °C, 3 h) |
[C, O, S, Na]d |
19.6–60.0/50.0 |
20.46 |
232 |
Carp roe |
HT (200 °C, 12 h) |
[66.0, —, 20.02, 13.98]c |
6.04–9.42/7.60 |
13.4e |
233 |
Fish scale collagen peptides |
HT (190 °C, 2 h) |
[65.10, —, 21.50, 13.40]c |
1.15–3.55/2.27 ± 0.48 |
9.29 |
234 |
MW (400 W, 3 min) |
[62.50, —, 21.10, 16.40]c |
5.75–9.75/7.58 ± 0.88 |
4.86 |
Sheep wool |
P (300 °C, 2 h); MW (700 W, 2 min) |
[4.74 : 1.72 : 1.0 (C : O : N atomic ratio)]c |
2.7–9.3/6.05 ± 1.67 |
22.5 |
235 |
Sheep wool |
MW-HT (200 °C, 1 h) |
[C, O, N, S]c |
1.5–4.5/2.8 |
16.3e |
236 |
Sheep wool |
HT (240 °C, 6 h) |
[62.54, —, 23.05, 13.66, 0.75 (S)]c |
4.0–8.0/5.9 |
25.6 |
237 |
Pig hair |
′′ |
— |
— |
20.1 |
Wool keratin |
HT (200 °C, 10 h) |
[C, O, 14.05 (N), S]c |
2.0–6.0/— |
8.0e |
238 |
![[thin space (1/6-em)]](https://www.rsc.org/images/entities/char_2009.gif) |
Human biomass-derived CDs |
Hair fiber |
AC (40 °C, 24 h) |
[61.22, —, 31.21, 5.43, 2.14 (S)]c |
4.0–10.0/7.5 |
11.1 |
239 |
AC (100 °C, 24 h) |
[59.73, —, 30.87, 5.37, 4.03 (S)]c |
2.0–7.0/4.2 |
4.02 |
AC (140 °C, 24 h) |
[60.56, —, 28.72, 5.64, 5.08 (S)]c |
2.0–5.0/3.1 |
5.38 |
Hair |
P (300 °C, 2 h, N2) |
[74.12, 6.46, 11.59, —]b |
—/2.3 |
17.3 |
240 |
Hair |
AT (200 °C, 24 h) |
[C, O, N]c |
2.0–8.0/4.56 |
10.75 |
241 |
Hair |
P (300 °C, 2 h, N2); MW (400 W, 4 min) |
[74.66, —, 14.75, 9.66, 0.93 (Ot.)]c |
2.0–7.0/3.57 |
86.06 |
209 |
Hair |
AT (180 °C, 24 h) |
[76.60, —, 13.80, 8.91]c |
7.0–13.0/11.0 |
38.0 |
242 |
MW (1100 W, 5 min) |
[84.98, —, 13.81, 1.21]c |
65.0–90.0/78.0 |
17.0 |
Hair |
P (300 °C, 1 h, air) |
[91.72, —, 3.22, 5.06]c |
1.0–2.6/1.76 |
38.0 |
243 |
Urine (unmodified diet) |
TD (200 °C, 12 h) |
— |
10.0–40.0/20.6 ± 8.4 |
5.3 |
244 |
Urine (vitamin C supplemented) |
′′ |
′′ |
2.0–28.0/11.4 ± 6.6 |
4.3 |
Urine (asparagus rich diet) |
′′ |
′′ |
10.0–70.0/38.8 ± 20.6 |
2.7 |
Urine + citron juice |
HT (180 °C, 7 h) |
[45.12, —, 38.30, 16.58]d |
2.5–5.5/4.0 |
34.5e |
245 |
Urine |
CN & GC |
[67.80, —, 27.57, 3.70, 0.65 (S), 0.28 (Fe)]c |
1.0–5.0/2.5 |
4.8 |
246 |
HT (200 °C, 8 h), GC |
[64.29, —, 27.20, 5.48, 2.79 (S), 0.23 (Fe)]c |
2.0–9.0/5.5 |
17.8 |
Fingernail + H2SO4 |
MW (400 W, 2 min) |
[57.80, —, 34.80, 5.90, 1.50 (S)]c |
1.8–3.0/2.2 |
42.8 |
247 |
Fingernail |
P (200 °C, 3 h, air) |
[55.80, —, 23.50, 19.20, 1.50 (S)]c |
2.0–4.5/3.5 |
81.4 |
248 |
Fingernail |
HT (200 °C, 3 h) |
— |
1.96–4.15/3.1 |
— |
249 |
5.1 Animal-derived precursors
The components of animal derivatives include an abundance of proteins, fats, carbohydrates, amino acids, vitamins, and minerals. These constituents hold potential to serve as viable biosources for the synthesis of both CDs and their doped analogues.
5.1.1 Animal egg derivatives as a carbon source. Chicken eggs contain a lot of proteins, carbohydrates, and fats together with heteroatoms, and therefore can be successfully used in the preparation of CDs via the bottom-up approach. Edible chicken egg (white and yolk) is probably the first animal-derived natural source employed for the synthesis of amphiphilic CDs via plasma-induced pyrolysis.126 Subsequently, different chicken egg-based products such as egg shell membrane,127,128 egg white,129–133 pigeon egg white/yolk,134 egg yolk oil,135 and egg yolk136,137 have been used for the preparation of doped CDs via various synthetic methods (Table 1). Among them, the treatment of egg white under HT129 or TD131 condition yielded N-CDs with a narrow size and excellent relative QY (43.0%). Recently, the MW-assisted synthesis of N,S-CDs from egg white was reported, which showed a low QY (7.93%).133 Also, egg yolk-derived N-CDs obtained via pyrolysis followed by HT treatment showed a high QY (35.0%), which was maintained even after 6 month (32.0%).136 Recently, egg yolk was also used to synthesize N-CDs via the pyrolysis method with a small average size of 2.04 nm and fairly high N content of 11.2 atomic%.137 Therefore, it can be concluded that the HT or TD/pyrolysis approach employing chicken egg white/yolk may be feasible to produce narrow-sized heteroatom-doped CDs with excellent QY.
5.1.2 Worm derivatives as a carbon source. Silk fibroin (SF), extracted from Bombyx mori silkworm/cocoon, is a nontoxic natural composite of proteins (fibroin, ∼75 wt%) and sericin (∼25 wt%). The N content in SF is as high as 18.0%, and therefore it can potentially be applied for the synthesis of doped CDs. However, despite its high N content, the earlier reports of SF-derived N-CDs synthesized via the HT method showed a low QY (Table 1).138,139 Subsequently, N-CDs directly derived from silkworm chrysalis via the MW-HT method showed a very high QY (46.0%) although they had a relatively large average diameter and small N content (Table 1).140 Interestingly, when SF was HT treated together with an equal amount of CA, narrow-sized N-CDs with a relatively high relative QY (61.1%) were obtained compared to the previous reports (Table 1).141 The high QY of N-CDs in this study is attributed to the surface passivation and formation of a pyrrolic structure via the effective N heteroatom doping in the carbon nanosheet-type core domain. Subsequent reports of N-CDs derived from spider silk,142 SF,143,144 and silk sericin145,146 showed appreciable QYs (Table 1). The high QY of 67.0% for the N-CDs (derived from silk sericin) prepared at a low pyrolysis temperature (150 °C) was ascribed to the formation of a carbon core together with O, N-based functional groups on its surface. Consequently, the created surface defects trapped excitons followed by radiative recombination to furnish a high QY.146 In another recent report, Horo et al.147 synthesized amine-functionalized CDs via the pyrolysis of SF (N source) and low molecular weight chitosan (LMWC, C source and functionalizing precursor) blends under an N2 atmosphere at 230 °C. They achieved a fairly high QY of 66.0% for the CDs obtained by LMWC
:
SF blends in a ratio of 1
:
1.5. The above-mentioned results clearly indicate that SF together with CA or LMWC and silk sericin may be a good biosource under the HT or pyrolysis condition to produce doped CDs with a narrow size distribution and high QY.
5.1.3 Processed animal products as a carbon source. The isolation of CDs from processed animal derivatives began with char from overcooked barbeque beef.148 Subsequently, CDs were extracted from roasted hamburger,149 grilled/roasted pike eel,150,151 baked lamb,152–154 roasted duck,155,156 roasted chicken/chicken breast,157–160 roasted mackerel fish,161,162 roasted salmon fish,163 roasted pork,164 and roasted beef.165 Among them, the excellent QYs of the roasted pike eel-derived N-CDs (68.7% and 80.16%) were remarkable, which were attributed to the abundant N-doping (15.91 and 16.39 atomic%, respectively) and surface-passivated amine-linkage or stable excited states.150,151 It was observed that the roasting/baking temperature149,151,153,156,158,159,164 or baking time154 had a profound impact on the structure–property relationship of the derived CDs. For example, N-CDs derived from roasted duck showed an increase in absolute QY of 10.53% (200 °C), 13.80% (250 °C), and 38.05% (300 °C) with an increase in the roasting temperature, which was ascribed to the increase in N contents in the N-CDs of 7.18%, 8.70%, and 12.73%, respectively. Moreover, the average particle size of N-CDs, i.e., 2.59 ± 0.57 nm, 2.00 ± 0.53 nm, and 1.95 ± 0.41 nm, respectively, decreased and gradually became uniform with an increase in the roasting temperature.156 Alternatively, the QY and average size of the baked lamb-extracted N,S-CDs (280 °C) at different baking times, i.e., 15/30/45 min, were found to be 2.93/10.89/13.97% and 4.1/3.7/2.6 nm, respectively. The authors suggested that the ingredients in the lamb initially produced large nanostructures, which subsequently turned into smaller CDs with an increase in the baking time.154
5.1.4 Bee derivatives as a carbon source. Honey, which mainly contains glucose, fructose, carbohydrates, proteins, amino acids, vitamins, enzymes, polyphenols, and minerals, can be obtained directly from bee hives. Earlier reports demonstrated the synthesis of N-CDs from honey166 and bee pollen167–169 using the HT method. The presence of nanoscale CDs (2.3–5.4 nm) in natural honey and improvement in QY after NaBH4 reduction (from 1.6% to 4.6%) via surface modification were further confirmed in a later report; however, the QY was much lower than the earlier report (Table 1).170 N,S-CDs prepared from the HT treatment of natural honey (C source), garlic (S source), and ammonia (N source) also yielded a similar QY as that of NaBH4-treated CDs (Table 1).171 Bee pollen biomass was further used to prepare N-CDs via the HT method. The bee pollen extract in water resulted in WBCDs (absolute QY: 2.15% and average size: ∼2.64 nm), while the pollen extract in ethanol produced EBCDs (absolute QY: 4.80% and average size: ∼1.59 nm).172 However, despite the high N content in EBCDs compared to WBCDs and previously reported bee pollen-derived N-CDs,167 the QY did not improve (Table 1), which signifies that high N doping alone may not be enough to achieve a high QY.
5.1.5 Marine crustacean derivatives as a carbon source. Marine crustaceans such as shrimp, crab, prawn, and crayfish are highly consumed seafood worldwide, and also recognized as natural sources for the synthesis of animal biomass-derived CDs. Heteroatom-doped CDs were successfully synthesized from shrimp egg,173 prawn shells,174–176 dried shrimp,177 crab shells,178–181 shrimp shells,182–184 crayfish shells,185–187 and mussel seafood188 using different synthetic protocols (Table 1). The applicability of the ultrasonication method in the synthesis of N-CDs from crab shells is shown in Fig. 5.179 Recently, the N-CDs synthesized from shrimp shell184 using the HT method possessed a size in the range of 8–10 nm and improved QY (27.14%) compared to the previously reported shrimp shell-derived doped CDs using the pyrolysis method (20.0%);183 however, it was much lower than that of the dried shrimp-derived N-CDs using the HT method (54.0%),177 justifying that dried shrimp is a good animal derivative and HT is an excellent synthetic process. The elemental analysis (EA) of N-CDs derived from dried shrimp by energy dispersive X-ray (EDX) spectroscopy showed the presence of 23.57 wt% of N atoms together with other elemental (S: 6.22 wt% and P: 0.32 wt%) peaks, and therefore the fairly high QY is attributed to the synergistic role of other elements together with the high N content for excellent fluorescence properties.177 A high absolute QY of 50.2%185 and good relative QY of 18.57%186 for N,S-CDs recently derived from crayfish shell using the HT method were also remarkable.
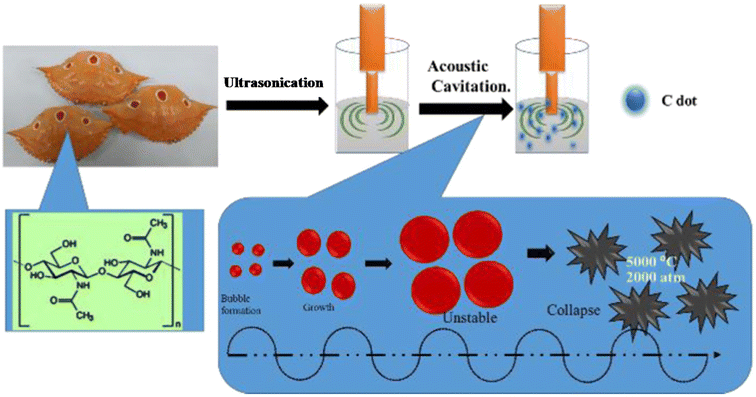 |
| Fig. 5 A schematic illustration of N-CDs obtained from crab shells by a sonochemical treatment. Reprinted from ref. 179, Copyright (2018), with permission from Elsevier. | |
5.1.6 Animal milk as a carbon source. Milk is another common animal derivative, which contains many nutrients such as proteins, fat, lipids, carbohydrates, lactose, and minerals. HT or MW-assisted CDs/doped analogues were successfully synthesized using milk in earlier reports.189–195 Some recent reports also evidenced the synthesis of doped CDs using cow milk/milk powder via the HT method.196–200 For example, Zhang et al.199 obtained N-CDs with a narrow size distribution (1.0–2.0 nm) and excellent QY (59.47%) using cow milk and the HT method. Another recent report also showed that HT-synthesized N,S-CDs from a mixture of milk powder and methionine (S source) showed a good QY (32.7%).200 Alternatively, pasteurized/expired/denatured milk-derived CDs resulted in relatively low QYs in the range of 5.7–13.0% (Table 1).201–204 These results clearly reflect that the HT treatment of pure milk is a good choice to obtain narrow-sized N-CDs with satisfactory QYs.
5.1.7 Birds and animal body parts as a carbon source. Birds and animal body parts such as goose/pigeon/chicken/hen feathers,134,205,206,207 pig skin,208,209 pork,210 pig skin collagen,211 pork rib bones,212 pork liver,213 chicken drumstick,214 animal bones,215 inner wall of chicken stomach (Galli Gigerii Endothelium Corneum, GGEC),216 chicken cartilage,217 and chicken bones218 are consumed for the preparation of heteroatom-containing CDs using various synthetic methods (Table 1). Noticeably, the effective self-doping of Ca element can be seen from animal bones-derived CDs; however, QYs are not as high as that in other animal body part-synthesized CDs (Table 1).212,215 For example, pig skin-derived N-CDs prepared by pyrolysis followed by MW irradiation possessed a high QY (51.35%).209 The synthetic steps involved in the preparation of N-CDs from GGEC via HT treatment at 240 °C for 8 h are shown in Fig. 6.216 The N-CDs in this report showed a small average particle size (∼2.46 nm) and good QY (19.45%) due to the effective graphitization of the core and increased N content in the core graphene, which resulted in more PL contribution from the core state rather than the molecular state. X-ray photoelectron spectroscopy (XPS) analysis also validated the low amino N-content compared to the graphene N-content in the synthesized N-CDs. Recently, hen feathers were applied as an animal source together with Zn/Mg salt for the synthesis of Zn/Mg-doped CDs. Interestingly, the Zn/Mg-doped CDs exhibited a smaller size (4.09/3.4 nm) and better QY (10.34/9.23%) compared to the undoped CDs (5.43 nm, 8.15%).207
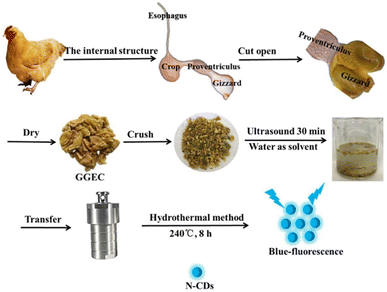 |
| Fig. 6 A systematic diagram of N-CDs derived from GGEC by the HT method. Reprinted from ref. 216, Copyright (2021), with permission from Elsevier. | |
5.1.8 Manure as a carbon source. As animal/bird biowaste, cow/pigeon manure is another source of carbon due to its high cellulose content. An earlier report showed that amine-passivated CDs (QY: 65%) can be obtained from the calcination of cow manure at 300 °C in air, followed by surface modification.219 The same research group further extended the surface functionalization of amine-passivated CDs via 4-formylphenylboronic acid (4-FPBA) covalent bonding, which resulted in PBA-CDs with N, S, and B hetero-elements.220 Heteroatom-doped CDs were also synthesized from pigeon manure134,221 with a QY as high as 33.5% via the simple pyrolysis of manure at 300 °C in air for 3 h.134
5.1.9 Fish by-products as a carbon source. Collagen protein-, fat-, and vitamin-rich fish scale can be employed as another waste bio-precursor for the synthesis of doped CDs. Fish scales of grass carp,222–224 crucian carp,225 Lethrinus lentjan,226 Ctenopharyngodon idella,227 Dicentrarchus labrax,228,229 Labeo rohita,230 and silver carp231 have been employed in the synthesis of heteroatom-containing CDs using the HT or MW synthetic protocol (Table 1). Besides fish scale, other fish byproducts such as shark cartilage,232 carp roe,233 and fish scale collagen peptides234 have also been employed to produce doped CDs. Table 1 clearly indicates that the QY of fish scale-derived doped CDs can reach up to 19.92%.224 Moreover, the QY of shark cartilage-derived doped CDs (20.46%) is also appreciable.232 A recent report on the preparation of fish scale collagen peptide-derived N-CDs under MW and HT conditions (Fig. 7a) showed a significant difference in their size, structure, and surface composition. The QY of the HT-derived N-CDs (9.29%) was found to be higher than that of the MW-synthesized N-CDs (4.86%) (Fig. 7a), which is ascribed to the higher carbonization and smaller size of N-CDs.234
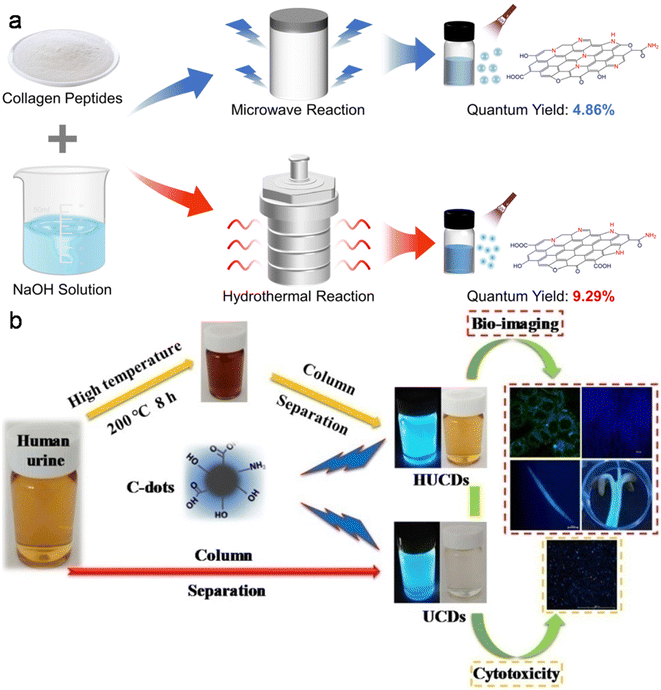 |
| Fig. 7 (a) MW and HT methods involved in the synthesis of N-CDs from fish scale collagen peptides. Reproduced/adapted from ref. 234 with permission from The Royal Society of Chemistry, 2023. (b) A schematic of the synthesis of UCDs/HUCDs from human urine and their applicability in bioimaging. Reprinted from ref. 246, Copyright (2019), with permission from Elsevier. | |
5.1.10 Animal wool and hair as a carbon source. Sheep wool235–237 and pig hair237 have also been used for the synthesis of doped CDs via different synthetic processes (Table 1), with the N,S-CDs obtained from the HT treatment of sheep wool showing a high QY (25.6%).237 Wool keratin (α-keratin), which contains cysteine (amino acid) as an important constituent, was also utilized by Song et al.238 to synthesize N,S-CDs via the HT method. The size of N,S-CDs was found to be in the range of 2–6 nm along with an N content as high as 14.05%. However, the absolute QY measured at an excitation wavelength of 365 nm was fairly low (8.0%) compared to the animal wool/hair-derived doped CDs (Table 1).
5.2 Human-derived precursors
Besides animal biomass, various nontoxic human-derived raw/waste sources such as hair, urine, and fingernails have also been applied to produce fluorescent CDs and their doped counterparts.
