DOI:
10.1039/D2BM02060B
(Review Article)
Biomater. Sci., 2023,
11, 3414-3430
3D bioprinting complex models of cancer
Received
15th December 2022
, Accepted 9th March 2023
First published on 14th March 2023
Abstract
Cancer is characterized by the uncontrolled division of cells, resulting in the formation of tumors. The tumor microenvironment (TME) consists of a variety of cell types present within a heterogeneous extracellular matrix (ECM). Current 2D culture methods for mimicking this microenvironment remain limited due to spatial constraints. Many different types of 3D cancer models have been developed in recent years using spheroids/organoids, biomaterial scaffolds, and cancer-on-chip systems. However, these models cannot precisely control the organization of multiple cell types inside of complex architectures. Bioprinted cancer models can incorporate both stromal and cancer cells inside of 3D constructs to generate custom models of this complex disease. 3D bioprinting can generate complex, multicellular, and reproducible constructs where the matrix composition and rigidity are tailored locally to the tumor. These capabilities make 3D bioprinting an attractive method for reproducing the tumor TME found in vivo. Recent advancements in biomaterial-based bioinks enable the generation of 3D bioprinted cancer models that accurately mimic the TM. Here we discuss recent examples of such 3D-bioprinted cancer models, including those of the lungs, prostate, skin, brain, and colon. We then highlight the advantages of using 3D bioprinting compared to other in vitro modeling techniques and detail its limitations.
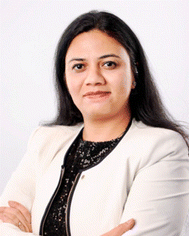 Ruchi Sharma | Ruchi Sharma earned her Ph.D. degree in June 2022 from the Department of Mechanical Engineering at the University of Victoria, Canada. She holds an M.Sc. degree in Biotechnology from Taipei, Taiwan, a B.Sc. degree in Life Science, and also an MBA degree in Marketing and Finance from Delhi, India. She is a Vice President of the Canadian Biomaterials Society (CBS), BC Chapter. She has attended several conferences in Canada and outside. She won several awards for her research during her Ph.D. and masters. Her research work is published in the esteemed journal Frontiers in Bioengineering and Biotechnology. Her doctoral work explores the significance of 3D bioprinted neural tissue models for drug screening and cell therapies for Parkinson's disease. Her research in biomedical engineering focuses on neural tissue engineering and regenerative medicine using biomaterials, stem cells, and drug delivery systems. |
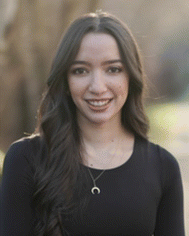 Milena Restan Perez | Milena has a Bachelor of Engineering degree in Biomedical Engineering from the University of Victoria and she currently works as an engineer at Axolotl Biosciences. Her research work has been focused on engineering tissues using 3D bioprinting and stem cells. She has also worked with patient-derived glioblastoma cells and is currently testing a potential drug delivery system for glioblastoma multiforme. |
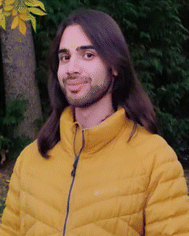 Victor Alisson da Silva | As a Biomedical Engineer with a Master's degree in Applied Science in Mechanical Engineering, Victor has a passion for developing innovative solutions to complex medical problems, particularly in the field of cancer screening and 3D bioprinting applications. He brings a unique blend of technical expertise, real-world experience, and a well-rounded, interdisciplinary perspective to the table. His background in data science and experience in working in R&D have equipped him with the skills to drive progress in these fields through the use of machine learning and advanced technologies. |
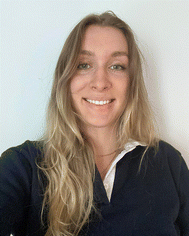 Jess Thomsen | Jess has a bachelor's degree in Biomedical Engineering from the University of Victoria. She worked in the Willerth Lab in 2022, including completing a co-op term in collaboration with Axolotl Biosciences. Her main research revolved around 3D bioprinting of stem cells, as well as investigating the mechanical properties of different types of 3D bio-ink. |
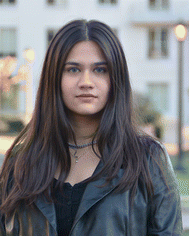 Lavanya Bhardwaj | Lavanya Bhardwaj is currently in her second year of undergraduate studies at UC Berkeley. She is majoring in Cognitive Science and has worked with mammalian cells at the Willerth Lab. |
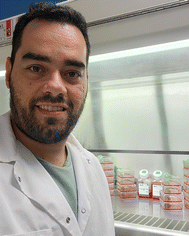 Thiago Andrade | Thiago received his undergraduate degree in Biology, Master's degree, and Ph.D. in Medical Sciences from the University of Sao Paulo (Brazil). He was Professor of Pathology and Immunology and Professor/Researcher at the Graduate Program in Biomedical Sciences at the University Center of Herminio Ometto Foundation – FHO|UNIARARAS (Brazil). His main research field has been about skin and wound healing therapeutics involving in vivo (animal experimentation and human clinical trials) and in vitro (skin cell and tissue culture) investigations. During his career, he supervised 21 graduate students and published more than 60 papers in the skin/wound healing field. He joined the Willerth Lab in September/2021 as a Postdoc working with 3D bioprinting of human skin co-culture models. |
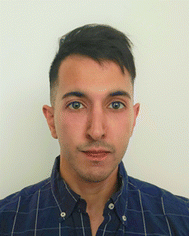 Abdulaziz Alhussan | Abdulaziz Alhussan is currently a PhD student in Medical Physics at the University of Victoria. He holds an M.S. degree in Medical Physics, an M.S. degree in Radiation Health Physics, and a Nuclear Engineering B.S. degree from the United States. His current project involves using a combined modality of radiotherapy, nanotechnology, and chemotherapy in an in vitro co-culture environment that mimics the in vivo tumor microenvironment. The application will involve modeling the complexity of cancer in a 3D structure and analyzing the effectiveness of such targeted therapy. The project will accelerate transitioning from cell culture preclinical studies to animal models and eventually to clinical trials. |
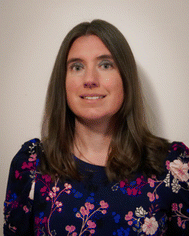 Stephanie M. Willerth | Dr Willerth, a Full Professor in Biomedical Engineering, holds a Canada Research Chair in Biomedical Engineering at the University of Victoria where she has dual appointments in the Department of Mechanical Engineering and the Division of Medical Sciences. She also holds an appointment with the School of Biomedical Engineering at the University of British Columbia. She recently founded the start-up company Axolotl Biosciences, which sells high quality bioinks for bioprinting human tissue models. She served as the Acting Director of the Centre for Biomedical Research and the Biomedical Engineering undergraduate program at the University of Victoria from 2018 to 2021 and as the President of the Canadian Biomaterials Society from 2018 to 2019. She was elected to the Royal Society of Canada's College of New Scholars and the Engineers and Geoscientists of B.C. awarded her their Teaching Award of Excellence in 2021. |
Introduction
Cancer is the second-leading cause of death globally.1 Tumors occur when cells develop the ability to penetrate and destroy healthy human tissue while uncontrollably proliferating – which is one of the hallmarks of cancer.2 Accordingly, cancer has the propensity to spread throughout the body.3 The overall five-year net cancer survival rate is currently 64%.4 It was estimated that 19.3 million new cases of cancer worldwide (18.1 million excluding nonmelanoma skin cancer) and nearly 10 million cancer deaths (9.9 million excluding nonmelanoma skin cancer) occurred in 2020.5,6 With an estimated 2.3 million new cases (11.7%), female breast cancer has surpassed lung cancer as the most frequently diagnosed cancer. Lung (11.4%), colorectal (10.0%), prostate (7.3%), and stomach (5.6%) cancers are the next most frequently diagnosed types of cancer.5,6 An estimated 46% of all cancer diagnoses in 2021 were for lung, breast, colorectal, and prostate. Even though thyroid cancer is less prevalent than many other types of cancers, the National Cancer Institute estimates that 44
000 new cases are reported each year in the United States. However, it has a very high 5-year survival rate regardless of the stage of ∼98% overall. Additionally, the incidence of melanoma (skin) cancer continues to rise despite being a largely preventable disease. Lung cancer (25%), colorectal cancer (11%), and pancreatic cancer (7%) are estimated to be the top three causes of mortality due to cancer in 2021.7
Most lung cancers develop from cells lining the bronchi and other areas of the lung, such as the bronchioles or alveoli. The most prevalent types of lung cancer are mesothelioma, non-small cell lung cancer, small cell lung cancer, and lung nodules.8 Surgical procedures, radiotherapy, chemotherapy, and immunotherapy are the most frequently used forms of treatment for lung cancer. Prostate cancer (PCa) can impair urinary system function because the prostate functions as a muscle-driven mechanical switch for urination. PCa is the second most common type of cancer in men and the fifth leading cause of death worldwide.9–12 A lot of androgen-dependent PCa initially responds to hormone therapy.13,14 Hormone therapy, chemotherapy, and radiotherapy are currently the treatments for prostate cancer. Unfortunately, these treatments ultimately make it difficult to prevent tumor metastasis and androgen resistance. Additionally, these forms of therapy are extremely toxic to normal tissues and promote drug resistance in addition to being unable to stop the growth and metastasis of tumors. Consequently, the search for reliable, safe, and especially androgen-targeting treatments continues to be important. Colorectal cancer (CRC) is the third most prevalent cancer and the second leading cause of death among carcinomas. Poor prognosis persists, especially for advanced diseases or specific molecular subtypes of CRC, emphasizing the immediate need for improved therapeutic approaches.
