DOI:
10.1039/D2EN00052K
(Critical Review)
Environ. Sci.: Nano, 2022,
9, 2237-2263
Emerging investigator series: metal nanoparticles in freshwater: transformation, bioavailability and effects on invertebrates†
Received
18th January 2022
, Accepted 25th April 2022
First published on 6th May 2022
Abstract
The increasing use of metal oxide-based nanoparticles (MNPs) and their release into the environment cast concerns about their environmental impacts. Massive efforts have been focused on environmental behaviours and ecotoxicities to figure out the potential threats posed by MNPs. This review systematically summarises and re-analyses published data about the MNP interactions and transformation processes in freshwater and the toxicological effects of MNPs on invertebrates. A case study was conducted through meta-analysis to examine the impacts of silver nanoparticle exposure to freshwater invertebrates. The conclusions categorized the current understanding of the outcome and ecotoxicity of MNPs in freshwater. The adverse outcome pathway (AOP) is recommended for environmental risk assessment as it provides a rapid and accurate risk assessment of an increasing number of novel compounds consuming fewer resources and animal tests. Invertebrates contribute significantly towards developing robust AOPs thanks to a shorter life cycle, allowing chronic and complete life cycle toxicity tests.
Environmental significance
MNPs may undergo different environmental processes in the aquatic system, consequently changing their mobility, bioavailability, and toxicity to organisms. This review summaries and re-analyses published data regarding the MNP interaction with environmental factors and transformation processes in freshwater and the toxicological effects of MNPs in three major groups of invertebrates by considering the bioavailability of MNPs as an essential step to understand their biological outcome. After ingestion by freshwater invertebrates, MNPs are likely to be accumulated in sensitive organs and induce ROS production, a predominant mechanism leading to toxicity. ROS production induced by MNPs is controlled by size, shape, surface, composition, solubility, aggregation and particle uptake. In addition, a meta-analysis was conducted to examine the impacts of silver nanoparticle exposure on freshwater invertebrates as a case study. Significant research gaps and recommendations for future research are also indicated.
|
1. Introduction
Nanoparticles (NPs), with at least two dimensions between 1 and 100 nm, possess physicochemical properties that offer many medical, societal and technological benefits.1 Metal and metal oxide-based NPs (MNPs) are the most commonly used materials and are particularly important to our life.2 For example, the Organization for Economic Co-operation and Development (OECD) has highlighted silver (Ag), zinc oxide (ZnO), titanium dioxide (TiO2) and cerium dioxide (CeO2) NPs as high interest due to their widespread applications and inherent properties.3 However, the mass application of products containing NPs inevitably results in nanoparticle pollution, which triggers concerns about their environmental impacts.4,5 Water ecosystems are among the most vulnerable to contamination because they receive and accumulate large amounts of pollutants, including nanomaterials, from rainfall, surface runoff, subsurface seepage or wastewater discharge.6 As the primary water environment in inland areas, freshwater ecosystems are undoubtedly the prime victim of nanomaterial pollution. The occurrence of NPs in the freshwater environment is globally observed.7,8 Numerous research studies show that the effects of nanomaterials on freshwater organisms exist at all biological levels and all stages of the organism's life cycle.9–11
Aquatic invertebrates represent well-established model organisms for MNP toxicological studies.12 Some invertebrates, including bivalves,13,14 gastropods15,16 and crustaceans,17,18 are considered good environmental quality indicators due to their wide geographic distribution, abundant numbers and easy availability. Bivalves (e.g., Mytilus spp.) are filter-feeding invertebrates in natural waters with highly developed endo- and phagocytosis pathways.14 Based on pollutant accumulation in these organisms, fitness can be adopted as a biological indicator of the load on the ecosystem.13 Gastropods (e.g., Lymnaea stagnalis) display significant advantages in high sensitivity and susceptibility to water contamination and straightforward laboratory maintenance.16 Such organisms are also an ethically acceptable alternative to an animal model in toxicity tests.15,19 Regarding crustaceans, Daphnia magna is one of the most sensitive organisms widely used and included in several guidelines and international standards for acute and chronic tests.17,18 It can thus be concluded that aquatic invertebrates are very promising for ecotoxicology research.
How MNPs affect freshwater ecosystems has drawn increasing attention in the last few years. One of the major concerns is the bioavailability of MNPs in freshwater organisms, which may undermine their fitness. Compared with marine water, there is more natural organic matter (NOM) in freshwater, which rapidly adsorbs onto the surface of MNPs, producing repulsive forces between particles and decreasing their aggregation.20 Furthermore, freshwater normally displays lower ionic strength (conductivity: 0.03–0.10 mS cm−1) than marine water (47.8–49.8 mS cm−1), where charged NPs are less neutralised by counterions and keep their colloidal stability and thus are less aggregated.21 This might lead to a longer residence time (which is related to the bioavailability) of NPs in freshwater rather than in marine waters.20 Many existing documentaries on the bioaccumulation of various MNPs, including AuNPs,22,23 AgNPs,24,25 and CuONPs are available.26,27 MNPs are primarily mediated by ingestion and subsequently accumulate in gut tissues of organisms and are often not readily taken up by the epithelial surface and transported via the circulatory system.28,29 Further, MNPs may be taken up by plasma membrane vesicles rather than being absorbed by the cell through transferring proteins and passive diffusion. Once ingested, MNPs remain in the digestive tracts of organisms for days up to weeks, allowing the transfer of NPs to the food web.30–32 Biomagnification may cause more significant impacts on freshwater ecosystems because it amplifies the concentrations of MNPs at upper trophic levels by an order of magnitude.30 Thus, understanding the capacity for MNPs to bioaccumulate in organisms and subsequently transfer through and biomagnify within food chains is crucial.
Another primary concern is how MNPs impact freshwater organisms after ingestion. Multiple recent reviews have summarized the MNP's toxicity to aquatic organisms and highlighted that additional research to improve our understanding of the adverse impacts of MNPs is necessary. The physicochemical characteristics of MNPs change as an environmental release with time under the influence of the surrounding environment, thereby affecting the impact on organisms. Abiotic factors such as media composition, sulfidation, irradiation, pH and ionic strength (IS) of media may also contribute to determining the outcome and toxicity of NPs.33 The overall potential adverse impacts of MNP pollution are considered challenging to predict. We need to integrate quantitative studies to produce a more comprehensive and objective evaluation of the differential biological responses triggered by MNP exposure.
A meta-analysis is a powerful tool to rigorously assess the findings of published independent research, which can help determine the effect size for result variables. A meta-analysis has been developed to study the toxicological TiO2 NPs on marine bivalves.34,35 Furthermore, due to the diversity of the MNPs, toxicity evaluation is challenging to access, and the limitation remains to test various MNPs' toxicity each time. To address these limitations, a toxicity mechanism-based approach, such as the adverse outcome pathway (AOP), is more practical than a substance-based approach. However, the majority of NP toxicity studies focused on ecotoxicity took apical endpoints, and only a few dealt with toxicity mechanisms.36–39 AOPs span numerous levels of biological organization, from the molecular level to an organism level or even to population levels for ecotoxicology scenarios.40 Since the AOP is an evidence-based framework, it is constantly updated following new evidence of toxicity mechanisms, improving its reliability and application.41 It also allows us to identify data gaps for future research based on the current AOP. Thus, although the study of toxicity mechanisms of MNPs is limited, identifying the currently available molecular-level toxicity information and linking it with AO is a tactical beginning for the risk assessment of MNPs.
This review summarizes the transformation processes that MNPs undergo and the effects of main physical–chemical properties and environmental factors on MNPs' transformation in freshwater. We present the records that examined the impact of MNPs on freshwater invertebrates, experimentally and under laboratory-controlled conditions. Based on these studies, we conduct a meta-analysis to investigate the importance of the MNP type, size, coating, concentration and exposure time effect of MNPs on freshwater invertebrates. Through the comprehensive analysis of the current research on the effects of MNPs, we propose an appropriate AOP to manage MNPs based on the existing literature and highlight research gaps, discuss methods, and identify recommendations and perspectives for future research.
2. Environmental transformations of MNPs in freshwater
Knowledge of the environmental fate and behaviour has essential implications in evaluating the MNPs' hazards and ecological and environmental risks. When NPs are released into the environment, these NPs, which have high surface activity, readily interact with substances in complex environments, causing changes in their surface structure, resulting in more unique environmental behaviours.42 The MNPs exposed to freshwater systems undergo various physical (e.g., aggregation, adsorption and sedimentation), chemical (e.g., dissolution and sulfidation), and biological (e.g., eco-bio-corona) processes.9,33,43 The above transformations are known to depend on the physicochemical properties (e.g., size, charge, surface coating, concentration) of MNPs and water conditions (e.g., IS, pH, NOM).43,44
2.1 Physical transformations
2.1.1 Aggregation.
Aggregation refers to MNP cluster formation in suspension, and such a process increases the NPs' size and density, which leads to gravitational settling in the sediment and impact on benthic organisms.45,46 NPs' size has been found to affect the aggregation, due to the higher percentage of atoms present on the surface of NPs with smaller size, resulting in surface chemistry change and subsequent layer charge decrease, thus promoting this process.47 For instance, in moderately hard water, smaller platinum (Pt) NPs (20 and 30 nm) formed a wide-size range and larger aggregates than larger sizes (75 nm).48 Similar results were also found in AuNPs when exposed to a sterile lake water medium, where 30 nm NPs aggregated more rapidly than 40 nm NPs.49 Generally, higher concentrations carry higher surface charges, which promotes the stability of NPs by limiting the inter-particle contact. For example, Al2O3NPs showed lower aggregation at 1 mg L−1 concentration compared with lower 0.1 mg L−1 in river water.50
The nature of the surface coating also acts as an essential factor in deciding the MNPs' aggregation. In lake water, compared with polyvinylpyrrolidone (PVP)-coated AgNPs, lipoic acid (Lip) and citrate (Cit) coatings demonstrate lower protective effects of AgNPs against aggregation.51 These results illustrate that the surface coating stabilising AgNPs (i.e., PVP, higher affinity) by steric repulsion is more effective than electrostatic repulsion (i.e., Cit and Lip, weak affinity). In both raw and filtered river water, polyethylene glycol (PEG) or carboxylated PEG (PEG–COOH)-coated AuNPs showed good stability, while branched polyethyleneimine (bPEI), amine-functionalized PEG (PEG-amine) and Cit-coated AuNPs have been shown to readily aggregate.52 These neutral or negatively-charged coatings (e.g., PEG, PEG–COOH-) could prevent homoaggregation of NPs via electrical double-layer (EDL) compression and are not affected by the presence of NOM in test medium.53 Although positively charged (e.g., bPEI-, PEG-amine-, Cit-) coatings could also serve to stabilize the NPs, they are more easily adsorbed with NOM and promote aggregation either by interparticle bridging or divalent cation bridging.52,53
The point of zero charge (pHPZC) refers to the pH when the net surface charge of NPs approaches zero. Theoretically, the smaller the difference between solution pH and pHPZC, the greater the aggregation rate. For example, in wastewater, when the pH value approaches the pHPZC of ZnONPs, due to the decreased repulsive interactions between NPs, the process of aggregation and deposition occurs within two hours.54 When the pH value of simulated lake water reached the pHPZC of TiO2NPs, large agglomerates were observed.55 In general, divalent electrolytes (Ca2+ and Mg2+) in freshwater could destabilize MNPs, and the enhanced IS tends to weaken electrostatic repulsion between particles, compressing the EDL surface and leading to aggregation.56,57 For instance, CuNPs' aggregate size correlated well with IS in ground water and freshwater.56 An increase in the particle size of AgNPs was reported in surface water with higher IS.57 After exposure to filtered river water, the AgNPs aggregate readily within one hour.58 Under freshwater relevant conditions, the aggregation level of Cit-AgNPs and TiO2NPs depends mainly upon the concentration of Ca2+.59
In freshwater, NOM represents the most critical ligand group, composed mainly of humic acids (HAs) and fulvic acids (FAs). Rich functional groups of NOM provide the high potential to adsorb MNPs via various mechanisms, including hydrophobic interactions, van der Waals interactions, surface ion chelation, cation bridging, etc.42,60 It is suggested that HAs can inhibit the aggregation and deposition of MNPs (e.g., Ag, Fe, Fe3O4, Al2O3, TiO2, SiO2 and ZnO) through electrostatic and spatial dislocation effects.42 However, in the presence of divalent cations, dissolved organic matter (DOM) can flocculate on the surface of MNPs through cationic bridging, causing the occurrence of aggregation and deposition of MNPs.61 In addition, different pH and ionic conditions affect the adsorption of NOM. For example, under acidic and basic conditions, DOM can be adsorbed on the surface of AgNPs via carboxyl groups and aliphatic and phenolic groups, respectively.62 In the presence of NOM, the larger-sized PVP-PtNPs (95 nm) are more affected than the smaller NPs (20 nm), which form larger agglomerates in artificial freshwater.48 Similar results were observed in FA coated CeO2NPs, which generated small aggregates in lake water, while large aggregates were obtained in the absence of FAs.63 In natural lake water, NOM could accelerate the heteroaggregation of TiO2NPs and subsequent sedimentation.64
2.1.2 Sedimentation.
The sedimentation process is critical for removing MNPs from water bodies and thus is essential in their fate studies.65 For example, after exposure to moderately hard water for 24 hours, around 28–53% of PtNPs were likely to settle out of the suspension.48 Homo-aggregation (MNPs' cluster) and hetero-aggregation (MNPs associated with suspended natural colloids) of MNPs lead to denser particles and are considered the main pathways for deposition in the sediment.33 The prevalence of NOM and IS in an aqueous environment are considered two key factors affecting NPs' sedimentation.
NOM can promote aggregation and aggravate sedimentation by bridging the function at low concentrations while inhibiting or slackening this process by increasing the surface charge and spatial resistance at high concentrations. For instance, in freshwater, almost complete sedimentations were observed for CuNPs, AlNPs and MnNPs within less than 15 min due to rapid aggregation.66 Furthermore, a higher concentration of NOM (e.g., HA and dihydroxy benzoic acid) enhanced the NPs' electrostatic stabilization, evidenced by lower sedimentation velocity.66 IS could promote sedimentation by compressing the EDL of NPs.56 For example, sedimentation of CuNPs and CuONPs were directly or inversely proportional to IS and organic content in lake water, respectively.56 A recent study highlights that heteroaggregation between AgNPs and suspended sediment (SS) played a predominant role in settlement behaviour under high IS conditions, while AgNPs distributed in the overlying waters under low-salinity and SS conditions.45 Similarly, CeO2NPs were stable in low pH, IS, and SS water, whereas aggregation occurred with increasing cation concentration, showing that the larger size NPs (>1000 nm) settle quickly to the bottom while the smaller particles are suspended in solution.67
2.2 Chemical transformations
2.2.1 Dissolution.
MNPs readily react with H+ and dissolved oxygen from water and release metal ions, a process usually referred to as dissolution.33 Some MNPs with active chemistry properties, such as AgNPs, CuONPs and ZnONPs, are susceptible to oxidation dissolution.33 In the case of AgNPs, Ag+ could be released via the redistribution of adsorbed Ag+ on the NPs' surface during the synthesis process and dissolution of the outer Ag2O oxide layer.68
Smaller NPs dissolve more quickly than larger ones due to the enhanced surface area, indicating that more available surface sites are involved in dissolution.69 For instance, in lake water medium and freshwater-like conditions, enhanced dissolution was found at smaller-sized (50 nm) ZnONPs than 100 nm and bulk form.70,71 In media relevant for freshwater, the smallest AgNPs (5 nm) promoted a higher dissolution rate than larger NPs (10 and 20 nm), which could be explained by the proton number that active molecular oxygen adsorbed by the surface.72 In freshwater, a significant dissolution percentage (∼81.98%) was found at lower concentrations (10 mg L−1) of ZnONPs compared with 1000 mg L−1, which exhibited ∼78.83% dissolution after one hour of exposure.73 Similar results were found in AgNPs after exposure to moderately hard reconstituted water, where dissolution rates of 5 μg mL−1 are much higher than that for 100 μg mL−1.74 This difference might be explained by the higher ratio of Ag+ to Ag complexing agents, which helps scavenge Ag+, or Ag+ could associate back with the NPs at high concentrations.74
The surface coating of NPs and test media also affects its solubilisation pattern. For some highly soluble MNPs (e.g., Ag, CuO, and ZnONPs), their dissolution rates showed a wide range of 1–80% under various environmental scenarios, which demonstrates the critical role of media constituents (e.g., pH, IS and NOM) in this process.75 For example, Cit-AgNPs showed higher dissolution when compared to PVP AgNPs76,77 in laboratory scenarios, where PVP-AgNPs are more prone to Ag+ release than Cit-AgNPs in natural waters.78 Selenium (Se) NPs displayed a higher dissolution rate (∼35.3%) in lake water when compared with ultrapure water (∼20.8%).79 The presence of NOM in freshwater reduces the dissolution of CuONPs, which might be via metal ion-chelating or coating particle surfaces.56 In synthetic freshwater, the HAs and dihydroxy benzoic acid (DHBA) could adsorb on CuNPs, AlNPs and MnNPs within one minute and, in particular, enhance the dissolution of AlNPs and CuNPs.66 The HA and DHBA could coordinate with the NP surface via forming mononuclear surface complexes, which weaken the bonds between the metal and oxygen in the surface oxide and thus promote the dissolution of CuNPs.80