5.2.1 Human hair as a carbon source. Keratin is the main component (∼99.0%) of hair, which is rich in C, O, and N elements. In the earlier reports on doped CDs derived from human hair,209,239–241 it was observed that narrow-sized N-CDs (2.0–6.0 nm) with a QY as high as 86.06% were obtained from the pyrolysis of hair followed by MW irradiation.209 Subsequently, Singh et al.242 produced N-CDs from hair under AT (OCDs) and MW (MCDs) conditions. Interestingly, OCDs obtained under the conventional AT carbonization showed a much higher QY (38.0%) compared to the MCDs (17.0%), surpassing the QY of previously reported N-CDs (10.75%) using a similar synthetic protocol.241 The average size of OCDs was considerably smaller (∼11.0 nm) and possessed a higher N content (8.91%) compared to MCDs (average size: ∼78.0 nm and N content: 1.21%), which indicates that the surface defects and surface constituents play an important role in the emission properties.242 A recent report on human hair-derived N-CDs synthesized under pyrolysis condition (300 °C, 1 h, air) also showed a high QY of 38.0% together with a narrow size distribution (1.0–2.6 nm).243
5.2.2 Human urine as a carbon source. The liquid portion of sewage waste is majorly contributed by urine, which contains a significant proportion of urea and some amount of salts (Na+, K+, and Cl−) and heterocyclic compounds (uric acid, creatinine, etc.). Therefore human urine may be upcycled as a green and waste source to produce doped CDs. Unmodified (UPDs) and diet-modified (CPDs and APDs) pee dots were synthesized in an earlier report with different sizes and QYs using human urine (Table 1).244 Subsequently, N-CDs derived from a mixture of human urine and citron fruit extract via the HT method showed a significantly improved absolute QY (34.5%).245 Human urine was further explored to obtain doped CDs via a Sephadex G-25 gel separation protocol (UCDs) and HT method followed by column chromatography (HUCDs) (Fig. 7b).246 Interestingly, the QY of HUCDs (17.8%) was fairly high compared to UCDs (4.8%) due to the high content of N (5.48%) together with S element (2.79%), leading to more surface-trapped excitons under the excitation process.
5.2.3 Human fingernail as a carbon source. Human fingernails are a natural, low-cost, and non-toxic precursor, which primarily consist of α-helical fibrous protein (keratin). The MW dielectric heating of a mixture of fingernails and conc. H2SO4 resulted in narrow-sized N,S-CDs (1.8–3.0 nm) with a high QY (42.8%).247 Subsequently, N,S-CDs produced by the same group via the simple pyrolysis of fingernails showed a much higher QY (81.4%).248 The authors proposed that the existence of N and S elements in the form of cysteine-like RHN–C–C–SH structures was responsible for the high QYs of N,S-CDs.247,248 Afterwards, fingernails were again used without hazardous H2SO4 to obtain small-sized CDs (∼3.1 nm) under HT condition.249Based on the overall summary discussed above, it can be concluded that HT treatment is the most versatile approach to produce animal/human biomass-derived CDs with a high QY, narrow size, and effective heteroatom doping; however, the pyrolysis, MW, and MW-HT methods are also applicable to various animal/human biomass precursors. The starting precursor and initial composition also play an important role in achieving good-quality CDs. Significant improvements in the QYs can also be observed in some of animal/human-derived precursors by tuning the experimental conditions.
5.3 Formation mechanism of CDs derived from animal/human biomass
Some research groups discussed the formation mechanism of animal/human-derived CDs in their reports. For instance, the formation mechanism of N-CDs from egg white begins with the hydrolysis of egg protein into peptides and amino acids during the HT process. Subsequently, partial polymerization, followed by the carbonization of amino acids results in the generation of a carbon core coated with oligomers. With the progression of the reaction, the oligomers are gradually eliminated from the core surface to form N-CDs with –OH and –COOH functionalities.129 Subsequently, a similar formation mechanism was proposed for doped CDs derived from egg albumin/egg white using the alkaline hydrolysis/MW synthetic method.130,133
He et al.139 presented the combined effect of the top-down/bottom-up strategy in the formation of N-CDs from cocoon silk. The ˙OH radicals generated from H2O2 under HT condition cleave silk into small microrods. As the reaction proceeds, the fragments from the microrods get hydrolyzed into small molecules. Subsequently, the dehydration and polymerization of these molecules nucleate nanospheres for the formation of N-CDs via intermolecular dehydration/polymerization.
The MW-assisted transformation of silk sericin into N-CDs was studied through gas chromatography-mass spectrometry, which exhibited the release of various gases (CO2, NH3, NxOy, CxHyOz, H2O, etc.) during operational condition. The high/instant temperature and generated OH− cleave sericin into amino acids and polypeptides. These active components get aggregated and carbonized in subsequent steps to form a graphitized carbon core attached with heteroatom-containing functional groups.145 The N-CDs produced from silk sericin under pyrolysis condition underwent dehydration, depolymerisation, carbonization, and self-passivation to form a carbon core decorated with functional groups. It was also observed that the graphitization of the core carbon (ID/IG: 0.95/0.69/0.63/0.51) and average size of N-CDs (5.3/6.7/8.4/9.6 nm) increased with an increase in the pyrolysis temperature (150/200/250/300 °C).146
Bi et al.151 discussed the formation mechanism of N-CDs extracted from pike eel at different roasting temperatures, indicating the intensified polymerization/carbonization of fish ingredients (carbohydrates, proteins, and lipids) at a higher roasting temperature. The light pink fish flesh (Fig. 8A) turned a golden colour (Fig. 8B) at 160 °C, which was composed of irregular microstructures (Fig. 8G) with weak fluorescence (Fig. 8M) due to its insufficient combustion. When the roasting temperature was increased to 200 °C, the flesh showed a brown/curled appearance (Fig. 8C) with the evolution of a few carbon nanoparticles in the microstructure (Fig. 8H) and weak fluorescence (Fig. 8N), indicating the disintegration of the large biopolymer clusters. The formation of char on the surface of the flesh (Fig. 8D) and shrinkage of the clusters (Fig. 8I) occurred at a roasting temperature of 230 °C due to the extension of carbonization, which also showed stronger fluorescence (Fig. 8O). A further increase in the roasting temperature (260 °C) resulted in more charred product on the flesh surface (Fig. 8E), possessing a large number of CDs (Fig. 8J) and intensified blue fluorescence (Fig. 8P). The disintegration of the biopolymer clusters was significant at this stage, which was further extended at 300 °C. Consequently, the flesh surface turned into charred mass (Fig. 8F) having small-sized CDs (Fig. 8K, 1.75–4.25 nm) with strong cyan fluorescence (Fig. 8Q). A similar observation/explanation of escalated carbonization at a higher processing temperature was also presented for N-CDs derived from duck156 and chicken.158
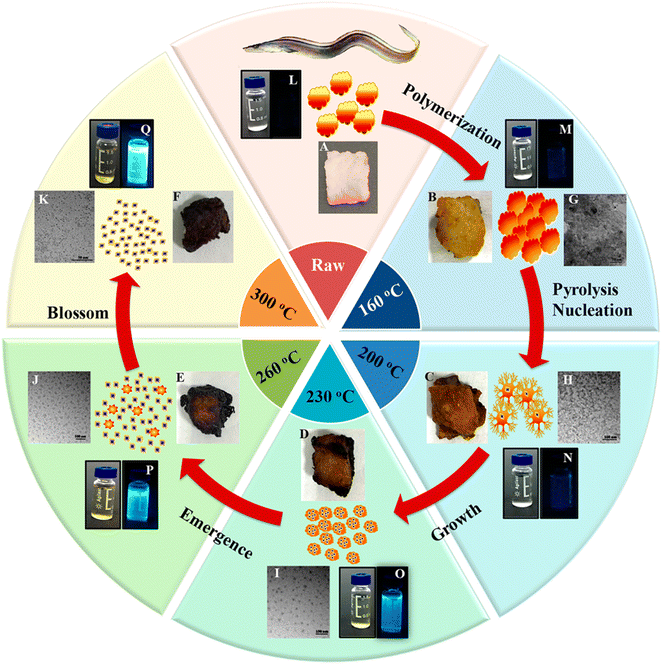 |
| Fig. 8 A stepwise illustration of the growth and formation mechanism of pike eel-derived N-CDs (red arrow). (A–F) The digital pictures of fish mass obtained at different roasting temperatures. (G–K) TEM images of corresponding fluorescent materials isolated at various stages. (L–Q) The digital photographs of aqueous soluble CDs under day per UV (365 nm) light. Reprinted (adapted) with permission from ref. 151, Copyright (2018), the American Chemical Society. | |
The growth mechanism of N-CDs derived from human hair using the AT/MW method starts with the cutting/unzipping of the tertiary structures present in hair to generate a significant amount of small hydrocarbon. Subsequently, the C–C bonds are disintegrated with the evolution of nitrogen (depolymerisation), followed by cross-linking to form N-CDs.242
6 Physiochemical properties of animal/human biomass-derived CDs
6.1 Structural characteristic and chemical composition
Structurally, most animal/human biomass-derived CDs are composed of a carbogenic core (predominantly sp2-hybridized carbon) and outer shell comprised of O- and/or N-containing functional groups attached to its surface. C, O, and N are found to be very common elements in these CDs; however, the successful doping of other elements (S, P, Fe, Zn, Mg, etc.) has also be reported, as shown in Table 1. There are numerous reports showing that the incorporation of heteroatoms significantly modifies the composition of CDs and effectively improves their QY (Table 1). The surface functionalities of the shell not only provide good aqueous solubility/dispersibility, but also tune the optical properties of animal/human biomass-derived CDs. Generally, surface zeta potential analysis is used to determine the strength of the electrostatic repulsion amongst CDs due to the presence of abundant surface charge. A large negative zeta potential value suggests strong electrostatic repulsion among the negatively charged functional groups and better stability of animal/human biomass-derived CDs in aqueous solution.128,129,131,132,140–142,144,185,189,191,197,203,207,208,215 Besides a negative zeta potential, animal/human-derived CDs with positive zeta potentials have also been reported.137,198,230,236,243 The functional groups and elemental compositions of animal/human biomass-derived CDs are often analyzed by Fourier transform infrared (FTIR) spectroscopy and XPS. Fig. 9a–d show the representative FTIR and XPS results of chicken breast-derived N-CDs, respectively.159 The N-CDs obtained at a roasting temperature of 200/300 °C presented broad absorption peaks at ∼3267/3425 cm−1 due to the stretching modes of the O–H or N–H bonds. The peaks at ∼2925/2932 cm−1 were assigned to the –CH2 stretching vibrations. The typical absorption peaks at ∼1593/1674 cm−1, 1395 cm−1, and ∼1121 cm−1 were attributed to the C
O stretching modes, vibrations corresponding to the C–N bonds, and C–O–C bonds, respectively. Interestingly, the peak intensities for the C–N and C–O–C bonds were low for the N-CDs extracted at 300 °C compared to that at 200 °C, which was ascribed to the partial breakdown of these bonds at a higher roasting temperature (Fig. 9a). Moreover, the XPS survey spectrum of the N-CDs synthesized at 300 °C exhibited C 1s (285.0 eV, 67.15%), N 1s (400.0 eV, 13.29%), and O 1s (531.0 eV, 18.80%) elements (Fig. 9b). The four deconvoluted peaks located at ∼284.5, 285.5, 286.4, and 288.6 eV in the C 1s core level peak are assigned to the C
C, C–N, C–O, and C
O bonds, respectively (Fig. 9c), while the high-resolution N 1s spectrum (Fig. 9d) showed two peaks at ∼398.1 eV (amide N bonds) and ∼398.6 eV (pyridinic N bonds). Many characterization techniques such as powder X-ray diffraction (PXRD), high-resolution transmission electron microscopy (HRTEM), and Raman spectroscopy are applied to investigate the crystalline nature and defect density of CDs. The PXRD spectra of CDs and doped CDs synthesized from animal/human biomass typically exhibit a diffraction peak in the 2θ range of 20° to 27°, which corresponds to the (002) graphitic plane. For example, the PXRD pattern of chicken cartilage-derived N-CDs showed an intense peak at 2θ = 23.2° due to the (002) graphitic planes (Fig. 9e).217 Moreover, a nearly spherical shape (Fig. 9f), narrow size distribution (Fig. 9g), and high crystallinity with 0.24 nm lattice spacing corresponding to the (100) graphitic plane (inset of Fig. 9f) were also revealed for these N-CDs by TEM and HRTEM analyses.217 Most animal/human biomass-derived CDs or their doped counterparts exhibit spherical/quasi-spherical shapes with a size of less than 10.0 nm (Table 1); however, the synthesis of large-sized CDs from animal biomass has also been reported. For example, CDs derived from silkworm chrysalis (∼19.0 nm),140 goose/chicken feathers (∼21.5/35.0 nm),205,206 pigeon manure (∼15.65 nm),221 fish scale (∼61.0 nm),227 and shark cartilage (∼50.0 nm).232 Biogenic CDs with an average size of above 10 nm were also prepared using human biomass such as human hair (∼11.0/78.0 nm)242 and human urine (UPDs: 20.6 nm, CPDs: 11.4 nm, and APDs: 38.8 nm).244 Generally, the Raman spectrum of carbonaceous nanomaterials including CDs comprised of a G band (first-order Raman band due to the in-plane vibration of sp2 carbon) and D band (defect centers induced by sp3 carbon). A high ID/IG ratio in nano-carbon indicates the presence of disordered structure. For example, the Raman spectra of OCDs and MCDs synthesized from human hair showed two typical peaks at ∼1316 cm−1 (D band) and ∼1584 cm−1 (G band) with ID/IG ratios of 1.12 and 1.14, respectively (Fig. 9h), suggesting the disordered structures of nano-carbon. Moreover, the shoulder peak at ∼1200 cm−1 in the spectrum of OCDs (dotted circle in Fig. 9h) is ascribed to the π–π charge transfer between OCDs and NO rings.242 The molecular weight and various functional groups/minute structural features of salmon fish-derived CDs were confirmed by matrix-assisted laser desorption ionization time-of-flight mass spectrometry (MALDI-TOF-MS) and 1H nuclear magnetic resonance (NMR) and 13C NMR spectra.163 The predominant peak at 1056 m/z indicated the small size of the isolated CDs (Fig. 9i). Moreover, the 1H NMR signals at 1.23, 2.96, and 3.12 ppm were attributed to the alkyl, R3 N–C–H, and –O–C–H protons, while the peaks in the range of 3.82–4.1 ppm and 7.0–9.0 ppm were ascribed to the protons attached to the alcohol groups and aromatic protons, respectively (Fig. 10a). The peaks in the range of 10–30 ppm, 30–40 ppm, 40–65 ppm, 65–90 ppm, and 110–180 ppm in the 13C NMR spectrum are assigned to the carbon of the –CH3 groups, carbon from the
CH/
C–/–C=C–O– groups, carbon in the –C
N–/
N–CH3/
C–N– structures, sp3 carbon of alcohols/ether groups, and aromatic carbon, respectively (Fig. 10b).
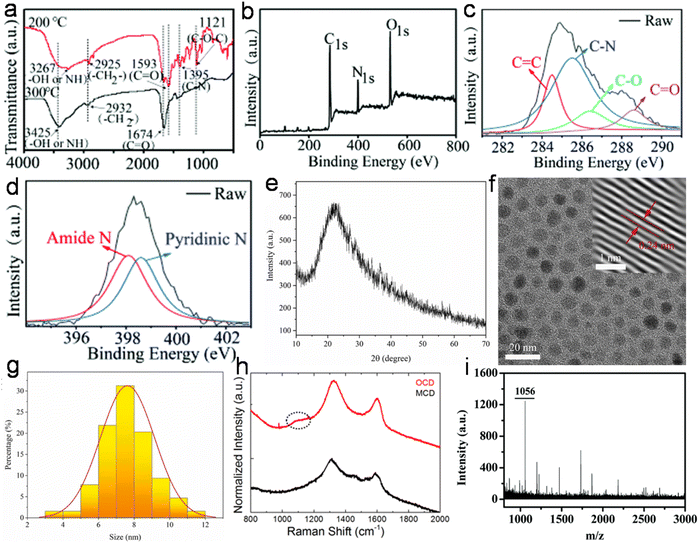 |
| Fig. 9 (a) The FTIR spectra of N-CDs extracted at 200/300 °C. (b) The XPS survey spectrum, (c) C 1s fine spectrum, and (d) N 1s fine spectrum of N-CDs obtained at 300 °C from chicken breast as animal biomass. Reproduced/adapted from ref. 159 with permission from The Royal Society of Chemistry, 2020. (e) The PXRD profile, (f) TEM/HRTEM (inset) image, and (g) size distribution of N-CDs derived from chicken cartilage. Reprinted from ref. 217, Copyright (2022), with permission from Elsevier. (h) The Raman spectra of N-CDs (OCD and MCD) synthesized from human hair. Reprinted from ref. 242, Copyright (2020), with permission from Elsevier. (i) The MALDI-TOF-MS spectrum of roasted salmon fish-derived CDs. Reproduced/adapted from ref. 163 with permission from The Royal Society of Chemistry, 2019. | |
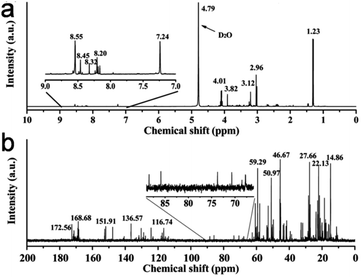 |
| Fig. 10 (a) The 1H NMR and (b) 13C NMR spectra of CDs isolated from roasted salmon fish. Reproduced/adapted from ref. 163 with permission from The Royal Society of Chemistry, 2019. | |
6.2 Optical properties
The remarkable optical features of CDs such as their absorption, emission wavelength, excitation-tunable emission, QY, lifetime, photo and chemical stability are crucial for their potential applications. Table S1† clearly reveals that most animal/human biomass-derived CDs are comprised of a distinct absorption in the UV range (200–400 nm) and tails extended into the visible region. The lower wavelength peak is mainly due to the core domain of CDs (featured by the sp2-hybridized carbon framework of conjugated π electrons), and generally recognized as the π → π* transitions of the extended aromatic rings and C
C segments (Table S1†). Meanwhile, the peak/shoulder at a relatively higher wavelength in the UV region is correlated with the low energy n → π* transitions of C
O bonds/other functional groups attached to the surface or trapped excited state energy on the surface/edge (Table S1†). Therefore, it can be concluded that irrespective of the animal/human biomass utilized as the starting precursor, the optical absorption of CDs originates from their core and/or surface defects/surface states/functional groups. For example, Mohamed et al.200 observed two sharp absorption peaks at ∼230 nm and ∼315 nm, corresponding to the π → π* transitions of C
C and n → π* transition of the C
O bonds in N,S-CDs derived from a mixture of milk and methionine (Fig. 11a). Additional modifications in the surface functional groups are also directed to improve the optical absorption of CDs. For example, when crab shell-derived N-CDs were conjugated with FA via EDS/NHS coupling reaction, it resulted in strong peak at ∼210 nm and higher absorption at ∼280 nm in comparison to the bare N-CDs (λabs = 260 nm).179
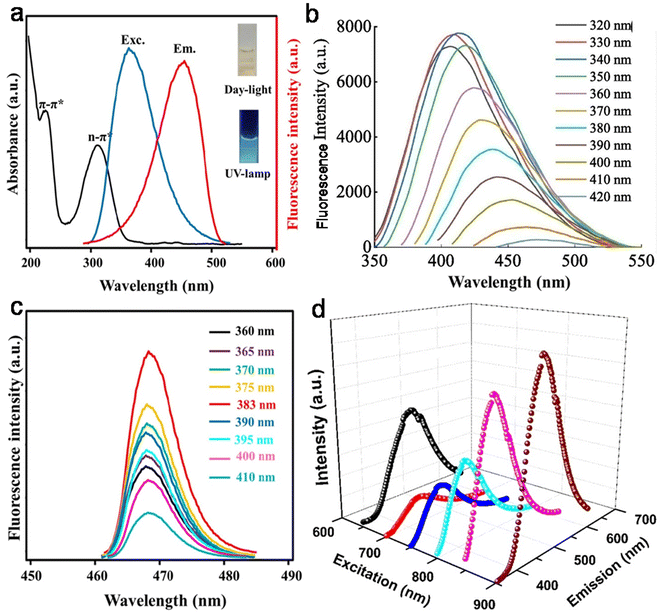 |
| Fig. 11 (a) The absorption (UV-vis) and (c) EWID fluorescence spectra of N,S-CDs derived from a mixture of milk powder and methionine (the inset of (a) the aqueous dispersion of N,S-CDs under day and UV light). Reprinted from ref. 200, Copyright (2023), with permission from Elsevier. (b) The EWD PL behavior of N-CDs synthesized from egg yolk. Reprinted from ref. 137, Copyright (2022), with permission from Elsevier. (d) The UCPL spectra of Bombyx mori SF-derived N-CDs. Reprinted from ref. 144, Copyright (2020), with permission from Elsevier. | |
PL is one of the most fascinating features of CDs, which determines their utility in sensing and bioimaging applications. Particularly, the excitation-wavelength dependent (EWD) emission of CDs is important for multicolor imaging applications. The PL emissions in most animal/human biomass-derived CDs exhibit the typical Stokes-type behavior (down-conversion, Table S1†). It was observed that some animal-derived CDs showed emission spectra with good symmetry and narrow full-width at half-maxima, which were correlated with the narrower size distributions of the CDs.130,201 Moreover, depending on the physiochemical characteristics of animal/human biomass-derived CDs, their PL spectra may follow either EWD emission or EWID behavior (Table S1†). The PL behavior of CDs is still under debate; however, there are some hypotheses for its origin, as follows: (i) core-based emission due to the band gap transitions in the conjugated π-skeletons, which is determined by the size of the carbogenic core (quantum size effect) and degree of graphitization; (ii) surface state-based emission (or trapping of excited state energy at the surface/edge states) induced by the surface/edge defects and/or passivation of the core surface via the attachment of functional groups; and (iii) molecular state-type emission due to the fluorophores (free or bonded) present in the CDs. A closer look at the emissions behavior and structural features of animal/human biomass-derived CDs indicated that either of the first two factors or their combination may influence the PL feature of CDs; however, one of them may be dominant in some cases (Table S1†). Fig. 11b shows the typical profile of the EWD-dependent PL spectra of N-CDs (egg yolk derived) in the λex range of 320 to 420 nm and maximum emission intensity at 340 nm excitation.137 Most of the animal/human source-derived CDs showed blue (inset of Fig. 11a) or blue-green luminescence under the irradiation of UV light (λex = 365 nm) (Table S1†). The EWD emission is a distinct observation (Table S1†); however, EWID emissions135,145,185,200,212,215,227,231 or the combination of both EWD and EWID223,225 were also noticed in animal biomass-derived CDs (Table S1†). For example, recently synthesized N,S-CDs (from a mixture of milk powder and methionine) exhibited EWID emission (λem = ∼ 470 nm) in the λex range of 360–410 nm and maximum emission intensity at λex = 383 nm (Fig. 11c).200 Interestingly, Ca-self-doped CDs specifically derived from animal bones (without additional purification steps) showed EWID behavior.212,215 The authors speculated that the functional groups attached to the surface of the CDs effectively passivated the surface defects, and therefore the EWID characteristics originated from the single radiative transition of the core sp2 carbon.