Glioblastoma (GBM), the most malignant type of primary brain tumor, occurs primarily in the cerebral hemisphere.15,16 GBM tumors arise from astrocytes, and these tumors can grow quickly and spread throughout the brain to the cerebellum, brainstem, or spinal cord.16,17 Complete removal of GBM tumors can be difficult and even after partial removal, its recurrent nature can be fatal. Additionally, chemotherapeutic drugs sometimes cannot cross the blood–brain barrier (BBB), making it difficult to treat. Current treatments for GBM consist of surgery to remove the tumor, radiation to treat any remaining tumor, and chemotherapy using temozolomide (TMZ). The prognosis for patients varies according to age, size of tumor, response to treatment and other patient-specific health factors.15,16 A recent study showed that patients who underwent a combination of TMZ chemotherapy and radiation therapy had a median survival rate of 14.6 months, and patients with no chemotherapy had a median survival rate of 12.1 months.18 Thus, a combination of chemotherapy and radiation therapy only increased the survival rate by 2.5 months. Although this is a significant increase in survival, there is room for new therapeutic approaches to increase patient survival rates.16 There are three main types of skin cancer: melanoma, squamous cell carcinoma (SCC), and basal cell carcinoma (BCC). SCC and BCC belong to the non-melanoma skin cancer (NMSC) group and represent most skin cancers. Melanoma is caused by UV-induced damage to the melanocytes’ DNA.19,20 SCC starts in the squamous cells of the epidermis, arising from the accumulation of dysplastic keratinocytes that display frequent mutations in the tumor suppressor gene p53, primarily caused by UV radiation, that grow to form a tumor faster than BCC.19 Finally, BCC arises from abnormal epidermal basal cells and tends to grow slowly. However, prompt treatment of BCC is vital because as the tumor grows, it becomes more dangerous and may grow into nearby tissue leading to deformity and significant morbidity.19,21
Malignant melanoma arises from melanocytes, which are epidermis cells that produce skin pigments. Thus, it typically affects the skin, the uvea and retinal pigmented epithelium, mucosae, and very rarely visceral organs.22,23 Therefore, early diagnosis and early therapies are crucial for a positive outcome. Sun exposure, mainly during childhood and adolescence, can induce melanocytic naevi (benign melanocytic tumors), and increased numbers of naevi are associated with a higher risk of melanoma.24 Currently, there are several types of therapeutic treatments available for patients diagnosed with melanoma. The standard treatments used for the removal of tumors include surgery, immunotherapy, targeted therapy, and less frequently chemotherapy. Other forms of therapy, such as oncolytic virus therapy, are also being researched for the treatment of melanoma.25 Among the different skin cancers that affect the human body, melanoma is one of the most aggressive and has a poor prognosis when it invades the dermis. The primary environmental driving factor of cutaneous melanoma arising over hair-bearing skin is exposure to UVR (direct sun exposure or through devices, such as tanning beds).25
The tumor microenvironment (TME) plays an important role in how cancers form and progress.26–31 The TME consists of tumor cells, tumor stromal cells, including stromal fibroblasts, endothelial cells, immune cells like microglia, macrophages, and lymphocytes, as well as extracellular matrix (ECM) substances like collagen, fibronectin, hyaluronan, and laminin, among others as shown in Fig. 1.
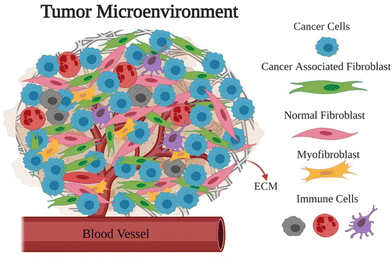 |
| Fig. 1 Schematic displaying the complexity of the tumor microenvironment (TME). | |
Biomaterials offer a way to create microenvironments both in vitro and in vivo.27,32–35 Important elements of the TME can be reconstructed utilizing 3D matrices in an in vitro cancer model.32,35 Most of our understanding of cancer development and progression is based on histological investigations of tumors developed in vitro in 2D.13,20,31 Animal models of cancer have limitations including species differences, difficulty in mimicking human disease progression, ethical concerns, and limited predictiveness for human response to treatment. These limitations hinder the accuracy and applicability of findings obtained from animal studies to human cancer.34 On the other hand, the ability of 2D culture techniques to imitate the cancer cell environment is severely constrained as cancer exists in an inherently 3D environment.36 Cancer drugs that reach the clinical trial phase have a high rate of failure.37–40 Several studies report that roughly 95% of anti-cancer drugs evaluated in phase I clinical trials never make it to the market.20,41–45 This high rate of failure can partially be attributed to inadequate pre-clinical methods for developing new pharmaceutical candidates.3,26,38 2D cell culture models are commonly used to screen potential drug targets for cancer.36 However, 2D cultures cannot accurately replicate the original TME, complex cellular composition, and dynamic interactions.26–30,46,47 Typically, cells are cultured in a 2D system on a plate or dish mainly made of polystyrene. The physiological characteristics of cells in 2D, such as interactions between the cellular and extracellular environments and changes in the cell morphology and polarity, and the cell division method, can differ significantly from the 3D environment. In addition to the limitations of tissue complexity and cell behaviour in 2D, 3D models also offer a high control for drug screening, tumor heterogeneity and the possibility to generate patient specific models. Other techniques such as the use of spheroids, organoids, cancer-on-a-chip technology, tissue engineered scaffolds, and bioprinting have been introduced to cancer models to create 3D microenvironments.30–33 The use of spheroids in cancer research allows for a more detailed analysis of molecular diffusion, while organoids can accurately replicate the organization of various cell types found in the body.33–38 Nevertheless, these models have the drawback of not being able to precisely control the arrangement of various cell types in complex architecture and are difficult to produce, especially spheroids. However, organoids are pluripotent stem cell-derived 3D cell culture systems that include tissue-specific cell types and mimic the characteristics of developing tissue.
Organoids may also serve as a platform for testing therapeutics in human cells and be a source for cell replacement therapies for conditions such as injury or disease. Despite the promising characteristics of organoids, their wide range of applications is constrained by several challenges that still need to be overcome. These obstacles include a lack of high-fidelity cell types, limited maturation, uncommon physiology, and a lack of realization, all of which may reduce their reliability for particular applications. To overcome the challenge of assessing a drug's cytotoxicity in an in vivo environment, cancer-on-chip technology employs microfluidics strategies to mimic the flow and migration patterns of cancer cells and bioprinting offers a highly reproducible approach to creating intricate architectural structures with promising implications for personalized medicine.15–20 Complex, multicellular, and reproducible constructs can be created using 3D bioprinting with the matrix's rigidity and composition being uniformly adjusted to match the tumor model. 3D bioprinting can potentially serve as an improved method for mimicking the TME because we can control the factors much more. Like all tissues, cancer behaves very differently outside of the human body than it does inside. In terms of drug discovery, 3D bioprinted constructs accurately mimic the in vivo cancer environment. The 3D bioprinting process entails the precise deposition of biomaterials and cells in specific patterns and layers which is based on a computer-aid design (CAD) model. These models can simulate the 3D heterogeneity of real tumors to provide cell–cell and cell–matrix interactions that are physiologically relevant. Cell function can be improved by using biomaterials that contain elements of the ECM. As a result of the interaction with biomaterials (both natural and synthetic biopolymers), cells will be better able to proliferate, differentiate, interact with one another, synthesize the ECM, and perform biological functions, resulting in the realization of cancer cell–environment interactions. A promising method for screening personalized cancer therapies is to use bioprinted cancer models that include stromal and cancer cells from the patient along with genetic material, extracellular matrix proteins, and growth factors. 3D cancer models hold great potential for advancing the study of cancer biology, which has been historically investigated in 2D cultures of tumor cells. Advanced 3D bioprinted cancer models have the potential to revolutionize the way to discover therapeutic targets, develop new drugs and personalize anticancer therapies in an accurate, reproducible, clinically translatable and robust manner. These ex vivo cancer models are already replacing existing in vitro systems and could, in the future, diminish or even replace the use of animal models.48 Although animals are physiologically competent, they often fail to reproduce human behaviours and responses, and 2D cell models are extremely simplified and not representative of what may happen in vivo. Therefore, 3D cell models are believed to better emulate the complex native tumor microenvironments.49 In this review, we will discuss 3D modelling of cancer using conventional techniques, 3D bioprinting techniques and bioinks used to develop 3D-bioprinted models of colorectal, lung, prostate, skin, and brain cancers as well as their advantages and challenges.
3D models of cancer
Many ways exist to model cancer in 3D settings in addition to the use of bioprinting. The variety of available models makes it hard to compare results stemming from different models. The choice of which model to use often stems from the intended application of the cancer model.50 Many different 3D in vitro models have been described in the literature with different uses and benefits.51 Each method has its own advantages and disadvantages. The methods that will be examined in detail are as follows: the use of spheroids/organoids and microfluidic devices, focusing on organ-on-a-chip devices.
Spheroids/organoids
Scaffold-free methods of 3D modelling tumors rely on self-aggregation of the cells in specialized plates, such as ultra-lower attachment microplates. These aggregates are known as spheroids/organoids, and have some similarities to in vivo tumors, including volume growth kinetics, cellular heterogeneity, and cell secretions.52 Spheroids derived from patient-specific cancer cells retain their genomic structure, allowing for the facilitation of patient-specific testing and drug development.53 The hanging drop method uses a specialized plate that allows for the formation of drops of media, with cells encapsulated, forcing self-aggregation due to the lack of an adhesion surface.54 Over the span of several days, spheroids form tumor-like structures. An ultra-low attachment plate relies on the same principle, allowing for a spheroid to form due to the lack of an adhesive surface. However, ultra-low attachment microplates have the advantage of allowing a larger diversity of cell growth capabilities, such as prolonged experimentation and the production of spheroids of 1–2 mm diameter, which are not easily reproduced using the hanging drop method due to its inconsistency.51 Cells seeded within hydrogels such as collagen and Matrigel can also form tumors that are similar to traditional tissue engineering. Naturally sourced hydrogels, which are 95% water by volume, provide a cell–liquid interface and have natural adhesive properties, allowing for high cell viability and controlled proliferation.29,55 Cancer cells can grow within the hydrogels, and combine to form 3D cancer systems, However, Matrigel is derived from mouse tumors and contains many components that are not well defined. Accordingly, these matrices can have different compositions, leading to the expression of different cancer phenotypes.56–58 As with most 3D models, a lack of vasculature remains a challenge to be addressed.37 The structure of spheroids causes an oxygen gradient to form,59 where there is less oxygen in the centre of the spheroid in comparison with the outermost environment. This can result in cell death in the core of the spheroid where hypoxia occurs. In contrast, the outside borders of a spheroid are in close contact with cell media, which means that this environment has good nutrient and oxygen exchange.37,59 One benefit is that the 3D geometry of spheroids does mimic the structure of tumors found in vivo. However, the only ECM present is secreted by the tumor cell, which does not accurately mimic the native environment of a tumor. Additionally, the distance of invasion cannot be determined using a spheroid model since there is limited compartmentalization between the tumor and the stromal cells. This issue remains even when a co-culture is performed. Finally, there is limited collagen density within spheroids, other than what is produced by the cells themselves.59 Thus, these spheroid models have certain advantages and limitations as a tool for modelling cancer.