2.2.2 Sulfidation.
Sulfidation plays an essential role in controlling metal ion concentration in the environment due to the high complexation tendency with sulfide ligands.33 Sulfide, which is generated by microbial sulfate reduction, commonly exists in hypoxic environments such as riverine, lake sediments and wastewater treatment plants.81,82 For instance, sparingly soluble silver sulfides (Ag2S) have been identified as a major Ag species in the sewage sludge taken from urban wastewater systems.81 In moderately hard reconstituted water, AgNPs could transform to Ag2S via direct or indirect oxysulfidation, depending on the concentration of sulfides.83 At high concentration (mg L−1) applied, the AgNPs undergo a fast, direct NPs–fluid reaction and generate Ag2S; meanwhile at low concentration, the AgNPs first develop into silver ions before reacting with sulfide ions and eventually generate Ag2S.83,84 Such a transformation process could be reversible, and one recent study proposed that Ag could be remobilized from Ag2S with the aid of Fe(III) in freshwater under light conditions.85
Generally, smaller sizes could enhance the sulfidation rate of AgNPs, probably due to the dependency of the reaction rate on the specific surface area of NPs.86 The enhanced ratio of HS−/Ag also contributes to the sulfidation of AgNPs, where Ag2S bridges are formed between NPs.86 Other factors, such as NOM, can also affect the sulfidation process. For example, the presence of HAs and FAs could slightly enhance or decrease the sulfidation rate of AgNPs, respectively.38 HAs might promote the sulfidation reaction via replacing the surface coating of NPs, giving rise to an extensive available surface area, while FAs diminish this process by blocking the surface of AgNPs. Notably, the sulfidation process is usually accompanied by more significant aggregation and sedimentation and a lower dissolution profile, which might influence the fate and bioavailability of NPs (e.g., AgNPs, ZnONPs).9,86 For instance, a recent study highlighted that the sulfidation process could diminish the toxicity of AgNPs in constructed wetlands.87
2.3 Biological transformations
The eco-bio-corona is the principal biological mediated transformation in the environment. Upon entering the aquatic environment, MNPs are rapidly encapsulated by biomolecules (e.g., extracellular polymeric substances (EPS), which are secreted mainly by aquatic organisms' metabolic activities), forming an eco-corona. Similarly, the bio-corona is formed via interacting with the adsorbed endogenous proteins in the presence of organisms.88,89 The eco-bio-corona can modify the distribution, accumulation, degradation, intracellular recognition and biotoxicity of NPs by altering their structure, kinetic behaviour and function.90 On the other hand, the biocompatibility of NPs within organisms can be improved by modulating the cellular uptake of NPs. For example, the bio-corona can control the interaction of NPs with outer membrane receptors for specific cellular uptake.91
Biomolecules have been known to bind with metals via electrostatic interaction and complexation, with the aid of many functional groups, including carboxyl, hydroxyl, etc.92 EPS are amphiphilic molecules with a hydrophobic region, facilitating adsorption onto organic substances. Studies have shown that positively charged NPs generally readily interact with negatively charged EPS such as polysaccharides or proteins, while hydrophobic components of EPS can act as stabilisers to stabilise NPs. For example, the EPS of freshwater biofilms could stabilize CeO2NPs and induce aggregation of AgNPs.93 Similarly, EPS adsorption enhanced the ZnONPs' stability with electrostatic attraction and surface complexation involved.94 EPS also exhibit reducing characteristics due to the reducing functional groups (i.e., hydroxyls, phenolic–OH, thiols and aldehydes).95 Recent research proposed that Ag+ could be reduced to AgNPs by EPS in natural water, and this process could be enhanced under light irradiation.96 Thus, EPS can change the environmental behaviour of NPs and might subsequently influence their fate and toxicity in the environment.39
In addition to the EPS derived from natural water, the biomolecules secreted by organisms could also interact with the MNPs' surface and form a new identity (i.e., eco-corona) which affects the stability and toxicity of the MNPs toward organisms in the surrounding environment. For example, a recent study showed that AuNPs could interact with protein secreted by D. magna and produce protein-corona, reducing the AuNP aggregation and potentially detoxifying AuNPs to D. magna by shielding their surface attraction.97 Conversely, for polystyrene NPs, a previous study highlighted the promoted aggregation of NPs with protein-corona in a dispersion medium previously conditioned with D. magna neonates.98 Many studies reviewed the bio-corona formation and associated biological effects.91,99,100 However, most of these focused on biomedical and human toxicology, and studies demonstrating this field in natural waters, including freshwater, are still elusive.99,100
To sum up, after entering the freshwater system, MNPs undergo physical, chemical, and biological transformational processes governed by NPs' characteristics and the chemical properties of water. In general, small size NPs easily form large clusters due to the large surface and high surface energy. Therefore, the concentration and surface coating of NPs demonstrate essential roles in determining the size by electrostatic and steric repulsion functions. The pH impacts the surface potential of NPs, and large clusters are formed as the pH approaches the pHPZC. The low IS and high NOM concentration in freshwater generally stabilize the NPs but the fate and behaviour of NPs are also affected by other factors due to the complex composition of the natural system. A schematic diagram showing the environmental transformations MNPs in aquatic environments is presented in Fig. 1.
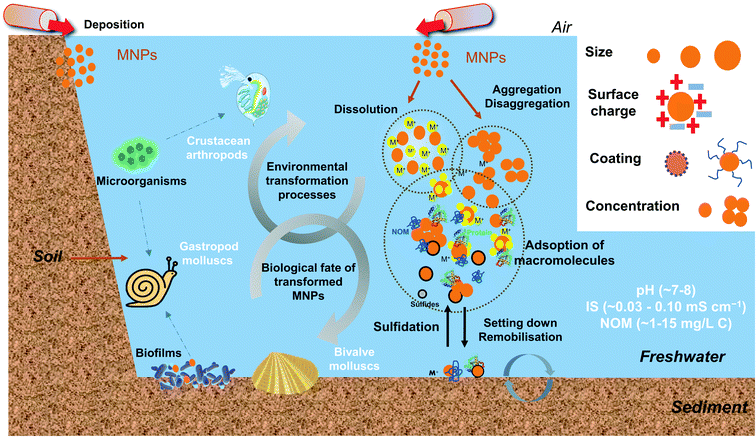 |
| Fig. 1 The schematic diagram illustrates the overview of the environmental transformations and the biological fate of transformed MNPs in freshwater environments. MNPs may undergo physical, chemical, and biological transformation processes. The MNP transformation differs mainly according to their intrinsic properties (e.g., size, surface charge, coating and concentration) and environmental conditions (e.g., pH, IS, NOM, related values are referenced from ref. 21, 56 and 64). The transformed MNPs may accumulate in some typical freshwater invertebrates, such as filter-feeding bivalve molluscs, gastropods molluscs and crustacean arthropods, and contribute to the trophic transfer of MNPs. | |
3. Bioavailability of MNPs in freshwater invertebrates
Studies on bioavailability and uptake are critically essential to link the environmental transformation of MNPs to biological responses.101 Individual free MNPs, homoaggregates and MNP heteroaggregates can accumulate and subsequently remain within organisms.18,32,102–104 In natural water, some soluble MNPs (e.g., ZnONPs, CuONPs and AgNPs) can release metal ions, which can cause latent free-ion toxicity, resulting in different toxic impacts than exposure to the pristine MNPs.18,105,106 Understanding the bioaccumulation of MNPs is pivotal for addressing their ecotoxicity and risk assessment because it determines the potential MNP concentration. Since mollusk and Arthropoda are the largest phyla of invertebrate animals, three commonly used classes for toxicology studies (bivalvia, gastropoda and crustacean arthropods) are discussed in separate sections.
3.1 Bivalve molluscs
Bivalve molluscs, sedentary-style filter-feeding invertebrates, are widely adopted as bioindicators in nanotoxicology.107 Filter-feeding bivalves may filter large water throughputs at high rates for respiratory and nutritional purposes and thus may ingest considerable amounts of MNPs dispersed in the water.3 Bivalves are considered an effective sink for MNPs.108 Suspended MNPs from the water, concentrated in faeces and pseudo-faeces, can be captured and ingested by benthic invertebrates and transferred into the aquatic food chain.109 As filter feeders, bivalves represent one of the most important pathways of MNPs into the human food chain.35,110
Bioaccumulation tests of MNPs in freshwater bivalves have primarily been conducted in Corbicula fluminea,22,102Anodonta cygnea,111 and Dreissena polymorpha.112,113 After exposure to AgNPs, most particles accumulated in the viscera (gills and digestive tract) of C. fluminea, while mantle, muscle, and foot tissue showed low accumulation potential.102 This indicates no significant transport of AgNPs through the different compartment tissues or hemolymph and thus points to the negligible bioavailability of AgNPs to C. fluminea. Gills and digestive glands are considered preferential organs of MNP accumulation in filter-feeding organisms, compared to mantle and foot organs.114A. cygnea in exposure to sub-lethal concentration of CuONPs (40 nm) for 12 d leads to a significant accumulation of Cu in mantle and foot than that in gill.111,115 It is worth noting that the MNP's surface properties could influence the bioaccumulation process. D. polymorpha accumulated Cit-CeO2NPs three times more than bare-CeO2NPs; the reason behind this difference is correlated with their distinct behaviours presented in the water column. Cit-CeO2NPs are more stable than bare-CeO2NPs in water, making it easier to be captured by mussels.112
Freshwater bivalves can directly ingest and rapidly accumulate MNPs in water (i.e., bioconcentration)102,111,112 or intake of MNPs via trophic transfer (e.g., from prey to predator).22 For example, in Arini's study,22 the rate constants for uptake of AuNPs from water (kuw) and food (kuf) were accessed. The former way is likely to be the primary exposure pathway for C. fluminea, considering the higher kuw than kuf. During the dietary exposure, C. fluminea decreased its ventilator activity, resulting in reduced gill filtration. Thus, Au accumulation presents a 30-fold reduction after dietary exposure to algae loaded with AuNPs compared to waterborne exposure.22 The results above revealed that MNPs might compromise bivalves' feeding capacity, but indeed, not just for bivalves, other freshwater species were also observed to exhibit filtration behaviour impairments as reviewed below.
3.2 Gastropod molluscs
Gastropod organisms are ubiquitous in the aquatic ecosystem. Most freshwater gastropods (e.g., Lymnaea stagnalis) are lung-breathing pulmonates; hence, they spend time at the water surface for air-breathing and ingestion.116 They are considered sentinel species for pollution biomonitoring in their wide geographic distribution, relatively sedentary life habits, and ease of availability.117
Current studies have indicated that the dissolved metal ion form (e.g., Ag+, Cu2+) was more bioavailable than their nanoparticulate forms in gastropod molluscs.24,27 For example, the total body burden of Cu in the whole freshwater snails Potamopyrgus antipodarum118 and Bellamya aeruginosa27 was significantly higher for those exposed to Cu2+ than both control and CuONPs treatments. Freshwater snails B. aeruginosa24 and Peringia ulvae119 also accumulated higher levels of Ag from Ag+ than from particulate Ag. This ingestion discrepancy could be related to the different uptake pathways between MNPs and dissolved metal ions. The former could be potentially internalized via endocytotic pathways: clathrin-mediated endocytosis, caveolae-mediated endocytosis, or macropinocytosis. Meanwhile the dissolved ions from MNPs can enter cells via transporter channels, including the proton-coupled Na+ channel.120 Furthermore, MNPs tend to agglomerate, aggregate and form a bio-eco-corona in natural water, and thus their bioavailability would be substantially reduced.89,121 However, some research showed the contrary. For example, B. aeruginosa accumulated a higher concentration of Ag from sediments spiked with Ag+ than AgNPs.24 Upon the same total metal sediment concentrations, CuO NPs were more bioavailable than aqueous Cu to deposit-feeding snail P. antipodarum,122 which is inconsistent with bioaccumulation results with B. aeruginosa.27 This discrepancy may result from differences in experimental design, particularly the exposure duration (56 d for the former vs. 28 d for the latter) to CuONPs. The elimination rate of CuONPs may be lower than that of dissolved Cu2+ over time. In addition, no significant Cu accumulation difference in Planorbarius corneus was found between matrix-embedded CuO NPs and controls with realistic low concentrations, which might make detecting Cu from the matrix and biological tissues more complicated.123
The physicochemical properties of the exposure media (e.g., NOM) can also affect the MNP bioaccumulation. Sikder et al. indicated the importance of (PtNPs) size and interfacial interactions with NOM on Pt bioavailability.48 Both dissolved and PtNPs (size range from 20 ∼95 nm) show good bioavailability to L. stagnalis. In the absence of NOM, the larger Pt NPs have higher bioavailability than the smaller ones, while it is the opposite in the presence of NOM.48 This may be explained by the surface adsorption of NOM on larger particles, making them susceptible to rapid precipitation and forming larger agglomerates, and suggests that PtNPs' in vivo transformation could have higher adverse effects on organisms than the dissolved metal ion form.
Diet-borne exposure plays a significant role in the bioaccumulation and toxicity of NPs in aquatic organisms, compared with single waterborne scenarios.105,111,124–127 For example, dietary exposures to ZnONPs and AgNPs suppressed the assimilation efficiency of the snail L. stagnalis.125,128 The reduced bioavailability of MNPs may be explained by the agglomeration/aggregation of the MNPs onto diatom mats or detrimental effects on digestion.125 Recent work highlights the higher Ag bioaccumulation potential in the Ag+ treatment compared with Ag2SNP exposures, but no biomagnification was observed from the freshwater snail Physa acuta to the planarian Girardia tigrina.129 To fully mimic the real environmental scenario with a food chain with more than three species, another study constructed a freshwater ecosystem (including clam C. fluminea, snail P. acuta, and water flea D. magna) and determined the bioaccumulation and biomagnification of CeO2NPs via long-term exposure. Results found that bioaccumulated Ce in all tested species was negatively correlated with its trophic level, showing no biomagnification of CeO2NPs through this food web.32 Conversely, the significant biomagnification of CeO2NPs was reported in terrestrial food chains.130 These findings implied that biomagnification of NPs in the natural environment might be complicated and affected by several factors, such as NPs' intrinsic characteristics and prey–predator dependence.131 Future studies addressing mechanisms underlying the trophic transfer of NPs are urgently needed.
3.3 Crustacean arthropods
Current studies about MNP bioavailability in freshwater crustacean taxa have mainly focused on Daphnia spp. Daphnia, the common water flea, is widely used as a model aquatic organism in toxicity studies.132 So far, MNP accumulation studies have been mainly performed with TiO2NPs,133–136 AgNPs25,137,138 and ZnNPs.103
Current studies have indicated that MNPs (e.g., TiO2NPs and CuONPs) mainly accumulated in the gut tissue of D. magna.18,133 As filter feeders, D. magna can trap MNPs inside the body upon filtration and appendage movement of D. magna can also contribute to NP ingestion if there was NP adherence to the carapace.18 Fan et al. investigated the effects of TiO2NPs' properties on the BCFs for the first time.136 They reported a higher BCF at lower concentrations (0.1 and 1 mg L−1) than at a higher dose (10 mg L−1) regarding TiO2NP exposure in D. magna.136 Under a higher concentration scenario, TiO2NPs might form larger aggregates and thus not be taken up by D. magna.
The diet has been demonstrated as the most critical route of uptake and bioaccumulation for trace metals. Exposure to MNP contaminated diet can suppress the feeding behaviour in Daphnia spp. Previous findings suggest that Daphnia spp. decrease AgNP intake during dietary exposure.137 Possible explanations for the feeding rate reductions may include the accumulation of MNPs in the gut or higher sedimentation of contaminated algae to the bottom of the container resulting in lower availability for filter-feeding.139 AgNPs were not eliminated from Daphnia over the depuration period (48 h), leading to further possible transport of AgNPs along the food chain.25 Indeed, it is well established that MNPs, such as AgNPs,137 TiO2NPs133–135 and AuNPs,140 can be transferred from freshwater algal diet to Daphnia spp. Moreover, the trophic transfer showed size dependence in the Chen et al. experiment133 which found that TiO2NPs' biomagnification factors (BMFs) decreased with increasing MNP size. Generally, due to the higher specific surface area, smaller TiO2NPs are more prone to attach to the algal cell than larger ones, causing the latter to be of lower bioavailability.133
To summarize, the bioavailability of MNPs relates to the subsequent toxicity to aquatic organisms; thus it is crucial to know the accumulation of MNPs in organisms to understand and predict their toxicity. The high potential of internalization and accumulation of MNPs in freshwater invertebrates has been mainly shown in bivalvia, gastropoda, and crustacean arthropods. Uptake of the MNPs occurs with different processes known as endocytotic pathways, clathrin-mediated endocytosis, caveolae-mediated endocytosis, macropinocytosis, etc. Moreover, the bioavailability of MNPs is influenced by the physicochemical characteristics of MNPs (e.g., size, concentration, and surface chemistry) and the employed experimental conditions (e.g., exposure time and routes).
4. Toxicity of MNPs to freshwater invertebrates
MNPs exhibit some unique characteristics compared to their bulk forms, such as a high surface-to-volume ratio and abundant reactive sites on the surface, which along with their mobility, lead to unexpected environmental hazards. In the last decade, specific physicochemical properties such as size, shape, and surface functionality of MNPs have influenced their toxicity.16,43,141 Furthermore, various aquatic organisms have been studied to demonstrate MNPs' toxic effects. In this part, we reviewed MNPs' toxicity related studies published after the year 2011 from the Web of Science (https://www.webofscience.com/wos/woscc/basic-search) and Google Scholar (https://www.scholar.google.com). Furthermore, we chose three major groups of freshwater organisms within section 3 to discuss and present a compilation of different toxicological measurements for various MNPs in Tables 1–3.