6.2.1 Up-conversion PL (UCPL). In addition to common down-conversion fluorescence, UCPL emission is a newly discovered phenomenon that has also been observed in animal/human biomass-derived CDs (Table S1†). It is an anti-Stokes process in which the emitted photons have higher energy (shorter emission wavelength) than the excited photons energy (longer excitation wavelength) and often interpreted by the multiple photon activation mechanism.190,211,223,225,239 The UCPL behavior in animal/human biomass-derived CDs was first observed in early 2013 in Bombyx mori silk-synthesized N-CDs.138 Subsequently, Wang et al.144 observed the UCPL property in N-CDs derived from the same bio-precursor, which showed red shifts in their emission peaks with an increase in λex from 650 nm to 900 nm (Fig. 11d). UCPL behavior was also observed in CDs derived from many other animal/human biomass such as human hair,239 honey,166 milk,190 pig skin collagen,211 and fish scales223–225 (Table S1†). The UCPL of these biogenic CDs may open a new development in bioscience as a cellular imaging platform with two-photon luminescence microscopy and design of efficient catalysts for energy technology.
6.2.2 Stability (effect of concentration, light, temperature, pH, solvent, and ionic strength). The effect of concentration and various environmental factors such as exposure to light, temperature, pH, and ionic strength on the PL response of animal/human biomass-derived CDs has been routinely investigated to establish their wide applicability. The optimum concentration of CDs is always required to obtain a high PL intensity. For example, recently reported N-CDs (egg white derived) showed the maximum PL intensity at the optimum solution concentration (0.1%), while a further increase in concentration resulted in a decreasing trend due to the inner filter effect (IFE) and aggregation-based quenching. Moreover, the gradual red shift in λem from 441 nm to 498 nm with higher concentrations of N-CDs (0.1% to 0.5%) was explained due to the increase in H-bonding between the surface-attached functional groups, and therefore decrease in highest occupied molecular orbital-lowest unoccupied molecular orbital energy gap.133The photo-bleaching resistivity of CDs is a desirable criterion for reliable fluorescent nanoprobes, and therefore frequently analyzed for animal/human source-derived CDs after exposure to UV/xenon light. Interestingly, the doped CDs isolated from grilled hamburger,149 baked lamb,153 and roasted chicken158 at higher temperature had better stability against photo-bleaching due to their smaller size and less functional groups. When bee pollen-derived N-CDs were exposed to a high-power UV source (24 h), they showed a reduction in PL intensity by up to 50.0% without much change in their absorption.172 This unique phenomenon was explained by the generation of ˙OH radicals, which effectively destroyed the surface emissive centers in N-CDs (responsible for their emission) rather than the nuclear states (mainly cause absorption). The recently reported doped CDs from crayfish shell,186,187 milk powder,200 fish scales,231 and fish scale collagen peptides234 also showed excellent photo-stability. The photo-stability of animal/human biomass-derived CDs was also demonstrated for different period of times in air134,150,172,174,183,247 and even after storage for many days/months/year under ambient conditions.126,136,184,185,193,204,205,208,212,221,224,235,237,239,247 The doped CDs obtained from spider silk,142 milk,189 pork,210 and pork liver213 showed insignificant changes in PL intensity even after long-term storage at 4 °C. Noticeably, the N-CDs derived from silkworm chrysalis,140 grilled fish,150 and pork210 maintained their fluorescence properties even after drying, which is vital for invisible ink application.
Meanwhile, the fluorescence intensity of doped CDs synthesized from different animal/human sources such as Bombyx mori silk,141 bee pollen,167,172 chicken cartilage,217 and human hair243 was barely affected by temperature, while doped CDs derived from cow milk,198 expired milk,202 pork liver,213 chicken bone,218 and cow manure219 showed temperature-dependent PL behavior. For example, the decreasing trend of PL intensity with an increase in temperature (27–77 °C) for cow milk-derived N-CDs was attributed to the thermal activation of non-radiative trapping sites, which resulted in an increase in non-radiative relaxation at elevated temperatures.198
The fluorescence intensities of the CDs derived from many animal/human biomass were very stable in a broad pH range. Moreover, a linear dependence was also observed in some animal-derived doped CDs,127,139,180 which is crucial for pH sensor application. The pH-dependent PL features of doped CDs derived from various animal/human sources such as egg white,133 Bombyx mori silk,138 cocoon silk,139 spider silk,142 roasted duck,156 bee pollen,172 prawn shell,174 crayfish shell,185–187 mussel seafood,188 cow milk,191,199 hen feathers,207 pig skin collagen,211 pigeon manure,221 fish scales,223,227 carp roe,233 human hair,239,240 human urine,245 and human fingernails247 were often explained by the protonation–deprotonation of the functional groups present on their surface. The protonation–deprotonation mechanism of doped CDs under different pH conditions were also endorsed by the changes in their zeta potential.138,140,207,239 For example, Zn/Mg-doped CDs (derived from hen feathers) showed a positive shift in zeta potential (−34.5/−36.0 mV to −0.145/−1.67 mV) when the basic pH changed to acidic due to the protonation of available surface sites. Consequently, the PL intensity dropped in acidic pH, which is ascribed to the photo-induced electron transfer (PET) and interaction of the lone pair electrons in the different N and metal-doped sites.207
A variety of solvents also had a significant effect on the fluorescence behavior (intensity and/or λem) of animal/human biomass-synthesized CDs due to their different degrees of solubility/dispersibility.126,129,132,133,138,167,172,198,201,239,240 In this case, the dielectric constant, refractive index, and coordination ability of the solvent may influence the fluorescence property of CDs. For example, polar protic solvents such as water, methanol, and ethanol resulted in high PL intensities in comparison to polar aprotic solvents (acetonitrile and acetone) for pork rib bone-synthesized doped CDs due to the H-bonding interaction.212 Additionally, animal/human biomass-derived CDs exhibited stable PL features in different solvents,141,166 which may be vital for their implementation in complex systems. Meanwhile, most animal/human biomass-derived CDs were not influenced much by salt solutions of different ionic strengths, indicating non-aggregative and salt tolerance nature.
6.2.3 Fluorescence lifetime (τ). Time-resolved or pulsed-laser excitation PL measurement is performed to assess the nanosecond τ and origin of fluorescence in fluorescent nanoparticles (NPs), where τ is the characteristic time required by a number of excited fluorophores to come back to a lower state via the radiative loss of energy.250 A short average τ (τav) of animal/human biomass-derived CDs suggests that their luminescence mechanism is based on the radiative recombination of electron–hole pairs (excitons)138,151,152,177,189,191,193,201,239 or singlet state emission.144 Interestingly, the N-CDs extracted from pike eel at various roasting temperatures (160 °C, 200 °C, 230 °C, 260 °C, and 300 °C) showed a continuous increase in τav (5.64, 6.13, 6.89, 7.02, and 7.17 ns), and correspondingly QY (12.86%, 31.35%, 42.10%, 50.70%, and 80.16%), which is ascribed to the formation of stable excited states at a higher temperature, respectively.151 A similar temperature effect on τav and QY was also noticed for N-CDs derived from chicken,158 pork,164 and mussel seafood.188 Furthermore, it was observed that the decay curves of animal/human biosource-derived CDs exactly fitted either mono-exponential or bi-/tri-exponential decay kinetics (Table S1†). Multiple τ means the involvement of different energy levels, defect states, and surface fluorophores, resulting in diverse recombination states of excitons.130,158,207,211,225,242 For example, the three components of τ in the case of human hair-derived OCDs/MCDs are attributed to the radiative transitions from three different emissive centers, i.e., σ*/π* → n (τ1: associated with the surface functional groups), π* → π (τ2: due to the carbogenic core), and π* → π via mid-gap states (τ3: originated from the functional groups and defects). Interestingly, the third component (τ3) is prolonged for OCDs (11.33 ns) in comparison to MCDs (8.36 ns), suggesting that OCDs had deeper trap states.242
7 Applications of animal/human biomass-derived CDs
Due to the unique properties of animal/human biomass-derived CDs such as small size, abundant surface functionality, low toxicity, aqueous solubility/dispersibility, and biocompatibility, they may function as excellent nanoprobes for sensing and biological applications.
7.1 Sensor application
When foreign species/analytes (metal ions, biomolecules, drugs, etc.) come in contact with CDs, they preferably interact with their surface functional groups, resulting in either a reduction/enhancement in their emission intensity (light-down/light-up) or shift in their PL peak or change in their absorption behavior. Therefore, it is desirable for the optical signal of CDs to be altered after the addition of the analyte to construct an efficient sensor probe. In this case, “turn on–off” of the fluorescence signal is the most common approach (Fig. 12a); however, the “turn on–off–on” approach (Fig. 12b) is also employed to design sensor platforms using animal/human biomass-derived CDs (Table 2). Moreover, the involvement of several quenching mechanisms in the sensing process such as static quenching effect (SQE), dynamic quenching effect (DQE), fluorescence resonance energy transfer (FRET), IFE, electron transfer (ET), aggregate-induced quenching (AIQ), coordination/interaction-induced quenching (CIQ/IIQ), and PET is also revealed in Table 2.
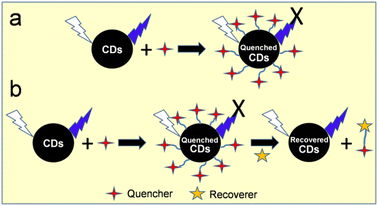 |
| Fig. 12 A schematic illustration of the fluorescence sensing strategy: (a) turn on–off and (b) turn-on–off–on. | |
Table 2 A summary of sensing parameters and mechanism of animal/human biomass-derived CDs for various analytesa