Microfluidic devices – organ-on-a-chip
3D in vitro systems can address the shortcomings of conventional 2D in vitro systems while providing novel biological perspectives. However, cell–cell interactions and spatial structures are only partially supported by conventional 3D devices. Additionally, a system's effectiveness as a high throughput screening platform is constrained by the substantial sample volume needed. As a result, there has been an increase in interest in innovative 3D culture systems that can offer better biological models and functionality while lowering necessary quantities and cost. Microscale 3D in vitro models provide a way to increase the functionality and throughput of conventional 3D systems. The advancement of microfluidic 3D cancer models, as well as their benefits and multiple applications to cancer, might be advantageous.38,60,61 Microfluidics uses miniature devices that process or manipulate small volumes of fluids through channels that have sizes between a few tens and many hundreds of micrometres. To create microfluidic chips, thin grooves or small wells are typically made on the surface of one layer, which is then enclosed by a second layer to create a chamber containing microchannels. It is essential for channels to be leak-proof, so the layers must be tightly bonded.61 However, their miniature nature means that organ-on-a-chip models do not allow for the recreation of the tumor at an accurate scale.34 They also lack ECM components unless they are added to the channels on the devices.9 Microfabrication limitations lead to a limited possible range of dimensions and structures.37 Furthermore, long term studies pose challenges, due to the inclusion of multiple tissue and cell types. When using media and consistent fluid flow, it is difficult to maintain cell viability, functionality, and the structural integrity of the model.34 There are many factors that influence the success of these models, and it is imperative that they all work together to achieve the desired functionality.37,62
3D bioprinting
Advances in tumor biology require a 3D microenvironment to replicate the complex interactions of tumor cells with their microenvironment.36 3D bioprinting holds great promise for the generation of cancer models as it can replicate the 3D microenvironment and enable diverse cultures under carefully regulated chemical and mechanical conditions. It creates 3D biological structures by depositing biomaterials combined with cells in predefined patterns, layer by layer, using a bottom-up assembly approach. Several bioprinting strategies have already been used for these applications, however, the ones that stand out most are extrusion, droplet, and laser-based.30,63,64Fig. 2 shows a summary of these techniques. Extrusion-based bioprinting, a widely used technique, utilizes air pressure, mechanical pistons, or screws to extrude bioinks into desired patterns. This method allows for the use of a wide range of materials and is relatively simple and cost-effective. However, the pressure applied during the process can be detrimental to the cells, resulting in lower survival rates and reduced function of the printed tissue. This type of approach provides versatility in cancer research as they can create models that mimic in vivo tissue architecture and represent different stages of cancer, allowing for a comprehensive understanding of the disease and potential treatment options.63 By utilizing extrusion bioprinters to deposit cells in the creation of compartmental tumoroids, Mazzaglia and Sheng closely mimicked the in vivo tumor environment.65 When combined with a collagen matrix rich in immune cells, this allows for the study of the interactions between the immune cells and the tumoroids, providing valuable insights into the mechanistic understanding of stromal cancer interactions as well as future drug testing approaches. In addition, these models can mimic different tumor stages and study the role of specific biomolecules and cell lines under controlled conditions.9,64 Inkjet or droplet bioprinting offers an alternative to extrusion-based bioprinters. This is a technique that uses energy sources such as thermal and piezoelectric to deposit precise droplets of cell-encompassing bioinks onto a supporting material. This allows for the creation of high-resolution structures at a relatively low cost. However, the technique's success is dependent on the physical properties of the bioink, such as viscosity and surface tension, which may limit the range of biomaterials that can be used.65 This approach has been effectively used to print uniform drops containing human breast cancer cellular spheroids into concave wells, providing a way to generate models for bio-imaging during cancer studies.37 Additionally, this technique can be used to print highly complex combinations of biomaterials such as hydroxyapatite,66 polyethyleneimine (PEI), and riboflavin sodium phosphate (HE) to create more realistic and informative cancer models.67 Likewise, laser-based bioprinting has also shown promising results when generating cancer models. Laser-based bioprinting is a cutting-edge technique that offers exceptional precision and accuracy in the placement of cells. It allows for the creation of highly detailed structures with unparalleled resolution. The laser beam is used to generate small, precise droplets of cell-laden materials, which are deposited according to the intended design. However, this method is complex and can only be used with a limited range of materials. Additionally, the wavelength of the laser used can impact the viability of the cells, leading to lower cell survival rates.
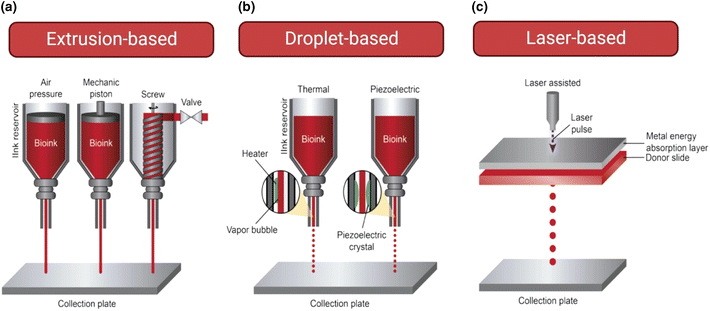 |
| Fig. 2 (A) Extrusion-based bioprinter: filaments of the bioink are deposited in response to pressure. (B) Droplet-based bioprinter: external energy causes fine droplets of the bioink to be ejected from the nozzle. (C) Laser-based bioprinter: a pulsed laser beam scans the surface of the reservoir and generates a specific cure of the bioink. Reproduced under Creative Commons Attribution License (CC BY) (https://creativecommons.org/licenses/by/4.0/).70 | |
The ability of this technique to produce complex images using light, highly complex designs, such as honeycomb branched structures with microchannels ranging from 25 to 120 μm, were generated to compare the behaviour of cancer cells and non-cancerous cell lines.68 Laser-induced forward transfer (LIFT) is a widely used laser-based bioprinting technique that has been shown to enhance drug-screening studies. By utilizing this technique, Kanaki and collaborators have been able to bioprint gemcitabine, a chemotherapy drug used to treat cancer, into microneedles. This allows for the investigation of ways to minimize side effects and optimize drug applications.69
3D bioprinting begins with selecting the appropriate bioink, whether it be natural or synthetic. However, there is no universal bioink, which makes it difficult to compare results between studies since it adds another variable. Artificially manufactured bioinks can cause low cell viability, but have good mechanical properties and printability, and the opposite is true for naturally occurring bioinks.71 Another example of inconsistencies in 3D bioprinted models includes the usage of different seeding densities. While there is currently a big push to develop new functional polymers to be used in bioinks, they may not have ideal mechanical properties such as rheological and viscoelastic properties for bioprintability and simultaneously possess bioactivity.59 Ideally, the polymers should be optimized so that they exhibit appropriate functional properties, mechanical strength, and cytotoxicity while still maintaining printability with 3D bioprinters. However, it is important to create 3D tissue models in vitro for the drug development process. Accordingly, it is essential to use biomaterials to enhance cell function and activity. For high-throughput drug screening, recent developments in 3D bioprinting technology have been extensively used to produce representative bioengineered tumor in vitro models that accurately reproduce the tumor tissues and microenvironment. Furthermore, bioprinted patient-specific cancer models can be used as a preclinical immunotherapy screening tool. The tumor microenvironment, which is made up of cancer cells, stromal tissue, and various immune cells like T cells and macrophages, can vary from patient to patient. The development, progression, and treatment of cancer are thought to be significantly influenced by the interactions between immune and tumor cells in this microenvironment. Furthermore, 3D bioprinted models are expensive to prepare at a large scale, and the collection of cells may present a challenge.37 3D cancer models for a preclinical screening tool would require creating a dynamic environment within the hydrogel environment.28,66
Case studies of bioprinting cancer
Bioprinted lung cancer models
3D bioprinting lung cancer models can enable the simulation of their invasion and metastasis characteristics. Wang et al. used a suspension of the human lung cancer cell A549/95-D to create a cell-laden hydrogel grid scaffold structure using the low-temperature molding principle of biological fabrication and 3D bioprinting.8 A549/95-D cells were collected in suspension at a density of roughly 3 × 106 ml−1, and mixed with gelatin–alginate solution at a volumetric ratio of 1
:
2 to create bio-inks for printing, resulting in a final cell density in the hydrogel of 106 ml−1. The printing parameters such as the speed and extruding speed were set to 3.5 mm s−1 and 0.15 ml s−1, respectively. In this experiment, standard dispensing needles with an inner diameter of 260 μm were used as printing needles. The eight-layer structure was 12 mm wide and 12 mm long. After printing, this structure was immersed for 3 minutes in a 3% (w/v) sterile calcium chloride solution to strengthen the cross-linking between the calcium ions and sodium alginate. After being washed 2 times in phosphate-buffered saline (PBS), the printed structures were cultured in DMEM containing 10% fetal bovine serum (FBS). These cells were evenly dispersed throughout the hydrogel that was printed and were still viable after printing, indicating that the cells were not significantly affected by the pressure and temperature changes that occurred during printing. Furthermore, matrix metalloproteinases 2 (MMP2) and MMP9, which are related to invasion migration, were chosen as target genes for quantitative PCR (qPCR) detection. This study performed a preliminary evaluation of the biological characteristics of the printed cells, focusing on cell invasion and migration abilities, using a scratch test and the assessment of MMP2 and MMP9 genes. The results showed that both types of cells showed greater expression of both genes in the 3D than in the 2D. After printing and culturing, the relative gene expression of 95-D cells in the 3D group increased several times, to more than ten times, while it was even higher for A549 cells. This result shows that A549 and 95-D cells both had higher invasion and migration potential in the bioprinted in vitro 3D tumor model than in 2D. Thus, 3D printed cells outperformed 2D cultured cells in terms of both in vitro and gene properties. These findings demonstrate that it is possible to create a 3D bioprinted lung cancer model that resembles a tumor. Although the ECM and environment could be somewhat mimicked by the gelatin–sodium alginate system, it was difficult to keep the constructed model in vitro for longer than three weeks because the structure would disintegrate, confirming the crucial roles of biomaterial selection in 3D bioprinting.8,72,73 Furthermore, more specific 3D bioprinted alveolar lung models could be used as disease models.