Table 1 Overview of the toxic effects of MNPs on bivalves according to species, and type of MNPs
Species |
MNPs |
Time |
End pointsa |
Ref. |
Type |
Size (nm) |
Conc. |
Glutathione (GSH), superoxide dismutase (SOD), POD catalase activity (CAT), malondialdehyde (MDA), glutathione peroxidase (GPx), glutathione-S-transferase (GST), reactive oxygen species (ROS) production, acetylhydrolase (AChE), hydrogen peroxide (H2O2), lipid hydroperoxide (LOOH), mitochondrial electron transport system (ETS), lactoperoxidase (LPO), alkaline phosphatase (ALP), phenoloxidase-like (PhO) activity, nitric oxide (NO), adenosine triphosphate (ATP), pyruvate kinase-lactate dehydrogenase (PK-LDH).
|
Bellamya aeruginosa
|
AgNPs |
20 |
1, 10, 100 μg g−1 |
14 d |
↑GSH (HP, F, gonad, DG), ↑SOD (HP), ↑POD (F), ↓CAT (HP, gonad) |
24
|
Coelatura aegyptiaca
|
AgNPs |
8–19 |
12.5, 25 and 50 mg L−1 |
6 d |
↑MDA, ↓GSH, ↓CAT, ↑NO concentration |
145
|
Corbicula fluminea
|
AgNPs |
27.66 ± 0.80 |
0.1, 0.5, 2 mg L−1 |
14 d |
↑SOD, ↑CAT, ↑GPx in the mediate concentration ↑GSH ↑GST ↓GPx in the highest concentration, ↓ammonia excretion and ↓feeding rates |
160
|
Elliptio complanata
|
AgNPs |
80 |
0.8, 4 and 20 μg L−1 |
48 h |
↓HSP72 protein, ↑digestive gland lipid peroxidation, ↑metallothioneins, ↑DNA strand breaks |
152
|
Elliptio complanata
|
AgNPs |
80 |
0.8, 4 and 20 μg L−1 |
48 h |
↑Lipid peroxidation, ↑phagocytosis activity, ↓cytotoxicity activity |
151
|
Sphaerium corneum
|
AgNPs |
15 |
5, 25, 50, 100 and 500 μg L−1 |
28 d |
↓Reproduction, ↑ROS, ↑CAT, ↓GPx, ↑GST, ↓Na+/K+-ATPase activity |
37
|
Ceriodaphnia cornuta
|
AgNPs |
10–50 |
20, 30, 40 and 50 μg mL−1 |
24 h |
↑Mortality and abnormal swimming behavior |
89
|
Dreissenna bugensis
|
AgNPs |
70–80 |
10 and 50 μg L−1 |
48 h |
↓PK-LDH, ↓F-actin, and ↓protein-ubiquitin (UB) |
158
|
Caelatura aegyptiaca
|
Ag/SNCs |
10–25 |
12.5, 25 and 50 mg L−1 |
6 d |
↑MDA, ↑NO concentration, ↓GSH, ↓CAT |
149
|
Corbicula fluminea
|
AuNPs |
10 |
0.5, 1,5,12, 24 ppm |
4 h |
↑Endocytosis gene expression, ↑oxidative stress gene expression, ↑immune system gene expression, ↑apoptosis gene expression |
222
|
Unio ravoisieri
|
Au/TiO2NPs |
10 |
100 and 200 μg L−1 |
7 d |
↓CAT, ↑GST, ↓AChE, ↑H2O2 |
223
|
Unio tigridis
|
Al2O3NPs |
40 |
0, 1, 3, 9 mg L−1 |
14 d |
↓SOD, ↓CAT, ↑GST, ↑GPx |
224
|
Corbicula fluminea
|
CeO2NPs |
20–25 |
10, 100 μg L−1 |
6 d |
↑DNA tail length, ↑Casp-3 activity in the highest concentration |
153
|
Dreissena polymorpha
|
CeO2 NPs |
3–4 |
1 mg L−1 |
21 d |
↓piGST mRNA expression, ↑hemocyte lysosomal system size, ↓CAT, ↓GST, ↓[LOOH] |
112
|
Dreissena polymorpha
|
CeO2NPs |
1385 (dH) |
100 μg L−1 |
14 d |
↑ETS, ↓ROS, ↓SOD, ↓CAT, ↓GPx, ↓GST, |
147
|
Dreissena polymorpha
|
CeO2NPs |
3 ± 1 |
10 and 100 μg L−1 |
4 d |
↓CAT, ↑GST, ↓haemolymph [Na+] |
225
|
Dreissena bugensis
|
CuONPs |
79 ± 10 |
2, 10 and 50 μg L−1 |
96 h |
↑Poly-ubiquitinylated protein, ↓LPO, ↓DNA strand breaks, ↓AChE |
150
|
Lamellidens marginalis
|
CuONPs |
34 ± 4.5 |
0.5, 1, 5 mg L−1 |
14 d |
↓Hemocyte count, ↓phagocytic efficacy, ↑SOD↓nitric oxide generation, ↓ total protein content in hemocytes, ↓CAT, ↓PhO |
148
|
Unio tigridis
|
CuONPs |
40 |
0, 1, 3, 9 mg L−1 |
14 d |
↓SOD, ↓CAT, ↑GST, ↑GPx |
224
|
Limnoperna fortunei
|
TiO2NPs |
20 |
1, 5, 10 and 50 μg mL−1 |
4 h |
↓SOD, ↓CAT, ↓protein sulfhydryl content |
143, 157 |
Limnoperna fortunei
|
TiO2NPs |
21 |
1, 5, 10 and 50 μg mL−1 |
4 h |
↑Tail DNA |
159
|
Unio tumidus
|
TiO2NPs |
<150 |
1.25 μM |
14 d |
↓ROS, ↓PhO ↑SOD, ↑lipofuscin accumulation, ↑TBAR, ↑GSH, ↑GSSG, ↑GSH/GSSG, ↑lactate/pyruvate, ↑ALP, ↑cathepsin D total activity, ↑cathepsin D free (outside lysosome) activity, ↓lysosomal membrane stability |
226
|
Unio tigridis
|
TiO2NPs |
21 |
0, 1, 3, 9 mg L−1 |
14 d |
↓SOD, ↓CAT, ↑GST, ↑GPx |
224
|
Unio tumidus
|
ZnONPs |
50–100 |
3.1 μM |
14 d |
↑Glycogen, ↓glucose, ↓lipids, ↑pyruvate, ↓lactate, ↓lactate/pyruvate, ↑ATP |
162
|
Table 2 Overview of the toxic effects of MNPs to gastropods according to species, and type of MNPs
Species |
MNPs |
Time |
End points |
Ref. |
Type |
Size (nm) |
Conc. |
Bellamya aeruginosa
|
AgNPs |
20, 40 and 80 |
1, 10 and 100 mg g−1 |
14 d |
↑Oxidative stress, ↑GSH, ↑SOD, ↑POD, ↑CAT |
24
|
Biomphalaria alexandrina
|
AgNPs |
— |
3–100 mg mL−1 |
24 h |
Molluscicide, cercaricide, and anti-parasitic effect |
227
|
Cipangopaludina chinensis
|
AgNPs |
20–60 |
20 and 60 mg L−1 |
14 d |
↑Bioaccumulation on biofilm. NP impacts on ecological receptors and food chains |
170
|
Biomphalaria glabrata
|
AgNPs |
115.17 ± 55.57 |
1.0, 2.5, and 5.0 mg L−1 |
30 d |
↓Reproduction rate; ↓egg per egg masses, ↓egg masses production per snail |
106
|
Lymnaea stagnalis
|
AgNPs |
10.3 ± 3.4 |
25 nM L−1 |
24 h |
The presence humic acid ↑uptake AgNPs PVP in contrast with cysteine but did not eliminate uptake of 25 nM L−1 |
228
|
12.8 ± 4.4 |
Lymnaea stagnalis
|
AgNPs |
100 |
5, 10 and 50 mg L−1 |
72 h |
↑Memory formation (10 mg L−1). Blocks memory formation (50 mg L−1). Memory recall is context-specific, thus snails trained in AgNPs do not |
171
|
Physella acuta
|
AgNPs |
24–190 |
0.001, 0.01, 0.1, 1, 10, 100 mg L−1 |
96 h and 28 d |
↑Mortality; ↓egg production; ↓snail size at first reproduction, ↑behavior changes |
176
|
Potamopyrgus antipodarum
|
AgNPs |
15 |
0.10–1000 mg L−1 |
28 d |
↓Reproduction. AgNPs in low concentrations can modulate 17a-ethynylestradiol activity |
229
|
Potamopyrgus antipodarum
|
AgNPs |
13 |
100 μg g−1 |
2 wk |
↓Growth, ↓reproduction |
230
|
Racesina luteola
|
AgNPs |
32.4 ± 2.6 |
4.01, 12.03 and 24.05 mg L−1 |
96 h |
↑Oxidative stress; ↑CAT; ↓GSH; ↓GST; ↓GPx; ↑MDA; ↑DNA damage |
231
|
Biomphalaria alexandrina
|
AuNPs |
— |
100–200 mg mL−1 |
24 h |
Modulation and prevention of the infectivity of cercariae and miracidia |
227
|
Bellamya aeruginosa
|
CuONPs |
41.6 ± 4.6 |
180 mg g−1 |
28 d |
↑Oxidative stress, ↑SOD, ↑CAT, ↑GST, ↑MDA |
232
|
Potamopyrgus antipodarum
|
CuONPs |
6 ± 1 |
0, 30, 60, 120 and 240 mg g−1 |
8 wk |
↓Growth rate, ↓feeding rate, ↓reproduction, and ↑bioaccumulation |
122
|
Bellamya purificata
|
CeO2NPs |
25 |
60 mg L−1 |
15 d |
High bioaccumulation factor. No mortality |
233
|
Lymnaea stagnalis
|
CuONPs |
7 |
Db: 4–50 mmol g1 and 50–175 nmol g−1 |
3–5 h (Db) |
Bioaccumulation associated to toxicity. Toxicity: Db exposures > Wb exposure |
125
|
Wb: 4–16 nM to 31 mM |
24 (Wb) |
Racesina luteola
|
CuONPs |
43.5 ± 1.5 |
7 and 21 mg L−1 |
5 d |
↑Oxidative stress; ↓GSH, ↓GPx, ↓GST. ↑LPO, ↑SOD (lower concentration, 1 d); ↓SOD (5 d). ↓CAT (2 d); ↑CAT (5 d, lower concentration). DNA damage mediated by oxidative stress |
168
|
Bellamya aeruginosa
|
CuONPs |
10 |
180 μg g−1 |
7, 14, and 28 d |
↑SOD, ↑CAT, and GST↑ (7 d), ↓SOD, ↓CAT, and ↓GST (>14 d) |
27
|
Biomphalaria glabrata
|
CdTeNPs |
3 |
50, 100, 200, 400 nM |
24 h |
Malformations and mortality of embryos and adult snails depending on the concentration. ↑Cytotoxicity (hemocyte apoptosis) |
234
|
Biomphalaria glabrata
|
γ-Fe2O3NPs |
5.7 |
1.0, 10, 100 mg L−1 |
10 d |
No effect on fecundity, fertility, mortality of adults, similar hatching rate, no malformation in embryos |
177
|
28 d |
Biomphalaria alexandrina
|
SiO2NPs |
80 |
50, 100, 200, 400, 600, 800, 1000, 1200 ppm |
3, 6, 12, 24, 36 h |
Non-embryonated egg masses (1400 ppm/24 h), embryonated pre-hatched one (1450 ppm/12 h). |
235
|
Bellamya aeruginosa
|
TiO2NPs |
11.6 ± 2.4 |
5 and 25 mg kg−1 |
21 d |
↑LPO, ↑PC, ↓Na /K -ATPase, ↑DNA damage |
236
|
Cipangopaludina chinensis
|
TiO2NPs |
5–10 |
1818.2 mg L−1 |
17 d |
Bioaccumulation through trophic transfer during plant consumption. ↑Uptake and bioaccumulation |
237
|
Cipangopaludina chinensis
|
TiO2NPs |
10–20 |
2 and 6 mg L−1 |
14 d |
Biomagnified through aquatic food chains. NPs show greater movement in the sediment than in the water in a simplified food chain. ↑Bioaccumulation in the semistatic exposition |
238
|
Racesina luteola
|
TiO2NPs |
34.1 ± 2.7 |
9 and 28 mg mL−1 |
7 d |
↓GSH, ↓GST, ↑MDA, ↓SOD, ↓CAT (9 mg mL), ↑CAT (28 mg mL). |
239
|
Racesina luteola
|
TiO2NPs |
34.1 ± 2.7 |
28, 56, 84 mg mL−1 |
96 h |
↓GSH, ↓GST, ↑Oxidative stress, ↑MDA |
172
|
Racesina luteola
|
ZnONPs |
22 |
10, 21 and 32 mg mL−1 |
96 h |
↓GSH, ↓GST, ↓GPx, ↑MDA, ↑CAT. genotoxicity mediated by oxidative stress |
166
|
Biomphalaria alexandrina
|
ZnONPs |
17.5 |
25–600 mg mL−1 |
24 h |
↑MDA, ↑NO, ↓GSH, ↓GST, ↓SOD, ↓PTN, ↓Alb,↑Ch, ↑AST, ↑ALT, ↑ALP,↑CAT |
169
|
21 d |
Table 3 Overview of the toxic effects of MNPs to crustacean according to species, and type of MNPs
Species |
MNPs |
Time |
End points |
Ref. |
Type |
Size (nm) |
Conc. |
Daphnia magna
|
AgNPs |
18.2 ± 10.1 |
0.5, 1, 3, 5, 10 μg L−1 |
48 h |
↑AChE, ↓ROS, ↑GSH, ↑CAT |
178
|
Daphnia magna
|
AgNPs |
6.3–8.4 |
50, 100, 200, 300 μg L−1 |
21 d |
↑Mortality (dose–effect) |
240
|
Gammarus fossarum
|
AgNPs |
20, 23 and 27 |
1, 3 μg L−1 |
72 h |
↓Haemolymph osmolality, no significant in antioxidant responses, defense mechanisms, cellular damage, energy reserves and ventilatory activity |
180
|
Daphnia magna
|
AgNPs |
40 and 110 |
2 μg L−1 |
24 h |
Citrate-coated AgNPs were more toxic than PVP-coated AgNPs, and 40 nm AgNPs were more toxic than 110 nm AgNPs |
76
|
Gammarus fossarum
|
AgNPs |
40 |
0, 0.5, 5 μg L−1 |
15 d |
↑Catalse and chitinase gene expression, ↑digestive lysosomal system, ↓locomotor activity |
23
|
Gammarus fossarum
|
AgNPs |
20, 40 and 80 |
1, 2, 4, 8, 10 μg L−1 |
72 h |
↑CuZnSOD gene expression |
241
|
Daphnia lumholtzi
|
AgNPs |
9.8 ± 0.8 |
0.1, 0.5, 1, 2, 5 μg L−1 |
21 d |
↑Time to first brood, ↓number of offspring per female, ↓survival |
183
|
Daphnia lumholtzi
|
AgNPs |
9.8 ± 0.8 |
0.2, 0.5 μg L−1 |
21 d |
↓Reproduction rate |
242
|
Ceriodaphnia cornuta
|
AgNPs |
— |
4, 5, 10, 15 and 20 μg ml−1 |
24 h |
↑Mortality rate, ↑DNA damage |
243
|
Moina macrocopa
|
AgNPs |
20 and 40 |
0.011 and 0.022 mg L−1 |
48 h |
↓AChE, ↓SOD, ↑CAT, ↑GST, ↓trypsin activity, ↓β-galactosidase activity, ↑phosphatase activity |
179
|
Paratya australiensis
|
AgNPs |
10.56 ± 2.27, 9.27 ± 1.29, 13.68 ± 0.76 |
30 μg L−1 |
28 d |
↑TBARS, ↑CAT |
138
|
Ceriodaphnia cornuta
|
AgNPs |
23 ± 2 |
10, 20 40 and 50 μg L−1 |
24 h |
↑Mortality, abnormal swimming, ↓heart rate, ↓thoracic limb movement |
186
|
Daphnia magna
|
AgNPs |
65 |
3.5, 8.1, 0.43, 1.05 μg L−1 |
24 h |
↓Sensory development, damage repair genes |
244
|
Daphnia magna
|
AgNPs |
5–50 |
10, 20, 30, 40 and 50 μg L−1 |
48 h |
↓Survival |
233
|
Cypridopsis vidua
|
AgNPs |
— |
10, 50, 150, 250, 350, 450, 550 and 1000 mg L−1 |
48 h |
↑Immobilization |
245
|
Ceriodaphnia cornuta
|
Sn-AgNPs |
10–50 |
1, 2, 5, 10, 20, 30, 40 and 50 μg ml−1 |
24 h |
↑Mortality, abnormal swimming behaviour |
89
|
Daphnia magna
|
Al2O3NP |
<50 |
3.12, 6.25, 12.5 and 25 mg L−1 |
21 d |
↓Survivors, ↓body length, ↓age at first brood, ↓neonates per surviving adult, ↑ROS, ↑CAT, ↓SOD, ↓GSH, ↑MDA, ↓average swimming distance of neonates (48 h) |
184
|
Daphnia magna
|
CeO2NPs |
5 |
10 and 100 μg L−1 |
48 h |
↓CAT (ceria@chitosan type), ↓GST (ceria@alginate type), ↑ROS (ceria@alginate type), ↑swimming activity, ↑swimming velocity (ceria@alginate type) |
182
|
Daphnia sp.
|
CuONPs |
45 ± 3 |
0.1, 1, 5, 10 and 25 mg L−1 |
24 h |
↓Number of motile counts |
246
|
Daphnia magna
|
CuONPs |
<50 |
0.5, 1, 1.5, 2, 2.5 and 3 mg L−1 |
120 h |
↓Survival |
247
|
Daphnia magna
|
CuONPs |
<50 |
0.07 and 15 mg L−1 |
14 d |
↑Mortality (Wb), ↓average number of neonates produced per adult at high concentration (Fb), ↓total number of broods produced per adult (Wb) at the high concentration, ↓total number of broods produced per adult (Fb) at the low concentration |
126
|
Daphnia magna
|
CuONPs |
110.34 ± 56.58, 38.27 ± 23.05 |
0.01, 0.05, 0.1, 0.5, 1, 2, 3, 5, 25, 50, 75 and 100 mg L−1 |
48 h |
↓Survival |
248
|
Daphnia magna
|
SiO2NPs, Fe3O4 NPs |
20–30, <20 |
5, 50, 100, 250, and 250 mg L−1 |
96 h |
↑Mortality rate |
249
|
Daphnia magna
|
TiO2NPs |
29 ± 8 |
1, 10, 100 ppm |
48 h |
↑Mortality, ↑swimming distance |
187
|
Daphnia magna
|
TiO2NPs |
<25 |
0.1 and 1 mg L−1 |
2 d |
No significant ROS increase and MT induction. |
250
|
Ceriodaphnia dubia
|
TiO2NPs |
9.5 ± 1 (anatase), 26 ± 3 (rod-shaped rutile) |
025, 0.5, 0.75, 1, 1.25, 1.5, and 1.75 toxic unit |
48 h |
↑Mortality rate |
251
|
Daphnia similis
|
TiO2NPs |
<25 |
1 and 10 mg L−1 |
96 h |
↓Growth rate |
181
|
Daphnia similis
|
TiO2NPs |
<25 |
7, 75, and 750 mg L−1 |
24 h |
↓CAT, ↓AP, ↓SOD |
181
|
Daphnia magna
|
ZnONPs |
20–40 |
0.009, 0.014, 0.027, 0.058, 0.131 mg L−1 |
21 d |
↓Average brood size, ↑time to first brood, ↓broods per female |
252
|
Daphnia pulex
|
ZnONPs |
61 ± 12 |
0.06 mg L−1 |
24 h |
↓Na+ /K+ ATPase, ↓RNA-binding protein, ↓rRNA methyltransferase, ↓signal recognition particle receptor, ↓signal peptidase |
253
|
Daphnia magna
|
ZnONPs |
10–30 |
0.2, 1, 5, 10, 25, 50 ppm |
72 h |
↓Alive account |
103
|
Daphnia magna
|
ZnONPs |
<50 |
0.1 and 0.3 mg L−1 |
21 d |
↓Survival probability |
254
|
Daphnia magna
|
ZnONPs |
63 ± 11 |
0.1 mg L−1 |
14 d |
↓Survival, ↓body length and embryo numbers of the first brood, ↓SOD, ↓GST, ↓CAT, ↓MDA |
124
|
4.1 Bivalvia molluscs
Bivalve molluscs are used as sentinel species for nanotoxicology owing to their high ability for the cellular internalization of MNPs. Table 1 describes the different bivalve species used in nanotoxicology studies and their toxic responses. Most articles reported the concentration effect in freshwater bivalves, focusing on short-term toxicity with an exposure time between 2 h and 14 d. Numerous studies indicate that MNPs impose bivalves' toxicity mainly through the functional parameters, such as immunotoxicity, oxidative stress, DNA damage, lysosomal damage in bivalve tissues, and protein expression changes (Table 1). In particular, the bivalve immune system represents a significant target for MNPs.110 Biochemical parameters are widely used to monitor the physiology of aquatic species to assess the impact of MNPs as early-warnings biomarkers. In invertebrates, parameters involved in antioxidant defenses (e.g., glutathione peroxidase, GPx; superoxide dismutase, SOD; catalase, CAT), oxidative stress (e.g., lipid peroxidation, LPO), and detoxification (e.g., glutathione S-transferases, GST) are commonly analyzed.142 In the literature, hepatopancreas, digestive gland, gonad, and hemocyte cells are the frequently tested organs to assess the effects of MNPs (e.g., AgNPs, CeO2NPs, CuONPs, and TiO2NPs).