Source |
Sensing process/mechanism |
Linear range (μM) |
LOD (μM) |
Analyte |
Ref. |
aParts per million, bμg mL−1, and capplicability for real sample analysis. |
Metal ions sensor |
Egg shell membrane |
Turn on–off–on, ET |
—, 0.5–80 |
—, 0.48 |
Cu2+, GSH |
127 |
Prawn shell |
Turn on–off, CIQ, IFE |
0.01–1.1 |
0.005 |
cCu2+ |
174 |
Human fingernail |
Turn on–off, CIQ |
0–1.0 |
0.001 |
Cu2+ |
249 |
Milk powder + methionine |
Turn on–off–on, FRET, IFE, DQE |
0.01–55, 5.02–883 |
0.003, 1.48 |
cCu2+, crisedronate sodium |
200 |
Honey |
Turn on–off, AIQ |
0.005–100 |
0.0017 |
cFe3+ |
166 |
Milk |
Turn on–off, CIQ, ET |
10–150 |
— |
cFe3+ |
193 |
Goose feather |
Turn on–off, CIQ, SQE, DQE |
2–7 |
0.196 |
cFe3+ |
205 |
Egg white |
Turn on–off, IIQ |
50–250 |
— |
Fe3+ |
129 |
Egg white |
Turn on–off, ET |
0–25 |
0.54 |
Fe3+ |
132 |
Sheep wool |
Turn on–off, CIQ, ET |
0.1–10.0 |
0.01 |
Fe3+ |
235 |
Bombyx mori silk + CA |
Turn on–off, AIQ |
0.5–4.0 |
0.38 |
Fe3+ |
141 |
Expired milk |
Turn on–off, CIQ |
500–1400 |
— |
Fe3+ |
202 |
Fish scale (crucian carp) |
Turn on–off, CIQ, ET, SQE |
1–78 |
0.54 |
cFe3+ |
225 |
Fish scale (Ctenopharyngodon idella) |
Turn on–off, IIQ |
0–6.25 |
0.144 |
cFe3+ |
227 |
Bee pollen (water)/(ethanol) |
Turn on–off, CIQ, ET, DQE |
50–100/20–100 |
27.0/15.0 |
Fe3+ |
172 |
Cow milk |
Turn on–off, CIQ, ET |
0.1–20, 20–100 |
0.6 |
cFe3+ |
199 |
Pig skin |
Turn on–off, IFE, SQE, DQE |
1–100 |
0.68 |
Co2+ |
208 |
Hair |
Turn on–off, CIQ, SQE, DQE |
0–75 |
0.01 |
cHg2+ |
241 |
Pigeon egg white/yolk |
Turn on–off, CIQ, IFE, ET |
0.05–1.2/0–1.6 |
0.0346/0.0349 |
Hg2+ |
134 |
Urine + citron juice |
Turn on–off–on, ET, DQE |
0–240, 1–10 |
0.15, 0.04 |
cHg2+, cCysteine |
245 |
Spider silk |
Turn on–off, CIQ, SQE |
0–0.08 |
0.0053 |
cHg2+ |
142 |
Fish scale (grass carp) |
Turn on–off, CIQ, ET |
0–30 |
0.014 |
cHg2+ |
224 |
Pigeon manure |
Turn on–off, CIQ |
12–120 |
18.5 |
cHg2+ |
221 |
Carbonized hen feather + Zn salt |
Turn on–off, ET, AIQ, DQE |
0–6a, 8–20a |
— |
Hg2+ |
207 |
Carbonized hen feather + Mg salt |
Turn on–off, ET, AIQ, DQE, SQE |
0–6a, 12–20a |
′′ |
′′ |
Sheep wool/pig hair |
Turn on–off, CIQ, ET |
0.05–100/same |
0.0168/0.0296 |
cCr6+ |
237 |
Fingernail |
Turn on–off, IFE, SQE |
0.0017–0.0675 |
0.0003 |
cCr6+ |
248 |
Denatured milk |
Turn on–off, IFE |
5–100 |
14.0 |
cCr6+ |
203 |
Shrimp shell |
Turn on–off, IFE, ET |
0–70 |
0.1 |
cCr6+ |
183 |
Denatured sour milk |
Turn on–off, IFE, SQE |
10–150 |
0.95 |
cAu3+ |
204 |
Crab shell |
Turn on–off, IIQ |
50–250 |
— |
Cd2+ |
181 |
Cow milk |
Turn on–off, CIQ, SQE, DQE |
0–50 |
17 |
Sn2+ |
198 |
Urine-unmodified diet |
Turn on-off, CIQ |
0–50, 0–30 |
2.7, 3.4 |
Hg2+, Cu2+ |
244 |
Urine-asparagus rich diet |
′′ |
′′ |
2.7, 2.9 |
′′ |
Urine-vitamin C supplemented |
′′ |
′′ |
1.8, 1.7 |
′′ |
Pigeon feather |
Turn on–off, CIQ, IFE, ET |
0–0.12, 0–1.6 |
0.0103, 0.0609 |
Hg2+, Fe3+ |
134 |
Bovine/pork/sheep bones |
Turn on–off, CIQ, SQE |
— |
0.04–4.0 |
cAg+, cCu2+, cHg2+, cPb2+, cFe3+ |
215 |
Wool keratin |
Turn on–off, CIQ, IFE, SQE |
2.5–50 |
0.01416 |
cCr6+ |
238 |
Turn on–off, CIQ, DQE |
0.25–125 |
0.113 |
Fe3+ |
![[thin space (1/6-em)]](https://www.rsc.org/images/entities/char_2009.gif) |
Other analytes sensor |
Fish scale (grass carp) |
Turn on–off, PET |
0–1000 |
— |
ClO− |
222 |
Lamb |
Turn on–off |
0–100 |
— |
H2O2 |
152 |
′′ |
10–300 |
2.9 |
cGlucose |
Cow manure |
PL enhancement, coordination |
105–106 |
— |
Glucose |
220 |
Chicken cartilage |
Turn on–off, DQE |
2–500, 500–1500 |
0.47 |
H2O2 |
217 |
Turn on–off |
5–1000 |
1.22 |
cGlucose |
Pork |
Turn on–off, CIQ, AIQ, SQE |
0.1–100, 100–500 |
0.05 |
cUA |
210 |
Milk powder + FeCl3 |
Turn on–off–on, IFE, IFE inhibition |
20–500 |
5.13 |
cAA |
196 |
Mussel seafood |
Turn on–off, FRET |
1–50 |
0.00606 |
cRiboflavin |
188 |
Prawn shell |
Turn on–off, Redox reaction, DQE |
0–1000 |
1.0 |
cNO2− |
175 |
Sheep wool |
Turn on–off–on, IFE, IFE inhibition |
0.148–14.8 |
0.071 |
cGlyphosate |
236 |
Pork rib bone |
Turn on–off–on, SQE, DQE, FRET |
0.15–5.0 |
0.064 |
cDimethoate |
212 |
Crayfish shell |
Turn on–off–on, oxidation, DQE, oxidation inhibition |
10–300 |
2.30 |
cPCP |
186 |
Egg white |
Turn on–off, IIQ, FRET |
0.78–50 |
0.04 |
cCurcumin |
131 |
Fish scale (grass carp) |
Turn on–off, CIQ, SQE |
185–1295 |
54.0 |
cLH |
223 |
Crab shell |
Turn on–off, IIQ, SQE |
0.02–1.0 |
0.009 |
cCFX |
180 |
Chicken drumstick |
Turn on–off, IFE |
0.0009–0.014 |
0.00044 |
cCFX |
214 |
Milk |
Turn on–off, IFE |
2–200 |
0.6 |
cTCs |
195 |
Carp roe |
Turn on–off, IFE, SQE |
0.1–50 |
0.0417 |
cTCs |
233 |
Pork liver |
Turn on–off, CIQ, IFE, SQE |
1–200 |
0.75 |
c6-TG |
213 |
GGEC |
Turn on–off, IFE, SQE |
0.267–100 |
0.267 |
cMNZ |
216 |
′′ |
0.067–80 |
0.067 |
cTNZ |
′′ |
0.133–200 |
0.133 |
cONZ |
′′ |
0.067–200 |
0.067 |
cSNZ |
Crayfish shell |
Turn on–off, CIQ, SQE |
0.14–0.84 |
0.077 |
cTAP |
185 |
Hair |
Turn on–off, IFE, SQE |
0.613–306.6 |
0.205 |
cCLZ |
243 |
Fingernail |
Turn on–off, IFE, SQE |
0.0003–0.0434 |
0.0001 |
cSY |
247 |
Egg white |
PL enhancement, PET |
0.609–6.09 |
0.092 |
cRK |
133 |
Hair |
PL enhancement, coordination |
0.0001–0.05a |
0.003a |
CHCl3 |
242 |
Crayfish shell |
Turn on–off, CIQ, SQE |
0–50 |
0.16 |
c4-NP |
187 |
Carbonized hen feather + Zn salt |
Turn on–off, IFE |
0–20a |
— |
4-NP |
207 |
Carbonized hen feather + Mg salt |
′′ |
′′ |
— |
′′ |
Chicken bone |
PL enhancement, interaction |
0–10b |
0.17b |
cLiby |
218 |
7.1.1 Metal ion sensor.
7.1.1.1 Copper ion (Cu2+) sensor. The fluorescence quenching of egg shell membrane-synthesized doped CDs in the presence of Cu2+ and restoration of their fluorescence signal after the addition of glutathione (GSH) were probably the first report where a metal ion (Cu2+) quenched the fluorescence signal of animal/human biomass-derived CDs.127 Subsequently, prawn shell-174 and human fingernail-249derived CDs were employed for the selective detection of Cu2+ with an appreciable limit of detection (LOD) (Table 2). Recently, the fluorescence signal of N,S-CDs (milk powder-derived; λem = 470 nm) was quenched by Cu2+/o-phenylenediamine through FRET and IFE due to the formation of 2,3-diaminophenazine (oxidized product of o-phenylenediamine, λem = 557 nm) (Fig. 13a), which resulted in the sensitive detection of Cu2+ in the linear range of 0.01–55 μM (Fig. 13b) and LOD as low as 3.0 nM. Moreover, the sensing platform could recognize risedronate sodium (bisphosphonate-containing drug) in a wider linear range of 5.02–883 μM with the LOD of 1.48 μM by the recovery of its fluorescence signal due to the chelation of the drug molecules with Cu2+ and inhibition of the oxidized product. The sensing platform was also found to be applicable for the detection of Cu2+ and risedronate sodium in real water and biological fluids.200
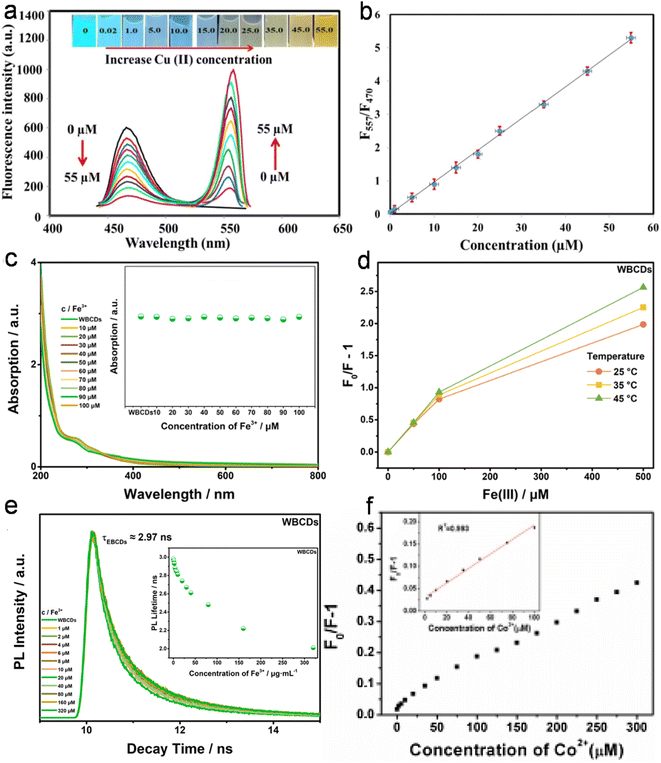 |
| Fig. 13 (a) Variation in the emission intensity of the sensor assembly (N,S-CDs and o-phenylenediamine) with different concentrations of Cu2+ (inset: the digital photograph of sensor system under UV-light after applying Cu2+ in different concentrations) and (b) the corresponding linear plot of 557/470 nm emission intensity (F557/F470) with respect to Cu2+ concentration. Reprinted from ref. 200, Copyright (2023), with permission from Elsevier. (c) The UV-vis absorption spectra (inset: variation in absorption intensity), (d) temperature-dependent Stern–Volmer curves, and (e) PL decay profile (inset: variation in the measured lifetime) of bee pollen-derived WBCDs with different concentrations of Fe3+. Reprinted from ref. 172, Copyright (2022), with permission from Elsevier. (f) The non-linear plot of (F0/F − 1) by applying different concentrations of Co2+ on pig skin-derived N-CDs (inset: linearity in the Co2+ concentration range of 1–100 μM). Reprinted from ref. 208, Copyright (2016), with permission from Elsevier. | |
7.1.1.2 Ferric ion (Fe3+) sensor. Fe3+ is the most common metal ion, which has been detected by animal/human biomass-derived CDs due to its strong affinity with their surface functional groups. Earlier reports showed the Fe3+ detection ability of CDs derived from honey,166 milk,193 goose feathers,205 egg white,129,132 sheep wool,235 Bombyx mori silk,141 expired milk,202 and fish scales,225,227 involving various quenching mechanisms and different LODs in the range of 1.7–540 nM (Table 2). Subsequently, bee pollen-derived N-CDs (WBCDs and EBCDs) were implemented for the rapid, sensitive, and selective detection of Fe3+ via fluorescence quenching, which was attributed to the transfer of electrons from the excited state of N-CDs to the vacant Fe3+ d-orbital through a non-radiative process. The involvement of DQE in the quenching process was verified by the following characteristics: (i) no changes in the absorption peak and intensity with different Fe3+ concentrations because the collision between N-CDs and Fe3+ occurs in the excited state (Fig. 13c), (ii) increase in quenching constant (measured from Stern–Volmer plot) with an increase in temperature (Fig. 13d), and (iii) decrease in τav after the addition of Fe3+ in comparison to the bare N-CDs (Fig. 13e).172 Recently, cow milk-derived N-CDs were also applied for the sensitive and selective detection of Fe3+ via a non-radiative ET process with a satisfactory LOD (0.6 μM).199
7.1.1.3 Cobalt ion (Co2+) sensor. Although cobalt is an essential trace element in biological systems, its excessive intake may have adverse health effects. N-CDs (derived from pig skin) were used for the selective/sensitive detection of Co2+ (linear range: 1–100 μM and LOD: 0.68 μM). The quenching of their fluorescence signal was due to both SQE and DQE because the plot of (F0/F − 1) vs. Co2+ concentration (1–300 μM) did not show a linear fitting in the entire concentration range (Fig. 13f). Moreover, the IFE-based fluorescence quenching was justified by the precise overlap of the Co2+ absorption band (400–550 nm) with the excitation/emission bands of N-CDs (460/521 nm; fluorophore).208
7.1.1.4 Mercury ion (Hg2+) sensor. The detection of Hg2+ is vital due to its serious toxic effect in living systems. Doped CDs derived from human hair,241 pigeon white/yolk,134 human urine,245 spider silk,142 and fish scales224 have been applied for the selective/sensitive detection of Hg2+ due to their strong affinity with attached functional groups. Interestingly, the LOD of Hg2+ with spider silk-derived N-CDs was very low (5.3 nM) and the sensor probe could also be applied in the real water samples.142 Subsequently, pigeon manure-derived N-CDs were also used for the detection of Hg2+ via CIQ.221 Recently, hen feathers were utilized to develop a Zn/Mg-doped CD-based sensor platform. It was observed that the fluorescence quenching of Mg-CDs (70%) was higher than that of Zn-CDs (52%) in the presence of 20 ppm Hg2+, which is primarily governed by DQE. Moreover, the significant involvement of SQE in the higher linear range (12–20 ppm) resulted in the better quenching response of Mg-CDs.207
7.1.1.5 Chromium ion (Cr6+) sensor. The development of sensor probes for Cr6+ is important due to its severe toxicity in humans and the ecosystem. In this case, doped CDs derived from sheep wool/pig hair,237 human fingernails,248 denatured milk,203 and shrimp shells183 showed potential to detect Cr6+. The quenching of the fluorescence signal in these CDs occurred either via ET or IFE (Table 2). Moreover, these sensing platforms were also applied for the detection of Cr6+-spiked real water samples with good recovery, accuracy, and sensitivity. Noticeably, the fingernail-derived N,S-CDs showed Cr6+ sensitivity in a wider linear range (1.7–67.5 nM) with the lowest LOD (0.3 nM).248
7.1.1.6 Gold ion (Au3+) sensor. Sharma et al.204 used N-CDs (denatured sour milk derived) with AA for the selective/sensitive detection of Au3+ (linear range: 10–150 μM and LOD: 0.95 μM). Mechanistic investigations showed the synergistic effect of IFE and SQE in the fluorescence quenching of N-CDs/AA with Au3+, which is initiated by the formation of AuNPs via the AA-mediated reduction of Au3+. Besides real field water sample analyses with satisfactory recoveries and error limits, N-CD-coated paper was used for the visual detection of AA-containing Au3+ solutions by the varying degrees of color response under UV-light illumination.
7.1.1.7 Cadmium ion (Cd2+) sensor. Among the harmful heavy metal ions, Cd2+ is widely spreading in the environment due to the chemical industry and human activities. In this case, crab shell-derived CDs have been used for the selective detection of Cd2+. Here, the fluorescence quenching ability of Cd2+ in the concentration range of 50–250 μM is promoted by strong interaction between Cd2+ and CDs.181
7.1.1.8 Tin ion (Sn2+) sensor. The sensitive and selective detection of Sn2+ is crucial because of its adverse effect in the human digestive and respiratory systems. Kumar et al.198 applied cow milk-derived N-CDs for the selective and sensitive detection of Sn2+ with a good LOD (17 μM). Here, the strong interaction between Sn2+ and N-CDs induced the formation of a complex, which resulted in fluorescence quenching via non-radiative recombination. Moreover, the involvement of SQE and DQE is based on the non-linear fitting of F/F0 in the entire concentration range of Sn2+ (0–900 μM).
7.1.1.9 Multi-metal ion sensor. Besides single metal ions, multiple metal ions have also detected using animal/human biomass-derived CDs. For example, CDs derived from human urine244 and pigeon feathers134 were successfully employed for the selective detection of Hg2+/Cu2+ and Hg2+/Fe3+ metal ions, respectively. Fu et al.215 developed hierarchical cluster and linear discriminant analysis methods to distinguish five heavy metal ions (Ag+, Hg2+, Pb2+, Cu2+, and Fe3+) and their binary/ternary mixture with 100% accuracy using animal bone-derived-doped CDs. Subsequently, the fluorescence intensity of wool keratin-derived N,S-CDs was quenched by Cr6+ and Fe3+. The coordination-based fluorescence quenching of N,S-CDs was ascribed to the co-existence of IFE and SQE for Cr6+ and DQE in the case of Fe3+. The detection of Cr6+ in a simulated electroplating wastewater solution (a mixture of Cu2+, Ni2+, Zn2+, and Cr6+) was also achieved by this sensor probe. Moreover, the N,S-CD-loaded hydrogel could visually sense both Cr6+/Fe3+ at a lower concentration upon exposure to UV-light (Fig. 14).238
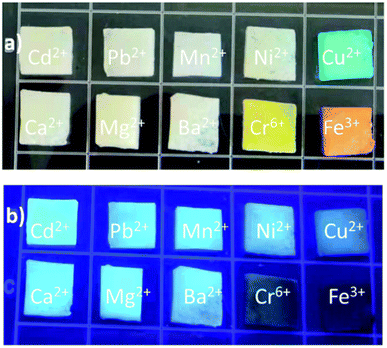 |
| Fig. 14 The photographs of wool keratin-derived N,S-CD-hydrogel incubated in different metal ion solutions under (a) day light and (b) UV irradiation. Reproduced/adapted from ref. 238 with permission from The Royal Society of Chemistry, 2022. | |
7.1.2 Other analyte sensor.
7.1.2.1 Reactive oxygen species (ROS) and biomolecule sensor. ROS refer to a wide variety of oxidants such as hypochlorite (ClO−) and H2O2, which are essential for different physiological functions.251 However, the detection of these species is paramount to combat their hazardous effect. Moreover, the detection of various biomolecules such as glucose, uric acid (UA), AA (vitamin C), and riboflavin (vitamin B2) is also vital for living beings. Fish scale-derived N-CDs have been employed for the selective detection of ClO− in the presence of other common metal ions through the fluorescence quenching process (PET from the N elements of doped CDs to ClO−).222 Heteroatom-doped CDs derived from baked lamb,152 cow manure,220 and chicken cartilage217 were applied for the sensitive/selective detection of glucose. Interestingly, the PL intensity of cow manure-derived PBA-CDs increased linearly with an increase in glucose concentration (0.1–1.0 M, light-up sensor), which was ascribed to the binding effect of glucose with the electron-donor groups of boronic acid (functional groups present on the surface of PBA-CDs).220 Besides, the N-CDs (chicken cartilage derived)-based biosensor platforms (CDs/Fe2+ and CDs/Fe2+/glucose/glucose oxidase (GOx)) showed the sensitive and selective determination of H2O2 and glucose with a wider linear range and a lower LOD compared to the previously reported baked lamb-derived N-CDs (Table 2).152,217 The produced H2O2 by the catalytic conversion of glucose (in the presence of GOx) and formation of ˙OH (via Fenton reaction) functioned as a bridge to detect glucose via DQE. Also, was proposed that the highly reactive ˙OH may partially destroy the surface functional groups and conjugated structures of N-CDs, resulting in the quenching phenomenon.152,217 N-CDs synthesized from pork were employed for the effective and selective sensing of UA (based on the combined effect of aggregation and the formation of a ground-state complex via electrostatic and H-bonding interactions).210 Fe-CDs (milk powder derived) were used for the colorimetric/fluorometric detection of AA. The oxidized product of TMB (oxTMB produced with Fe-CDs; TMB = 3,3′,5,′-tetramethylbenzidine) effectively quenched the fluorescence signal of Fe-CDs via IFE, and the fluorescence further restored in the presence of AA due to the inhibition of oxTMB. Consequently, the characteristic absorption of oxTMB (∼652 nm) was reduced with the evolution of the Fe-CDs emission (∼460 nm) for the dual-signal detection of AA with a colorimetric/fluorometric LOD as low as 8.59/5.13 μM.196 Subsequently, a biosensor was developed based on mussel seafood-derived N-CDs for the detection of riboflavin (through FRET) with an LOD as low as 6.06 nM.188
7.1.2.2 Anion sensor. Prawn shell-derived N-CDs were used for the fluorometric detection of nitrite ion (NO2−) in water. The fluorescence quenching-based sensor system could detect NO2− with an LOD as low as 1.0 μM, which is much lower than the maximum permissible limit set by the World Health Organization (WHO) in drinking water (65 μM). The DQE-based quenching occurred due to the redox reaction between light-induced excited oxidation states of N-CDs and analyte. However, I− is recognized as a potential interfering anion in the selectivity of NO2−.175
7.1.2.3 Herbicide and pesticide sensor. The consumption of glyphosate as an environmentally safe herbicide is very common in agricultural and non-agricultural cultivation due to its low toxicity.252 However, its excessive use may lead to high levels of accumulation in the soil and agricultural products, resulting in adverse health effects in human and animals.253,254 Alternatively, dimethoate and pentachlorophenol (PCP) are widely used organophosphate and organochlorine pesticides, respectively, which accumulate in the ecosystem (soil, water, and agriculture products) and cause adverse toxicological impacts in humans and other living beings.255,256 In this case, a CD/AgNP-based fluorometric platform was developed using N,S-CDs (sheep wool derived) for the highly sensitive and selective determination of glyphosate. The fluorescence quenching of CDs by AgNPs (turn-off), and its subsequent recovery (turn-on) in the presence of glyphosate are due to the IFE and IFE inhibition, respectively.236 Liu et al.212 constructed a turn on–off–on-based fluorescent probe for the sensitive and selective detection of dimethoate. The dithizone-induced fluorescence quenching of doped CDs (pork rib bone derived) was attributed to the coexistence of SQE (formation of ground-state complex between dithizone and Ca element present in the CDs, as evidenced by the change in UV-vis spectrum, Fig. 15a), DQE (change in τav in the absence and presence of dithizone, Fig. 15b and c, respectively), and FRET effect. Furthermore, the fluorescence intensity was gradually restored after the addition of dimethoate due to the displacement of dithizone (bound at the surface of CDs) via its preferential bonding with the hydrolyzed product of dimethoate, which resulted in the inhibition of the quenching process (Fig. 15d). Recently, a turn on–off–on fluorescence sensor was developed using crawfish shell-derived N,S-CDs for the sensitive and selective assessment of PCP. The sensing strategy was based on DQE-type fluorescence quenching in the presence of horseradish peroxidase/H2O2 (the generated ˙OH oxidized the functional groups in N,S-CDs) and its subsequent recovery by the addition of PCP (inhibition of oxidation) with a satisfactory LOD (2.3 μM). Moreover, the sensing platform was suitable for the detection of PCP in spiked fresh crawfish, aquaculture water, and lake water samples with recoveries in the range of 95.62–105.17% and relative standard deviation (RSD) in the range of 1.20–4.50%.186
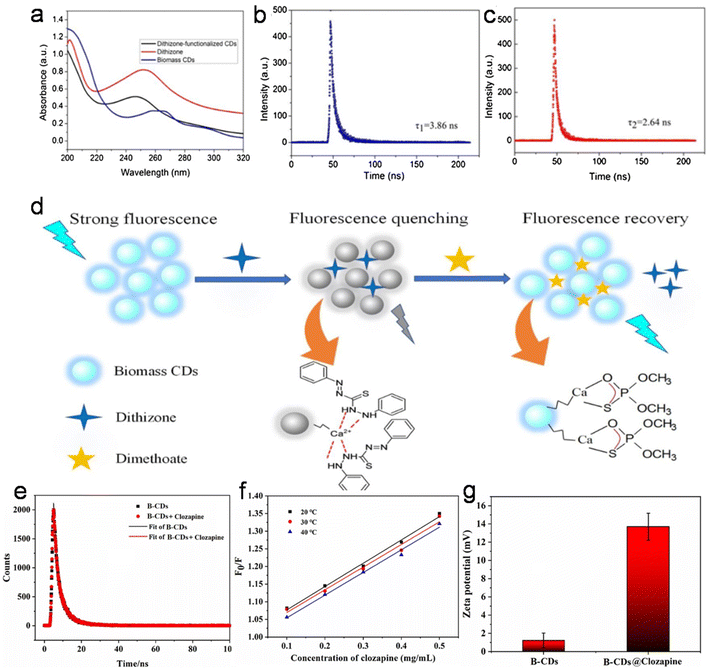 |
| Fig. 15 (a) The UV-vis spectra of pork rib bone-derived doped CDs, dithizone, and doped CDs-dithizone complex, (b and c) the fluorescence decay curves of doped CDs and doped CDs-dithizone complex, and (d) a schematic of dimethoate detection via the fluorescence turn-on–off–on mechanism. Reprinted from ref. 212, Copyright (2020), with permission from Elsevier. (e) The fluorescence decay profile, (f) temperature-dependent Stern–Volmer plots, and (g) zeta potentials of human hair synthesized N-CDs and N-CD-CLZ adduct. Reprinted from ref. 243, Copyright (2023), with permission from Elsevier. | |
7.1.2.4 Drug molecule sensor. Animal/human biomass-derived doped CDs have been successfully applied to detect various drug molecules such as curcumin,131 lidocaine hydrochloride (C14H22N2O·HCl, LH),223 ceftriaxone (CFX),180,214 tetracyclines (TCs),195,233 6-thioguanine (6-TG),213 nitroimidazoles,216 thiamphenicol (TAP),185 and clozapine (CLZ)243 via the turn on–off-based quenching process (Table 2). Noticeably, the LOD of CFX drug with chicken drumstick-derived N-CDs (0.44 nM)214 was much improved compared to the previously reported crab shell-derived N-CDs (9.0 nM).180 Moreover, the significant improvement in the LOD for the IFE-based detection of TCs with carp roe-derived N-CDs (41.7 nM)233 rather than milk-derived N,P-CDs (600 nM)195 is also remarkable. Besides, the sensitive and selective detection of 6-TG with N-CDs (pork liver derived) showed temperature-, pH-, and response time-dependent fluorescence quenching.213 Among the various nitroimidazole drugs, the LOD of tinidazole (TNZ)/secnidazole (SNZ) was found to be superior to metronidazole (MNZ)/ornidazole (ONZ) using GGEC-derived N-CDs (Table 2).216 Subsequently, the N,S-CDs derived from crayfish shell were employed for the sensitive detection of TAP (linear range: 50–300 μg L−1 and LOD: 27.5 μg L−1 or 0.077 μM).185 Recently, carbonized human hair-derived N-CDs were used as an IFE/SQE-based fluorescence quenching probe for the sensitive detection of CLZ with an LOD as low as 67 ng mL−1 (0.205 μM), which is far below than the minimum effective amount in the blood (0.35 μg mL−1). The insignificant change in τav (Fig. 15e) and decrease in the quenching constant with an increase in temperature (calculated from Stern–Volmer plot, Fig. 15f) endorsed that the quenching was based on SQE. Moreover, the significant increase in the zeta potential (1.229 to 13.7 mV) after the quenching process justified the coordination ability of CLZ with the functional groups attached to the surface of N-CDs (Fig. 15g). The sensing platform also exhibited satisfactory recoveries of CLZ in actual tablet (103.26%) and blood samples (92.92–102.45%).243
7.1.2.5 Food/beverage colorant or flavoring agent sensor. Sunset yellow (SY) and raspberry ketone (RK) are a widely used food/beverage colorant and flavoring agent, respectively, which can have adverse health impacts upon excessive consumption.257,258 In an earlier report, human fingernail-synthesized N,S-CDs were explored as a fluorescence quenching nanoprobe (IFE and SQE based) for the sensitive detection of SY (LOD: 0.1 nM).247 Recently, N-CDs (egg white derived) were executed for the wider (0.609–6.09 μM) and low LOD (0.092 μM) detection of RK. Interestingly, N-CDs showed an enhancement in their absorbance and emission spectra after incubation with RK, which was ascribed to the PET between the N-CD functionalities and RK via radiative recombination. Moreover, the sensor was also validated for the detection of RK in dietary supplement capsules.133
7.1.2.6 Organic molecule sensor. The strong oxidizing ability of HClO and ClO− towards organic matter during the chlorine disinfection process leads to the generation of various trihalomethanes, including chloroform (CHCl3), as major by-products, which are potentially toxic to humans and aquatic organisms.259 Singh et al.242 showed the CHCl3 sensing capability of human hair-derived N-CDs with a low LOD of 3.0 ppb (parts per billion), which is well below the WHO guideline for drinking water (300 ppb). The CHCl3 molecules bound to N-CDs behaved like a passivating agent to prevent interactions between the solvent molecule (water) and electron density of the exposed pyridinic N–O groups to develop a PL enhancement-based sensor platform. Recently, crayfish shell-187 and hen feather207-derived doped CDs were employed to assemble a sensor platform for the detection of another hazardous organic molecule, namely, 4-nitrophenol (4-NP). A wider linear range of 0–20 ppm and more than 90% PL quenching of both Zn/Mg-doped CDs using 20 ppm 4-NP in acidic condition (pH = 2) clearly justified their efficiency to detect toxic pollutants in an aqueous environment.207
7.1.2.7 Laundry powder sensor. Laundry powder is a commonly used household item, which contains phosphate and surfactant as major aquatic pollutants.260 Recently, a fluorescence enhancement-based sensor system was developed using chicken bone-derived CDs for the detection of laundry powder (Liby) with an appreciable LOD (0.17 μg mL−1). The recovery rates (85.2–106%) and RSDs (1.01–5.3%) of the sensor in spiked water samples (mineral/tap/river water) showed its applicability in real water systems.218
7.2 Bioimaging application
Compared to metal-based QDs, fluorescent CDs are found to be more suitable for bio-applications due to their small size, high water dispersibility/solubility, low cytotoxicity, superior cell viability, high photo-stability, and good bio-degradability. Multimodal bioimaging through CDs is an effective approach for diagnosis and cell labeling. It is known that CDs may have critical effects on living cells and model organisms.261–263 Therefore, before applying CDs in various bio-environments, it is necessary to understand their in vitro and/or in vivo cytotoxicity as well as bio-distribution and cellular uptake for safety purpose. In this section, the toxic evaluation, bio-distribution, cell uptake, and bioimaging applications of animal/human biomass-derived CDs are summarized.