Bioprinted prostate cancer models
Studying intercellular interactions and performing drug development studies will be a lot easier with the help of a reliable, reproducible 3D bioprinted model of a prostate tumor.9,11,13,74 The use of a 3D model allows for high throughput testing in a far more accurate way than presently capable in monolayer models widely used in the lab. Spheroids, for example, allow for the inclusion of a concentration gradient as well as the ECM, which are all present in the in vivo tumor environment. The inclusion of this more complex method of testing will accelerate the appropriate modelling of the TME and theoretically reduce the cost and time for translation into the clinic. 3D spheroid models have the advantage of creating smaller tumor sizes, that might not be easily attainable using 3D-bioprinters, to suit the application it is intended to be used for. For example, for testing the IC-50 of chemotherapeutic drugs such as docetaxel (DTX), a size of ∼300–400 μm is the optimum choice, because larger spheroid sizes introduce a necrotic core and a rise in the percentage of quiescent cells could thus impact the experimental findings.75
3D bioprinted prostate models are also feasible, effectively representing its TME which could help with pre-clinical drug testing.74,76 Using the advantages of bioprinting, in vitro engineered bone structure can be prepared and used as a platform for PCa metastasis research. Collagen accounts for more than 12% of the 120 types of ECM proteins in the prostate, and deregulation of collagen metabolism was also associated with the PCa Gleason score and may be a determinant of patient survival. Collagen is a major ECM component in urological organs and has been linked to the progression of urological disease.9,10,76 To mimic the morphological and functional humanized organ bone, Holzapfel et al. used a 3D printing extrusion system along with a rotating structure to prepare a hollow tube using medical-grade PCL (mPCL) with a diameter of about 6 mm; it could be used as a homing site for PCa cell metastasis. The recombinant human bone morphogenetic protein-7 (rhBMP-7) growth factor was used to stimulate the metabolism and production of ECM components after human mesenchymal progenitor cells were seeded on the bone structure. PCa cells showed a propensity for the engineered bone structures, proliferated, and developed macro-metastases following further culture. The mechanism of PCa cell behavior after metastasizing to the bone microenvironment might be investigated using this model.76 3D bioprinted prostate models are also feasible, effectively representing its TME which could help with pre-clinical drug testing. The use of 3D printed prostate cancer models is becoming more common for patient education and urological surgical planning.10 Multi-colour extrusion fused deposition modeling (FDM) can be used to produce 3D prostate cancer models. These studies on 3D printed models frequently focus on creating expensive, high-quality models. The use of new generation FDM printers with multiple extrusion is a promising path to 3D printed patient-specific models in the field of medicine.11
Colorectal cancer (CRC)
Using tumor-associated stromal cells, Chen et al. created an in vitro 3D model of tumor tissue that replicated in vivo cell physiological function.77 The collagen-PCL scaffolds, which were prepared, were used to assemble the 3D bioprinted tumor tissue. A uniquely engineered electro-hydrodynamic jet (E-jet) 3D printing system, which includes a 3D collection platform, a liquid feed system, a high voltage power supply, and an observation system, was used to create the 3D scaffolds used in this study. An electric field was used to induce fluid flow from micronozzles to print fibres at the micro- and nanoscale levels. These scaffolds were put in a Petri dish with a diameter of 10 cm and sterilised for one hour with UV light. The tumor cells (HCT116) and activated stromal cells (CAFs and TECs) were combined in a 5
:
1
:
1 (HCT116
:
CAF
:
TEC) ratio before being seeded (1 × 106 cells per mL) onto the 3D scaffolds. In order to prevent uneven cell seeding brought on by scaffold floating, the scaffolds were incubated for 2 mL of DMEM in an incubator for 2–4 hours. 8 mL of medium was added following the cell adhesion to the scaffolds. The scaffolds were seeded with colorectal cancer cells, cancer-associated fibroblasts, and tumor-associated endothelial cells to create an ECM that facilitated cell processes like adhesion, cell survival, proliferation, and vascularization. Cancer stem cells (CSCs)-enriched spheroid models for drug screening are created using 3D bioprinting with cell-laden gelatin methacrylate (GelMA)-nanoclay hydrogels. GelMA-nanoclay hydrogels were highly porous with appropriate mechanical properties and good cytocompatibility with CRC cells. Additionally, the CRC CSCs were stimulated and enriched by the hydrogels, which endowed them with better in vitro self-renewal capacity and in vivo tumorigenic potential. In addition, the mechanism governing stemness regulation was investigated, and GelMA-nanoclay hydrogel-activated Wnt/-catenin signaling was found to contribute to the induction and enrichment of CSCs.78 To construct a TME, normal stromal cells were activated and reprogrammed into tumor-related stromal cells. Their results showed that tumor-related markers were overexpressed by the activated stromal cells, and they modified the ECM. Furthermore, tumor progression results in 3D in vitro models were similar to those found in vivo tumor models. These tumor progression results were assessed by metabolic activities such as up regulation of glycolysis, glutaminolysis, lipid metabolism, the pentose phosphate pathway, and mitochondrial biogenesis. In conclusion, this model is appropriate for CRC tumor biology research studies and the creation of highly personalized cancer treatments.2,77,78
Glioblastoma multiforme (GBM)
Current GBM 3D bioprinted models aim to better understand disease progression and as a potential tool for drug testing. This is being done by engineering improved methods to control the spatial organization of the tumor and by printing multiple cell types to create a co-culture model that better mimics the tumor. The most common GBM cell line used when creating 3D models are U87-MG's which was obtained from a male patient who had GBM.17,18,79,80 This cell line is readily available and easy to handle, hence why it is the most common cell type when constructing GBM models. However, a 2016 study showed that this cell line had a different DNA profile when compared to the original tumor. The next most common cell lines are (i) U118-MG, derived from a 50-year-old male patient with grade IV GBM;13,14,81 and U251-MG, derived from a malignant GBM tumor.82 Although less common, mouse glioblastoma cells (GL261) have been used to study the crosstalk between GBM cells and GBM-associated macrophages which were also derived from mouse.16,31,79,83–85 In this case, since the macrophages were mouse-derived, it was most ideal to use a mouse-derived GBM cell line rather than a human cell line. Patient-derived GBM cells are the most ideal for the study of patient-specific disease progression and drug testing. For example, Maloney et al. used patient-derived GBM cells at a concentration of ten million cells per construct, an extrusion-based bioprinter, and a collage-hyaluronic acid bioink to bioprint ununiformed droplets. This showed how bioprinted tumor models could be used for patient-specific drug screening, which would allow oncologists to choose the best drug for the specific tumor.3
In addition to GBM cells, various studies use glioblastoma stem cells (GSCs) which self-renew and have multi-lineage differentiation capacity. They are also associated with tumor initiation, contribute to disease progression, and cause therapeutic resistance.31,79,85–88 For example, Tang et al. bioprinted cylindrical constructs containing human patient-derived GSCs (TS576) at a concentration of ten billion cells per millilitre of bioink in the center of the cylinder, and endothelial cells in co-culture in the perimeter of the construct, using a digital light processing (DLP) bioprinter and a bioink consisting of glycidyl methacrylate hyaluronic acid (GMHA) and GelMA to create a biochemically relevant microenvironment.85 This study showed that biophysical cues contribute to varying tumor cell behaviour and cause different molecular subtypes of GBM to differentiate, with stiffer models causing higher drug resistance to TMZ.85 Another study found that GSCs that were incorporated into a bioprinted construct that had a diameter of 3 mm, showed more resistance to chemotherapeutic drugs in 3D when compared to 2D monolayer cultures.79 Most multicellular models also include endothelial cells such as human umbilical vein endothelial cells (HUVECs) for vascularization since without this, the model would lack angiogenesis which is a critical characteristic of cancer.18,79,82,85 Vascularization was assessed by staining the printed tissues with anti-CD31 which is a protein that is expressed in intercellular junctions when cells form capillary tubes. Finally, some studies incorporate stromal cells, microglia, and/or healthy astrocytes into their multicellular 3D models. A study by Smits et al. bioprinted a half-spherical construct containing U87-MG cells at a concentration of one million cells per millilitre of bioink, with human astrocytes to determine what effect an N-cadherin antagonist had on the cells. They found that the antagonist prevented spheroid formation, but it also caused the astrocytes to detach from the substrate and become rounded; however, at a lower concentration of the antagonist, the astrocytes did not become detached.80 This study shows how 3D bioprinted co-culture models with healthy and diseased cells can be used to study new drugs and determine an appropriate concentration that will not adversely affect healthy cells.