Currently, reactive oxygen species (ROS) and free radical production may account for the mechanism of cytotoxic effects exerted by MNPs in bivalves.143,144 The MNPs may release extracellular metal ions, which penetrate the cell and induce oxidative stress by free radicals or ROS production and/or metallothionein (MT) induction. The oxidative stress induced by MNPs mainly includes the disruption of the antioxidant defense system (SOD, CAT, GPx, GST),24,37,145–148 LPO,149–152 increased protein modification (e.g., ubiquitination),150 and DNA damage (DNA strand breaks).150,152,153 Oxidative damage induced by MNPs in bivalves depends on the size, composition, concentration, and exposure time (Table 1). The MNP's size is the dominant factor determining the oxidative stress change and is associated with its high surface area. For example, 80 nm AgNPs induced greater MT levels, LPO, and DNA strand breaks in the digestive gland of freshwater mussel Elliptio complanata, compared to 20 nm AgNPs.152 The above result suggests a more critical release of dissolved Ag from larger AgNPs. However, the relationship between the hydrodynamic diameter and morphology of MNP aggregates and oxidative stress in bivalves has not been well-established. Notably, previous studies also showed that smaller sizes could enter cells via endocytosis more easily than larger sizes,76,154 and might generate severe effects on organisms.76,155 Oxidative stress induced by MNPs also depends on types of tissues and cells. For example, the gills of C. aegyptiaca are more susceptible to oxidative stress induced by AgNPs than the hepatopancreas.145
The immune system of bivalves is a sensitive target of MNP toxicity. Hemocytes are the most investigated cell type among the analyzed species (Table 1). Generally, upon exposure and crossing the epithelium of digestive gland tubules, MNPs can translocate from the digestive system into the circulatory hemocytes.143 Furthermore, the endocytic and lysosomal pathways are the major subcellular fate of MNPs in bivalve species.156 For example, TiO2NPs have been demonstrated to internalize into the hemocytes of the golden mussel Limnoperna fortunei, which can penetrate and be phagocytosed by hemolymph cells, being able to damage the hemocyte membrane.157 The TiO2NPs can induce a redox imbalance in mussel cells, decrease SOD and CAT activities, and induce protein sulfhydryl content decrease after TiO2NP exposure.157 MNPs induce ROS production that leads to changes in the immune system due to inflammatory processes (reduction in phagocytic activity and hemocyte viability). Changes in phagocytosis activity, cell viability/density, stimulation of lysosomal enzyme release, ROS production, mitochondrial damage, and DNA damage were observed in bivalve hemocytes after exposure to different ENMs, such as AgNPs, CuNPs, and TiO2NPs.37,148,158,159
Behavioural biomarkers, such as the feeding rate and valve opening, are essential tools to assess the MNPs' toxicity in bivalves. As exposure concentrations of AgNPs are elevated, the ammonia excretion and feeding rates of C. fluminea diminished initially and then increased.160 This tendency indicated that lower concentration exposures (0.1 and 0.5 mg L−1) induced ROS accumulation in the body, which resulted in a certain degree of oxidative damage in cells. In contrast, the organisms' antioxidant enzyme defense system had not yet been activated. When the concentration of AgNPs increased to 2 mg L−1, the antioxidant enzyme defense system produced many enzymes to eliminate ROS, protecting the body from oxidative damage and increasing feeding and excretion capacity.160 Bioenergetic-related traits provide essential advantages for environmental stress assessment as they permit integration of the physiological effects of environmental stressors with different mechanisms of action and provide a direct link between the physiological change and the organism's fitness.161 ZnONP exposure (14 d) significantly decreased the glycogen, glucose, and lipids of U. tumidus.162 Meanwhile no damage to proteins and lipids was found in marine clam Ruditapes philippinarum subjected to environmentally relevant ZnONP concentrations for a seven day exposure period.163 The species difference may reflect a short exposure time insufficient to trigger a stress response in the latter study. It also emphasizes the need for more investigations of long-term exposures to MNPs to assess their biological and toxic impacts.
4.2 Gastropod molluscs
Toxic effects in gastropods induced by MNPs depended on the size, aggregation capacity, target cell and tissue. In general, small MNPs have a high surface area and dissolution potential, enter the cell membrane, and cause oxidative stress.76 On the other hand, small particles may also tend to aggregate or be more readily absorbed by organic matter or the sediment present in the environment.42 For example, the toxicity of larger AgNPs (40 and 80 nm) to B. aeruginosa was higher than small AgNPs (20 nm) after a 14 d exposure period.24 The small CeO2NPs (3.8 vs. 185 nm) induced a high accumulation rate and trophic transfer potential since small MNPs were more bioavailable in the water column.164 In contrast, the larger MNPs remained associated with the sediment.
The primary mechanism of action and toxicity of MNPs to gastropods is mainly associated with the oxidative stress-related mechanism.16 As we reviewed in Table 2, oxidative stress is the primary effect in gastropods among the toxicity induced by MNPs. MNPs led to an imbalance between ROS production and the capacity of the antioxidant defense system (SOD, CAT, GPx, and GST), and increased LPO of different tissues (e.g., hepatopancreas, digestive gland, mantle) and hemolymph cells24,165–167 (Table 2). Furthermore, MNPs can also induce protein carbonylation (PC),167 increase the total lipids and cholesterol levels as well as aspartate aminotransferase (AST), alanine aminotransferase (ALT), and alkaline phosphatase (ALP),167 inhibit the Na+/K+ ATPase,27 and induce DNA damage.165,166,168,169 The oxidative damage induced by MNP exposure in gastropods also was concentration and exposure time-dependent, which has been demonstrated for AgNPs, CuONPs, TiO2NPs, and ZnONPs.24,27,170,171 Generally, prolonged exposure and higher concentration of MNPs induced more significant oxidative damage. For example, short-term exposure (7 d) to CuO-NPs led to oxidative stress of freshwater snail B. aeruginosa. In comparison, long-term exposure (>14 d) led to oxidative damage, which means prolonged exposure will enhance the MNPs' ecotoxicity risk to organisms.27 Moreover, exposure under high concentration (21 μg L−1) induced more significant oxidative stress of freshwater snail Lymnaea luteola than 7 μg L−1.172 Cell and tissue-specific responses to oxidative damage induced by MNPs were reported for snails. Among the most studied organs concerning oxidative stress induced by MNPs is the digestive gland, possibly due to its higher accumulation capacity and role in metal detoxification. The digestive gland of B. aeruginosa exposed to AgNPs was more susceptible to oxidative stress than gonads, visceral mass, and foot/muscle.24 A similar tissue-specific response also was observed in B. aeruginosa exposed to CuONPs.167 The hemocytes are immune cells representing the first line from external stressors by rapidly initiating the immune response. Hemocytes are also well-known as the most studied cells to assess the effect of oxidative stress caused by MNPs. The hemocytes of B. Alexandrine demonstrated oxidative stress after ZnONPs, while the visceral mass response was observed only at the highest level.
Another possible toxicity mechanism of MNPs is linked to the release of metal ions in freshwater. However, the Croteau group predicted that around 80% of the bioaccumulation of Cit-AgNPs by L. stagnalis was driven by uptake of particulate Ag.173 The freshwater snails L. stagnalis and Physa acuta exposed to waterborne Ag showed comparable uptake rate constants for Ag+ and AgNPs.128,174 However, notably, the Ag+ elimination rate was not as high as the AgNP form, suggesting that the ion form may have more time to trigger the stress.174 Unlike the endocytosis pathway for MNPs, the uptake mechanism of the metal ion is mainly via ion transport channels, such as the proton-coupled Na+ channels.127 The uptake pathway is a vitally important factor in determining the intracellular fate and toxicity of the AgNPs in the estuarine mud snail Peringia ulvae.120 MNPs usually are endocytosed by the clathrin-mediated pathway, which directs towards lysosomal degradation.120 Meanwhile the conclusive demonstrations of intracellular fates of metal ions remain elusive.
Fecundity has been suggested to be the most sensitive endpoint to assess the likely effects of contaminant exposure in freshwater organisms. For egg-clutches per snail Biomphalaria glabrata, there was significant inhibition after AgNP exposure.175 Similar low egg production was observed in the snail Physa acuta under AgNP exposure.176 In P. antipodarum, after nine-week CuONP exposure, ∼70% of the snails stopped their reproduction.118 On the other hand, the hatching success of the snail P. acuta was more sensitive to silver in the ionic form than the AgNP exposure.175 The egg masses have mucous components, and compared with AgNPs, Ag+ may penetrate through the egg mass membrane more easily, while AgNPs may be embedded on the egg mass surface which lowered their penetration.106 However, γ-Fe2O3 NPs generate no effects on the fecundity, hatching rate and mortality of B. glabrata, and no malformation in embryos.177
4.3 Crustacean arthropods
AgNPs and Daphnia spp. were the most studied MNPs and crustacean arthropod species, as summarized in Table 3. At present, most of the results have been analysed following the OECD guidelines for the short-term in vitro assay method. Primary outcome measures include mortality, time to first brood, number of offspring per female, reproduction rate, and swimming behaviours (Table 3). Biomarkers for early-warning purposes are also extensively used (Table 3).
One of the most widely accepted toxicity mechanisms proposed for AgNPs is the generation of ROS, which can lead to lipid peroxidation inducing, finally, oxidative stress.178 Implementation of biochemical biomarkers (e.g. SOD, CAT and GST) is considered a promising tool for ecotoxicological applications as early warning indicators, which play a significant role in relieving ROS-associated stress. AgNPs enhanced the SOD, CAT and GST activity of Moina macrocopa.179 Similar results were also found in D. magna under AgNP exposure.178 Meanwhile no significant alterations in antioxidant responses, defense mechanisms and cellular damage were be detected in G. fossarum.180 In addition, many other opposite results have also been reported.124,181,182 An apparent increase in AChE activity in D. magna and M. macrocopa was observed upon exposure to AgNPs.178,179 However, CeO2 NP exposure did not affect the AChE activity in D. magna.182 Whether the alteration of antioxidative stress enzymes affects freshwater invertebrates' cholinergic system needs further research.
AgNPs enter into an aquatic system mainly through ingestion. The overall growth is closely associated with its digestive capacity to break food into small absorbable molecules in the digestive tract, and digestive enzymes perform this task. For example, AgNPs inhibited the digestive enzymes (trypsin, amylase and β-galactosidase) of M. macrocopa. Moreover, AgNPs of 40 nm cause more hazards to the digestive enzyme than NPs of 20 nm. This is likely due to the rapid clearance of smaller AgNPs from the digestive system compared to the large ones.179 Experiments examining chronic stress and its impacts on Daphnia spp. have focused on growth and reproduction. MNP exposure can lead to short body length, delayed breeding time, decreased offspring, etc.126,183,184 The MNPs can physically damage D. magna by adhering to surfaces such as tentacles, skin, and cases through physical adsorption. For example, TiO2 NPs have also been attached to the D. magna shell, forming a stable ‘shell layer’ that affects the molting process.36
Moreover, after 21 d of exposure, the longevity, growth, and reproduction of D. magna decreased significantly with increasing Al2O3 NP concentration from 6.25 to 25 mg L−1.184 The survival, growth and reproduction of Daphnia lumholtzi were also reduced after AgNP exposure from 0.1 to 5 μg L−1 for 21 d.183 Considering that dietary exposure may pose different effects than direct routes, the toxic effects of CuONPs on D. magna were evaluated for two chronic exposure scenarios, i.e., indirect feeding and direct waterborne exposure.126 The results evidenced that the total number of broods produced per adult decreased in the direct exposure and feeding direction. This indicates that CuONP exposures could impact the reproduction of D. magna regardless of the exposure scenario.
The swimming behaviour of Daphnia could be an excellent biomarker in toxicity assessment.185 Decreased swimming activity, shorter cumulative distance and loss of trajectory orientation of swimming were observed in D. magna.89,182,184,186,187 Such behaviour damage could be related to the interactions of MNPs with the carapace of the daphnids and perhaps related to the high energy demand and nervous system disorders.185 The decreased swimming capacity after exposure to MNPs may indicate that the daphnids are directing energy to conserve processes essential to neutralize the toxicity of the MNPs, leading to reduced feeding and a decrease in growth and reproduction.185
In summary, the toxic effects of MNPs on freshwater invertebrates are influenced by the type, size, concentration, morphology of the MNPs and exposure time. The mechanisms of toxicity are currently characterized by ion release and the small size effects of MNPs. In addition, it is also related to the physiological properties of the test organisms themselves, as different species could pose different tolerances to nanomaterials, which induce different organismal defense systems. The overview of MNP bioaccumulation with three major freshwater organisms induced by environmental transformation and the mechanisms of cell damage by MNPs are illustrated in Fig. 2.
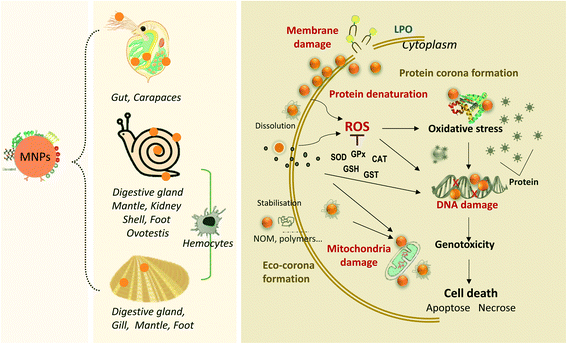 |
| Fig. 2 Overview of MNP bioaccumulation with three major species of freshwater organisms and the mechanisms of cell damage by MNPs (damage of membranes, DNA and mitochondria; lysosome dysfunction, generation of reactive oxygen species, ROS, disturbance of protein functions). | |
Remarkably, when assessing the deleterious effects MNPs, the above results are more based on laboratory simulations.18,104,158,182 However, complex environmental factors (e.g., pH, NOM, IS, ligands) in natural waters make the toxicity results more complicated than the laboratory culture medium. Very few researchers have addressed the comparison of toxic effects of MNPs in different test media. One recent study investigated the toxicity of AgNPs to D. magna in culture medium (M4) and surface water.188 They found that AgNPs exhibited lower toxicity in surface water than in M4 medium, showing a higher median effect concentration (EC50) within 48 h. Furthermore, a higher survival rate was observed in the surface water medium after 21-day exposure.
As mentioned, NOM may contribute to the aggregation of MNPs via coating or binding, and di- and multi-valent cations in natural water may also enhance the aggregation.42,60 Moreover, NOM exhibited strong Ag+-binding capabilities, protecting the body from injuries caused by AgNPs and free Ag+.189,190 Similarly, TiO2NPs and AgNPs in the standard culture medium displayed more severe toxic effects (e.g., impaired growth, higher mortality, and lower reproductive access) on D. magna than those in synthetic European Class V lowland water.191 The above results indicate that a suitable medium plays an important role when accessing the potential hazards of MNPs. Based on limited information, many presently available research studies may underestimate the truth, and additional work is required to understand possible mechanisms of toxicity in real exposure scenarios.
In toxicology experiments, exposure concentration is critical to evaluate physiological endpoints. A recent review summarizes the measured environmental concentrations (given by ng L−1) of several common MNPs.192 It could be argued that the exposure concentrations used in most of the existing studies are too high to be of physiological significance. Notably, given that MNP concentration in natural environments is forecast to increase drastically by the next century,193,194 MNP pollution may become a major threat to organisms in the future. Thus, more attention is required. High concentration exposure provides implications on further environmental change, and the environmental-related concentration scenarios can inform us of current MNP pollution governance. Recent technological breakthroughs in single-particle ICP-MS (SP-ICP-MS) have allowed the analysis of size as small as 4.9 nm for AgNPs and concentration as low as 27 particles per μL.195 Without technical limitations, environmental-related exposure is encouraged to be conducted to better reflect more plausible results.