7.2.1 In vitro/in vivo cytotoxicity. Many animal/human source-derived CDs exhibit excellent biocompatibility and low cytotoxicity to various investigated cell lines using either the standard MTT (3-(4,5-dimethylthiazolyl-2)-2,5-diphenyltetrazolium bromide) assay or other cell counting protocols (Table S2†). Horo et al.147 evaluated the in vitro cytotoxicity of CDs synthesized from SF, their biotin conjugate (BT-CDs), and 5-fluorouracil-loaded BT-CDs (FU-BT-CDs) through cancerous (human breast cancer (MCF-7) and human cervical cancer (HeLa)) and normal (human embryonic kidney cells (HEK-293)) cell lines. The results showed the higher cytotoxicity of FU-BT-CDs in the cancer cell lines compared to the normal cell line, which signified the positive/negative degree of biotin expression in the cancer/normal cell lines. Furthermore, the large value of IC50 in HEK-293 (138.1 μM) compared to MCF-7 (28.19 μM) and HeLa (38.17 μM) suggested the low cytotoxicity of FU-BT-CDs in the normal cell line for better target specificity because of biotin conjugation. It was observed that EWCDs (egg white derived) could be easily excreted through zebrafish metabolism and showed ∼80% cell viability in the BEAS-2B (human bronchial epithelial) cell line.132 The cytotoxicity evaluation of two types of N-CDs (WBCDs and EBCDs, derived from bee pollen) exhibited the toxic effects of WBCDs to HeLa, HCT-116 (human colorectal carcinoma cells), and HepG2 (human hepatocellular liver carcinoma cells), but no obvious toxicity to the SHSY5Y (human neuroblastoma cells) and RAW264.7 (mouse monocyte/macrophage cells) cell lines. Furthermore, the EBCDs showed slight toxicity to all five cells.172 Besides, severe in vitro cytotoxicity was observed for cow milk-derived CDs on mouse hippocampus cells (HT22, cell viability <60%) even at the lowest concentration of 0.4 mg mL−1.197The toxicity study of hamburger-, lamb-, chicken-, and chicken breast-derived doped CDs exhibited the more cytotoxic effect of CDs obtained at a higher temperature in comparison to that synthesized at a relatively lower temperature (Table S2†) due to their surface functional groups and large amount of carbon core via effective carbonization.149,153,158,159 Interestingly, the lamb-derived N,S-CDs obtained at a longer baking time (CD-3: 45 min and cell viability: 45.0%) had much higher cytotoxicity than that from the shorter baking times (CD-1: 15 min, cell viability: 95.0% and CD-2: 30 min, cell viability: 74.0%), which is again related to the degree of carbonization and surface passivation. Moreover, the adverse effects of CD-3 were also judged by the perturbation of energy, amino acid, purine, and lipid metabolism.154 Thus, the results suggest that a lower processing temperature/time for animal-based food stuff is favorable to human health.
Dose-dependent cytotoxicity has also been observed in some animal/human biomass-derived CDs.154,177,201,208,220,243–245 For example, the low cytotoxicity of cow manure-derived PBA-CDs at a concentration of 0.01 mg mL−1 to B16F10 (murine melanoma cells) and NIH3T3 (fibroblast cells) cells was explained based on the surface-confined negative charges present in the boronic groups. However, the PBA-CDs showed significant cytotoxicity at a higher concentration (0.1 mg mL−1, cell viability: 60–70%).220
Besides in vitro cytotoxicity, in vivo biocompatibility has also been demonstrated in animal/human biomass-derived CDs. For example, N-CDs (derived from human hair/pig skin)209 and FA@Gd-CDs (FA-conjugated Gd-CDs derived from crab shell)178 exhibited no adverse effect on phenotypic development, survival rate, and hatching rate in zebrafish embryos. Similarly, an oral/injected dose of doped/modified CDs derived from SF,143 pork,164 milk,195 and cow manure220 showed an insignificant toxicological effect on BALB/c mice. In vivo cytotoxic assessment of the doped CDs (shark cartilage derived) also showed good tolerance to zebrafish larvae even up to the maximum concentration of 0.1 mg mL−1.232
7.2.2 In vitro/in vivo bioimaging. Bombyx mori silk-derived N-CDs were the first example of animal/human biomass showing in vitro cellular uptake in A549 (human lung adenocarcinoma epithelial cells) cancerous cell nuclei.138 The possibilities of in vitro/in vivo bioimaging and cellular uptake via the endocytosis process using CDs derived from animal/human biomass are summarized in Table 3, which reveals that the internalization of CDs preferably occurs in the cytoplasm or cell interior/membrane instead of the nucleus. As evidenced by the intense blue fluorescence localized in the cytoplasm under UV irradiation, FA-conjugated N-CDs (derived from crab shell) were selectively targeted to the folate receptor over-expressing cancerous HeLa cells in comparison to the normal HEL (human erythroleukemia) cells.179 Two types of human urine-derived doped CDs (UCDs and HUCDs) were used to assess their in vitro distributions in the cytoplasm of HeLa and onion epidermal cells. Additionally, the in vivo imaging showed the location of UCDs/HUCDs in the pharynx, intestine, and anus regions of Caenorhabditis elegans (C. elegans) organism and interior of bean sprout plant tissue. Interestingly, in all the fluorescent labelling experiments, HUCDs exhibited brighter fluorescence than UCDs despite their large size, which is ascribed to their higher QY.246 The successful internalization of CDs (Fig. 16a) and BT-CDs (Fig. 16b) in MCF-7 cancer cells was evidenced by their bright blue fluorescence. It was observed that the CDs were only localized in the cytoplasm (Fig. 16a), while BT-CDs were distributed in the entire cell (Fig. 16b) – preferably in the nucleus due to receptor (BT)-mediated endocytosis. Moreover, the cellular uptake of BT-CDs was more specific towards cancer cells (MCF-7) in comparison to the normal HEK-293 cells due to the positive BT expression.147
Table 3 In vitro and in vivo cell imaging results of the animal/human biomass-derived CDsa,b
Source |
Mode |
Time (h) |
Cell/plant/organism (amount of CDs in mg mL−1) |
Uptake region/emission color |
Ref. |
aMinutes, bdays, ccells, dbacteria, eorganism, fmg kg−1 of mouse, gplant, hμM, and iintracellular bioimaging of metal ions/other analytes. Bcap-37: human breast cancer cells, S. aureus: Staphylococcus aureus, KB: epithelial carcinoma cells, MC3T3-E1: mouse osteoblast cells, PC12: rat pheochromocytoma cells, NRK: normal rat kidney epithelial cells, HEp-2: human larynx carcinoma cells, LoVo: human colon carcinoma cells, B. parachinensis: Brassica parachinensis, SK-Hep-1: human hepatoma cell lines, SH-SY5Y: human neuroblastoma cells, U87: human brain glioma tumor cells, SMMC-7721: human liver cancer cells, U-251 MG: human malignant glioblastoma cell lines, HUVEC: human umbilical vein endothelial cells, MDA-MB-231: human breast cancer cells, Caco-2: human colorectal adenocarcinoma cells, DU-145: human prostate cancer cells, KG-1a: promyeloblast macrophage cells, hMSCs: human mesenchymal stem cells, and MEF: mouse embryonic fibroblast cells. |
Egg white |
In vitro |
0.5 |
HeLac (0.04) |
Cytosol/green |
129 |
Egg white (albumin) |
In vitro |
24 |
Bcap-37c (2.0) |
Cytoplasm/blue, green |
130 |
Egg white |
In vitro |
02a |
E. colid, S. aureusd (0.1) |
Entire bacteria/blue, green, red |
131 |
Egg white |
In vivo |
24 |
Zebrafishe (2.0) |
Entire embryo/blue, green, red |
132i |
Bombyx mori silk |
In vitro |
24 |
A549c (1.0) |
Nucleus/blue, green, red |
138 |
Silkworm chrysalis |
In vitro |
06 |
HeLac (6.0) |
Cell interior/blue, green, red |
140 |
Spider silk |
In vitro |
24 |
HepG2c (0.5) |
Cell interior/blue |
142i |
Silk fibroin |
In vitro |
04 |
KBc (0.1) |
Cytoplasm, nucleus/green |
143 |
In vivo |
18 |
BALB/c Micee (50f) |
Liver, lung/green |
Silk fibroin + LMWC |
In vitro |
24 |
MCF-7c (0.1) |
Cytoplasm (CDs)/blue; entire cell, nucleus (BT-CDs)/blue |
147 |
Hamburger |
In vitro |
24 |
MC3T3-E1c (0.5) |
Cytoplasm/blue, green, red |
149 |
In vivo |
05b |
Mung bean sproutg (3.2) |
Root, stem, cotyledon, vascular system/blue |
Pike eel fish |
In vitro |
24 |
MC3T3-E1c (1.5) |
Cell membrane, cytoplasm, nucleus/blue, green, red |
150 |
Pike eel fish |
In vitro |
24 |
MC3T3-E1c (1.5) |
Cytoplasm/blue, green, red |
151 |
Lamb |
In vitro |
07 |
HepG2c (1.0) |
Cell membrane, cytoplasm/blue, green, red |
153 |
Duck |
In vitro |
24 |
PC12c (1.0) |
Cytoplasm/blue |
156 |
In vivo |
24 |
C. eleganse (15.0) |
Intestine/blue |
Chicken |
In vitro |
24 |
HepG2c (1.0) |
Cytoplasm/blue |
158 |
Ex vivo |
02 |
BALB/c Micee (2000f) |
Brain/blue |
Chicken breast |
In vitro |
12 |
NRK |
Lysosome |
159 |
Chicken |
Ex vivo |
2–6 |
BALB/c Micee (2000f) |
Stomach, intestine, testis, kidney |
160 |
Pork |
In vivo |
0.5–6 |
BALB/c Micee (2000f) |
Kidney, heart, brain, lung, intestine, testis, liver, stomach |
164 |
′′ |
24 |
C. eleganse (10.0) |
Epithelium, digestive system/blue, green, red |
Honey |
In vitro |
01 |
HEp-2c, HeLac (1.0) |
Cell interior/green |
166 |
Rapeseed bee pollen |
In vitro |
12 |
LoVoc (0.5) |
Cytoplasm/blue, green, red |
167 |
Bee pollen |
In vivo |
20b |
B. parachinensis L.g (3.5) |
Periplasmic space/blue |
168 |
Rapeseed bee pollen |
In vitro |
02 |
Lettuceg (1.0) |
Leaf vein, stem, root/blue |
169 |
Bee pollen |
In vitro |
15a |
HepG2c (0.1) |
Cytoplasm/blue |
172 |
Shrimp egg |
In vitro |
24 |
SK-Hep-1c (0.2) |
Cell membrane, cytoplasm/blue, green, red |
173 |
Dried shrimp |
In vitro |
3/48 |
MCF-7c, SH-SY5Yc (0.1) |
Cytoplasm/blue, green, red |
177 |
Crab shell + GdCl3 |
In vitro |
24 |
HeLac, HepG2c (0.5) |
Cytoplasm/blue |
178 |
Crab shell |
In vitro |
24 |
HeLac (0.5) |
Cytoplasm/blue |
179 |
Shrimp shell |
In vivo |
— |
Nematodee (—) |
Entire body/green |
184 |
Crayfish shell |
In vitro |
24 |
HeLac (0.55) |
Cytoplasm/red |
185i |
Mussel seafood |
In vitro |
24 |
HepG2c (1.5) |
Cytoplasm/blue |
188 |
In vitro |
10a |
Onion epidermal cellg (1.5) |
Cell wall/blue |
In vivo |
48 |
Zebrafishe (1.5) |
Liver, yolk, intestine/blue |
Milk |
In vitro |
02 |
U87c (0.01) |
Cell interior/blue |
189 |
Milk |
In vitro |
01 |
SMMC-7721c (50h) |
Cell interior/blue, green |
190 |
Milk |
In vitro |
— |
HeLac (—) |
Cell membrane, cytoplasm/blue, green, red |
193 |
In vivo |
— |
Tumour bearing BALB/c Micee (0.5) |
Tumour tissue |
Milk |
In vitro |
02 |
HeLac (0.2) |
Cytoplasm/green |
194 |
Pasteurized milk |
In vitro |
02 |
HeLac (0.01) |
Lysosome/blue (CDs); Cytoplasm/blue (Lis-CDs) |
201 |
Expired milk |
In vitro |
06 |
HeLac (0.2) |
Cytoplasm/green |
202 |
Denatured sour milk |
In vitro |
72 |
U-251 MGc (0.05) |
Cell membrane, cytoplasm/blue |
204 |
Pigeon feather |
In vitro |
01 |
HUVECc (2.0) |
Cell membrane, cytoplasm/blue |
134i |
Pig skin |
In vitro |
0.5 |
HeLac (0.35) |
Cell interior/blue, green, red |
208i |
Pig skin |
In vivo |
20 |
Zebrafishe (0.2–1.6) |
Entire embryo/blue, green, red |
209i |
Pig skin collagen |
In vitro |
24 |
RL-14c (0.5) |
Cytoplasm/bright |
211 |
GGEC |
In vitro |
04 |
Melanomac (1.0) |
Cytoplasm/blue |
216 |
Cow manure |
In vitro |
0.5 |
MCF-7c, HUVECc, MDA-MB-231c, Caco-2c, DU-145c (2.5) |
Cytoplasm/green (unmodified CDs); Nucleolus/green (modified CDs) |
219 |
Cow manure |
In vitro |
— |
MCF-7c (—) |
Mitochondria/green, red |
220 |
In vivo |
2–48 |
C57BL/6 Micee (0.16–0.32) |
Entire body |
Fish scale (crucian carp) |
In vitro |
24 |
KG-1ac (0.125) |
Cell interior/blue |
225i |
Fish scale (Lethrinus lentjan) |
In vitro |
06 |
hMSCsc (0.025) |
Cytoplasm, nucleus/blue, green, red |
226 |
Shark cartilage (chondroitin sulphate) |
In vivo |
24 |
Zebrafishe (0.1) |
Intestine/blue, green |
232 |
Sheep wool |
In vitro |
0.5 |
HeLac (0.3) |
Cytoplasm, nucleus/blue, green |
235i |
Hair |
In vitro |
04 |
HeLac (0.2) |
Cell membrane, cytoplasm/blue |
239 |
Hair |
In vivo |
20 |
Zebrafishe (0.2–0.8) |
Entire embryo/blue, green, red |
209i |
Urine |
In vitro |
10a |
MEFc (0.2) |
Cytoplasm/green, red |
244 |
Urine |
In vitro |
04 |
HeLac (1.0) |
Cytoplasm/blue, green |
246 |
In vivo |
24 |
C. eleganse (20) |
Pharynx, intestine, anus/blue |
In vitro |
06 |
Onion epidermalg (3.0) |
Cytoplasm/blue |
In vivo |
24 |
Bean sproutsg (20) |
Tissue interior/blue |
Fingernail |
In vitro |
02 |
A549c, MDA-MB-231c, HeLac (0.2) |
Cytoplasm/blue |
247 |
Fingernail |
In vitro |
02 |
A549c, HeLac, MDA-MB-231c, HEK-293c (0.2) |
Perinuclear region/blue |
248 |
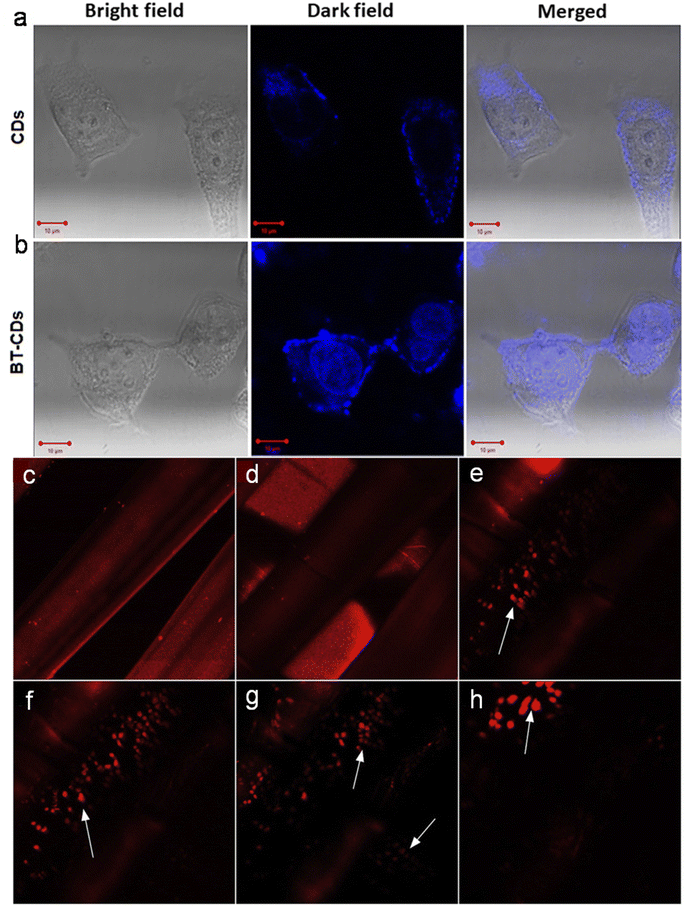 |
| Fig. 16 Confocal microscopy images showing the uptake of (a) CDs and (b) BT-CDs in MCF-7 cell lines as evidenced by bright blue fluorescence. Reprinted from ref. 147, Copyright (2021), with permission from Elsevier. The 3D-printed scaffold imaging of cell nuclei at variable depths: (c) outer layer, (d) z = 600 μm, (e) z = 800 μm, (f) z = 900 μm, (g) z = 1000 μm, and (h) z = 1500 μm. Reprinted from ref. 211, Copyright (2018), with permission from Elsevier. | |
Distinct distributions of animal-derived CDs in living cells via two-photon excited fluorescence imaging (with longer wavelength femtosecond laser, 800/780 nm) were also noticed without obvious auto-fluorescence, which is advantageous for cell imaging without photon-induced damage.194,202,211 For example, Dehghani et al.211 performed two-photon imaging of RL-14 (human fetal ventricular cardiomyocytes) cells under a 780 nm pulse laser to confirm the uptake of N-CDs (pig skin collagen derived) inside the cytoplasm. Moreover, the 3D luminescent scaffolds with a tailored design could visualize the cell structures at various depths (z = 0 to 1500 μm) using two-photon microscopy (Fig. 16c–h).