Several biomaterials used to properly mimic the ECM of a GBM tumor include alginate, collagen, and fibrin. The most commonly used hydrogel is alginate, a biocompatible, non-toxic material whose mechanical properties can be manipulated to resemble native tissue. However, cells placed in alginate do not show any receptor-mediated engagement with the hydrogel which causes a spherical cell morphology. To prevent this from happening, a study modified alginate with peptide sequences which allowed U87-MG GBM cells to spread and adhere to the alginate matrix.79 Other studies have incorporated biomaterials such as fibrin into an alginate-based bioink to promote cell growth and stem cell-like expression in tumor models.17,18 An issue with fibrin is that it does not provide structural integrity after extrusion. For this reason, Heinrich et al. added gelatin to their bioink so that the construct is stable immediately after extrusion.83 Another study included gelatin in their GAF (gelatine/alginate/fibrin) bioink because it could be easily crosslinked at low temperatures.18 Additionally, gelatin has been included in several bioinks for extrusion-based bioprinting because it has shear-thinning properties, which protects the cells from the inherent shear stress in extrusion-based printing.87 Another popular material in GBM models is GelMA because its mechanical properties can easily be adjusted while having negligible effects on biochemical cues is highly biocompatible and has shear-thinning properties.31,85 In bioinks where GelMA is used, hyaluronic acid (HA) is typically integrated into the bioink at a constant concentration.85 HA can influence various cellular behaviours such as survival, proliferation, adhesion, and migration. Thus, the concentration of GelMA is adjusted to mimic the stiffness of the tumor and HA is kept constant to prevent any potential impacts on the GBM cells.85 Finally, collagen is the least common biomaterial used when modelling GBM. However, type I collagen has been used in a collagen-HA bioink because it allows cells to reorganize by bundling collagen fibrils, which generates collagen architectures that play a critical role in diseased tissue where the ECM changes over time. Most studies with bioinks that are alginate-, fibrin-, or gelatin-based utilize an extrusion-based bioprinter, such as a coaxial and/or a microfluidic bioprinter. On the other hand, studies using gelatin-methacrylate (GelMA) bioinks utilize a digital light processing-based (DLP-based) bioprinter. This bioprinter projects a still image using light that causes the bioink to gel in the projected pattern.31,85 In summary, the ability to accurately replicate the in vivo characteristics of GBM 3D models in vitro is dependent on the appropriate integration of bioinks, cellular components, and mechanical properties.17,31,83
Skin cancer
Accurate and practical model systems are crucial aspects to be considered when developing potential skin cancer treatments. Animal models are limited by their ethical concerns and accuracy. Every different skin region of an animal's body presents some differences among them in terms of quantity and thickness of the dermis and epidermis layers, which differ when compared to similar regions of the human body.89 The ex vivo alternative is restricted by the availability of living tissue. 2D cultures are anchorage-dependent systems where the cells grow attached to a surface, and despite their simplicity, the cells are restricted by their incapacity to mimic the 3D in vivo structure and behavior. Moreover, cell stratification and differentiation are poor and they may show hyperproliferative growth, making 2D cultures a poor choice for studying skin biology. Several important signaling pathways function only when the cells are placed in a 3D structure. In vitro 3D models, on the other hand, provide an in vivo like structure containing human skin cells and components of the ECM like collagen.19–21,90–92
Then, recent advances in tissue engineering have provided novel models to study skin cancer treatments in a more reliable fashion. The human skin in general is divided histologically into the epidermis, the dermis, and the hypodermis. The epidermis consists of the basal cell layer (stratum basale), the deepest sublayer of the epidermis composed of basal keratinocytes (stem cells), which renews the strata above and starts SCC when there is an accumulation of dysplastic keratinocytes; the stratum spinosum, the prickle cell layer above basal cells that make their shape somewhat flatter; the stratum granulosum, granular cells where cornification or keratinization of keratinocytes begins; the stratum lucidum: it can only be found in soles and palms, where cells become flatter and more densely packed during turn-over; stratum corneum, corneocytes (mortal differentiation of keratinocytes) responsible for the barrier function of the skin that are constantly renewed.93 The dermis has a rich supply of blood vessels.94 Sebaceous glands, sweat glands, and hair follicles rise to the surface of the skin from the dermis and subcutaneous layer where they originate. The dermis has the following sublayers: the papillary layer, the upper sublayer of the dermis, loosely connected tissue and includes a large number of nerve fibers, capillaries, water and fibroblasts. Collagen fibers form a finer network than those of the reticular layer; the reticular layer is the lower part of the dermis that undergoes continuous transition to the hypodermis. Hypodermis (or subcutis) is an elastic layer and includes a large number of fat cells that work as a shock absorber for blood vessels and nerve endings. However, the actual thickness differs from person to person, along the age, and it also depends on each body region.93Then, real and standardized construction of the skin layers and of the skin cancer is possible using 3D bioprinting and human cells, and it is crucial to have accuracy and more practical model systems.95,96 However, engineering these complex multi-layered, multicellular anatomic structures of the skin as realistic as possible is technically challenging. In order to promote the physiological conditions of epidermal differentiation (keratinocyte differentiation into corneocytes for stratum corneum formation), the 3D bioprinted skin tissue97 (and in 3D non-printed models as well) is raised to the air–liquid interface (ALI). In contrast to ordinary immersed cultures, ALI culture cells in some layers of the 3D bioprinted construct are grown on a basement membrane, that is raised to the air–medium interface after eligible culture periods, resulting in exposure to air. For the modelling of skin equivalents, this technique is the gold standard. These equivalents offer high-end complexity and provide physiological behaviour regarding the interplay of fibroblasts and keratinocytes, resulting in an abundant case of use for modelling cancer.98 Carcinoma cell lines, for instance, can be grown as pure cultures at an air–liquid interface to simulate a carcinoma in situ, or they can be grown as mixtures with normal keratinocytes to mimic an earlier stage of epithelial dysplasia. The reason is that the ALI accelerates the invasive growth of cutaneous squamous cell carcinomas relative to invasive growth under submerged conditions. The ALI is thus considered to be a critical factor for both normal and neoplastic growth of skin cells.99
3D culture models that reflect the architecture and cellular composition of a tumor are essential in immuno-oncology studies. Nowadays, there are several 3D culture methods of skin cancer such as spheroids and organoids, which are applicable to immuno-oncology.72 To further drug discovery and improve clinical translation, a 3D human melanoma model was developed as an alternative to animal testing, based on primary human skin cells and melanoma cell lines (A375, Malme 3M, RPMI 7951 and SK-MEL 28 from ATCC) while including a key feature for tumor progression: blood and lymphatic capillaries. Chronic treatment with vemurafenib was applied to the model and elicited a dose-dependent response on proliferation and apoptosis of tumor cells, making it a promising tool to test new compounds in a human-like environment.91 For an accurate evaluation of potential treatment efficacy, it is important to study 3D models as close as possible to the in vivo conditions. Then a 3D model of B16F10 spheroids was used. The behavior of a well-known cytostatic, doxorubicin (DOX), was evaluated in spheroids as compared to classical 2D culture conditions. It was confirmed that a much higher DOX concentration is necessary to produce similar effects compared with the monolayer. The 3D model developed in this study was suitable to investigate drug penetration in time. Those findings may explain the decrease of the doxorubicin therapeutic effect, suggesting the need for maintaining the drug concentration at the tumoral place for at least 2 h upon administration.90
3D bioprinting skin models are very much needed for the testing of drugs and cosmetics and to investigate cancer skin, in view of bans being imposed on product testing on animals. This has been mainly motivated by the unprecedented ability of these technologies in depositing cells, biomaterials, and bioactive molecules in predefined 3D locations with high precision and reproducibility. Given this greater need, 3D bioprinting is a promising technology that can achieve rapid and reliable production of biomimetic cellular skin substitutes, satisfying both clinical and industrial needs.95,96 The analysis of the skin cancer complexity is an important parameter to consider, not only in the search for new therapies, but also for the patients’ identification and stratification likely to respond to immunotherapy. 3D bioprinting cancer models exhibit varying levels of biological function and complexity at structural, material, and cellular levels, and have been fabricated using different bioprinting technologies including vat polymerisation, inkjet, laser-assisted, and extrusion. There are different types of cell lines, such as fibroblasts, endothelial cells, adipocytes, pericytes, stem cells, induced pluripotent stem cells (iPSCs), melanocytes, and keratinocytes.25 A major advantage of bioprinting skin models relies on the ability to closely mimic the architecture of the native skin through the deposition of bioinks comprised of skin cells and bioinstructive materials into a 3D construct with a tissue-specific organisation. It has been demonstrated that increasing the complexity and biomimicry of bioprinted skin translates into improved biological function, predictive value, and healing ability.100
The feasibility of skin bioprinting has been demonstrated not only in vitro but also in situ by pre-clinical studies showing that the deposition of cells (stem cells, fibroblasts and/or keratinocytes) directly onto the wound bed of mouse or porcine models stimulates healing.101,102 However, the reported achievements in bioprinting diseased skin models are more modest so far. To engineer a realistic TME that can recapitulate not only cancer progression but also angiogenesis and metastasis, many complex interacting factors must be considered. Strategies based on 3D bioprinting are now being investigated, which could simulate the TME by bioprinting living human cells. These approaches allow the accurate placement of normal cancer cells and bioactive macromolecules to monitor cancer progression, facilitate drug screening, and provide the design of new generations of anticancer therapies.62
Advantages of 3D bioprinted cancer models
Cancer research has shown significant improvements in recent years. The ability to detect the disease early and the methods used to treat cancer have been revamped. Nevertheless, the demand for customized therapies has grown over time given that each patient has a distinct set of inter-patient variations in terms of prognosis, clinical outcomes, and reactions.32 Due to the recent advancements, the need for preclinical models that can diagnose each patient with a personalized regime has grown rapidly. In recent years, 3D bioprinting has been utilized for the progression of improved cancer research cellular models as detailed in this review.103 With the use of 3D bioprinting, researchers may simulate the disease in a lab setting using models created from cancer cells taken from actual patients. The ability to automate the fabrication of these 3D bioprinted models while maintaining high repeatability and reliability matches up well with scalable production. The long-term objective of 3D bioprinting is the layer-by-layer fabrication of highly functional 3D tissue-engineered constructs. As a result, crucial cell–cell and cell–ECM interactions would be stimulated, and the complex ECM structures found in 3D tissue-engineered constructs would be mimicked. Despite being in its infancy, 3D bioprinting has a lot of potential for automating the fabrication of extremely complex 3D tissue constructs in a scalable and repeatable manner. Some of the bioprinting methods used to create 3D cancer models include extrusion-based, laser-based, and droplet-based. Each form of technology has its own set of benefits, and its utilization is determined by how well it matches the demands of the tissue being produced.84 Although each type of technology has its own feature, a commonality they all tend to share is the platform they have provided which has been crucial for the study of cancer pathology and biology. Moreover, these technologies have permitted the screening of anticancer drugs, have the potential to address a variety of medical research problems and have been used in various areas, including functional organ replacement, regenerative medicine, and drug delivery.103 Additionally, 3D bioprinted cancer constructs have proved to present the physiological cancer tissues better when it comes to the microenvironment of the tumor and cellular behavior with the characteristic spatial distribution of cells.32,60
3D bioprinting enables the creation of artificial culture environments with a specialized spatial arrangement of specific cell types. The biophysical properties of the tissue can also be fine-tuned by adjusting the porosity, stiffness, and biochemical properties of the ECM.84 Chen et al. showed that a 3D porous model promoted the formation of tumor cell spheroids compared to 2D models, and it also immensely increased the invasiveness and chemotherapeutic resistance of tumor cells.77 Compared to 2D models, 3D cancer models can better mimic the TME which includes metastasis, anti-cancer drug resistance, and angiogenesis.84 This is possible since the 3D constructs can accomplish biophysical characteristics which are like the microenvironment of the native tissue, which allows for the study of tumorigenesis and cancer progression. Also, the 3D construct itself degrades over time as cells secrete the native ECM, which supports the original bioprinted structure. Moreover, in relation to the study of cell–cell and cell–matrix interactions, 3D bioprinting provides a stronger solution by yielding biomimetic microenvironments.35,84 Furthermore, 3D bioprinted models may include multiple cell types which are capable of secreting the ECM, such as cancer and normal cells related to the microenvironment of the tumor. It allows for the formation of vessel-like structures which are vital to study the metastatic process and evaluate anti-cancer drug delivery and responses. It also helps modulate the composition of the exogenous ECM which consists of numerous growth factors or signalling molecules and the inorganic matrix.104,105 Furthermore, 3D cancer modelling allows for the use of human immune cells, rather than using traditional animal models. For example, cancer models can be created with patient derived xenografts using mice with deficient immunity, however, this work is time consuming, laborious, and the difference in microenvironments between mice and humans must be taken into consideration.106 Creating a 3D cancer model with human immune cells is a great advantage over traditional animal models using immunocompromised mice.28
Challenges of 3D bioprinted models
First and foremost, an important factor of 3D bio-modelling is that all materials used need to be biocompatible and sterile. These factors may seem trivial to mention, however, this limits the number of materials that one can choose from when bioprinting. For example, polymers are frequently used in 3D bioprinting. However, many polymers have limited ways to be sterilized.39 This necessity leads to restricted options when it comes to what materials can be used to fabricate 3D bioprinted cancer models. Furthermore, there are different techniques for creating 3D cancer models, and this can be considered another limitation. Since there is no standardized method, there are challenges when it comes to the comparison and reproducibility of results. There are an increasingly large number of methods to choose from, and the choice is often made depending on the intended application of the 3D model.50 Finally, the ability to observe and oversee cells in real time is currently unavailable and acquiring this capability would enable researchers to monitor 3D cancer models more closely. The variability of 3D cancer models presents a challenge as it limits the standardization, reproducibility, and ability to use the models as tools for drug development.26 Furthermore, the ability to vascularize 3D cancer models remains one of the foremost limitations in this field of study. This is a limitation for almost all 3D cancer models.37 Vascularization is critical for cell proliferation and metastasis in tumor tissues.66 Moreover, there are several other challenges associated with creating heterogeneous cancer constructs, including the isolation of a significant number of cells, maintaining the heterogeneity of primary cells, bioprinting, and rapidly conducting drug testing that will enable the selection of the most suitable chemotherapy. In most cases, the development of 3D cancer models is commonly accomplished using patient derived cells. However, these cells are accustomed to the native environment from which they are obtained. This tumor environment is specific to each patient, and therefore using patient derived cells is often better for creating personalized therapeutics rather than the development of a generalized 3D cancer model using cell lines. Additionally, the use of immortalized cell lines poses an issue since these cells are manipulated over a long period of time, which results in very high passage numbers.28
Conclusions
The creation and application of 3D bioprinting to produce heterogeneous and intricate tumor models for drug screening, therapeutic interventions, and cancer diagnosis were reviewed in this article. 3D bioprinting has catalysed the in vitro development of a high throughput TME. The integration of precisely tailored biomaterials, 3D biofabrication technologies, and computational tools might lead to the development of improved integrated 3D in vitro platforms. Biomaterials may be mixed to create composite bioinks with more realistic, adaptable, and customizable microphysical properties, which better imitate the distinctive aspects of in vivo tumors, such as mechanical signals, which in turn affect cell responsiveness to therapy. 3D bioprinting technology can provide a level of customization, architectural control, and biomimicry that cannot be achieved with other 3D cell culture methods. Overall, 3D bioprinting may open the door to a revolutionary, yet effective, morphodynamical hallmark of tumor severity and a novel biophysical approach to predicting the tumor spreading potential.