5. Case study: understanding the effect of AgNP transformation on the toxicity in freshwater invertebrates by meta-analysis
As mentioned above, studies on the toxic effects of MNPs clarified that ecotoxicity is dependent on the intrinsic properties of MNPs, the composition of the environmental media, and the experimental ways (waterborne and dietborne). Results of the different assays of toxic responses to MNPs are sometimes controversial. It is worth investigating the causes of inconsistent results from experiments. The meta-analysis includes independent results, is an approach to explore a correlation between target variables and toxic responses, and identifies the main variables that potentially contribute to heterogeneity in conclusions. We selected studies in 2011 to include in our meta-analyses via Google scholar, using the term “Freshwater invertebrate AND Nanoparticle OR Bivalve OR Gastropoda OR Crustacean OR viability OR reproduction OR metabolic stress OR physicochemical barriers OR Immunocytes OR Stem cells OR Protein corona OR Cytokine-like protein OR Omic.” This initial search yielded 1320 papers, 260 duplicates, and 621 citations after the title and abstract screen were excluded. 1060 full texts were assessed, and 84 articles were identified to be related to quantitative experimental research on MNPs (Fig. 3). Of these, AgNPs are of particular interest in the MNP-related toxicological profile (Fig. 4). On top of AgNPs, TiO2 NPs, ZnONPs, and CuO NPs also attract considerable attention. Based on these research studies, we chose AgNPs as a case study and finally, 14 articles were included for meta-analysis. Oxidative stress is convenient in measuring ecotoxicity because cells respond to oxidative stress by exerting several protective responses measured by enzymatic or genetic expression responses.196
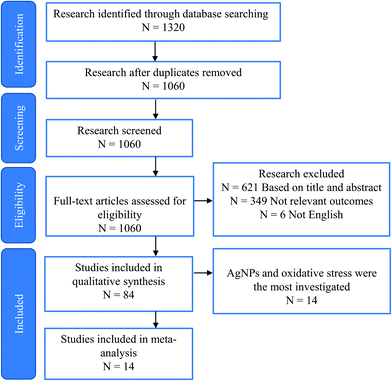 |
| Fig. 3 Flow chart of the study selection process. | |
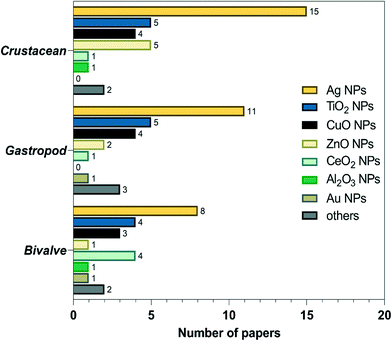 |
| Fig. 4 MNP research areas sorted by the number of articles published. | |
During data assessment, when the original data from the experiment could not be referred to in the article, the numerical values were extracted by reading the graphs with a digital ruler (GetData Graph Digitizer). We extracted the following information from each study: publication characteristics (title of the study, first author and publication year), data on the experimental and control groups (n, mean, SD), the species, type, size, and concentration of NPs, and exposure period. A complete list of information extracted is included in Table S1.†
A random-effects model was chosen for the meta-analysis. Continuous variables were estimated as standardized mean differences (SMDs) with 95% confidence intervals (95% CI) between the experimental and control groups. The absolute effect sizes (SMDs) was interpreted as follows: SMD < 0.2 = “negligible”, SMD 0.2 to 0.5 = “small”, SMD 0.5 to 0.8 = “medium”, and SMD > 0.8 = “large”, as per Cohen's classification.197 The heterogeneity between studies was assessed using the Chi-squared test, with inconsistency index (I2) > 50% and p < 0.1 considered significant heterogeneity.198 Meta-regression analyses were also conducted to identify independent sources of between-study heterogeneity. Then subgroup analyses were performed in terms of MNP size, concentration and exposure time. Funnel plots were used to investigate the existence of publication bias in studies with a total of more than ten included data. All analyses were implemented in Stata MP 16.0 (Stata Corp., College Station, Texas, TX, USA, 2017).
Analyses showed that ROS, SOD, CAT, GSH, LPO and AChE levels were higher in the experimental group than in the control group with medium effect sizes (SMD 0.52–3.99, p = 0–0.008, I2 = 41.3–93.7%). In contrast, GST and GPx were lower than controls also with large effect sizes (SMD −1.12–−1.47, p < 0.001, I2 = 85.6–86.5%) (Table S2†). Based on the significant statistical heterogeneity observed for almost all the biomarkers (I2 > 50%), the meta aggression results identified a specific heterogeneity source for each biomarker (Table S1†). Among the various potential covariates, the size, coating of AgNPs, exposure concentration, and time were associated with study heterogeneity (Table S1†). The subgroup analysis explored the source of heterogeneity by the particle size of AgNPs (<30 nm and >30 nm), coating (CIT, PVP, tyrosine and bared), concentration (<30 μg L−1 and ≥30 μg L−1), and exposure time (<14 d and 14 d) (Table S2†). The SMD values of ROS and CAT indicated that they had been induced more by AgNPs with >30 nm than smaller sizes (p < 0.01, Fig. S1a and c†). It was worth noting here that the SMD value for ROS is as high as 16.86.
In terms of coating, PVP and tyrosine decreased the AChE activity with large SMD values (−8.34 and −8.68, respectively), while Cit and bare enhanced AChE also with large SMD values (0.8 and 1.72, respectively) (Fig. S1h†). According to our results, lower exposure concentration (<30 μg L−1) triggered higher toxicity in GST (p < 0.01, Fig. S1d†), while higher concentration (≥30 μg L−1) had higher activity for inducing a rise in the LPO (p < 0.01, Fig. S1g†) and decrease in GPx (p < 0.01, Fig. S1f†). Our result showed that SOD and GSH in freshwater invertebrates are mainly affected by the exposure duration, in which acute exposure time (<14 d) produces a more drastic decrease in SOD activity (p = 0.003, Fig. S1b†) and GSH activity (p < 0.01, Fig. S1e†).
Lipid peroxidation damage marks the oxidative stress endpoint, which was observed when the exposure concentration was higher than 30 μg L−1. The absent toxicity of lower exposure concentration could be reasoned with the high tolerability of organisms. On the other hand, a short exposure time in these studies might also be a part of the explanations. However, the occurrence of toxic effects is a complex process influenced by many factors. Ideally, prolonging the exposure could exert higher toxicity with increased probability of organism-MNPs contact. However, these results could also be overestimated, and the accumulation and elimination process could co-occur with increasing concentration. For example, a recent study reported the elimination rates of D. magna as the ZnONP concentration increased.103 However acute responses are usually determined simultaneously and thus do not track the cumulative exposures over time.199 This evaluation enables the prioritization of providing information for pollution risk assessment. Indeed, limited information is available from such data without considering the potential chronic effects. Thus, short time and prolonged exposure for months or even an entire life cycle is needed to characterise acute and chronic toxicity.
ROS generation is an appropriate parameter considered as one of the potential mechanisms for MNP toxicity in freshwater organisms. In our meta-analysis, size and time are the most influential drivers affecting ROS production (Table S1†). In terms of physical properties, the decrease in the particle size of MNPs usually leads to changes in the crystalline surface structure, increase in the specific surface area, and curvature. In terms of chemical properties, the decrease in particle size of MNPs increases the density of surface functional groups, surface energy, and surface charge density. The surface energy and surface charge density also increase.200 These changes lead to the excess energy and surface activity of MNPs, leading to interfacial reactions. It was found that the pore sizes of biological membranes such as cell membranes (0.4–1 nm) and nuclear membranes (50–70 nm) are mainly in the nanometer range,201 and the diameter of cell wall pores is also in the range of 5–20 nm.202 Theoretically, when the particle size of MNPs is smaller than the size of cell wall pores and biofilm micropores, MNPs can directly enter the cell and even the organelle.203 Smaller size MNPs are more quickly absorbed by organisms, thus accumulating more in the organism, leading to more toxic results. For example, AgNPs of 20 nm were found to be more toxic than 40 nm with a lower LC50 value.179
Although smaller-sized nanoparticles have a larger surface area, the evolution of MNPs (uptake, aggregation, elimination) inside the body of an organism under study is a crucial determinant of the toxicity of variably sized nanoparticles.204 Snails B. aeruginosa exposed to 40 nm and 80 nm AgNPs were found to show significantly enhanced oxidative stress compared to those exposed to 20 nm AgNPs.24 This comprises our present meta-analysis. It can be explained by the size similarity of AgNPs around 20 nm (18.0 ± 7.7 nm) and the sediment (14.60 ± 0.19 nm), and the NPs being adsorbed readily into sediment particles, which reduces the bioavailability.24 MNPs are greatly influenced by the specific NOM type or other natural particles (e.g., colloids) present in freshwater. The smaller-sized NPs reacted rapidly with the substances in the solution, causing aggregation, which may have caused lower toxicity.7 The mechanism behind this higher toxicity triggered by larger size remains more fully elucidated.
6. Development of MNP AOPs in freshwater invertebrates
Emerging numbers and diversity of chemical pollutants are urgently needed for toxicological profile access with higher speed and accuracy, lower resource consumption and fewer experimental animals.205 To address this challenge, a novel conceptual framework called the adverse outcome pathway (AOP) was proposed in 2010 by the US Environmental Protection Agency205 and adopted in 2012 by OECD for ecological risk assessment.206 The AOP framework focuses on identifying the biologically plausible and empirically supported links between molecular-level perturbations of a biological system caused by a stressor and an adverse outcome at a higher level of biological organization (i.e., an organism or population).206 Generally, the elements of the AOP include: 1) a molecular initiating event (MIE) where a stressor interacts with a biomolecule to create a perturbation; 2) a series of related key events (KEs) at the cellular, tissue, and organ levels that are caused by the MIE and are essential for the progression to an adverse outcome; and 3) adverse outcome (AO) at the organismal or population levels.7,205 The MIE is known to directly trigger ROS production for nanoparticles, one of the most significant reasons for adverse MNs effects. Likewise, oxidative stress is a known contributor to MNP-induced cell damage and toxicity.7,16,141 Possible AO may be causally related to key events (KEs), for example, MPs may lead to sequential interactions at the molecular (e.g., LPO), cellular levels (e.g., DNA damage, gene expression) and then the organ levels (e.g., altered physiology, organic function and histopathology). These KEs may contribute to the AOs at the organism level expressed (e.g., individual development, reproductive output and offspring viability) and population levels (e.g., altered structure or reduced recruitment).
The AOP framework has been well developed as a tool to identify key initiators and predict effects induced by nanomaterials in mammalian species207 and vertebrate species.208–211 For example, PVP-AgNPs and AgNO3 exposed freshwater fish Pimephales promelas both affected pathways involved in Na+, K+, and H+ homeostasis and oxidative stress, and the MIE of Ag exposure is ROS production.210 ROS production on gonad tissue of zebrafish was also identified as the MIE of AgNP exposure, and these were associated with the mitochondrion-mediated apoptosis pathway.211
Invertebrates perform essential roles in most ecosystems. Published papers have made progress in using invertebrate species as model organisms. However, MNPs' environmental impacts on invertebrates and toxicity mechanisms are yet to be fully elucidated, and the knowledge about using invertebrates in the development of AOPs remains scarce. The short life cycle offers valuable opportunities to study the impact of chemical exposure at environmentally relevant concentrations over regular periods.
To date, only a few invertebrate AOPs have been developed. Those are aimed at abundant organisms with short life cycles, such as planktonic crustaceans Daphnia, roundworm Caenorhabditis elegans, and common fruit fly Drosophila genus.212 A recent study developed two conceptual AOPs for hazard and risk assessment of ionizing radiation and associated radionuclides to D. magna.213 The studies indicated that multiple toxicity pathways were potentially involved in the γ radiation-mediated reproductive effects, such as the DNA damage-oocyte apoptosis pathway and the lipid peroxidation-ATP depletion pathway.213
AOPs for MNPs in environmental species are virtually absent in research papers, let alone the freshwater invertebrates. CuO NPs may generate adverse outcomes (i.e. reproduction impairment) to soil invertebrates Enchytraeus crypticus via affecting the Notch signalling pathway with consequences at cellular division and differentiation.214 Considering their fundamental importance in ecosystem structure and function, investigations using key invertebrate species are vital when obtaining data from which to develop AOPs. Several aquatic invertebrates were identified as potential model organisms such as D. magna; rotifer, Brachionus koreanus; copepod Tigriopus japonicus, and L. stagnalis due to the extensive genomics information available for these species.215,216
Omics technologies, defined as high-content datasets with measurements of genes, mRNA, proteins, and metabolites, have been proved by increased studies on the potential applications of omics for ecology risk assessments.217,218 It is thought-provoking how omics data can support a more predictive approach to the AOP framework, including facilitating the identification of molecular-level changes (e.g., MIEs and early KEs). In real-life scenarios, omics are deployed for discovering informative biomarkers in organisms. For example, the Antczak group used transcriptomics to reveal a calcium-dependent mechanism for narcotic chemical toxicity in D. magna.219 However, the application of omics in AOPs has not been widely investigated to date. Omics technologies, if properly applied, are likely to dramatically increase our ability to characterize from the molecular responses to the populational level. Hence, it may provide us with a unique opportunity to better recognize the consequences of MNP release into the environment. Fig. 5 shows the flow diagram illustrating an AOP, representing MNPs triggering molecular initiating events leading to a sequential series of higher order effects to produce an adverse outcome. As discussed above in section 4, the molecular biomarkers in MNP exposure of invertebrates can help identify different MOAs on the subcellular level and thus associate them with different AOPs in environmental risk assessment.
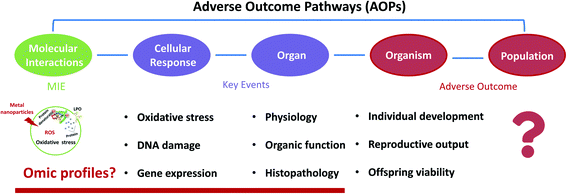 |
| Fig. 5 Flow diagram illustrating an AOP. The putative MIEs, KEs and AOs are illustrated based on the published research on the toxic mechanisms of nanomaterials (such as ZnONPs, CuONPs and AgNPs) in organisms. The cellular response (including oxidative stress, DNA damage and gene expression) and organ damage (i.e. physiology response, organic function and histopathology) are essential integrators of multiple upstream KEs. Upon the above effects, MNPs could eventually lead to sequential higher-order effects producing adverse outcomes (i.e. influencing the individual development, reproductive output and offspring viability). If NPs diminish the fitness of organisms, the population dynamics might be affected and possibly affect ecosystem stability and functioning. | |
7. Conclusion and perspectives
As MNP-based commodities are increasingly widespread, further studies are demanded on the effects, interactions, uptake, translocation and evolution of MNPs in organisms and the environment.220 Despite significant advances in understanding the toxicity of MNPs, the field is still in its infancy, with much to be investigated.
The present review summarizes the environmental impact of MNPs and potential toxicity endpoints in freshwater invertebrates. Once released into the environment, the MNPs are transformed from their previously prepared form. This transformation remains a challenging issue for the realistic evaluation of their ecotoxicity.221 Present studies show that different studies within the same subject vary enormously and even produce conflicting results. The reasons for these seemingly contradictory findings are diverse. Therefore, all possible factors should be considered as much as possible when conducting ecotoxicological studies on MNPs, e.g. different environmental conditions and pre-treatment methods of MNPs.
We summarise some factors that may contribute to differences in experimental results:
(1) For the same MNP type, the particle size, crystalline shape and morphology may be different from experiments.
(2) Different pretreatments lead to different degrees of polymerisation of MNPs in solution;
(3) Different solvents are used.
(4) Variation in experimental environmental conditions, such as temperature, pH, light intensity, etc.
(5) Experimental organisms being different subspecies, or in different life cycles, or different routes of administration.
As the MNP research goes deeper, the following five aspects deserve additional attention:
1) Toxicity testing was performed using bivalves, gastropods and crustaceans as bioindicators and suitable model organisms and they were widely used in acute toxicity studies. However, there exist differences in the physical–chemical properties between the natural water and lab culture medium, and toxicity research in natural water needs to be considered in future investigations.
2) The chronic exposure concentrations in research materials are higher than environmental-related concentrations. Moreover, the duration of MNPs' action in the natural environment is much longer than that simulated in the laboratory. Therefore, studies on the long-term effects of low concentrations of MNPs on organisms need to be strengthened.
3) The data on the bioaccumulation of MNPs by the above-discussed model organisms are not yet perfect, as the experimental exposure concentrations vary greatly, and the methods of representing the results are not uniform, making it difficult to compare the bioaccumulation capacity between studies. Hence, there is a need to establish a standard method for MNP bioaccumulation and to optimize the establishing exposure concentration criteria; additionally, MNPs are often not entirely excreted by organisms, and the morphological transformation, long-term chronic toxicity, intergenerational transfer and trophic transport of these residual MNPs need to be studied in depth.
4) The investigations for multiple changes allow a much better understanding of toxicological pathways than the single end-point approach. Hence, there is a call to use the AOP approach to mechanistically link the molecular and cellular phenotypes to adverse organismal outcomes, determine the role of environmental stress responses at the cellular and tissue levels, and translate them into the organism's fitness consequences.
5) Genomics, transcriptomics, proteomics and metabolomics have been applied in toxicology for more than a decade and the omics approach has only been deployed in aquatic invertebrates of MNP investigations in the last few years. The integration of multi-omics data shall lead to deeper biological insights and a more comprehensive understanding of toxic mechanisms of action. In addition, multi-omics data can improve the confidence of chemical dose extrapolation, facilitate chemical identification, taxonomic characterisation and screening, and play an essential role in identifying chemical-related exposure, effect and susceptibility markers.
Author contributions
All the authors have contributed to the discussions leading to the manuscript.
Conflicts of interest
The authors declare no conflicts of interest.
Acknowledgements
The authors acknowledge the financial support of the Swiss National Science Foundation under grant agreement number 197322.
References
- B. Klębowski, J. Depciuch, M. Parlińska-Wojtan and J. Baran, Applications of noble metal-based nanoparticles in medicine, Int. J. Mol. Sci., 2018, 19, 4031 CrossRef PubMed.
- M. J. Sweet, A. Chessher and I. Singleton, Metal-based nanoparticles; size, function, and areas for advancement in applied microbiology, Adv. Appl. Microbiol., 2012, 80, 113–142 CrossRef CAS PubMed.
-
S. R. Baker, OECD Health Data: Where does the US stack up?, Notes of a radiology watcher, Springer, 2014, pp. 69–72 Search PubMed.
- C. Peng, W. Zhang, H. Gao, Y. Li, X. Tong and K. Li,
et al., Behavior and potential impacts of metal-based engineered nanoparticles in aquatic environments, Nanomaterials, 2017, 7, 21 CrossRef PubMed.
- M. A. Saifi, W. Khan and C. Godugu, Cytotoxicity of nanomaterials: Using nanotoxicology to address the safety concerns of nanoparticles, Pharm. Nanotechnol., 2018, 6, 3–16 CrossRef CAS PubMed.
- R. Klaper, J. Crago, J. Barr, D. Arndt, K. Setyowati and J. Chen, Toxicity biomarker expression in daphnids exposed to manufactured nanoparticles: Changes in toxicity with functionalization, Environ. Pollut., 2009, 157, 1152–1156 CrossRef CAS PubMed.
-
S. Lekamge, A. S. Ball, R. Shukla and D. Nugegoda, The toxicity of nanoparticles to organisms in freshwater, Reviews of environmental contamination and toxicology, 2018, vol. 248, pp. 1–80 Search PubMed.
- B. Zhang, J. Chao, L. Chen, L. Liu, X. Yang and Q. Wang, Research progress of nanoplastics in freshwater, Sci. Total Environ., 2020, 757, 143791 CrossRef PubMed.
- J. R. Lead, G. E. Batley, P. J. Alvarez, M. N. Croteau, R. D. Handy and M. J. McLaughlin,
et al., Nanomaterials in the environment: Behavior, fate, bioavailability, and effects—an updated review, Environ. Toxicol. Chem., 2018, 37, 2029–2063 CrossRef CAS PubMed.
- T. Andreani, V. Nogueira, A. Gavina, S. Fernandes, J. L. Rodrigues and V. V. Pinto,
et al., Ecotoxicity to freshwater organisms and cytotoxicity of nanomaterials: Are we generating sufficient data for their risk assessment?, Nanomaterials, 2021, 11, 66 CrossRef CAS PubMed.