Zebrafish was used as a model organism to investigate the transport/distribution of animal-derived CDs via in vivo imaging (Table 3).132,188,209,232 For example, the in vivo imaging of EWCDs (derived from egg white) confirmed their internalization in zebrafish embryo via chorion as evidenced by the characteristic blue/green/red emission of 2 hpf (1 hpf = 24 h post-fertilization) zebrafish embryo and 5 dpf (1 dpf = 1 day post-fertilization) zebrafish larvae (Fig. 17a).132 N-CDs (derived from mussels) were localized outside the cell walls/cytoplasm when applied for in vitro bioimaging in onion epidermal/HepG2 cells, while in vivo blue fluorescent monitoring showed their presence in the liver, yolk, and intestine of zebrafish larva.188
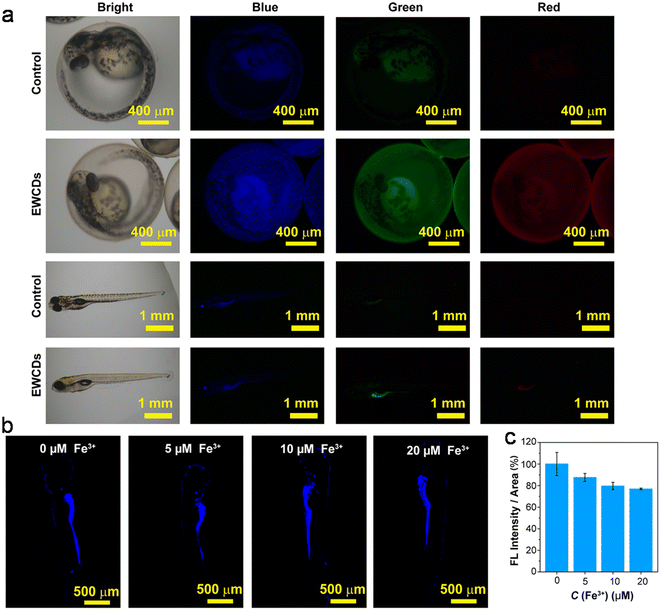 |
| Fig. 17 (a) The bright-field and fluorescent field (blue, green, and red) imaging of zebrafish treated with egg white-derived EWCDs, (b) zebrafish larva incubated with EWCDs and different concentrations of Fe3+, and (c) average fluorescence statistics from confocal laser scanning microscopy images. Reprinted (adapted) with permission from ref. 132, Copyright (2021), the American Chemical Society. | |
Animal-derived doped/modified CDs were also supplemented in mice to access their fluorescence imaging/spectral contrast-based distributions (Table 3).143,158,160,164,193,220 For example, the ex vivo oral administration of N-CDs (chicken derived) in BALB/c mice suggested their maximum appearance in the digestive system including stomach and intestine (2 h), followed by testis and kidney (2–6 h), while a minimal effect on the lung, liver, heart, and spleen (Fig. 18a). The subsequent reduction of the internalized N-CDs in the digestive system after 20 h metabolic process could be seen by the change in fluorescence intensity (Fig. 18a and b). Moreover, the in vitro studies demonstrated several adverse effects of N-CDs on the digestibility of SPI (soy protein isolate), digestion product (polypeptides and free amino acids), and pepsin activity, which suggested their unfavorable interaction with digestive enzymes.160
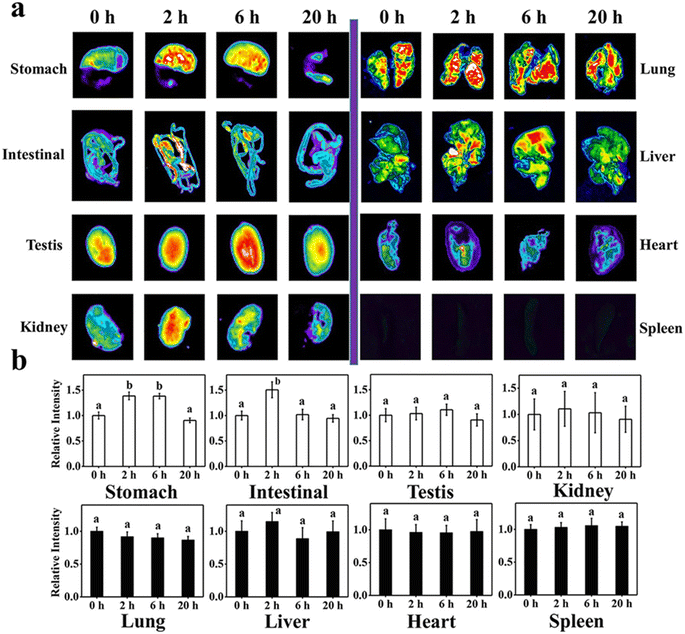 |
| Fig. 18 (a) Fluorescence images acquired from the different major organs of BALB/c mice immediately after the oral supplementation of chicken-derived N-CDs and after 2, 6, and 20 h. (b) Plots of relative fluorescence intensity of imaged organs with corresponding time (a and b letters in (b) represent the major difference in the relative intensity at P < 0.05). Reprinted from ref. 160, Copyright (2022), with permission from Elsevier. | |
Besides in vivo/in vitro bioimaging, animal biomass-derived CDs are also consumed for the intracellular sensing of metal ions132,134,142,208,209,225,235 or other analytes (TAP)185 through suppressed fluorescence signaling. For example, the excellent in vitro selectivity of egg white-derived EWCDs with Fe3+ (Table 2) was further implemented for the in vivo detection of Fe3+ in zebrafish. A gradual decrease in surface-based fluorescence intensity with an increase in Fe3+ concentration can be seen in Fig. 17b and c, suggesting the permeation of EWCDs and absorption of Fe3+ in zebrafish embryos.132 Similarly, the intracellular monitoring of TAP in HeLa cells showed a significant decrease in red fluorescence (originated from N,S-CDs) with an increase in TAP concentration.185
7.3 Other applications
The promotion of plant growth using animal biomass-derived CDs is another growing area of interest.168,169,172 For instance, bee pollen-derived EBCDs exhibited better promotion capability on the growth of the classical Arabidopsis plant model in comparison to WBCDs. The high growth-promoting ability of EBCDs was ascribed to their high content of N- and O-heteroatoms, which resulted in greater biocompatibility and bioactivity.172
The antimicrobial activity of animal-derived CDs has also been investigated.171,181,184 For example, crab shell-derived CDs were used as an antibacterial agent against highly noxious human pathogenic bacteria (Escherichia coli (E. coli) and Klebsiella pneumonia). The high inhibition activity of CDs in this study was ascribed to the effective damage of the cell membrane via the generation of ROS on the surface of CDs.181
Animal biomass-derived CDs have also been investigated for therapeutic application.132,220 For example, boronic acid-functionalized PBA-CDs (derived from cow manure) were employed as an immunotherapy agent to treat melanoma skin cancer-bearing mice, which exhibited interaction with tumour tissues and stimulated the immune system against the disease. The histopathological imaging of the cancer-bearing mice after treatment with PBA-CDs exhibited no obvious damage to the brain and kidney tissues, while the liver, spleen, and lung tissues showed inflammatory focus, white pulp hyperplasis, and fibrosis/inflammatory infiltrate, respectively. The histological analyses also revealed necrosis and inflammatory infiltrate on large areas of tumour tissues after PBA-CD treatment.220
The drug delivery applications of doped CDs derived from dried shrimps,177 crab shell,178 and pasteurized milk201 are also appreciable. For instance, magneto-fluorescent Gd-CDs (derived from a mixture of crab shell and GdCl3) showed potential for diagnostic and theranostic application. FA@Gd-CDs specifically targeted HeLa cells (folate receptor-positive) via folate receptor-mediated endocytosis. Moreover, doxorubicin (DOX, anti-cancer drug) conjugated with FA@Gd-CDs (∼74.5% loading) exhibited higher release kinetics at low pH (5.0) in comparison to high pH (7.4), which was ascribed to the weakened DOX binding with CDs due to the protonation of the –NH2 groups present in DOX.178
Animal-derived CDs have also been employed to prepare fluorescent ink for multi-colour coating, patterning, and word writing, which is vital for staining, information encryption, and anti-counterfeiting applications.126,145,166,193,202,204,221,235 Li et al.145 employed silk sericin-derived N-CDs to prepare multimodal quick response/bar security codes and Morse code-based encryption, which could be recognized under UV irradiation and immediately after switching-off of the UV light. Moreover, the N-CD ink coated on different fabrics (silk, cotton, and terylene) was visible once the UV light is turned-off after exposure.
The catalytic and photocatalytic applications of animal/human biomass-derived CDs have also been investigated.148,167,206,228,249 Recently, Din et al.206 demonstrated 92% degradation of methylene blue (MB) by chicken feather-derived CDs during 60 min exposure to sunlight. The catalyst also showed reusability of up to 5 cycles. The photo-generated electrons/holes on the surface of the CDs induced the formation of superoxide anion radical (O2˙−)/˙OH, which eventually decomposed the aromatic structure of the dye molecules.
Athika et al.203 investigated denatured milk-derived CDs for supercapacitor application. The rectangular shape of the cyclic voltammetry curves and almost symmetrical galvanostatic charge-discharge (GCD) profiles implied the existence of double layer capacitance. The specific capacitance was estimated to be 95.0 F g−1 together with 100% columbic efficiency/cyclic stability during 1000 GCD cycles at 0.12 A g−1.
The free radical scavenging activity of animal source-derived CDs is also remarkable.153,161 Mackerel fish-derived N-CDs were utilized for unique free radical (˙OH and ˙CH3) scavenging activity, which showed potential to protect against oxidative stress under biological condition. The scavenging of ˙OH radicals via Fenton reaction resulted in strong fluorescence quenching due to the changes in the energy levels of the N-CDs. The dose-dependent scavenging exhibited 90% disappearance of the ˙OH signal using 15.0 mg mL−1 N-CDs. Additionally, the 41.4% decrease in the reaction rate constant implied the remarkable reduction of the MB (source of ˙CH3 generation by visible-light photosensitization) degradation rate via ˙CH3 scavenging.161
The non-linear optical (NLO) phenomenon refers to a process that non-linearly depends on the excitation light parameters. An aqueous dispersion of N,S-codoped CDs (obtained from a mixture of honey, garlic extract, and NH3) showed a self-defocusing and strong NLO response. The third-order NLO susceptibility was estimated to be significantly high (2.29 × 10−6 esu) together with reverse saturable absorption in the open aperture curve, which indicated the suitability of doped CDs for NLO application.171
8 Challenges and future prospects
Considering the significant utilization of animal/human biomass in the synthesis and potential applications of sustainable CDs, there are several challenges and prospects that require attention in the foreseeable future, as follows:
(1) Exploring novel precursors capable of self-doping and surface passivation, coupled with refined bottom-up synthetic protocols can potentially enhance both the performance and fluorescent QY of CDs.
(2) Attaining precise control of the size distribution, degree of graphitization, and surface functionalization remains crucial for achieving exceptional optical properties in CDs, necessitating further improvement.
(3) The presence of impurities in CDs has the potential to impede the desired performance outcomes. Therefore, developing new, uncomplicated, and cost-effective purification protocols is essential to achieve optimal results.
(4) Although the applications of animal/human biomass-derived CDs have shown promise at the laboratory scale, achieving their commercial-scale production has proven to be a challenge. Thus, comprehensive research is needed to enable the large-scale synthesis of CDs from these readily available precursors while retaining their inherent small-scale properties.
(5) Despite the clear influence of synthetic parameters and starting sources, the actual underlying growth mechanism of these CDs remains unknown, requiring comprehensive understanding through empirical/in situ experimental evidence.
(6) The precise origins of the PL and EWD/EWID features in these CDs still need to be elucidated, making it a pivotal area for in-depth investigation.
(7) In addition to the common down-conversion fluorescence, some animal/human biomass-derived CDs exhibit UCPL (Table S1†), which holds potential for near infrared (NIR) cellular imaging via two-photon luminescence and catalyst fabrication for energy-related applications. Consequently, these application areas offer fertile ground for new research.
(8) The design of CDs with long λem using animal/human biomass remains uncharted. Therefore, dedicated efforts should be devoted to creating CDs with NIR absorption and emission properties, leveraging the low biotoxicity and efficient tissue penetration capabilities of NIR light.
(9) Many sensors for metal ions and their tracking in biological systems using these CDs rely on light-down (fluorescence quenching) mechanisms. However, the development of light-up (fluorescence enhancement, Table 2) phenomena in the presence of analytes can be a valuable advancement, addressing the challenges posed by the high background fluorescence in biological environments.
(10) Opportunities exist to enhance the sensitivity and selectivity of analyte detection and tracking in real samples and biological systems by producing CD nanoprobe sensors using new precursors or modifying chemical composition/structure. Furthermore, there is untapped potential to develop sensing platforms for various toxic metal ions such as Mn2+, Pb2+, As3+, Po3+, and other unexplored analytes using these CDs in the future.
(11) Investigation of the long-term acute toxicity of animal/human-derived CDs on vertebrates and humans is insufficient. Moreover, advancement in the in situ tracking of these CDs may facilitate the in depth understanding and assessment of the metabolic process.
9 Conclusions
This review article presented a comprehensive overview of CDs synthesized from biomass of animal and human origin, aiming to provide researchers with insights for novel experimental implications and advancements in this field. This type of biomass, which is often considered inexpensive or waste materials, offers a sustainable and eco-friendly resource that can be converted into value-added products. The choice of precursor and synthetic method significantly influences the fundamental structure, heteroatom doping, and surface functional groups of the resulting CDs. Consequently, both the CDs and their doped counterparts can benefit from size control, leading to an enhanced QY. Many of these CDs exhibit self-passivation through various functional groups, offering opportunities for tailored modifications catering to specific applications. Notably, the optical properties of these CDs are closely related to their structure and chemical composition. In addition to the common emission known as EWD fluorescence, these CDs display EWID and UCPL behaviours. Their EWD fluorescence properties are primarily influenced by the quantum size effect of the core structure, the presence of surface emission centers arising from the presence of functional groups or defect sites, and the radiative recombination of excitons trapped at the surface. Conversely, their UCPL characteristics stem from a multi-photon activation process. The outstanding PL properties of these CDs combined with their attributes such as photo-stability, low cytotoxicity, and high biocompatibility make them promising candidates for various sensing, bioimaging, and many more applications. Consequently, the utilization of animal and human biomass has potential to emerge as a sustainable and eco-friendly platform for creating fluorescent CDs characterized by excellent optical properties, thereby opening diverse avenues for their application.
Conflicts of interest
There are no conflicts to declare for financial interests or personal relationships.
Acknowledgements
PD thanks to University of Allahabad, Prayagraj, India for infrastructural facility and Science & Engineering Board (SERB), New Delhi, India for financial support through the fast track grant (SB/FT/CS-190/2011). He also thanks to Mr Shishir Singh, IIT Kanpur and Professor S. K. Pandey, Department of Chemistry, BHU to provide unsubscribed contents used in this article.
References
- Q. Lu, J. Deng, Y. Hou, H. Wang, H. Li, Y. Zhang and S. Yao, Chem. Commun., 2015, 51, 7164–7167 RSC.
- L. Li, Y. Li, Y. Ye, R. Guo, A. Wang, G. Zou, H. Hou and X. Ji, ACS Nano, 2021, 15, 6872–6885 CrossRef CAS PubMed.
- L. Li and T. Dong, J. Mater. Chem. C, 2018, 6, 7944–7970 RSC.
- M. Behi, L. Gholami, S. Naficy, S. Palomba and F. Dehghani, Nanoscale Adv., 2022, 4, 353–376 RSC.
- X. Xu, R. Ray and Y. Gu, J. Am. Chem. Soc., 2004, 126, 12736–12737 CrossRef CAS PubMed.
- Y. P. Sun, B. Zhou and Y. Lin, J. Am. Chem. Soc., 2006, 128, 7756–7757 CrossRef CAS PubMed.
- B. Wang, G. I. N. Waterhouse and S. Lu, Trends Chem., 2023, 5, 76–87 CrossRef CAS.
- F. Yan, Y. Jiang, X. Sun, J. Wei, L. Chen and Y. Zhang, Nano Res., 2020, 13, 52–60 CrossRef CAS.
- X. Yang, X. Li, B. Wang, L. Ai, G. Li, B. Yang and S. Lu, Chinese Chem. Lett., 2022, 33, 613–625 CrossRef CAS.
- T. Zhang, X. Wang, Z. Wu, T. Yang, H. Zhao, J. Wang, H. Huang, Y. Liu and Z. Kang, Nanoscale Adv., 2021, 3, 6949–6955 RSC.
- A. Terracina, A. Armano, M. Meloni, A. Panniello, G. Minervini, A. Madonia, M. Cannas, M. Striccoli, L. Malfatti and F. Messina, ACS Appl. Mater. Interfaces, 2022, 14, 36038–36051 CrossRef CAS PubMed.
- P. Zhao and L. Zhu, Chem. Commun., 2018, 54, 5401–5406 RSC.
- L. Tong, X. Wang, Z. Chen, Y. Liang, Y. Yang, W. Gao, Z. Liu and B. Tang, Anal. Chem., 2020, 92, 6430–6436 CrossRef CAS PubMed.
- J. D. Stachowska, A. Murphy, C. Mellor, D. Fernandes, E. N. Gibbons, M. J. Krysmann, A. Kelarakis, E. Burgaz, J. Moore and S. G. Yeates, Sci. Rep., 2021, 11, 10554 CrossRef CAS PubMed.
- M. Perikala, R. Valoor, N. Bhaskar, A. Bhardwaj and B. Basu, ACS Appl. Mater. Interfaces, 2023, 15, 281–291 CrossRef CAS PubMed.
- Q. Tang, W. Zhu, B. He and P. Yang, ACS Nano, 2017, 11, 1540–1547 CrossRef CAS PubMed.
- Q. Guan, R. Su, M. Zhang, R. Zhang, W. Li, D. Wang, M. Xu, L. Fei and Q. Xu, New J. Chem., 2019, 43, 3050–3058 RSC.
- C. Ji, Y. Zhou, R. M. Leblanc and Z. Peng, ACS Sens., 2020, 5, 2724–2741 CrossRef CAS PubMed.
- C. Lu, Q. Su and X. Yang, Nanoscale, 2019, 11, 16036–16042 RSC.
- I. A. Baragau, N. P. Power, D. J. Morgan, R. A. Lobo, C. S. Roberts, M. M. Titirici, V. Middelkoop, A. Diaz, S. Dunn and S. Kellici, ACS Sustainable Chem. Eng., 2021, 9, 2559–2569 CrossRef CAS.
- Z. Ye, G. Li, J. Lei, M. Liu, Y. Jin and B. Li, ACS Appl. Bio Mater., 2020, 3, 7095–7102 CrossRef CAS PubMed.
- Z. Zhang, G. Yi, P. Li, X. Zhang, H. Fan, Y. Zhang, X. Wang and C. Zhang, Nanoscale, 2020, 12, 13899–13906 RSC.
- T. Lee, S. Won, Y. Park and W. Kwon, ACS Appl. Nano Mater., 2021, 4, 2462–2469 CrossRef CAS.
- Y. Han, Y. Liu, H. Zhao, A. Vomiero and R. Li, J. Mater. Chem. B, 2021, 9, 4111–4119 RSC.
- S. D. Hettiarachchi, E. K. Cilingir, H. Maklouf, E. S. Seven, S. Paudyal, S. Vanni, R. M. Graham and R. M. Leblanc, Nanoscale, 2021, 13, 5507–5518 RSC.
- H. Zhang, S. Li, L. Xu, R. Momen, W. Deng, J. Hu, G. Zou, H. Hou and X. Ji, Adv. Energy Mater., 2022, 12, 2200665 CrossRef CAS.
- L. Jiang, H. Cai, W. Zhou, Z. Li, L. Zhang and H. Bi, Adv. Mater., 2023, 35, 2210776 CrossRef CAS PubMed.
- A. R. Nallayagari, E. Sgreccia, R. Pizzoferrato, M. Cabibbo, S. Kaciulis, E. Bolli, L. Pasquini, P. Knauth and M. L. D. Vona, J. Nanostructure Chem., 2022, 12, 565–580 CrossRef CAS.
- Z. Wei, W. Lu, X. Wang, J. Ni, U. H. Prova, C. Wang and G. Huang, J. Mater. Chem. C, 2022, 10, 1932–1967 RSC.
- V. Sharma, P. Tiwari and S. M. Mobin, J. Mater. Chem. B, 2017, 5, 8904–8924 RSC.
- R. Das, R. Bandyopadhyay and P. Pramanik, Mater. Today Chem., 2018, 8, 96–109 CrossRef CAS.
- S. Bhandari, D. Mondal, S. K. Nataraj and R. G. Balakrishna, Nanoscale Adv., 2019, 1, 913–936 RSC.
- W. Meng, X. Bai, B. Wang, Z. Liu, S. Lu and B. Yang, Energy Environ. Mater., 2019, 2, 172–192 CrossRef CAS.
- H. Fan, M. Zhang, B. Bhandari and C. H. Yang, Trends Food Sci. Technol., 2020, 95, 86–96 CrossRef CAS.
- Y. Wang, J. Sun, B. He and M. Feng, Green Chem. Eng., 2020, 1, 94–108 CrossRef.
- C. Kang, Y. Huang, H. Yang, X. F. Yan and Z. P. Chen, Nanomaterials, 2020, 10, 2316 CrossRef CAS PubMed.
- A. Sekar, R. Yadav and N. Basavaraj, New J. Chem., 2021, 45, 2326–2360 RSC.
- M. Kurian and A. Paul, Carbon Trends, 2021, 3, 100032 CrossRef CAS.
- N. K. K. Anuar, H. L. Tan, Y. P. Lim, M. S. Soaib and N. F. A. Bakar, Front. Energy Res., 2021, 9, 626549 CrossRef.
- L. J. Desmond, A. N. Phan and P. Gentile, Environ. Sci.: Nano, 2021, 8, 848–862 RSC.
- S. Perumal, R. Atchudan, T. N. J. I. Edison and Y. R. Lee, J. Environ. Chem. Eng., 2021, 9, 105802 CrossRef CAS.
- T. C. Wareing, P. Gentile and A. N. Phan, ACS Nano, 2021, 15, 15471–15501 CrossRef CAS PubMed.