Author contributions
Conceptualization: R. S. and S. M. W.; project administration: R. S. and M. R. P.; writing – original draft: R. S., M. R. P., V. A. S., J. T., L. B., T. A., and A. A.; writing – review and editing: R. S. and S. M. W.
Conflicts of interest
S. M. W. is the co-founder and C. E. O. of Axolotl Biosciences.
Acknowledgements
S. M. W. acknowledges the funding from the Canada Research Chairs Program, the UVIC Health Research Grant program, the Natural Sciences and Engineering Research Council, and the Canadian Institutes of Health Research. R. S. acknowledges support from MITACS, and T. A. acknowledges funding from FAPESP.
References
- H. Nagai and Y. H. Kim, Cancer prevention from the perspective of global cancer burden patterns, J. Thorac. Dis., 2017, 9(3), 448–451, DOI:10.21037/jtd.2017.02.75
.
- Y. Sbirkov,
et al., A Colorectal Cancer 3D Bioprinting Workflow as a Platform for Disease Modeling and Chemotherapeutic Screening, Front. Bioeng. Biotechnol., 2021, 9, 755563, DOI:10.3389/fbioe.2021.755563
.
- E. Maloney,
et al., Immersion Bioprinting of Tumor Organoids in Multi-Well Plates for Increasing Chemotherapy Screening Throughput, Micromachines, 2020, 11(2), 208, DOI:10.3390/mi11020208
.
-
Release notice - Canadian Cancer Statistics, Health Promot. Chronic Dis Prev. Can., 2021, 41, 11, pp. 399–399 Search PubMed.
- R. L. Siegel, K. D. Miller, H. E. Fuchs and A. Jemal, Cancer statistics, 2022, CA Cancer J. Clin., 2022, 72(1), 7–33, DOI:10.3322/caac.21708
.
- H. Sung,
et al., Global Cancer Statistics 2020: GLOBOCAN Estimates of Incidence and Mortality Worldwide for 36 Cancers in 185 Countries, CA Cancer J. Clin., 2021, 71(3), 209–249, DOI:10.3322/caac.21660
.
- F. Bray, J. Ferlay, I. Soerjomataram, R. L. Siegel, L. A. Torre and A. Jemal, Global cancer statistics 2018: GLOBOCAN estimates of incidence and mortality worldwide for 36 cancers in 185 countries, CA Cancer J. Clin., 2018, 68(6), 394–424, DOI:10.3322/caac.21492
.
- X. Wang,
et al., Tumor-like lung cancer model based on 3D bioprinting, 3 Biotech, 2018, 8(12), 501, DOI:10.1007/s13205-018-1519-1
.
- N. Wake,
et al., Patient-specific 3D printed and augmented reality kidney and prostate cancer models: impact on patient education, 3D Print. Med., 2019, 5(1), 4, DOI:10.1186/s41205-019-0041-3
.
- K. Xu, Y. Han, Y. Huang, P. Wei, J. Yin and J. Jiang, The application of 3D bioprinting in urological diseases, Mater. Today Bio, 2022, 16, 100388, DOI:10.1016/j.mtbio.2022.100388
.
- M. Y. Chen, J. Skewes, M. A. Woodruff, P. Dasgupta and N. J. Rukin, Multi-colour extrusion fused deposition modelling: a low-cost 3D printing method for anatomical prostate cancer models, Sci. Rep., 2020, 10(1), 10004, DOI:10.1038/s41598-020-67082-7
.
- P. Rawla, Epidemiology of Prostate Cancer, World J. Oncol., 2019, 10(2), 63–89, DOI:10.14740/wjon1191
.
- K. Bromma,
et al., Enhancing nanoparticle accumulation in two dimensional, three dimensional, and xenograft mouse cancer cell models in the presence of docetaxel, Sci. Rep., 2022, 12(1), 13508, DOI:10.1038/s41598-022-17752-5
.
- H. Suzuki,
et al., Hormonal therapy for prostate cancer: current topics and future perspectives, Int. J. Urol., 2010, 17(4), 302–313, DOI:10.1111/j.1442-2042.2010.02460.x
.
- F. G. Davis, T. R. Smith, H. R. Gittleman, Q. T. Ostrom, C. Kruchko and J. S. Barnholtz-Sloan, Glioblastoma incidence rate trends in Canada and the United States compared with England, 1995-2015, Neuro-Oncology, 2020, 22(2), 301–302, DOI:10.1093/neuonc/noz203
.
- A. Shergalis, A. Bankhead 3rd, U. Luesakul, N. Muangsin and N. Neamati, Current Challenges and Opportunities in Treating Glioblastoma, Pharmacol. Rev., 2018, 70(3), 412–445, DOI:10.1124/pr.117.014944
.
- C. Lee, E. Abelseth, L. de la Vega and S. M. Willerth, Bioprinting a novel glioblastoma tumor model using a fibrin-based bioink for drug screening, Mater. Today Chem., 2019, 12, 78–84, DOI:10.1016/j.mtchem.2018.12.005
.
- S. Han,
et al., 3D Bioprinted Vascularized Tumour for Drug Testing, Int. J. Mol. Sci., 2020, 21(8), 2993, DOI:10.3390/ijms21082993
.
- M. D. Gober, H. M. Bashir and J. T. Seykora, Reconstructing skin cancers using animal models, Cancer Metastasis Rev., 2013, 32(1–2), 123–128, DOI:10.1007/s10555-012-9410-8
.
- D. S. Hill,
et al., A Novel Fully Humanized 3D Skin Equivalent to Model Early Melanoma Invasion, Mol. Cancer Ther., 2015, 14(11), 2665–2673, DOI:10.1158/1535-7163.MCT-15-0394
.
- V. Samarasinghe and V. Madan, Nonmelanoma skin cancer, J. Cutan. Aesthet. Surg., 2012, 5(1), 3–10, DOI:10.4103/0974-2077.94323
.
- L. van der Weyden, T. Brenn, E. E. Patton, G. A. Wood and D. J. Adams, Spontaneously occurring melanoma in animals and their relevance to human melanoma, J. Pathol., 2020, 252(1), 4–21, DOI:10.1002/path.5505
.
- A. Padhye and J. D'Souza, Oral malignant melanoma: A silent killer?, J. Indian Soc. Periodontol., 2011, 15(4), 425–428, DOI:10.4103/0972-124X.92587
.
- G. Emri,
et al., Ultraviolet radiation-mediated development of cutaneous melanoma: An update, J. Photochem. Photobiol., B, 2018, 185, 169–175, DOI:10.1016/j.jphotobiol.2018.06.005
.
- S. Fernandes, C. Vyas, P. Lim, R. F. Pereira, A. Virós and P. Bártolo, 3D Bioprinting: An Enabling Technology to Understand Melanoma, Cancers, 2022, 14, 3535, DOI:10.3390/cancers14143535
.
- M. A. G. Barbosa, C. P. R. Xavier, R. F. Pereira, V. Petrikaite and M. H. Vasconcelos, 3D Cell Culture Models as Recapitulators of the Tumor Microenvironment for the Screening of Anti-Cancer Drugs, Cancers, 2022, 14(1), 190, DOI:10.3390/cancers14010190
.
- R. Curvello, V. Kast, M. H. Abuwarwar, A. L. Fletcher, G. Garnier and D. Loessner, 3D Collagen-Nanocellulose Matrices Model the Tumour Microenvironment of Pancreatic Cancer, Front Digit. Health, 2021, 3, 704584, DOI:10.3389/fdgth.2021.704584
.
- P. Datta, M. Dey, Z. Ataie, D. Unutmaz and I. T. Ozbolat, 3D bioprinting for reconstituting the cancer microenvironment, npj Precis. Oncol., 2020, 4, 18, DOI:10.1038/s41698-020-0121-2
.
- F. Fontana, M. Marzagalli, M. Sommariva, N. Gagliano and P. Limonta, In Vitro 3D Cultures to Model the Tumor Microenvironment, Cancers, 2021, 13(12), 2970, DOI:10.3390/cancers13122970
.
- J. M. Grolman, D. Zhang, A. M. Smith, J. S. Moore and K. A. Kilian, Rapid 3D Extrusion of Synthetic Tumor Microenvironments, Adv. Mater., 2015, 27(37), 5512–5517, DOI:10.1002/adma.201501729
.
- M. Tang,
et al., Three-dimensional bioprinted glioblastoma microenvironments model cellular dependencies and immune interactions, Cell Res., 2020, 30(10), 833–853, DOI:10.1038/s41422-020-0338-1
.
- R. Augustine,
et al., 3D Bioprinted cancer models: Revolutionizing personalized cancer therapy, Transl. Oncol., 2021, 14(4), 101015, DOI:10.1016/j.tranon.2021.101015
.