- N. B. Turan, H. S. Erkan, G. O. Engin and M. S. Bilgili, Nanoparticles in the aquatic environment: Usage, properties, transformation and toxicity—a review, Process Saf. Environ. Prot., 2019, 130, 238–249 CrossRef CAS.
- A. Baun, N. B. Hartmann, K. Grieger and K. O. Kusk, Ecotoxicity of engineered nanoparticles to aquatic invertebrates: A brief review and recommendations for future toxicity testing, Ecotoxicology, 2008, 17, 387–395 CrossRef CAS PubMed.
- N. Abramenko, P. Mashkin, S. Volkov, V. Olshanskiy and L. Kustov, Fresh-water mollusks as biomonitors for ecotoxicity of nanomaterials, Nanomaterials, 2021, 11, 944 CrossRef CAS PubMed.
- L. Canesi, C. Ciacci, R. Fabbri, A. Marcomini, G. Pojana and G. Gallo, Bivalve molluscs as a unique target group for nanoparticle toxicity, Mar. Environ. Res., 2012, 76, 16–21 CrossRef CAS PubMed.
-
OECD, 243: Lymnaea stagnalis reproduction test, OECD Guidelines for the Testing of Chemicals, Section, 2016, vol. 2 Search PubMed.
- M. B. Caixeta, P. S. Araújo, B. B. Gonçalves, L. D. Silva, M. I. Grano-Maldonado and T. L. Rocha, Toxicity of engineered nanomaterials to aquatic and land snails: A scientometric and systematic review, Chemosphere, 2020, 127654 CrossRef CAS PubMed.
-
OECD, Test no. 202: Daphnia sp. Acute immobilisation test, OECD Guidelines for the Testing of Chemicals, OECD Publishing, Paris, 2004, vol. 2 Search PubMed.
- J. R. Santos-Rasera, A. S. Neto, R. Monteiro, C. Gestel and H. Carvalho, Toxicity, bioaccumulation and biotransformation of Cu oxide nanoparticles in Daphnia magna, Environ. Sci.: Nano, 2019, 6, 2897–2906 RSC.
- K. Ruppert, C. Geiß, C. Askem, R. Benstead, R. Brown and M. Coke,
et al., Development and validation of an oecd reproductive toxicity test guideline with the mudsnail Potamopyrgus antipodarum (mollusca, gastropoda), Chemosphere, 2017, 181, 589–599 CrossRef CAS PubMed.
- K. L. Garner and A. A. Keller, Emerging patterns for engineered nanomaterials in the environment: A review of fate and toxicity studies, J. Nanopart. Res., 2014, 16, 1–28 CrossRef.
- C.-H. Lee and J. K.-H. Fang, Effects of temperature and particle concentration on aggregation of nanoplastics in freshwater and seawater, Sci. Total Environ., 2022, 817, 152562 CrossRef CAS PubMed.
- A. Arini, F. Pierron, S. Mornet and M. Baudrimont, Bioaccumulation dynamics and gene regulation in a freshwater bivalve after aqueous and dietary exposures to gold nanoparticles and ionic gold, Environ. Sci. Pollut. Res., 2020, 27, 3637–3650 CrossRef CAS PubMed.
- K. Mehennaoui, S. Cambier, L. Minguez, T. Serchi, F. Guerold and A. C. Gutleb,
et al., Sub-chronic effects of AgNPs and AuNPs on Gammarus fossarum (crustacea amphipoda): From molecular to behavioural responses, Ecotoxicol. Environ. Saf., 2021, 210, 111775 CrossRef CAS PubMed.
- S. Bao, J. Huang, X. Liu, W. Tang and T. Fang, Tissue distribution of ag and oxidative stress responses in the freshwater snail Bellamya aeruginosa exposed to sediment-associated Ag nanoparticles, Sci. Total Environ., 2018, 644, 736–746 CrossRef CAS PubMed.
- J. Kalman, K. B. Paul, F. R. Khan, V. Stone and T. F. Fernandes, Characterisation of bioaccumulation dynamics of three differently coated silver nanoparticles and aqueous silver in a simple freshwater food chain, Environ. Chem., 2015, 12, 662–672 CrossRef CAS.
- L. Yang and W.-X. Wang, Comparative contributions of copper nanoparticles and ions to copper bioaccumulation and toxicity in barnacle larvae, Environ. Pollut., 2019, 249, 116–124 CrossRef CAS PubMed.
- T. Ma, S. Gong and B. Tian, Effects of sediment-associated cuo nanoparticles on cu bioaccumulation and oxidative stress responses in freshwater snail Bellamya aeruginosa, Sci. Total Environ., 2017, 580, 797–804 CrossRef CAS PubMed.
- L. Mao, M. Hu, B. Pan, Y. Xie and E. J. Petersen, Biodistribution and toxicity of radio-labeled few layer graphene in mice after intratracheal instillation, Part. Fibre Toxicol., 2015, 13, 1–12 CrossRef PubMed.
- L. Mao, C. Liu, K. Lu, Y. Su, C. Gu and Q. Huang,
et al., Exposure of few layer graphene to Limnodrilus hoffmeisteri modifies the graphene and changes its bioaccumulation by other organisms, Carbon, 2016, 109, 566–574 CrossRef CAS PubMed.
- T. Lammel, A. Thit, C. Mouneyrac, A. Baun, J. Sturve and H. Selck, Trophic transfer of Cuo NPs and dissolved Cu from sediment to worms to fish–a proof-of-concept study, Environ. Sci.: Nano, 2019, 6, 1140–1155 RSC.
- N. Mei, J. Hedberg, M. T. Ekvall, E. Kelpsiene, L.-A. Hansson and T. Cedervall,
et al., Transfer of cobalt nanoparticles in a simplified food web: From algae to zooplankton to fish, Applied Nano, 2021, 2, 184–205 CrossRef.
- X. Zhao, M. Yu, D. Xu, A. Liu, X. Hou and F. Hao,
et al., Distribution, bioaccumulation, trophic transfer, and influences of CeO2 nanoparticles in a constructed aquatic food web, Environ. Sci. Technol., 2017, 51, 5205–5214 CrossRef CAS PubMed.
- Q. Abbas, B. Yousaf, M. U. Ali, M. A. M. Munir, A. El-Naggar and J. Rinklebe,
et al., Transformation pathways and fate of engineered nanoparticles (ENPs) in distinct interactive environmental compartments: A review, Environ. Int., 2020, 138, 105646 CrossRef CAS PubMed.
- Z. Luo, Z. Li, Z. Xie, I. M. Sokolova, L. Song and W. J. Peijnenburg,
et al., Rethinking nano-TiO2 safety: Overview of toxic effects in humans and aquatic animals, Small, 2020, 16, 2002019 CrossRef CAS PubMed.
- Z. Li, M. Hu, H. Song, D. Lin and Y. Wang, Toxic effects of nano-TiO2 in bivalves—a synthesis of meta-analysis and bibliometric analysis, J. Environ. Sci., 2021, 104, 188–203 CrossRef CAS PubMed.
- A. Dabrunz, L. Duester, C. Prasse, F. Seitz, R. Rosenfeldt and C. Schilde,
et al., Biological surface coating and molting inhibition as mechanisms of TiO2 nanoparticle toxicity in Daphnia magna, PLoS One, 2011, 6, e20112 CrossRef CAS PubMed.
- C. Völker, I. Kämpken, C. Boedicker, J. Oehlmann and M. Oetken, Toxicity of silver nanoparticles and ionic silver: Comparison of adverse effects and potential toxicity mechanisms in the freshwater clam Sphaerium corneum, Nanotoxicology, 2015, 677–685 CrossRef.
- W. Zhang, B. Xiao and T. Fang, Chemical transformation of silver nanoparticles in aquatic environments: Mechanism, morphology and toxicity, Chemosphere, 2018, 191, 324–334 CrossRef CAS PubMed.
- X. Cao, L. Xu, Y. P. Chen, A. W. Decho, Z. Cui and J. R. Lead, Contribution, composition, and structure of eps by in vivo exposure to elucidate the mechanisms of nanoparticle-enhanced bioremediation to metals, Environ. Sci. Technol., 2022, 56, 896–906 CrossRef CAS PubMed.
- M. Leist, A. Ghallab, R. Graepel, R. Marchan, R. Hassan and S. H. Bennekou,
et al., Adverse outcome pathways: Opportunities, limitations and open questions, Arch. Toxicol., 2017, 91, 3477–3505 CrossRef CAS PubMed.
- D. L. Villeneuve, D. Crump, N. Garcia-Reyero, M. Hecker, T. H. Hutchinson and C. A. LaLone,
et al., Adverse outcome pathway (AOP) development I: Strategies and principles, Toxicol. Sci., 2014, 142, 312–320 CrossRef CAS PubMed.
- Z. Wang, L. Zhang, J. Zhao and B. Xing, Environmental processes and toxicity of metallic nanoparticles in aquatic systems as affected by natural organic matter, Environ. Sci.: Nano, 2016, 3, 240–255 RSC.
- W. J. Peijnenburg, M. Baalousha, J. Chen, Q. Chaudry, F. Von der Kammer and T. A. Kuhlbusch,
et al., A review of the properties and processes determining the fate of engineered nanomaterials in the aquatic environment, Crit. Rev. Environ. Sci. Technol., 2015, 45, 2084–2134 CrossRef CAS.
- W. Zhang, S. Ke, C. Sun, X. Xu, J. Chen and L. Yao, Fate and toxicity of silver nanoparticles in freshwater from laboratory to realistic environments: A review, Environ. Sci. Pollut. Res., 2019, 26, 7390–7404 CrossRef CAS PubMed.
- J. Zhao, Y. Li, X. Wang, X. Xia, E. Shang and J. Ali, Ionic-strength-dependent effect of suspended sediment on the aggregation, dissolution and settling of silver nanoparticles, Environ. Pollut., 2021, 279, 116926 CrossRef CAS PubMed.
- S. Bao, H. Wang, W. Zhang, Z. Xie and T. Fang, An investigation into the effects of silver nanoparticles on natural microbial communities in two freshwater sediments, Environ. Pollut., 2016, 219, 696–704 CrossRef CAS PubMed.
- P. Bélteky, A. Rónavári, D. Zakupszky, E. Boka, N. Igaz and B. Szerencsés,
et al., Are smaller nanoparticles always better? Understanding the biological effect of size-dependent silver nanoparticle aggregation under biorelevant conditions, Int. J. Nanomed., 2021, 16, 3021 CrossRef PubMed.
- M. Sikder, M.-N. Croteau, B. A. Poulin and M. Baalousha, Effect of nanoparticle size and natural organic matter composition on the bioavailability of polyvinylpyrrolidone-coated platinum nanoparticles to a model freshwater invertebrate, Environ. Sci. Technol., 2021, 55, 2452–2461 CrossRef CAS PubMed.
- V. Iswarya, J. Manivannan, A. De, S. Paul, R. Roy and J. Johnson,
et al., Surface capping and size-dependent toxicity of gold nanoparticles on different trophic levels, Environ. Sci. Pollut. Res., 2016, 23, 4844–4858 CrossRef CAS PubMed.
- A. F. Nanja, W. W. Focke and N. Musee, Aggregation and dissolution of aluminium oxide and copper oxide nanoparticles in natural aqueous matrixes, SN Appl. Sci., 2020, 2, 1–16 Search PubMed.
- J. Jiménez-Lamana and V. I. Slaveykova, Silver nanoparticle behaviour in lake water depends on their surface coating, Sci. Total Environ., 2016, 573, 946–953 CrossRef PubMed.
- M. C. Surette and J. A. Nason, Nanoparticle aggregation in a freshwater river: The role of engineered surface coatings, Environ. Sci.: Nano, 2019, 6, 540–553 RSC.
- M. C. Surette and J. A. Nason, Effects of surface coating character and interactions with natural organic matter on the colloidal stability of gold nanoparticles, Environ. Sci.: Nano, 2016, 3, 1144–1152 RSC.
- Y.-H. Peng, Y.-C. Tsai, C.-E. Hsiung, Y.-H. Lin and Y.-H. Shih, Influence of water chemistry on the environmental behaviors of commercial zno nanoparticles in various water and wastewater samples, J. Hazard. Mater., 2017, 322, 348–356 CrossRef CAS PubMed.
- F. Loosli, P. Le Coustumer and S. Stoll, Impact of alginate concentration on the stability of agglomerates made of TiO2 engineered nanoparticles: Water hardness and pH effects, J. Nanopart. Res., 2015, 17, 1–9 CrossRef CAS.
- J. R. Conway, A. S. Adeleye, J. Gardea-Torresdey and A. A. Keller, Aggregation, dissolution, and transformation of copper nanoparticles in natural waters, Environ. Sci. Technol., 2015, 49, 2749–2756 CrossRef CAS PubMed.
- Y. Yin, X. Yang, X. Zhou, W. Wang, S. Yu and J. Liu,
et al., Water chemistry controlled aggregation and photo-transformation of silver nanoparticles in environmental waters, J. Environ. Sci., 2015, 34, 116–125 CrossRef CAS PubMed.
- G. Metreveli, A. Philippe and G. E. Schaumann, Disaggregation of silver nanoparticle homoaggregates in a river water matrix, Sci. Total Environ., 2015, 535, 35–44 CrossRef CAS PubMed.
- E. Topuz, L. Sigg and I. Talinli, A systematic evaluation of agglomeration of Ag and TiO2 nanoparticles under freshwater relevant conditions, Environ. Pollut., 2014, 193, 37–44 CrossRef CAS PubMed.
- A. Philippe and G. E. Schaumann, Interactions of dissolved organic matter with natural and engineered inorganic colloids: A review, Environ. Sci. Technol., 2014, 48, 8946–8962 CrossRef CAS PubMed.
-
K. Rosales-Segovia, Y. Bai, C. Wang, L. Huang, W. Cheng and Y. Yamashita, et al., Building an effective concentration signature for Zn availability from 4 techniques to a stream, Program and Abstract, 2021, vol. 2, p. 21 Search PubMed.
- L. Gutierrez, A. Schmid, N. Zaouri, D. Garces and J. P. Croue, Colloidal stability of capped silver nanoparticles in natural organic matter-containing electrolyte solutions, NanoImpact, 2020, 19, 100242 CrossRef.
- O. Oriekhova and S. Stoll, Stability of uncoated and fulvic acids coated manufactured CeO2 nanoparticles in various conditions: From ultrapure to natural lake geneva waters, Sci. Total Environ., 2016, 562, 327–334 CrossRef CAS PubMed.
- D. L. Slomberg, P. Ollivier, H. Miche, B. Angeletti, A. Bruchet and M. Philibert,
et al., Nanoparticle stability in lake water shaped by natural organic matter properties and presence of particulate matter, Sci. Total Environ., 2019, 656, 338–346 CrossRef CAS PubMed.
- V. S. Sousa and M. R. Teixeira, Removal of a mixture of metal nanoparticles from natural surface waters using traditional coagulation process, J. Water Process. Eng., 2020, 36, 101285 CrossRef.
- S. Pradhan, J. Hedberg, J. Rosenqvist, C. M. Jonsson, S. Wold and E. Blomberg,
et al., Influence of humic acid and dihydroxy benzoic acid on the agglomeration, adsorption, sedimentation and dissolution of copper, manganese, aluminum and silica nanoparticles–a tentative exposure scenario, PLoS One, 2018, 13, e0192553 CrossRef PubMed.
- B. Lv, C. Wang, J. Hou, P. Wang, L. Miao and Y. Li,
et al., Influence of shear forces on the aggregation and sedimentation behavior of cerium dioxide (CeO2) nanoparticles under different hydrochemical conditions, J. Nanopart. Res., 2016, 18, 1–12 CrossRef CAS.
- J. Zhao, X. Wang, S. A. Hoang, N. S. Bolan, M. Kirkham and J. Liu,
et al., Silver nanoparticles in aquatic sediments: Occurrence, chemical transformations, toxicity, and analytical methods, J. Hazard. Mater., 2021, 418, 126368 CrossRef CAS PubMed.
- P. Borm, F. C. Klaessig, T. D. Landry, B. Moudgil, J. R. Pauluhn and K. Thomas,
et al., Research strategies for safety evaluation of nanomaterials, part v: Role of dissolution in biological fate and effects of nanoscale particles, Toxicol. Sci., 2006, 90, 23–32 CrossRef CAS PubMed.
- M. Bhuvaneshwari, V. Iswarya, R. Nagarajan, N. Chandrasekaran and A. Mukherjee, Acute toxicity and accumulation of ZnO NPs in ceriodaphnia dubia: Relative contributions of dissolved ions and particles, Aquat. Toxicol., 2016, 177, 494–502 CrossRef CAS PubMed.
- M.-L. Avramescu, P. Rasmussen, M. Chénier and H. Gardner, Influence of pH, particle size and crystal form on dissolution behaviour of engineered nanomaterials, Environ. Sci. Pollut. Res., 2017, 24, 1553–1564 CrossRef CAS PubMed.
- B. Molleman and T. Hiemstra, Time, pH, and size dependency of silver nanoparticle dissolution: The road to equilibrium, Environ. Sci.: Nano, 2017, 4, 1314–1327 RSC.
- J. Du, Y. Zhang, R. Guo, F. Meng, Y. Gao and C. Ma,
et al., Harmful effect of nanoparticles on the functions of freshwater ecosystems: Insight into nanozno-polluted stream, Chemosphere, 2019, 214, 830–838 CrossRef CAS PubMed.
- J. M. Zook, S. E. Long, D. Cleveland, C. L. A. Geronimo and R. I. MacCuspie, Measuring silver nanoparticle dissolution in complex biological and environmental matrices using uv–visible absorbance, Anal. Bioanal. Chem., 2011, 401, 1993–2002 CrossRef CAS PubMed.
- S. R. Tangaa, H. Selck, M. Winther-Nielsen and F. R. Khan, Trophic transfer of metal-based nanoparticles in aquatic environments: A review and recommendations for future research focus, Environ. Sci.: Nano, 2016, 3, 966–981 RSC.
- J. Hou, Y. Zhou, C. Wang, S. Li and X. Wang, Toxic effects and molecular mechanism of different types of silver nanoparticles to the aquatic crustacean Daphnia magna, Environ. Sci. Technol., 2017, 51, 12868–12878 CrossRef CAS PubMed.
- B. M. Angel, G. E. Batley, C. V. Jarolimek and N. J. Rogers, The impact of size on the fate and toxicity of nanoparticulate silver in aquatic systems, Chemosphere, 2013, 93, 359–365 CrossRef CAS PubMed.
- J. Dobias and R. Bernier-Latmani, Silver release from silver nanoparticles in natural waters, Environ. Sci. Technol., 2013, 47, 4140–4146 CrossRef CAS PubMed.