- S. Chahal, J. R. Macairan, N. Yousefi, N. Tufenkji and R. Naccache, RSC Adv., 2021, 11, 25354–25363 RSC.
- Y. Lou, X. Hao, L. Liao, K. Zhang, S. Chen, Z. Li, J. Ou, A. Qin and Z. Li, Nano Sel., 2021, 2, 1117–1145 CrossRef CAS.
- M. H. Chan, B. G. Chen, L. T. Ngo, W. T. Huang, C. H. Li, R. S. Liu and M. Hsiao, Pharmaceutics, 2021, 13, 1874 CrossRef CAS PubMed.
- H. S. Shahraki, A. Ahmad and R. Bushra, FlatChem, 2022, 31, 100310 CrossRef.
- V. Ahuja, A. K. Bhatt, S. Varjani, K. Y. Choi, S. H. Kim, Y. H. Yang and S. K. Bhatia, Chemosphere, 2022, 293, 133564 CrossRef CAS PubMed.
- P. Kaur and G. Verma, Mater. Today Sustain., 2022, 18, 100137 CrossRef.
- V. Manikandan and N. Y. Lee, Environ. Res., 2022, 212, 113283 CrossRef CAS PubMed.
- S. Xiang and M. Tan, Environ. Sci.: Nano, 2022, 9, 3206–3225 RSC.
- N. Ullal, K. Muthamma and D. Sunil, Chem. Papers, 2022, 76, 6097–6127 CrossRef CAS.
- J. Fan, L. Kang, X. Cheng, D. Liu and S. Zhang, Nanomaterials, 2022, 12, 4473 CrossRef CAS PubMed.
- Q. Xu, F. Xiao and H. Xu, Critical Rev. Food Sci. Nutrition, 2023, 1–18 Search PubMed.
- P. Singh, Arpita, S. Kumar, P. Kumar, N. Kataria, V. Bhankar, K. Kumar, R. Kumar, C. T. Hsieh and K. S. Khoo, Nanoscale, 2023, 15, 16241–16267 RSC.
- M. Fang, B. Wang, X. Qu, S. Li, J. Huang, J. Li, S. Lu and N. Zhou, Chinese Chem. Lett., 2024, 35, 108423 CrossRef CAS.
- F. J. Chao-Mujica, L. Garcia-Hernández, S. Camacho-López, M. Camacho-López, M. A. Camacho-López, D. R. Contreras, A. Pérez-Rodríguez, J. P. Peña-Caravaca, A. Páez-Rodríguez, J. G. Darias-Gonzalez, L. Hernandez-Tabares, O. A. de Fuentes, E. Prokhorov, N. Torres-Figueredo, E. Reguera and L. F. Desdin-García, J. Appl. Phys., 2021, 129, 163301 CrossRef CAS.
- C. Doñate-Buendia, R. Torres-Mendieta, A. Pyatenko, E. Falomir, M. Fernández-Alonso and G. Mínguez-Vega, ACS Omega, 2018, 3, 2735–2742 CrossRef PubMed.
- A. Kaczmarek, J. Hoffman, J. Morgiel, T. Moscicki, L. Stobinski, Z. Szymanski and A. Małolepszy, Materials, 2021, 14, 729 CrossRef CAS PubMed.
- D. Rocco, V. G. Moldoveanu, M. Feroci, M. Bortolami and F. Vetica, ChemElectroChem, 2023, 10, e202201104 CrossRef CAS PubMed.
- J. Zhou, C. Booker, R. Li, X. Zhou, T. K. Sham, X. Sun and Z. Ding, J. Am. Chem. Soc., 2007, 129, 744–745 CrossRef CAS PubMed.
- Q. Zhao, X. Wang, Q. Song, Z. Zang, C. Fan, L. Li, X. Yu, Z. Lu and X. Zhang, J. Mater. Chem. C, 2023, 11, 14439–14447 RSC.
- R. Kumar, V. B. Kumar and A. Gedanken, Ultrason. Sonochem., 2020, 64, 105009 CrossRef CAS PubMed.
- A. Ventrella, A. Camisasca, A. Fontana and S. Giordani, RSC Adv., 2020, 10, 36404–36412 RSC.
- J. Xu, K. Cui, T. Gong, J. Zhang, Z. Zhai, L. Hou, F. Zaman and C. Yuan, Nanomaterials, 2022, 12, 312 CrossRef CAS PubMed.
- T. Xing, J. Sunarso, W. Yang, Y. Yin, A. M. Glushenkov, L. H. Li, P. C. Howlett and Y. Chen, Nanoscale, 2013, 5, 7970–7976 RSC.
- Y. Han, Y. Chen, N. Wang and Z. He, Mater. Technol., 2019, 34, 336–342 CrossRef CAS.
- G. Jeong, C. H. Park, D. Yi and H. Yang, J. Cleaner Prod., 2023, 392, 136250 CrossRef CAS.
- C. He, X. Q. Li, G. L. Feng and W. J. Long, Green Chem., 2022, 24, 5842–5855 RSC.
- M. Saikia, A. Singh, A. Dihingia, P. Khare, J. Kalita and B. K. Saikia, Chem. Eng. J., 2022, 433, 133633 CrossRef CAS.
- S. Liu, Y. He, Y. Liu, S. Wang, Y. Jian, B. Li and C. Xu, Chem. Commun., 2021, 57, 3680–3683 RSC.
- T. Ghosh, R. Sahoo, S. K. Ghosh, P. Banerji and N. C. Das, Front. Chem. Sci. Eng., 2023, 17, 536–547 CrossRef CAS.
- N. K. Quang, N. N. Hieu, V. V. Q. Bao, V. T. Phuoc, L. X. D. Ngoc, L. Q. Doc, N. M. Tri, L. V. T. Son, L. V. T. Son and C. T. C. Ha, New Carbon Mater., 2022, 37, 595–602 CrossRef CAS.
- J. Hou, X. Gao, G. Li, H. Liu, Q. Chen, J. Sun and G. Yang, New J. Chem., 2023, 47, 17649–17656 RSC.
- G. Dong, K. Lang, H. Ouyang, W. Zhang, L. Bai, S. Chen, Z. Zhang, Y. Gao, Z. Mu and X. Zhao, RSC Adv., 2020, 10, 33483–33489 RSC.
- Y. Hao, L. Yu, T. Li, L. Chen, X. Han and F. Chai, Spectrochim. Acta, Part A, 2023, 285, 121865 CrossRef CAS PubMed.
- T. V. de Medeiros, J. Manioudakis, F. Noun, J. R. Macairan, F. Victoria and R. Naccache, J. Mater. Chem. C, 2019, 7, 7175–7195 RSC.
- V. Bressi, A. M. Balu, D. Iannazzo and C. Espro, Curr. Opin. Green Sustain. Chem., 2023, 40, 100742 CrossRef CAS.
- Q. Li, X. Wu, X. Zhang, W. Su, Y. Tan, P. Fan, J. Liu and S. Yang, Anal. Methods, 2023, 15, 1569–1575 RSC.
- B. K. Korah, C. R. Thara, N. John, B. K. John, S. Mathew and B. Mathew, Food Control, 2023, 147, 109608 CrossRef CAS.
- A. S. K. Zaman, T. L. Tan, Y. A. /P. Chowmasundaram, N. Jamaludin, A. R. Sadrolhosseini, U. Rashid and S. A. Rashid, Opt. Mater., 2021, 112, 110801 CrossRef.
- Z. Bagheri, H. Ehtesabi, M. Rahmandoust, M. M. Ahadian, Z. Hallaji, F. Eskandari and E. Jokar, Sci. Rep., 2017, 7, 11013 CrossRef PubMed.
- M. Chen, C. Liu, J. Zhai, Y. An, Y. Li, Y. Zheng, H. Tian, R. Shi, X. He and X. Lin, RSC Adv., 2022, 12, 18779–18783 RSC.
- M. Picard, S. Thakur, M. Misra and A. K. Mohanty, RSC Adv., 2019, 9, 8628–8637 RSC.
- S. S. Jayan, J. S. Jayan, B. Sneha and K. Abha, Mater. Today: Proc., 2021, 43, 3821–3825 CAS.
- Z. A. Qiao, Y. Wang, Y. Gao, H. Li, T. Dai, Y. Liu and Q. Huo, Chem. Commun., 2010, 46, 8812–8814 RSC.
- B. Luo, H. Yang, B. Zhou, S. M. Ahmed, Y. Zhang, H. Liu, X. Liu, Y. He and S. Xia, ACS Omega, 2020, 5, 5540–5547 CrossRef CAS PubMed.
- T. Ghosh, R. Ghosh, U. Basak, S. Majumder, R. Ball, D. Mandal, A. K. Nandi and D. P. Chatterjee, J. Mater. Chem. A, 2018, 6, 6476–6492 RSC.
- C. Hu, Y. Zhu and X. Zhao, Spectrochim. Acta, Part A, 2021, 250, 119325 CrossRef CAS PubMed.
- S. Sawalha, M. Assali, A. Nasasrah, M. Salman, M. Nasasrah, M. Jitan, H. S. Hilal and A. Zyuod, RSC Adv., 2022, 12, 4490–4500 RSC.
- Z. He, H. Huang, R. Jiang, L. Mao, M. Liu, J. Chen, F. Deng, N. Zhou, X. Zhang and Y. Wei, Mater. Sci. Eng. C, 2020, 108, 110376 CrossRef CAS PubMed.
- M. L. Liu, B. B. Chen, C. M. Li and C. Z. Huang, Green Chem., 2019, 21, 449–471 RSC.
- D. Qu and Z. Sun, Mater. Chem. Front., 2020, 4, 400–420 RSC.
- G. He, M. Shu, Z. Yang, Y. Ma, D. Huang, S. Xu, Y. Wang, N. Hu, Y. Zhang and L. Xu, Appl. Surf. Sci., 2017, 422, 257–265 CrossRef CAS.
- L. Cao, M. Zan, F. Chen, X. Kou, Y. Liu, P. Wang, Q. Mei, Z. Hou, W. F. Dong and L. Li, Carbon, 2022, 194, 42–51 CrossRef CAS.
- S. J. Mohammed, K. M. Omer and F. E. Hawaiz, RSC Adv., 2023, 13, 14340–14349 RSC.
- R. Qiang, S. Yang, K. Hou and J. Wang, New J. Chem., 2019, 43, 10826–10833 RSC.
- C. Shen, C. Dong, L. Cheng, X. Shi and H. Bi, J. Environ. Chem. Eng., 2022, 10, 107020 CrossRef CAS.
- J. Fan, L. Kang, D. Liu and S. Zhang, ChemistrySelect, 2023, 8, e202300062 CrossRef CAS.
- S. Munusamy, T. R. Mandlimath, P. Swetha, A. G. Al-Sehemi, M. Pannipara, S. Koppala, P. Shanmugam, S. Boonyuen, R. Pothu and R. Boddula, Environ. Res., 2023, 231, 116046 CrossRef CAS PubMed.
- K. G. Nguyen, I. A. Baragau, R. Gromicova, A. Nicolaev, S. A. J. Thomson, A. Rennie, N. P. Power, M. T. Sajjad and S. Kellici, Sci. Rep., 2022, 12, 13806 CrossRef CAS PubMed.
- F. Du, Z. Cheng, W. Tan, L. Sun and G. Ruan, Spectrochim. Acta, Part A, 2020, 226, 117602 CrossRef CAS PubMed.
- H. Lu, C. Li, H. Wang, X. Wang and S. Xu, ACS Omega, 2019, 4, 21500–21508 CrossRef CAS PubMed.
- G. Kalaiyarasan, J. Joseph and P. Kumar, ACS Omega, 2020, 5, 22278–22288 CrossRef CAS PubMed.
- H. K. Sadhanala, S. Pagidi and A. Gedanken, J. Mater. Chem. C, 2021, 9, 1632–1640 RSC.
- L. Gu, J. Zhang, G. Yang, Y. Tang, X. Zhang, X. Huang, W. Zhai, E. K. Fodjo and C. Kong, Food Chem., 2022, 376, 131898 CrossRef CAS PubMed.
- X. Wen, G. Wen, W. Li, Z. Zhao, X. Duan, W. Yan, J. F. Trant and Y. Li, Mater. Sci. Eng. C, 2021, 123, 112022 CrossRef CAS PubMed.
- Y. Wang, Y. Zhang, M. Jia, H. Meng, H. Li, Y. Guan and L. Feng, Chem. –Eur. J., 2015, 21, 14843–14850 CrossRef CAS PubMed.
- L. Lin, Y. Luo, P. Tsai, J. Wang and X. Chen, Trends Anal. Chem., 2018, 103, 87–101 CrossRef CAS.
- T. Li, Y. Ning, J. Pang, L. Chen, F. Zhang and F. Chai, New J. Chem., 2023, 47, 147–155 RSC.
- Y. Q. Dang, B. Li, X. Feng, J. Jia, K. Li and Y. Zhang, ChemPhotoChem, 2023, 7, e202200156 CrossRef CAS.
- Q. Zhang, G. Yang, L. Zhang, N. Li, Y. Hou, R. Zhang, W. Wang, X. Du, F. Chen and B. Li, J. Photochem. Photobiol., A, 2024, 446, 115135 CrossRef CAS.
- H. Wang, L. Ai, Z. Song, M. Nie, J. Xiao, G. Li and S. Lu, Chem. –Eur. J., 2023, 29, e202302383 CrossRef CAS PubMed.
- M. Ortega-Munoz, P. Vargas-Navarro, S. Plesselova, M. D. Giron-Gonzalez, G. R. Iglesias, R. Salto-Gonzalez, F. Hernandez-Mateo, A. V. Delgado, F. J. Lopez-Jaramillo and F. Santoyo-Gonzalez, Mater. Chem. Front., 2021, 5, 8151–8160 RSC.
- F. Yuan, Y. K. Wang, G. Sharma, Y. Dong, X. Zheng, P. Li, A. Johnston, G. Bappi, J. Z. Fan, H. Kung, B. Chen, M. I. Saidaminov, K. Singh, O. Voznyy, O. M. Bakr, Z. H. Lu and E. H. Sargent, Nat. Photonics, 2020, 14, 171–176 CrossRef CAS.
- M. Algarra, B. B. Campos, K. Radotic, D. Mutavdozic, T. Bandosz, J. Jimenez-Jimenez, E. Rodriguez-Castellon and J. C. G. Esteves da Silva, J. Mater. Chem. A, 2014, 2, 8342–8351 RSC.
- Z. Ye, R. Tang, H. Wu, B. Wang, M. Tan and J. Yuan, New J. Chem., 2014, 38, 5721–5726 RSC.
- H. Rao, W. Liu, Z. Lu, Y. Wang, H. Ge, P. Zou, X. Wang, H. He, X. Zeng and Y. Wang, Microchim. Acta, 2016, 183, 581–588 CrossRef CAS.
- K. Suzuki, L. Malfatti, M. Takahashi, D. Carboni, F. Messina, Y. Tokudome, M. Takemoto and P. Innocenzi, Sci. Rep., 2017, 7, 5469 CrossRef PubMed.
- M. Zhang, P. He, H. Zhang, S. Dong and T. Huang, Colloids Surf., A, 2023, 676, 132164 CrossRef CAS.
- S. Li, Z. Guo, R. Feng, Y. Zhang, W. Xue and Z. Liu, RSC Adv., 2017, 7, 4975–4982 RSC.
- Y. Jiang, Z. Wang and Z. Dai, ACS Appl. Mater. Interfaces, 2016, 8, 3644–3650 CrossRef CAS PubMed.
- D. Lei, W. Yang, Y. Gong, J. Jing, H. Nie, B. Yu and X. Zhang, Sens. Actuators, B, 2016, 230, 714–720 CrossRef CAS.
- H. Jin, R. Gui, Y. Wang and J. Sun, Talanta, 2017, 169, 141–148 CrossRef CAS PubMed.
- M. Wang, R. Shi, M. Gao, K. Zhang, L. Deng, Q. Fu, L. Wang and D. Gao, Food Chem., 2020, 318, 126506 CrossRef CAS PubMed.
- S. Liu, J. Tian, L. Wang, Y. Zhang, X. Qin, Y. Luo, A. M. Asiri, A. O. Al-Youbi and X. Sun, Adv. Mater., 2012, 24, 2037–2041 CrossRef CAS PubMed.
- J. Wang, C. F. Wang and S. Chen, Angew. Chem., Int. Ed., 2012, 51, 9297–9301 CrossRef CAS PubMed.
- Q. Wang, X. Liu, L. Zhang and Y. Lv, Analyst, 2012, 137, 5392–5397 RSC.
- S. Pramanik, S. Chatterjee, G. S. Kumar and P. S. Devi, Phys. Chem. Chem. Phys., 2018, 20, 20476–20488 RSC.
- Z. Zhang, W. Sun and P. Wu, ACS Sustainable Chem. Eng., 2015, 3, 1412–1418 CrossRef CAS.
- X. Hu, X. An and L. Li, Mater. Sci. Eng. C, 2016, 58, 730–736 CrossRef CAS PubMed.
- M. M. F. Baig and Y. C. Chen, J. Colloid Interface Sci., 2017, 501, 341–349 CrossRef CAS PubMed.
- L. Yu, M. He, S. Liu, X. Dou, L. Li, N. Gu, B. Li, Z. Liu, G. Wang and J. Fan, ACS Appl. Mater. Interfaces, 2021, 13, 54677–54689 CrossRef CAS PubMed.
- S. H. Abdelaal, A. M. El-Kosasy and M. H. Abdelrahman, Chem. Papers, 2023, 77, 3867–3879 CrossRef CAS.
- Q. Ye, F. Yan, Y. Luo, Y. Wang, X. Zhou and L. Chen, Spectrochim. Acta, Part A, 2017, 173, 854–862 CrossRef CAS PubMed.
- Y. Zhao, Y. Zhang, X. Liu, H. Kong, Y. Wang, G. Qin, P. Cao, X. Song, X. Yan, Q. Wang and H. Qu, Sci. Rep., 2017, 7, 4452 CrossRef PubMed.
- S. Wang, H. Wang, R. Zhang, L. Zhao, X. Wu, H. Xie, J. Zhang and H. Sun, J. Alloy Compd., 2018, 746, 567–575 CrossRef CAS.
- X. Su, H. Lin, B. Fu, S. Mei, M. Lin, H. Chen, Z. Zheng, H. Bo, D. P. Yang and Y. Lin, Food Chem., 2023, 405, 134813 CrossRef CAS.
- Z. L. Wu, P. Zhang, M. X. Gao, C. F. Liu, W. Wang, F. Leng and C. Z. Huang, J. Mater. Chem. B, 2013, 1, 2868–2873 RSC.
- J. He, B. Lei, H. Zhang, M. Zheng, H. Dong, J. Zhuang, Y. Xiao and Y. Liu, RSC Adv., 2015, 5, 95744–95749 RSC.
- J. Feng, W. J. Wang, X. Hai, Y. L. Yu and J. H. Wang, J. Mater. Chem. B, 2016, 4, 387–393 RSC.
- H. Liu, Y. Zhang, J. H. Liu, P. Hou, J. Zhou and C. Z. Huang, RSC Adv., 2017, 7, 50584–50590 RSC.
- N. K. Sahoo, S. Das, G. C. Jana, M. N. Aktara, A. Patra, A. Maji, M. Beg, P. K. Jha and M. Hossain, Microchem. J., 2019, 144, 479–488 CrossRef CAS.
- N. R. Ko, M. Nafiujjaman, K. Cherukula, S. J. Lee, S. J. Hong, H. N. Lim, C. H. Park, I. K. Park, Y. K. Lee and I. K. Kwon, Part. Part. Syst. Charact., 2018, 35, 1700300 CrossRef.
- Q. Wang, J. Cai, G. V. Biesold-McGee, J. Huang, Y. H. Ng, H. Sun, J. Wang, Y. Lai and Z. Lin, Nano Energy, 2020, 78, 105313 CrossRef CAS.
- S. Li, H. Wang, H. Lu, X. Liang, H. Wang, M. Zhang, K. Xia, Z. Yin, Y. Zhang, X. Zhang and Y. Zhang, Small, 2021, 17, 2103623 CrossRef CAS PubMed.
- A. B. Padasalagi and M. H. K. Rabinal, Part. Part. Syst. Charact., 2022, 39, 2200041 CrossRef CAS.
- H. Horo, M. Saha, H. Das, B. Mandal and L. M. Kundu, Carbohydr. Polym., 2022, 277, 118862 CrossRef CAS PubMed.
- J. Wang, S. Sahu, S. K. Sonkar, K. N. Tackett II, K. W. Sun, Y. Liu, H. Maimaiti, P. Anilkumar and Y. P. Sun, RSC Adv., 2013, 3, 15604–15607 RSC.
- Y. Li, J. Bi, S. Liu, H. Wang, C. Yu, D. Li, B. W. Zhu and M. Tan, Food Funct., 2017, 8, 2558–2565 RSC.
- J. Bi, Y. Li, H. Wang, Y. Song, S. Cong, D. Li, D. Zhou, B. W. Zhu and M. Tan, New J. Chem., 2017, 41, 8490–8496 RSC.
- J. Bi, Y. Li, H. Wang, Y. Song, S. Cong, C. Yu, B. W. Zhu and M. Tan, J. Agric. Food Chem., 2018, 66, 2862–2869 CrossRef CAS PubMed.