- D. F. Duarte Campos, A. Bonnin Marquez, C. O'Seanain, H. Fischer, A. Blaeser, M. Vogt, D. Corallo and S. Aveic, Exploring Cancer Cell Behavior In Vitro in Three-Dimensional Multicellular Bioprintable Collagen-Based Hydrogels, Cancers, 2019, 11(2), 180, DOI:10.3390/cancers11020180
.
- G. Imparato, F. Urciuolo and P. A. Netti, Organ on Chip Technology to Model Cancer Growth and Metastasis, Bioengineering, 2022, 9, 28, DOI:10.3390/bioengineering9010028
.
- R. Staros, A. Michalak, K. Rusinek, K. Mucha, Z. Pojda and R. Zagozdzon, Perspectives for 3D-Bioprinting in Modeling of Tumor Immune Evasion, Cancers, 2022, 14(13), 3126, DOI:10.3390/cancers14133126
.
- M. Pickl and C. H. Ries, Comparison of 3D and 2D tumor models reveals enhanced HER2 activation in 3D associated with an increased response to trastuzumab, Oncogene, 2008, 28(3), 461–468, DOI:10.1038/onc.2008.394
.
- D. Caballero, S. Kaushik, V. M. Correlo, J. M. Oliveira, R. L. Reis and S. C. Kundu, Organ-on-chip models of cancer metastasis for future personalized medicine: From chip to the patient, Biomaterials, 2017, 149, 98–115, DOI:10.1016/j.biomaterials.2017.10.005
.
- Q. R. Guo,
et al., Multifunctional microfluidic chip for cancer diagnosis and treatment, Nanotheranostics, 2021, 5(1), 73–89, DOI:10.7150/ntno.49614
.
- R. Li, Y. H. Ting, S. H. Youssef, Y. Song and S. Garg, Three-Dimensional Printing for Cancer Applications: Research Landscape and Technologies, Pharmaceuticals, 2021, 14(8), 787, DOI:10.3390/ph14080787
.
- D. Murata, K. Arai and K. Nakayama, Scaffold-Free Bio-3D Printing Using Spheroids as Bio-Inks for Tissue (Re-)Construction and Drug Response Tests, Adv. Healthc. Mater., 2020, 9(15), e1901831, DOI:10.1002/adhm.201901831
.
- M. Bydon,
et al., CELLTOP Clinical Trial: First Report From a Phase 1 Trial of Autologous Adipose Tissue-Derived Mesenchymal Stem Cells in the Treatment of Paralysis Due to Traumatic Spinal Cord Injury, Mayo Clin. Proc., 2020, 95(2), 406–414, DOI:10.1016/j.mayocp.2019.10.008
.
- F. Conforti, L. Pala, T. De Pas, Y. He and G. Giaccone, Investigational drugs for the treatment of thymic cancer: a focus on phase 1 and 2 clinical trials, Expert Opin. Invest. Drugs, 2022, 31(9), 895–904, DOI:10.1080/13543784.2022.2113373
.
- R. K. Jain,
et al., Phase I oncology studies: evidence that in the era of targeted therapies patients on lower doses do not fare worse, Clin. Cancer Res., 2010, 16(4), 1289–1297, DOI:10.1158/1078-0432.CCR-09-2684
.
- D. J. Martini,
et al., Clinical outcomes of advanced stage cancer patients treated with sequential immunotherapy in phase 1 clinical trials, Invest. New Drugs, 2019, 37(6), 1198–1206, DOI:10.1007/s10637-019-00736-0
.
- P. Petrou,
et al., Safety and Clinical Effects of Mesenchymal Stem Cells Secreting Neurotrophic Factor Transplantation in Patients With Amyotrophic Lateral Sclerosis: Results of Phase 1/2 and 2a Clinical Trials, JAMA Neurol., 2016, 73(3), 337–344, DOI:10.1001/jamaneurol.2015.4321
.
- E. Tomas-Bort, M. Kieler, S. Sharma, J. B. Candido and D. Loessner, 3D approaches to model the tumor microenvironment of pancreatic cancer, Theranostics, 2020, 10(11), 5074–5089, DOI:10.7150/thno.42441
.
- D. Wu, J. Berg, B. Arlt, V. Röhrs, M. A. Al-Zeer, H. E. Deubzer and J. Kurreck, Bioprinted Cancer Model of Neuroblastoma in a Renal Microenvironment as an Efficiently Applicable Drug Testing Platform, Int. J. Mol. Sci., 2022, 23(1), 122, DOI:10.3390/ijms23010122
.
- T. Liu, C. Delavaux and Y. S. Zhang, 3D bioprinting for oncology applications, J. 3D Print. Med., 2019, 3(2), 55–58, DOI:10.2217/3dp-2019-0004
.
- T. Liu, 3D bioprinting for oncology applications, J. 3D Print. Med., 2019, 3(2), 55–58 CrossRef PubMed
.
- M. E. Katt, A. L. Placone, A. D. Wong, Z. S. Xu and P. C. Searson, In Vitro Tumor Models: Advantages, Disadvantages, Variables, and Selecting the Right Platform, Front. Bioeng. Biotechnol., 2016, 4, 12, DOI:10.3389/fbioe.2016.00012
.
- S. A. Langhans, Three-Dimensional in Vitro Cell Culture Models in Drug Discovery and Drug Repositioning, Front. Pharmacol., 2018, 9, 6, DOI:10.3389/fphar.2018.00006
.
- W. Mueller-Klieser, Multicellular spheroids. A review on cellular aggregates in cancer research, J. Cancer Res. Clin. Oncol., 1987, 113(2), 101–122, DOI:10.1007/BF00391431
.
- S. Nath and G. R. Devi, Three-dimensional culture systems in cancer research: Focus on tumor spheroid model, Pharmacol. Ther., 2016, 163, 94–108, DOI:10.1016/j.pharmthera.2016.03.013
.
- R. Foty, A simple hanging drop cell culture protocol for generation of 3D spheroids, J. Visualized Exp., 2011, 51 DOI:10.3791/2720
.
- S. Sathaye,
et al., Rheology of peptide- and protein-based physical hydrogels: are everyday measurements just scratching the surface?, Wiley Interdiscip. Rev.: Nanomed. Nanobiotechnol., 2015, 7(1), 34–68, DOI:10.1002/wnan.1299
.
- J. Borlak, P. K. Singh and I. Rittelmeyer, Regulation of Liver Enriched Transcription Factors in Rat Hepatocytes Cultures on Collagen and EHS Sarcoma Matrices, PLoS One, 2015, 10(4), e0124867, DOI:10.1371/journal.pone.0124867
.
- C. S. Hughes, L. M. Postovit and G. A. Lajoie, Matrigel: a complex protein mixture required for optimal growth of cell culture, Proteomics, 2010, 10(9), 1886–1890, DOI:10.1002/pmic.200900758
.
- S. Koutsopoulos and S. Zhang, Long-term three-dimensional neural tissue cultures in functionalized self-assembling peptide hydrogels, matrigel and collagen I, Acta Biomater., 2013, 9(2), 5162–5169, DOI:10.1016/j.actbio.2012.09.010
.
- J. Pape, M. Emberton and U. Cheema, 3D Cancer Models: The Need for a Complex Stroma, Compartmentalization and Stiffness, Front. Bioeng. Biotechnol., 2021, 9, 660502, DOI:10.3389/fbioe.2021.660502
.
- R. Augustine, A. H. Aqel, S. N. Kalva, K. S. Joshy, A. Nayeem and A. Hasan, Bioengineered microfluidic blood-brain barrier models in oncology research, Transl. Oncol., 2021, 14(7), 101087, DOI:10.1016/j.tranon.2021.101087
.
- A. G. Niculescu, C. Chircov, A. C. Bîrcă and A. M. Grumezescu, Fabrication and Applications of Microfluidic Devices: A Review, Int. J. Mol. Sci., 2021, 22(4), 2011, DOI:10.3390/ijms22042011
.
- H. Samadian, S. Jafari, M. R. Sepand, L. Alaei, S. Sadegh Malvajerd, M. Jaymand, F. Ghobadinezhad, F. Jahanshahi, M. R. Hamblin, H. Derakhshankhah and Z. Izadi, 3D bioprinting technology to mimic the tumor microenvironment: tumor-on-a-chip concept, Mater. Today Adv., 2021, 12, 100160, DOI:10.1016/j.mtadv.2021.100160
.
- K. Ling,
et al., Bioprinting-Based High-Throughput Fabrication of Three-Dimensional MCF-7 Human Breast Cancer Cellular Spheroids, Engineering, 2015, 1(2), 269–274, DOI:10.15302/j-eng-2015062
.
- W. Zhu, B. Holmes, R. I. Glazer and L. G. Zhang, 3D printed nanocomposite matrix for the study of breast cancer bone metastasis, Nanomedicine, 2016, 12(1), 69–79, DOI:10.1016/j.nano.2015.09.010
.
- C. Mazzaglia, Y. Sheng, L. N. Rodrigues, I. M. Lei, J. D. Shields and Y. Y. S. Huang, Deployable extrusion bioprinting of compartmental tumoroids with cancer associated fibroblasts for immune cell interactions, Biofabrication, 2023, 15(2), 025005, DOI:10.1088/1758-5090/acb1db
.
- Y. Kang, P. Datta, S. Shanmughapriya and I. T. Ozbolat, 3D Bioprinting of Tumor Models for Cancer Research, ACS Appl. Bio Mater., 2020, 3(9), 5552–5573, DOI:10.1021/acsabm.0c00791
.
- W. Wan, Z. Li, X. Wang, F. Tian and J. Yang, Surface-Fabrication of Fluorescent Hydroxyapatite for Cancer Cell Imaging and Bio-Printing Applications, Biosensors, 2022, 12(6), 419 CrossRef CAS PubMed
. [Online]. Available: https://www.mdpi.com/2079-6374/12/6/419.
- T. Q. Huang, X. Qu, J. Liu and S. Chen, 3D printing of biomimetic microstructures for cancer cell migration, Biomed. Microdevices, 2013, 16(1), 127–132, DOI:10.1007/s10544-013-9812-6
.
- Z. Kanaki,
et al., Laser-Induced Forward Transfer Printing on Microneedles for Transdermal Delivery of Gemcitabine, Int. J. Bioprint., 2022, 8(2), 554, DOI:10.18063/ijb.v8i2.554
.
- R. Seyedmahmoud, M. J. Messler and E. G. Loboa, 3D Bioprinting Technologies for Tissue Engineering: A Mini Review, J. Stem Cells Res. Dev. Ther., 2020, 6, 046 Search PubMed
.