- Y. Chen, W. Liu, X. Leng and S. Stoll, Toxicity of selenium nanoparticles on poterioochromonas malhamensis algae in waris-h culture medium and lake geneva water: Effect of nanoparticle coating, dissolution, and aggregation, Sci. Total Environ., 2022, 808, 152010 CrossRef CAS PubMed.
- L.-F. Wang, N. Habibul, D.-Q. He, W.-W. Li, X. Zhang and H. Jiang,
et al., Copper release from copper nanoparticles in the presence of natural organic matter, Water Res., 2015, 68, 12–23 CrossRef CAS PubMed.
- M. Kraas, K. Schlich, B. Knopf, F. Wege, R. Kägi and K. Terytze,
et al., Long-term effects of sulfidized silver nanoparticles in sewage sludge on soil microflora, Environ. Toxicol. Chem., 2017, 36, 3305–3313 CrossRef CAS PubMed.
- K. A. Johnston, L. M. Stabryla, L. M. Gilbertson and J. E. Millstone, Emerging investigator series: Connecting concepts of coinage metal stability across length scales, Environ. Sci.: Nano, 2019, 6, 2674–2696 RSC.
- J. Liu, F. Zhang, A. J. Allen, A. C. Johnston-Peck and J. M. Pettibone, Comparing sulfidation kinetics of silver nanoparticles in simulated media using direct and indirect measurement methods, Nanoscale, 2018, 10, 22270–22279 RSC.
- R. D. Kent, J. G. Oser and P. J. Vikesland, Controlled evaluation of silver nanoparticle sulfidation in a full-scale wastewater treatment plant, Environ. Sci. Technol., 2014, 48, 8564–8572 CrossRef CAS PubMed.
- L. Li, Q. Zhou, F. Geng, Y. Wang and G. Jiang, Formation of nanosilver from silver sulfide nanoparticles in natural waters by photoinduced Fe (ii, iii) redox cycling, Environ. Sci. Technol., 2016, 50, 13342–13350 CrossRef CAS PubMed.
-
P. Prasher, M. Sharma, H. Mudila, A. Verma and P. Bhatt, Silver nanoparticles in natural ecosystems: Fate, transport, and toxicity, in Green synthesis of silver nanomaterials, Elsevier, 2022, pp. 649–668 Search PubMed.
- C. Cao, J. Huang, C.-N. Yan, X.-X. Zhang and Y.-X. Ma, Impacts of ag and Ag2S nanoparticles on the nitrogen removal within vertical flow constructed wetlands treating secondary effluent, Sci. Total Environ., 2021, 777, 145171 CrossRef CAS PubMed.
- M. Junaid and J. Wang, Interaction of nanoplastics with extracellular polymeric substances (EPS) in the aquatic environment: A special reference to eco-corona formation and associated impacts, Water Res., 2021, 201, 117319 CrossRef CAS PubMed.
- L. Natarajan, M. A. Jenifer and A. Mukherjee, Eco-corona formation on the nanomaterials in the aquatic systems lessens their toxic impact: A comprehensive review, Environ. Res., 2020, 194, 110669 CrossRef PubMed.
- C. C. Fleischer and C. K. Payne, Nanoparticle–cell interactions: Molecular structure of the protein corona and cellular outcomes, Acc. Chem. Res., 2014, 47, 2651–2659 CrossRef CAS PubMed.
- L. Canesi, T. Balbi, R. Fabbri, A. Salis, G. Damonte and M. Volland,
et al., Biomolecular coronas in invertebrate species: Implications in the environmental impact of nanoparticles, NanoImpact, 2017, 8, 89–98 CrossRef.
- L. Xu, M. Xu, R. Wang, Y. Yin, I. Lynch and S. Liu, The crucial role of environmental coronas in determining the biological effects of engineered nanomaterials, Small, 2020, 16, 2003691 CrossRef CAS PubMed.
- A. Kroll, R. Behra, R. Kaegi and L. Sigg, Extracellular polymeric substances (EPS) of freshwater biofilms stabilize and modify CeO2 and Ag nanoparticles, PLoS One, 2014, 9, e110709 CrossRef PubMed.
- H. Xu and H. Jiang, Effects of cyanobacterial extracellular polymeric substances on the stability of ZnO nanoparticles in eutrophic shallow lakes, Environ. Pollut., 2015, 197, 231–239 CrossRef CAS PubMed.
- G.-P. Sheng, J. Xu, W.-H. Li and H.-Q. Yu, Quantification of the interactions between Ca2+, Hg2+ and extracellular polymeric substances (EPS) of sludge, Chemosphere, 2013, 93, 1436–1441 CrossRef CAS PubMed.
- X. Zhang, C.-W. Yang, H.-Q. Yu and G.-P. Sheng, Light-induced reduction of silver ions to silver nanoparticles in aquatic environments by microbial extracellular polymeric substances (EPS), Water Res., 2016, 106, 242–248 CrossRef CAS PubMed.
- K. Mattsson, R. Aguilar, O. Torstensson, D. Perry, K. Bernfur and S. Linse,
et al., Disaggregation of gold nanoparticles by daphnia magna, Nanotoxicology, 2018, 12, 885–900 CrossRef CAS PubMed.
- F. Nasser and I. Lynch, Secreted protein eco-corona mediates uptake and impacts of polystyrene nanoparticles on Daphnia magna, J. Proteomics, 2016, 137, 45–51 CrossRef CAS PubMed.
- G. Pulido-Reyes, F. Leganes, F. Fernández-Piñas and R. Rosal, Bio-nano interface and environment: A critical review, Environ. Toxicol. Chem., 2017, 36, 3181–3193 CrossRef CAS PubMed.
- L. Zeng, J. Gao, Y. Liu, J. Gao, L. Yao and X. Yang,
et al., Role of protein corona in the biological effect of nanomaterials: Investigating methods, TrAC, Trends Anal. Chem., 2019, 118, 303–314 CrossRef CAS.
- K. L. Garner, Y. Qin, S. Cucurachi, S. Suh and A. A. Keller, Linking exposure and kinetic bioaccumulation models for metallic engineered nanomaterials in freshwater ecosystems, ACS Sustainable Chem. Eng., 2018, 6, 12684–12694 CrossRef CAS.
- S. Kuehr, B. Meisterjahn, N. Schröder, B. Knopf, D. Völker and K. Schwirn,
et al., Testing the bioaccumulation of manufactured nanomaterials in the freshwater bivalve Corbicula fluminea using a new test method, Environ. Sci.: Nano, 2020, 7, 535–553 RSC.
- D. Danabas, M. Ates, B. E. Tastan, I. C. C. Cimen, I. Unal and O. Aksu,
et al., Effects of Zn and Zno nanoparticles on Artemia salina and Daphnia magna organisms: Toxicity, accumulation and elimination, Sci. Total Environ., 2020, 711, 134869 CrossRef CAS PubMed.
- M. Li, Z. Luo, Y. Yan, Z. Wang, Q. Chi and C. Yan,
et al., Arsenate accumulation, distribution, and toxicity associated with titanium dioxide nanoparticles in Daphnia magna, Environ. Sci. Technol., 2016, 50, 9636 CrossRef CAS PubMed.
- C. W. Huang, C. H. Chang, S. W. Li, P. L. Yen and H. C. Liao, Co-exposure to foodborne and waterborne zno nanoparticles in aquatic sediment environments enhances DNA damage and stress gene expression in freshwater asian clam Corbicula fluminea, Environ. Sci.: Nano, 2020, 7, 1252–1265 RSC.
- E. C. Oliveira-Filho, D. H. D. F. Muniz, E. L. D. Carvalho, P. Vélez and C. K. Grisolia, Effects of agnps on the snail Biomphalaria glabrata: Survival, reproduction and silver accumulation, Toxics, 2019, 7, 12 CrossRef CAS PubMed.
- M. N. Moore, J. Readman, J. W. Readman, D. M. Lowe, P. E. Frickers and A. Beesley, Lysosomal cytotoxicity of carbon nanoparticles in cells of the molluscan immune system: An in vitro study, Nanotoxicology, 2009, 3, 40–45 CrossRef CAS.
- D. Cleveland, S. E. Long, P. L. Pennington, E. Cooper, M. H. Fulton and G. I. Scott,
et al., Pilot estuarine mesocosm study on the environmental fate of silver nanomaterials leached from consumer products, Sci. Total Environ., 2012, 421, 267–272 CrossRef PubMed.
- S. Kuehr, V. Kosfeld and C. Schlechtriem, Bioaccumulation assessment of nanomaterials using freshwater invertebrate species, Environ. Sci. Eur., 2021, 33, 1–36 CrossRef.
- M. Rosa, J. E. Ward and S. E. Shumway, Selective capture and ingestion of particles by suspension-feeding bivalve molluscs: A review, J. Shellfish Res., 2018, 37, 727–746 CrossRef.
- F. Moëzzi, S. A. Hedayati and A. Ghadermarzi, Copper bioaccumulation kinetics in swan mussel, Anodonta cygnea (linnaeus, 1758) during waterborne exposure to CuO nanoparticles, Bull. Environ. Contam. Toxicol., 2019, 102, 46–51 CrossRef PubMed.
- M. Garau, M. Auffan, S. Devin, V. Felten and L. Giamberini, Integrated assessment of ceria nanoparticle impacts on the freshwater bivalve dreissena polymorpha, Nanotoxicology, 2016, 10, 935–944 CrossRef PubMed.
- A. Bourgeault, C. Cousin, V. Geertsen, C. Cassier-Chauvat, F. Chauvat and O. Durupthy,
et al., The challenge of studying TiO2 nanoparticle bioaccumulation at environmental concentrations: Crucial use of a stable isotope tracer, Environ. Sci. Technol., 2015, 49, 2451–2459 CrossRef CAS PubMed.
- L. Canesi, R. Fabbri, G. Gallo, D. Vallotto, A. Marcomini and G. Pojana, Biomarkers in Mytilus galloprovincialis exposed to suspensions of selected nanoparticles (nano carbon black, C60 fullerene, nano-TiO2, nano-SiO2), Aquat. Toxicol., 2010, 100, 168–177 CrossRef CAS PubMed.
- F. Moëzzi, S. A. Hedayati and A. Ghadermazi, Ecotoxicological impacts of exposure to copper oxide nanoparticles on the gill of the swan mussel, Anodonta cygnea (linnaeus, 1758), Molluscan Res., 2018, 38, 187–197 CrossRef.
-
J. H. Thorp and A. P. Covich, Ecology and classification of north american freshwater invertebrates, Academic press, 2009, pp. 208–209 Search PubMed.
- A. N. Samsi, R. Asaf, A. Santi and M. I. Wamnebo, Gastropods as a bioindicator and biomonitoring metal pollution, Aquac. Indones., 2017, 18, 1–8 Search PubMed.
- T. Ramskov, H. Selck, G. Banta, S. K. Misra, D. Berhanu and E. Valsami-Jones,
et al., Bioaccumulation and effects of different-shaped copper oxide nanoparticles in the deposit-feeding snail Potamopyrgus antipodarum, Environ. Toxicol. Chem., 2015, 33, 1976–1987 CrossRef PubMed.
- F. R. Khan, S. K. Misra, J. Garcia-Alonso, B. D. Smith, S. Strekopytov and P. S. Rainbow,
et al., Bioaccumulation dynamics and modeling in an estuarine invertebrate following aqueous exposure to nanosized and dissolved silver, Environ. Sci. Technol., 2012, 46, 7621 CrossRef CAS PubMed.
- F. R. Khan, S. K. Misra, N. R. Bury, B. D. Smith, P. S. Rainbow and S. N. Luoma,
et al., Inhibition of potential uptake pathways for silver nanoparticles in the estuarine snail Peringia ulvae, Nanotoxicology, 2015, 9, 493–501 CrossRef CAS PubMed.
- V. Pareek, A. Bhargava, V. Bhanot, R. Gupta and J. Panwar, Formation and characterization of protein corona around nanoparticles: A review, J. Nanosci. Nanotechnol., 2018, 18, 6653–6670 CrossRef CAS PubMed.
- C. Pang, H. Selck, S. K. Misra, D. Berhanu, A. Dybowska and E. Valsami-Jones,
et al., Effects of sediment-associated copper to the deposit-feeding snail, Potamopyrgus antipodarum: A comparison of Cu added in aqueous form or as nano- and micro-CuO particles, Aquat. Toxicol., 2012, 106–107, 114–122 CrossRef CAS PubMed.
- M. Auffan, W. Liu, L. Brousset, L. Scifo, A. Pariat and M. Sanles,
et al., Environmental exposure of a simulated pond ecosystem to a CuO nanoparticle-based wood stain throughout its life cycle, Environ. Sci.: Nano, 2018, 5, 2579–2589 RSC.
- Y. Chen, F. Wu, W. Li, T. Luan and L. Lin, Comparison on the effects of water-borne and dietary-borne accumulated ZnO nanoparticles on Daphnia magna, Chemosphere, 2017, 189, 94–103 CrossRef CAS PubMed.
- M. N. Croteau, S. K. Misra, S. N. Luoma and E. V. Jones, Bioaccumulation and toxicity of CuO nanoparticles by a freshwater invertebrate after waterborne and dietborne exposures, Environ. Sci. Technol., 2014, 48, 10929–10937 CrossRef CAS PubMed.
- W. Fan, A. Bortvedt, B. J. Harper, L. E. Crandon and S. L. Harper, Uptake and toxicity of cuo nanoparticles to Daphnia magna varies between indirect dietary and direct waterborne exposures, Aquat. Toxicol., 2017, 190, 78–86 CrossRef PubMed.
- F. Ribeiro, C. A. Van Gestel, M. D. Pavlaki, S. Azevedo, A. M. Soares and S. Loureiro, Bioaccumulation of silver in Daphnia magna: Waterborne and dietary exposure to nanoparticles and dissolved silver, Sci. Total Environ., 2017, 574, 1633–1639 CrossRef CAS PubMed.
- M.-N. Croteau, S. K. Misra, S. N. Luoma and E. Valsami-Jones, Silver bioaccumulation dynamics in a freshwater invertebrate after aqueous and dietary exposures to nanosized and ionic Ag, Environ. Sci. Technol., 2011, 45, 6600–6607 CrossRef CAS PubMed.
- P. V. Silva, C. Pinheiro, R. G. Morgado, R. A. Verweij, C. A. van Gestel and S. Loureiro, Bioaccumulation but no biomagnification of silver sulfide nanoparticles in freshwater snails and planarians, Sci. Total Environ., 2022, 808, 151956 CrossRef CAS PubMed.
- S. Majumdar, J. Trujillo-Reyes, J. A. Hernandez-Viezcas, J. C. White, J. R. Peralta-Videa and J. L. Gardea-Torresdey, Cerium biomagnification in a terrestrial food chain: Influence of particle size and growth stage, Environ. Sci. Technol., 2016, 50, 6782–6792 CrossRef CAS PubMed.
- F. Dang, Y. Huang, Y. Wang, D. Zhou and B. Xing, Transfer and toxicity of silver nanoparticles in the food chain, Environ. Sci.: Nano, 2021, 8(6), 1519–1535 RSC.
- S. Kuehr, V. Kosfeld and C. Schlechtriem, Bioaccumulation assessment of nanomaterials using freshwater invertebrate species, Environ. Sci. Eur., 2021, 33, 1–36 CrossRef.
- X. Chen, Y. Zhu, K. Yang, L. Zhu and D. Lin, Nanoparticle TiO2 size and rutile content impact bioconcentration and biomagnification from algae to daphnia, Environ. Pollut., 2019, 247, 421–430 CrossRef CAS PubMed.
- J. Chen, H. Li, X. Han and X. Wei, Transmission and accumulation of nano-TiO2 in a 2-step food chain (Scenedesmus obliquus to Daphnia magna), Bull. Environ. Contam. Toxicol., 2015, 95, 145–149 CrossRef CAS PubMed.
- V. Iswarya, M. Bhuvaneshwari and N. Chandrasekaran,
et al., Trophic transfer potential of two different crystalline phases of TiO2 NPs from Chlorella sp. to Ceriodaphnia dubia, Aquat. Toxicol., 2018, 197, 89–97 CrossRef CAS PubMed.
- W. Fan, L. Liu, R. Peng and W. X. Wang, High bioconcentration of titanium dioxide nanoparticles in Daphnia magna determined by kinetic approach, Sci. Total Environ., 2016, 1224–1231 CrossRef CAS PubMed.
- J. McTeer, A. P. Dean, K. N. White and J. K. Pittman, Bioaccumulation of silver nanoparticles into Daphnia magna from a freshwater algal diet and the impact of phosphate availability, Nanotoxicology, 2014, 8, 305–316 CrossRef CAS PubMed.
- S. Lekamge, A. F. Miranda, B. Pham, A. S. Ball, R. Shukla and D. Nugegoda, The toxicity of non-aged and aged coated silver nanoparticles to the freshwater shrimp Paratya australiensis, J. Toxicol. Environ. Health, Part A, 2019, 82, 1207–1222 CrossRef CAS PubMed.
- C. M. Zhao and W. X. Wang, Comparison of acute and chronic toxicity of silver nanoparticles and silver nitrate to Daphnia magna, Environ. Toxicol. Chem., 2011, 30, 885–892 CrossRef CAS PubMed.
- W.-M. Lee, S.-J. Yoon, Y.-J. Shin and Y.-J. An, Trophic transfer of gold nanoparticles from Euglena gracilis or Chlamydomonas reinhardtii to Daphnia magna, Environ. Pollut., 2015, 201, 10–16 CrossRef CAS PubMed.
- J. Hedberg, E. Blomberg and I. Odnevall Wallinder, In the search for nanospecific effects of dissolution of metallic nanoparticles at freshwater-like conditions: A critical review, Environ. Sci. Technol., 2019, 53, 4030–4044 CrossRef CAS PubMed.
- G. Atli, E. Canli and A. Eroglu,
et al., Characterization of antioxidant system parameters in four freshwater fish species, Ecotoxicol. Environ. Saf., 2016, 126, 30–37 CrossRef CAS PubMed.
- L. Canesi, C. Ciacci, D. Vallotto, G. Gallo, A. Marcomini and G. Pojana, In vitro effects of suspensions of selected nanoparticles (C60 fullerene, TiO2, SiO2) on Mytilus hemocytes, Aquat. Toxicol., 2010, 96, 151–158 CrossRef CAS PubMed.
- T. Gomes, O. Araújo, R. Pereira, A. C. Almeida, A. Cravo and M. J. Bebianno, Genotoxicity of copper oxide and silver nanoparticles in the mussel Mytilus galloprovincialis, Mar. Environ. Res., 2013, 84, 51–59 CrossRef CAS PubMed.