- H. Wang, Y. Xie, S. Liu, S. Cong, Y. Song, X. Xu and M. Tan, J. Agric. Food Chem., 2017, 65, 7553–7559 CrossRef CAS PubMed.
- H. Wang, Y. Xie, X. Na, J. Bi, S. Liu, L. Zhang and M. Tan, Food Chem., 2019, 286, 405–412 CrossRef CAS PubMed.
- L. Zhang, X. Na, B. Lai, Y. Song, H. Wang and M. Tan, Food Chem., 2021, 338, 127832 CrossRef CAS PubMed.
- S. Cong, J. Bi, X. Song, C. Yu and M. Tan, Food Funct., 2018, 9, 2490–2495 RSC.
- S. Cong, K. Liu, F. Qiao, Y. Song and M. Tan, Methods, 2019, 168, 76–83 CrossRef CAS PubMed.
- X. Song, L. Cao, S. Cong, Y. Song and M. Tan, J. Agric. Food Chem., 2018, 66, 7522–7530 CrossRef CAS PubMed.
- X. Song, H. Wang, R. Zhang, C. Yu and M. Tan, Food Funct., 2018, 9, 6227–6235 RSC.
- Y. Wu, X. Song, N. Wang, S. Cong, X. Zhao, R. Rai and M. Tan, Food Funct., 2020, 11, 10105–10113 RSC.
- X. Song, Y. Song, Z. Guo and M. Tan, Food Chem., 2022, 385, 132714 CrossRef CAS PubMed.
- J. Li, L. Cao, D. Li, C. Yu and M. Tan, LWT–Food Sci. Technol., 2019, 111, 588–593 CrossRef CAS.
- G. Cui, Y. Song, K. Liu and M. Tan, Foods, 2021, 10, 2336 CrossRef CAS PubMed.
- Y. Song, L. Cao, J. Li, S. Cong, D. Li, Z. Bao and M. Tan, Food Funct., 2019, 10, 3706–3716 RSC.
- X. Zhao, S. Shan, J. Li, L. Cao, J. Lv and M. Tan, Nanotoxicology, 2019, 13, 1310–1323 CrossRef CAS PubMed.
- K. Liu, Y. Song and M. Tan, J. Agric. Food Chem., 2020, 68, 9789–9795 CrossRef CAS PubMed.
- X. Yang, Y. Zhuo, S. Zhu, Y. Luo, Y. Feng and Y. Dou, Biosens. Bioelectron., 2014, 60, 292–298 CrossRef CAS PubMed.
- J. Zhang, Y. Yuan, G. Liang and S. H. Yu, Adv. Sci., 2015, 2, 1500002 CrossRef PubMed.
- Y. Zheng, H. Zhang, W. Li, Y. Liu, X. Zhang, H. Liu and B. Lei, RSC Adv., 2017, 7, 33459–33465 RSC.
- Y. Zheng, G. Xie, X. Zhang, Z. Chen, Y. Cai, W. Yu, H. Liu, J. Shan, R. Li, Y. Liu and B. Lei, ACS Omega, 2017, 2, 3958–3965 CrossRef CAS PubMed.
- S. Mandani, D. Dey, B. Sharma and T. K. Sarma, Carbon, 2017, 119, 569–572 CrossRef CAS.
- P. Surendran, A. Lakshmanan, S. S. Priya, K. Balakrishnan, P. Rameshkumar, K. Kannan, P. Geetha, T. A. Hegde and G. Vinitha, Nano-Struct. Nano-Objects, 2020, 24, 100589 CrossRef CAS.
- F. Shan, L. Fu, X. Chen, X. Xie, C. Liao, Y. Zhu, H. Xia, J. Zhang, L. Yan, Z. Wang and X. Yu, Chinese Chem. Lett., 2022, 33, 2942–2948 CrossRef CAS.
- P. Y. Lin, C. W. Hsieh, M. L. Kung, L. Y. Chu, H. J. Huang, H. T. Chen, D. C. Wu, C. H. Kuo, S. L. Hsieh and S. Hsieh, J. Biotechnol., 2014, 189, 114–119 CrossRef CAS PubMed.
- G. Gedda, C. Y. Lee, Y. C. Lin and H. F. Wu, Sens. Actuators, B, 2016, 224, 396–403 CrossRef CAS.
- H. Zhang, S. Kang, G. Wang, Y. Zhang and H. Zhao, ACS Sens., 2016, 1, 875–881 CrossRef CAS.
- R. Devi and R. Dhamodharan, ACS Sustainable Chem. Eng., 2018, 6, 11313–11325 CrossRef CAS.
- S. L. D'souza, B. Deshmukh, J. R. Bhamore, K. A. Rawat, N. Lenka and S. K. Kailasa, RSC Adv., 2016, 6, 12169–12179 RSC.
- Y. Y. Yao, G. Gedda, W. M. Girma, C. L. Yen, Y. C. Ling and J. Y. Chang, ACS Appl. Mater. Interfaces, 2017, 9, 13887–13899 CrossRef CAS PubMed.
- K. Dehvari, K. Y. Liu, P. J. Tseng, G. Gedda, W. M. Girma and J. Y. Chang, J. Taiwan Inst. Chem. Eng., 2019, 95, 495–503 CrossRef CAS.
- N. Pourmahdi, A. H. M. Sarrafi and A. Larki, J. Fluoresc., 2019, 29, 887–897 CrossRef CAS PubMed.
- D. Elango, J. S. Packialakshmi, V. Manikandan and P. Jayanthi, Mater. Lett., 2022, 313, 131822 CrossRef CAS.
- X. Zhang, R. Liu, Y. Zang, G. Liu, S. Liu, G. Wang, Y. Zhang, H. Zhang and H. Zhao, Inorg. Chem. Front., 2016, 3, 910–918 RSC.
- D. Tai, C. Liu and J. Liu, Spectrosc. Lett., 2019, 52, 194–199 CrossRef CAS.
- D. Elango, J. S. Packialakshmi, V. Manikandan and P. Jayanthi, Mater. Lett., 2022, 312, 131667 CrossRef CAS.
- S. Chen, W. Ouyang, Y. Zhu, L. He, L. Zou, X. Ao, S. Liu, Y. Yang and J. Li, Foods, 2022, 11, 2414 CrossRef CAS PubMed.
- J. Chen, X. Xia, P. Li, H. Yu, Y. Xie, Y. Guo, W. Yao, H. Qian and Y. Cheng, Food Chem., 2023, 405, 134802 CrossRef CAS PubMed.
- J. Chen, X. Xia, P. Li, H. Yu, Y. Xie, Y. Guo, W. Yao, H. Qian and Y. Cheng, Food Agri. Immun., 2023, 34, 36–47 CrossRef CAS.
- W. Zhao, Y. Zhang, B. Cao, Z. Li, C. Sun, X. Cao and S. Cong, Foods, 2022, 11, 2451 CrossRef CAS PubMed.
- L. Wang and H. S. Zhou, Anal. Chem., 2014, 86, 8902–8905 CrossRef CAS PubMed.
- D. Wang, X. Wang, Y. Guo, W. Liu and W. Qin, RSC Adv., 2014, 4, 51658–51665 RSC.
- S. Han, H. Zhang, J. Zhang, Y. Xie, L. Liu, H. Wang, X. Li, W. Liu and Y. Tang, RSC Adv., 2014, 4, 58084–58089 RSC.
- S. Han, H. Zhang, Y. Xie, L. Liu, C. Shan, X. Li, W. Liu and Y. Tang, Appl. Surf. Sci., 2015, 328, 368–373 CrossRef CAS.
- J. Wang, F. Peng, Y. Lu, Y. Zhong, S. Wang, M. Xu, X. Ji, Y. Su, L. Liao and Y. He, Adv. Opt. Mater., 2015, 3, 103–111 CrossRef CAS.
- D. Wang, L. Zhu, C. Mccleese, C. Burda, J. F. Chen and L. Dai, RSC Adv., 2016, 6, 41516–41521 RSC.
- B. Al-Hashimi, K. M. Omer and H. S. Rahman, Arab. J. Chem., 2020, 13, 5151–5159 CrossRef CAS.
- P. Fan, C. Liu, C. Hu, F. Li, X. Lin, S. Yang and F. Xiao, New J. Chem., 2022, 46, 2526–2533 RSC.
- H. Shabbir, K. Wojtaszek, B. Rutkowski, E. Csapó, M. Bednarski, A. Adamiec, M. Głuch-Lutwin, B. Mordyl, J. Druciarek, M. Kotańska, P. Ozga and M. Wojnicki, Molecules, 2022, 27, 8728 CrossRef CAS PubMed.
- A. Kumar, I. Kumar and A. K. Gathania, Sci. Rep., 2022, 12, 22495 CrossRef CAS PubMed.
- L. Zhang, B. Li, Y. Zhou, Y. Wu, T. Le and Q. Sun, J. Sol–Gel Sci. Technol., 2023, 106, 173–185 CrossRef CAS.
- R. M. K. Mohamed, S. H. Mohamed, A. M. Asran, I. H. Alsohaimi, H. M. A. Hassan, H. Ibrahim and M. M. El-Wekil, Spectrochim. Acta, Part A, 2023, 293, 122444 CrossRef CAS PubMed.
- V. N. Mehta, S. S. Chettiar, J. R. Bhamore, S. K. Kailasa and R. M. Patel, J. Fluoresc., 2017, 27, 111–124 CrossRef CAS PubMed.
- R. Su, D. Wang, M. Liu, J. Yan, J. X. Wang, Q. Zhan, Y. Pu, N. R. Foster and J. F. Chen, ACS Omega, 2018, 3, 13211–13218 CrossRef CAS PubMed.
- M. Athika, A. Prasath, E. Duraisamy, V. S. Devi, A. S. Sharma and P. Elumalai, Mater. Lett., 2019, 241, 156–159 CrossRef CAS.
- A. S. Sharma, J. Xuing, A. Viswadevarayalu, Y. Rong, D. Sabarinathan, S. Ali, A. A. Agyekum, H. Li and Q. Chen, J. Photochem. Photobiol., A, 2020, 401, 112788 CrossRef.
- R. Liu, J. Zhang, M. Gao, Z. Li, J. Chen, D. Wu and P. Liu, RSC Adv., 2015, 5, 4428–4433 RSC.
- M. I. Din, M. Ahmed, M. Ahmad, S. Saqib, W. Mubarak, Z. Hussain, R. Khalid, H. Raza and T. Hussain, J. Chem., 2023, 9956427, DOI:10.1155/2023/9956427.
- S. Das, U. S. Mondal and S. Paul, Appl. Surf. Sci., 2023, 638, 157998 CrossRef CAS.
- X. Wen, L. Shi, G. Wen, Y. Li, C. Dong, J. Yang and S. Shuang, Sens. Actuators, B, 2016, 235, 179–187 CrossRef CAS.
- J. H. Zhang, A. Niu, J. Li, J. W. Fu, Q. Xu and D. S. Pei, Sci. Rep., 2016, 6, 37860 CrossRef CAS PubMed.
- C. Zhao, Y. Jiao, F. Hu and Y. Yang, Spectrochim. Acta, Part A, 2018, 190, 360–367 CrossRef CAS PubMed.
- A. Dehghani, S. M. Ardekani, M. Hassan and V. G. Gomes, Carbon, 2018, 131, 238–245 CrossRef CAS.
- H. Liu, J. Ding, L. Chen and L. Ding, J. Photochem. Photobiol., A, 2020, 400, 112724 CrossRef CAS.
- W. Chen, J. Fan, X. Wu, D. Hu, Y. Wu, Z. Feng, M. Yan, X. Gao and J. Xie, New J. Chem., 2021, 45, 5114–5120 RSC.
- S. Narimani and N. Samadi, Microchem. J., 2021, 168, 106372 CrossRef CAS.
- L. Fu, T. Liu, F. Yang, M. Wu, C. Yin, L. Chen and N. Niu, J. Photochem. Photobiol., A, 2022, 424, 113638 CrossRef CAS.
- L. Yang, L. Zeng, Y. Tao, D. Wang, K. Zhang, M. Tian, Z. Xia and D. Gao, Microchem. J., 2022, 174, 107089 CrossRef CAS.
- L. Wu, W. Pana, H. Ye, N. Liang and L. Zhao, Colloids Surf. A, 2022, 638, 128330 CrossRef CAS.
- H. Ye, B. Liu, J. Wang, C. Zhou, Z. Xiong and L. Zhao, Molecules, 2022, 27, 6479 CrossRef CAS PubMed.
- C. D. E. S. Barbosa, J. R. Correa, G. A. Medeiros, G. Barreto, K. G. Magalhaes, A. L. de Oliveira, J. Spencer, M. O. Rodrigues and B. A. D. Neto, Chem. –Eur. J., 2015, 21, 5055–5060 CrossRef CAS PubMed.
- F. H. Horst, C. V. D. S. Rodrigues, P. H. P. R. Carvalho, A. M. Leite, R. B. Azevedo, B. A. D. Neto, J. R. Correa, M. P. Garcia, S. Alotaibi, M. Henini, S. B. Chaves and M. O. Rodrigues, RSC Adv., 2021, 11, 6346–6352 RSC.
- S. K. Baskaya, B. Tahta, S. Urus, H. Eskalen, M. Cesme and S. Ozgan, Biomass Convers. Biorefin., 2022 DOI:10.1007/s13399-022-03017-8.
- G. Wu, M. Feng and H. Zhan, RSC Adv., 2015, 5, 44636–44641 RSC.
- Y. Zhang, Z. Gao, W. Zhang, W. Wang, J. Chang and J. Kai, Sens. Actuators, B, 2018, 262, 928–937 CrossRef CAS.
- G. Liu, H. Jia, N. Li, X. Li, Z. Yu, J. Wang and Y. Song, Microchem. J., 2019, 145, 718–728 CrossRef CAS.
- Y. Zhang, Z. Gao, X. Yang, J. Chang, Z. Liu and K. Jiang, RSC Adv., 2019, 9, 940–949 RSC.
- J. Athinarayanan, V. S. Periasamy and A. A. Alshatwi, Mater. Sci. Eng. C, 2020, 117, 111313 CrossRef CAS PubMed.
- Q. F. Yao, D. S. Zhou, J. H. Yang and W. T. Huang, Sust. Chem. Pharm., 2020, 17, 100305 Search PubMed.
- C. Campalani, E. Cattaruzza, S. Zorzi, A. Vomiero, S. You, L. Matthews, M. Capron, C. Mondelli, M. Selva and A. Perosa, Nanomaterials, 2021, 11, 524 CrossRef CAS PubMed.
- C. Campalani, V. Causin, M. Selva and A. Perosa, ACS Appl. Mater. Interfaces, 2022, 14, 35148–35156 CrossRef CAS PubMed.
- P. M. Ashraf, S. Stephen and P. K. Binsi, Appl. Nanosci., 2021, 11, 1929–1947 CrossRef CAS.
- C. Xu, X. Xiao, C. Cai, Q. Cheng, L. Zhu, J. Zhang, B. Wei and H. Wang, Environ. Sci. Pollut. Res., 2023, 30, 54616–54627 CrossRef CAS PubMed.
- K. W. Kim, T. Y. Choi, Y. M. Kwon and J. Y. H. Kim, Electron. J. Biotechnol., 2020, 47, 36–42 CrossRef CAS.
- X. Tang, L. Wang, H. Ye, H. Zhao and L. Zhao, J. Photochem. Photobiol., A, 2022, 424, 113653 CrossRef CAS.
- C. Xu, J. Kang, Y. Zhao, L. Zhu, J. Zhang, B. Wei and H. Wang, New J. Chem., 2023, 47, 3159–3166 RSC.
- L. Shi, B. Zhao, X. Li, G. Zhang, Y. Zhang, C. Dong and S. Shuang, Sens. Actuators, B, 2016, 235, 316–324 CrossRef CAS.
- L. Wang, Y. Bi, J. Hou, H. Li, Y. Xu, B. Wang, H. Ding and L. Ding, Talanta, 2016, 160, 268–275 CrossRef CAS.
- S. Wang, H. Niu, S. He and Y. Cai, RSC Adv., 2016, 6, 107717–107722 RSC.
- Y. Song, N. Qi, K. Li, D. Cheng, D. Wang and Y. Li, RSC Adv., 2022, 12, 8108–8118 RSC.
- D. Sun, R. Ban, P. H. Zhang, G. H. Wu, J. R. Zhang and J. J. Zhu, Carbon, 2013, 64, 424–434 CrossRef CAS.
- S. S. Liu, C. F. Wang, C. X. Li, J. Wang, L. H. Mao and S. Chen, J. Mater. Chem. C, 2014, 2, 6477–6483 RSC.
- Y. Guo, L. Zhang, F. Cao and Y. Leng, Sci. Rep., 2016, 6, 35795 CrossRef CAS PubMed.
- A. Singh, E. Eftekhari, J. Scott, J. Kaur, S. Yambem, F. Leusch, R. Wellings, T. Gould, K. Ostrikov, P. Sonar and Q. Li, Sustainable Mater. Technol., 2020, 25, e00159 CrossRef CAS.
- Y. Tao, R. Wan, J. Wang, Q. Liu, M. Tian, L. Wang, Y. Yang, Y. Zou, Y. Luo, F. Ke, Q. Zhou, D. Wang and D. Gao, Spectrochim. Acta, Part A, 2023, 298, 122803 CrossRef CAS PubMed.
- J. B. Essner, C. H. Laber, S. Ravula, L. P. Parada and G. A. Baker, Green Chem., 2016, 18, 243–250 RSC.
- S. S. J. Xavier, G. Siva, J. Annaraj, A. R. Kim, D. J. Yoo and G. G. Kumar, Sens. Actuators, B, 2018, 259, 1133–1143 CrossRef CAS.
- X. D. Zhang, J. Li, J. N. Niu, X. P. Bao, H. D. Zhao and M. Tan, Methods, 2019, 168, 84–93 CrossRef CAS PubMed.
- T. Chatzimitakos, A. Kasouni, L. Sygellou, I. Leonardos, A. Troganis and C. Stalikas, Sens. Actuators, B, 2018, 267, 494–501 CrossRef CAS.
- T. G. Chatzimitakos, A. I. Kasouni, A. N. Troganis and C. D. Stalikas, ACS Appl. Mater. Interfaces, 2018, 10, 16024–16032 CrossRef CAS.
- J. Y. Tai, K. H. Leong, P. Saravanan, S. T. Tan, W. C. Chong and L. C. Sim, J. Environ. Chem. Eng., 2021, 9, 104622 CrossRef CAS.
- M. Y. Berezin and S. Achilefu, Chem. Rev., 2010, 110, 2641–2684 CrossRef CAS.
- H. Sies, V. V. Belousov, N. S. Chandel, M. J. Davies, D. P. Jones, G. E. Mann, M. P. Murphy, M. Yamamoto and C. Winterbourn, Nat. Rev. Mol. Cell Biol., 2022, 23, 499–515 CrossRef CAS PubMed.
- S. O. Duke and S. B. Powles, Pest Manag. Sci., 2008, 64, 319–325 CrossRef CAS PubMed.
- M. Hagner, J. Mikola, I. Saloniemi, K. Saikkonen and M. Helander, Sci. Rep., 2019, 9, 8540 CrossRef.
- C. Peillex and M. Pelletier, J. Immunotoxicol., 2020, 17, 163–174 CrossRef CAS PubMed.
- N. Nazam, M. I. Lone, A. Hamid, T. Qadah, A. Banjar, Q. Alam, M. Saeed and W. Ahmad, Toxics, 2020, 8, 80 CrossRef CAS.
- N. Maheshwari, A. A. Khan and R. Mahmood, Pestic. Biochem.
Physiol., 2023, 190, 105318 CrossRef CAS PubMed.
- F. Colakoglu and M. L. Selcuk, Vet. Sci., 2021, 8, 31 CrossRef PubMed.
- L. Hao, D. Kasatriya, X. Li, A. Badrinath, Z. Szmacinski, M. J. Goedken, M. Polunas and N. T. Bello, Food. Chem. Toxicol., 2020, 143, 111512 CrossRef CAS PubMed.
- D. L. Sedlak and U. V. Gunten, Science, 2011, 331, 42–43 CrossRef CAS PubMed.
- A. Sobrino-Figueroa, Environ. Sci. Pollut. Res., 2018, 25, 13283–13291 CrossRef CAS PubMed.
- M. Havrdova, K. Hola, J. Skopalik, K. Tomankova, M. Petr, K. Cepe, K. Polakova, J. Tucek, A. B. Bourlinos and R. Zboril, Carbon, 2016, 99, 238–248 CrossRef CAS.
- Y. Y. Liu, N. Y. Yu, W. D. Fang, Q. G. Tan, R. Ji, L. Y. Yang, S. Wei, X. W. Zhang and A. J. Miao, Nature Commun., 2021, 12, 812 CrossRef CAS PubMed.
- J. Chen, D. Sun, H. Cui, C. Rao, L. Li, S. Guo, S. Yang, Y. Zhang and X. Cao, Environ. Sci.: Nano, 2022, 9, 173–188 RSC.
|
This journal is © The Royal Society of Chemistry 2023 |
Click here to see how this site uses Cookies. View our privacy policy here.