- N. Germain, M. Dhayer, S. Dekiouk and P. Marchetti, Current Advances in 3D Bioprinting for Cancer Modeling and Personalized Medicine, Int. J. Mol. Sci., 2022, 23(7), 3432, DOI:10.3390/ijms23073432
.
- N. Boucherit, L. Gorvel and D. Olive, 3D Tumor Models and Their Use for the Testing of Immunotherapies, Front. Immunol., 2020, 11, 603640, DOI:10.3389/fimmu.2020.603640
.
- J. Ye,
et al., Preparation and characterization of gelatin-polysaccharide composite hydrogels for tissue engineering, PeerJ, 2021, 9, e11022, DOI:10.7717/peerj.11022
.
- J. Coles-Black, S. Ong and J. Teh,
et al., 3D printed patient-specific prostate cancer models to guide nerve-sparing
robot-assisted radical prostatectomy: a systematic review, J. Rob. Surg., 2023, 17, 1–10, DOI:10.1007/s11701-022-01401-0
.
- K. Bromma, A. Alhussan, M. Mesa Perez, P. Howard, W. Beckham and D. B. Chithrani, Three-dimensional Tumor Spheroids as a Tool for Reliable Investigation of Combined Gold Nanoparticle and Docetaxel Treatment, Cancers, 2021, 13, 1465, DOI:10.3390/cancers13061465
.
- B. M. Holzapfel,
et al., Species-specific homing mechanisms of human prostate cancer metastasis in tissue engineered bone, Biomaterials, 2014, 35(13), 4108–4115, DOI:10.1016/j.biomaterials.2014.01.062
.
- H. Chen,
et al., 3D printed in vitro tumor tissue model of colorectal cancer, Theranostics, 2020, 10(26), 12127–12143, DOI:10.7150/thno.52450
.
- Y. Zhang,
et al., 3D Bioprinted GelMA-Nanoclay Hydrogels Induce Colorectal Cancer Stem Cells Through Activating Wnt/beta-Catenin Signaling, Small, 2022, 18(18), e2200364, DOI:10.1002/smll.202200364
.
- M. A. Hermida,
et al., Three dimensional in vitro models of cancer: Bioprinting multilineage glioblastoma models, Adv. Biol. Regul., 2020, 75, 100658, DOI:10.1016/j.jbior.2019.100658
.
- I. P. M. Smits, O. W. Blaschuk and S. M. Willerth, Novel N-cadherin antagonist causes glioblastoma cell death in a 3D bioprinted co-culture model, Biochem. Biophys. Res. Commun., 2020, 529(2), 162–168, DOI:10.1016/j.bbrc.2020.06.001
.
- M. Ozaki,
et al., Evaluation of the immunogenicity of human iPS cell-derived neural stem/progenitor cells in vitro, Stem Cell Res., 2017, 19, 128–138, DOI:10.1016/j.scr.2017.01.007
.
- N. Dusserre, M.-L. Stachowicz, C. Medina, B. Henri and J.-C. Fricain,
et al., Microvalve bioprinting as a biofabrication tool to decipher tumor and endothelial cell crosstalk: Application to a simplified glioblastoma model, Bioprinting, 2021, 24, e00178, DOI:10.1016/j.bprint.2021.e00178
.
- M. A. Heinrich, R. Bansal, T. Lammers, Y. S. Zhang, R. Michel Schiffelers and J. Prakash, 3D–Bioprinted Mini–Brain: A Glioblastoma Model to Study Cellular Interactions and Therapeutics, Adv. Mater., 2019, 31(14), 1806590, DOI:10.1002/adma.201806590
.
- S. W. Leong, S. C. Tan, M. N. Norhayati, M. Monif and S. Y. Lee, Effectiveness of Bioinks and the Clinical Value of 3D Bioprinted Glioblastoma Models: A Systematic Review, Cancers, 2022, 14(9), 2149, DOI:10.3390/cancers14092149
.
- M. Tang,
et al., Rapid 3D Bioprinting of Glioblastoma Model Mimicking Native Biophysical Heterogeneity, Small, 2021, 17(15), e2006050, DOI:10.1002/smll.202006050
.
- X. Wang,
et al., Coaxial extrusion bioprinted shell-core hydrogel microfibers mimic glioma microenvironment and enhance the drug resistance of cancer cells, Colloids Surf., B, 2018, 171, 291–299, DOI:10.1016/j.colsurfb.2018.07.042
.
- X. Wang,
et al., Bioprinting of glioma stem cells improves their endotheliogenic potential, Colloids Surf., B, 2018, 171, 629–637, DOI:10.1016/j.colsurfb.2018.08.006
.
- X. Wang,
et al., 3D bioprinted glioma microenvironment for glioma vascularization, J. Biomed. Mater. Res., Part A, 2021, 109(6), 915–925, DOI:10.1002/jbm.a.37082
.
- F. M. Hendriks, D. Brokken, C. W. Oomens, D. L. Bader and F. P. Baaijens, The relative contributions of different skin layers to the mechanical behavior of human skin in vivo using suction experiments, Med. Eng. Phys., 2006, 28(3), 259–266, DOI:10.1016/j.medengphy.2005.07.001
.
- D. D. Baciu, A. M. Dumitrascu, V. Vasile, B. Palade and A. Salageanu, Generation of a 3D melanoma model and visualization of doxorubicin uptake by fluorescence imaging, In Vitro Cell. Dev. Biol.: Anim., 2022, 58(1), 44–53, DOI:10.1007/s11626-021-00636-9
.
- J. Bourland, J. Fradette and F. A. Auger, Tissue-engineered 3D melanoma model with blood and lymphatic capillaries for drug development, Sci. Rep., 2018, 8(1), 13191, DOI:10.1038/s41598-018-31502-6
.
- D. A. Monico, I. R. Calori, C. Souza, E. M. Espreafico, H. Bi and A. C. Tedesco, Melanoma spheroid-containing artificial dermis as an alternative approach to in vivo models, Exp. Cell Res., 2022, 417(1), 113207, DOI:10.1016/j.yexcr.2022.113207
.
-
S. K. Nayar, K. Nishino and T. Igarashi, The Appearance of Human Skin: A Survey, in Foundations and Trends® in Computer Graphics and Vision, 2007, vol. 3(1), pp. 1–95, DOI:10.1561/0600000013
.
- S. N. Andrews, E. Jeong and M. R. Prausnitz, Transdermal delivery of molecules is limited by full epidermis, not just stratum corneum, Pharm. Res., 2013, 30(4), 1099–1109, DOI:10.1007/s11095-012-0946-7
.
- S. Ramasamy, P. Davoodi, S. Vijayavenkataraman, J. H. Teoh, A. M. Thamizhchelvan, K. S. Robinson, B. Wu, J. Y. H. Fuh, T. DiColandrea, H. Zhao, E. B. Lane and C. H. Wang, Optimized construction of a full thickness human skin equivalent using 3D bioprinting and a PCL/collagen dermal scaffold, Bioprinting, 2021, 21, e00123, DOI:10.1016/j.bprint.2020.e00123
.
- W. C. Yan,
et al., 3D bioprinting of skin tissue: From pre-processing to final product evaluation, Adv. Drug Delivery Rev., 2018, 132, 270–295, DOI:10.1016/j.addr.2018.07.016
.
- V. Lee,
et al., Design and fabrication of human skin by three-dimensional bioprinting, Tissue Eng., Part C, 2014, 20(6), 473–484, DOI:10.1089/ten.TEC.2013.0335
.
- M. Bauer, M. Metzger, M. Corea, B. Schädl, J. Grillari and P. Dungel, Novel 3D-Printed Cell Culture Inserts for Air–Liquid Interface Cell Culture, Life, 2022, 12(8), 1216 CrossRef CAS PubMed
. [Online]. Available: https://www.mdpi.com/2075-1729/12/8/1216.
- M. W. Carlson, A. Alt-Holland, C. Egles and J. A. Garlick, Three-dimensional tissue models of normal and diseased skin, Curr. Protoc. Cell Biol., 2008 DOI:10.1002/0471143030.cb1909s41
, Chapter 19, Unit 19.9.
- B. S. Kim,
et al., 3D cell printing of in vitro stabilized skin model and in vivo pre-vascularized skin patch using tissue-specific extracellular matrix bioink: A step towards advanced skin tissue engineering, Biomaterials, 2018, 168, 38–53, DOI:10.1016/j.biomaterials.2018.03.040
.
- M. Albanna, K. W. Binder and S. V. Murphy,
et al., In Situ Bioprinting of Autologous Skin Cells Accelerates Wound Healing of Extensive Excisional Full-Thickness Wounds, Sci. Rep., 2019, 9(1), 1856, DOI:10.1038/s41598-018-38366-w
.
- A. Skardal, S. V. Murphy, K. Crowell, D. Mack, A. Atala and S. Soker, A tunable hydrogel system for long-term release of cell-secreted cytokines and bioprinted in situ wound cell delivery, J. Biomed. Mater. Res., Part B, 2017, 105(7), 1986–2000, DOI:10.1002/jbm.b.33736
.
- H. Herrada-Manchon, L. Celada, D. Rodriguez-Gonzalez, M. Alejandro Fernandez, E. Aguilar and M. D. Chiara, Three-dimensional bioprinted cancer models: A powerful platform for investigating tunneling nanotube-like cell structures in complex microenvironments, Mater. Sci. Eng., C, 2021, 128, 112357, DOI:10.1016/j.msec.2021.112357
.
- A. M. Hughes, A. D. Kolb, A. B. Shupp, K. M. Shine and K. M. Bussard, Printing the Pathway Forward in Bone Metastatic Cancer Research: Applications of 3D Engineered Models and Bioprinted Scaffolds to Recapitulate the Bone-Tumor Niche, Cancers, 2021, 13(3), 507, DOI:10.3390/cancers13030507
.
- R. Schmid, S. K. Schmidt, J. Hazur, R. Detsch, R. E. Maurer, A. R. Boccaccini, J. Hauptstein, J. Teßmar, T. Blunk, S. Schrüfer, D. W. Schubert, R. E. Horch, A. K. Bosserhoff, A. Arkudas and A. Kengelbach-Weigand, Comparison of Hydrogels for the Development of Well-Defined 3D Cancer Models of Breast Cancer and Melanoma, Cancers, 2020, 12(8), 2320, DOI:10.3390/cancers12082320
.
- T. Namekawa, K. Ikeda, K. Horie-Inoue and S. Inoue, Application of Prostate Cancer Models for Preclinical Study: Advantages and Limitations of Cell Lines, Patient-Derived Xenografts, and Three-Dimensional Culture of Patient-Derived Cells, Cells, 2019, 8(1), 74, DOI:10.3390/cells8010074
.
|
This journal is © The Royal Society of Chemistry 2023 |
Click here to see how this site uses Cookies. View our privacy policy here.