- A. S. Mohamed, S. B. Dajem, M. Al-Kahtani, S. B. Ali and S. R. Fahmy, Silver/chitosan nanocomposites induce physiological and histological changes in freshwater bivalve, J. Trace Elem. Med. Biol., 2021, 65, 126719 CrossRef CAS PubMed.
- E. G. Canli and M. Canli, Characterization of atpases in the gill of freshwater mussel (Unio tigridis) and effects of ionic and nanoparticle forms of aluminium and copper, Comp. Biochem. Physiol., Part C: Toxicol. Pharmacol., 2021, 247, 109059 CAS.
- C. D. Torre, D. Maggioni, L. Nigro, F. Farè and A. Binelli, Alginate coating modifies the biological effects of cerium oxide nanoparticles to the freshwater bivalve Dreissena polymorpha, Sci. Total Environ., 2021, 773, 145612 CrossRef PubMed.
- A. Ray, A. Gautam, S. Das, K. Pal, S. Das and P. Karmakar,
et al., Effects of copper oxide nanoparticle on gill filtration rate, respiration rate, hemocyte associated immune parameters and oxidative status of an indian freshwater mussel, Comp. Biochem. Physiol., Part C: Toxicol. Pharmacol., 2020, 237, 108855 CAS.
- A. S. Mohamed, S. B. Dajem, M. Al-Kahtani, S. B. Ali and S. R. Fahmy, Freshwater clam as a potential bioindicator for silver/saponin nanocomposites toxicity, Bull. Environ. Contam. Toxicol., 2020, 105, 1–8 CrossRef PubMed.
- J. Auclair, P. Turcotte, C. Gagnon, C. Peyrot and F. Gagne, Comparative toxicity of copper oxide nanoparticles and dissolved copper to freshwater mussels, International Journal of Zoological Investigations, 2020, 6, 135–147 Search PubMed.
- F. Gagné, J. Auclair, M. Fortier, A. Bruneau and C. Gagnon, Bioavailability and immunotoxicity of silver nanoparticles to the freshwater mussel Elliptio complanata, J. Toxicol. Environ. Health, Part A, 2013, 76, 767–777 CrossRef PubMed.
- F. Gagné, J. Auclair, P. Turcotte and C. Gagnon, Sublethal effects of silver nanoparticles and dissolved silver in freshwater mussels, J. Toxicol. Environ. Health, 2013, 76, 479–490 CrossRef PubMed.
- V. Koehle-Divo, C. Cossu-Leguille, S. Pain-Devin, C. Simonin and L. Giamberini, Genotoxicity and physiological effects of CeO2 NPs on a freshwater bivalve (Corbicula fluminea), Aquat. Toxicol., 2018, 198, 141–148 CrossRef CAS PubMed.
- T. H. Kim, M. Kim, H. S. Park, U. S. Shin, M. S. Gong and H. W. Kim, Size-dependent cellular toxicity of silver nanoparticles, J. Biomed. Mater. Res., Part A, 2012, 100, 1033–1043 CrossRef PubMed.
- Y. Cong, G. T. Banta, H. Selck, D. Berhanu, E. Valsami-Jones and V. E. Forbes, Toxicity and bioaccumulation of sediment-associated silver nanoparticles in the estuarine polychaete, Nereis (Hediste) diversicolor, Aquat. Toxicol., 2014, 156, 106–115 CrossRef CAS PubMed.
- T. L. Rocha, T. Gomes, V. S. Sousa, N. C. Mestre and M. J. Bebianno, Ecotoxicological impact of engineered nanomaterials in bivalve molluscs: An overview, Mar. Environ. Res., 2015, 111, 74–88 CrossRef CAS PubMed.
- F. Girardello, C. C. Leite, C. S. Branco, M. Roesch-Ely, A. N. Fernandes and M. Salvador,
et al., Antioxidant defences and haemocyte internalization in Limnoperna fortunei exposed to TiO2 nanoparticles, Aquat. Toxicol., 2016, 176, 190–196 CrossRef CAS PubMed.
- J. Auclair, C. Peyrot, K. J. Wilkinson and F. Gagné, The geometry of the toxicity of silver nanoparticles to freshwater mussels, Comp. Biochem. Physiol., Part C: Toxicol. Pharmacol., 2020, 239, 108841 Search PubMed.
- F. Girardello, C. C. Leite, I. V. Villela, M. Machado and J. A. P. Henriques, Titanium dioxide nanoparticles induce genotoxicity but not mutagenicity in golden mussel Limnoperna fortunei, Aquat. Toxicol., 2016, 170, 223–228 CrossRef CAS PubMed.
- W. Liu, Z. Zeng, A. Chen, G. Zeng, R. Xiao and G. Zhi,
et al., Toxicity effects of silver nanoparticles on the freshwater bivalve Corbicula fluminea, J. Environ. Chem. Eng., 2018, 6, 4236–4244 CrossRef CAS.
- I. M. Sokolova, M. Frederich, R. Bagwe, G. Lannig and A. A. Sukhotin, Energy homeostasis as an integrative tool for assessing limits of environmental stress tolerance in aquatic invertebrates, Mar. Environ. Res., 2012, 79, 1–15 CrossRef CAS PubMed.
- H. I. Falfushynska, L. L. Gnatyshyna, A. V. Ivanina, V. V. Khoma, O. B. Stoliar and I. M. Sokolova, Bioenergetic responses of freshwater mussels Unio tumidus to the combined effects of nano-ZnO and temperature regime, Sci. Total Environ., 2018, 650, 1440–1450 CrossRef PubMed.
- I. Marisa, V. Matozzo, M. Munari, A. Binelli, M. Parolini and A. Martucci,
et al., In vivo exposure of the marine clam Ruditapes philippinarum to Zinc oxide nanoparticles: Responses in gills, digestive gland and haemolymph, Environ. Sci. Pollut. Res., 2016, 23(15), 15275–15293 CrossRef CAS PubMed.
- N. K. Geitner, J. L. Cooper, A. Avellan, B. T. Castellon, B. G. Perrotta and N. Bossa,
et al., Size-based differential transport, uptake, and mass distribution of ceria (CeO2) nanoparticles in wetland mesocosms, Environ. Sci. Technol., 2018, 52, 9768–9776 CrossRef CAS PubMed.
- D. Ali, Oxidative stress-mediated apoptosis and genotoxicity induced by silver nanoparticles in freshwater snail Lymnea luteola, Biol. Trace Elem. Res., 2014, 162, 333–341 CrossRef CAS PubMed.
- D. Ali, S. Alarifi, S. Kumar, M. Ahamed and M. A. Siddiqui, Oxidative stress and genotoxic effect of zinc oxide nanoparticles in freshwater snail Lymnaea luteola, Aquat. Toxicol., 2012, 124, 83–90 CrossRef PubMed.
- T. Ma, W. Meng, S. Gong and B. Tian, Impacts of sediment organic matter content and ph on ecotoxicity of coexposure of TiO2 nanoparticles and cadmium to freshwater snails Bellamya aeruginosa, Arch. Environ. Contam. Toxicol., 2016, 72, 1–13 Search PubMed.
- D. Ali, Evaluation of environmental stress by comet assay on freshwater snail Lymnea luteola exposed to titanium dioxide nanoparticles, Toxicol. Environ. Chem., 2014, 96, 1185–1194 CrossRef CAS.
- S. R. Fahmy, F. Abdel-Ghaffar, F. A. Bakry and D. A. Sayed, Ecotoxicological effect of sublethal exposure to zinc oxide nanoparticles on freshwater snail Biomphalaria alexandrina, Arch. Environ. Contam. Toxicol., 2014, 67, 192–202 CrossRef CAS PubMed.
- H. G. Park, J. I. Kim, K. H. Chang, B. C. Lee, I. C. Eom and P. Kim,
et al., Trophic transfer of citrate, PVP coated silver nanomaterials, and silver ions in a paddy microcosm, Environ. Pollut., 2018, 235, 435–445 CrossRef CAS PubMed.
- A. Young, K. Lukowiak and A. Protheroe, Silver nanoparticles alter learning and memory formation in an aquatic organism, Lymnaea stagnalis, Environ. Pollut., 2017, 225, 403–411 CrossRef CAS PubMed.
- D. Ali and H. Ali, Susceptibility of the freshwater pulmonate snail Lymnea luteola l. to copper oxide nanoparticle, Toxicol. Environ. Chem. Rev., 2015, 97, 576–587 CrossRef CAS.
- M.-N. Croteau, A. D. Dybowska, S. N. Luoma, S. K. Misra and E. Valsami-Jones, Isotopically modified silver nanoparticles to assess nanosilver bioavailability and toxicity at environmentally relevant exposures, Environ. Chem., 2014, 11, 247–256 CrossRef CAS.
- P. V. Silva, C. A. Van Gestel, R. A. Verweij, A. G. Papadiamantis, S. F. Gonçalves and I. Lynch,
et al., Toxicokinetics of pristine and aged silver nanoparticles in Physa acuta, Environ. Sci.: Nano, 2020, 7, 3849–3868 RSC.
- E. C. Oliveira-Filho, D. F. Muniz and E. L. de Carvalho,
et al., Effects of AgNPs on the snail Biomphalaria glabrata: Survival, reproduction and silver Accumulation, Toxics, 2019, 7, 12 CrossRef CAS PubMed.
- R. J. Bernot and M. Brandenburg, Freshwater snail vital rates affected by non-lethal concentrations of silver nanoparticles, Hydrobiologia, 2013, 714, 25–34 CrossRef CAS.
- E. C. Oliveira-Filho, J. S. Filho, L. A. Novais, W. S. Peternele, R. B. Azevedo and C. K. Grisolia, Effects of γ-Fe2O3 nanoparticles on the survival and reproduction of Biomphalaria glabrata (Say, 1818) and their elimination from this benthic aquatic snail, Crit. Rev. Environ. Sci. Technol., 2016, 23, 18362–18368 CAS.
- L. Ulm, A. Krivohlavek, D. Jurašin, M. Ljubojević, G. Šinko and T. Crnković,
et al., Response of biochemical biomarkers in the aquatic crustacean Daphnia magna exposed to silver nanoparticles, Crit. Rev. Environ. Sci. Technol., 2015, 22, 19990–19999 CAS.
- H. P. Borase, S. V. Patil and R. S. Singhal,
Moina macrocopa as a non-target aquatic organism for assessment of ecotoxicity of silver nanoparticles: Effect of size, Chemosphere, 2019, 219, 713–723 CrossRef CAS PubMed.
- K. Mehennaoui, A. Georgantzopoulou, V. Felten, J. Andreï, M. Garaud and S. Cambier,
et al., Gammarus fossarum (crustacea, amphipoda) as a model organism to study the effects of silver nanoparticles, Sci. Total Environ., 2016, 566, 1649–1659 CrossRef PubMed.
- Z. Clemente, V. Castro, C. Jonsson and L. Fraceto, Minimal levels of ultraviolet light enhance the toxicity of TiO2 nanoparticles to two representative organisms of aquatic systems, J. Nanopart. Res., 2014, 16, 1–16 CrossRef CAS.
- S. Villa, D. Maggioni, H. Hamza, V. Di Nica, S. Magni and B. Morosetti,
et al., Natural molecule coatings modify the fate of cerium dioxide nanoparticles in water and their ecotoxicity to Daphnia magna, Environ. Pollut., 2020, 257, 113597 CrossRef CAS PubMed.
- T.-L. Pham, Toxicity of silver nanoparticles to tropical microalgae scenedesmus acuminatus, chaetoceros gracilis and crustacean Daphnia lumholtzi, Turkish J. Fish. Aquat. Sci., 2019, 19, 1009–1016 Search PubMed.
- D. J. Nogueira, V. P. Vaz, O. S. Neto, M. L. N. da Silva, C. Simioni and L. C. Ouriques,
et al., Crystalline phase-dependent toxicity of aluminum oxide nanoparticles toward Daphnia magna and ecological risk assessment, Environ. Res., 2020, 182, 108987 CrossRef CAS PubMed.
- A. Bownik, Daphnia swimming behaviour as a biomarker in toxicity assessment: A review, Sci. Total Environ., 2017, 601, 194–205 CrossRef PubMed.
- S. Vijayakumar, B. Malaikozhundan, P. Ramasamy and B. Vaseeharan, Assessment of biopolymer stabilized silver nanoparticle for their ecotoxicity on Ceriodaphnia cornuta and antibiofilm activity, J. Environ. Chem. Eng., 2016, 4, 2076–2083 CrossRef CAS.
- J. M. Farner, R. S. Cheong, E. Mahé, H. Anand and N. Tufenkji, Comparing TiO2 nanoparticle formulations: Stability and photoreactivity are key factors in acute toxicity to daphnia magna, Environ. Sci.: Nano, 2019, 6, 2532–2543 RSC.
- Y. Hu, X. Chen, K. Yang and D. Lin, Distinct toxicity of silver nanoparticles and silver nitrate to Daphnia magna in M4 medium and surface water, Sci. Total Environ., 2018, 618, 838–846 CrossRef CAS PubMed.
- M. P. Mousavi, I. L. Gunsolus, C. E. P. De Jesús, M. Lancaster, K. Hussein and C. L. Haynes,
et al., Dynamic silver speciation as studied with fluorous-phase ion-selective electrodes: Effect of natural organic matter on the toxicity and speciation of silver, Sci. Total Environ., 2015, 537, 453–461 CrossRef CAS PubMed.
- B. Dong, G. Liu, J. Zhou, J. Wang and R. Jin, Transformation of silver ions to silver nanoparticles mediated by humic acid under dark conditions at ambient temperature, J. Hazard. Mater., 2020, 383, 121190 CrossRef CAS PubMed.
- L.-J. A. Ellis, E. Valsami-Jones and I. Lynch, Exposure medium and particle ageing moderate the toxicological effects of nanomaterials to Daphnia magna over multiple generations: A case for standard test review?, Environ. Sci.: Nano, 2020, 7, 1136–1149 RSC.
- J. Zhao, M. Lin, Z. Wang, X. Cao and B. Xing, Engineered nanomaterials in the environment: Are they safe?, Crit. Rev. Environ. Sci. Technol., 2021, 51, 1443–1478 CrossRef.
- K. L. Garner, S. Suh and A. A. Keller, Assessing the risk of engineered nanomaterials in the environment: Development and application of the nanofate model, Environ. Sci. Technol., 2017, 51, 5541–5551 CrossRef CAS PubMed.
- B. Giese, F. Klaessig, B. Park, R. Kaegi, M. Steinfeldt and H. Wigger,
et al., Risks, release and concentrations of engineered nanomaterial in the environment, Sci. Rep., 2018, 8, 1–18 CAS.
- M. Hadioui, G. V. Knapp, A. Azimzada, I. Jreije, L. Frechette-Viens and K. J. Wilkinson, Lowering the size detection limits of ag and TiO2 nanoparticles by single particle ICP-MS, Anal. Chem., 2019, 91, 13275–13284 CrossRef CAS PubMed.
-
M. Kovochich, T. Xia, J. Xu, J. I. Yeh and A. E. Nel, Principles and procedures to assess nanomaterial toxicity, Environmental nanotechnology: applications and impacts of nanomaterials, McGraw Hill, New York, 2007, pp. 205–229 Search PubMed.
- J. Cohen, A power primer, Psychol. Bull., 1992, 112, 155 CAS.
- J. P. Higgins, S. G. Thompson, J. J. Deeks and D. G. Altman, Measuring inconsistency in meta-analyses, BMJ, 2003, 327, 557–560 CrossRef PubMed.
- A. Kopp-Schneider, P. Prieto, A. Kinsner-Ovaskainen and S. Stanzel, Design of a testing strategy using non-animal based test methods: Lessons learnt from the acutetox project, Toxicol. In Vitro, 2013, 27, 1395–1401 CrossRef CAS PubMed.
- Y. Qu, X. Liang, X. Kong and W. Zhang, Size-dependent cohesive energy, melting temperature, and debye temperature of spherical metallic nanoparticles, Phys. Met. Metallogr., 2017, 118, 528–534 CrossRef CAS.
- A. E. Porter, M. Gass, K. Muller, J. N. Skepper, P. A. Midgley and M. Welland, Direct imaging of single-walled carbon nanotubes in cells, Nat. Nanotechnol., 2007, 2, 713–717 CrossRef CAS PubMed.
- M. Moore, Do nanoparticles present ecotoxicological risks for the health of the aquatic environment?, Environ. Int., 2006, 32, 967–976 CrossRef CAS PubMed.
- O. Choi and Z. Hu, Size dependent and reactive oxygen species related nanosilver toxicity to nitrifying bacteria, Environ. Sci. Technol., 2008, 42, 4583–4588 CrossRef CAS PubMed.
- J. Kim, S. Lee, C.-M. Kim, J. Seo, Y. Park and D. Kwon,
et al., Non-monotonic concentration–response relationship of TiO2 nanoparticles in freshwater cladocerans under environmentally relevant uv-a light, Ecotoxicol. Environ. Saf., 2014, 101, 240–247 CrossRef CAS PubMed.
- G. T. Ankley, R. S. Bennett, R. J. Erickson, D. J. Hoff, M. W. Hornung and R. D. Johnson,
et al., Adverse outcome pathways: A conceptual framework to support ecotoxicology research and risk assessment, Environ. Toxicol. Chem., 2010, 29, 730–741 CrossRef CAS PubMed.
- M. Hecker and C. A. LaLone, Adverse outcome pathways: Moving from a scientific concept to an internationally accepted framework, Environ. Toxicol. Chem., 2019, 38, 1152–1163 CrossRef CAS PubMed.
- S. Halappanavar, S. Van Den Brule, P. Nymark, L. Gaté, C. Seidel and S. Valentino,
et al., Adverse outcome pathways as a tool for the design of testing strategies to support the safety assessment of emerging advanced materials at the nanoscale, Part. Fibre Toxicol., 2020, 17, 1–24 CrossRef PubMed.
- E. J. Perkins, G. T. Ankley, K. M. Crofton, N. Garcia-Reyero, C. A. LaLone and M. S. Johnson,
et al., Current perspectives on the use of alternative species in human health and ecological hazard assessments, Environ. Health Perspect., 2013, 121, 1002–1010 CrossRef PubMed.
- N. Garcia-Reyero, B. L. Escalon, E. Prats, J. K. Stanley, B. Thienpont and N. L. Melby,
et al., Effects of BDE-209 contaminated sediments on zebrafish development and potential implications to human health, Environ. Int., 2014, 63, 216–223 CrossRef CAS PubMed.
- N. L. Garcia-Reyero, A. J. Kennedy, B. L. Escalon, T. Habib, J. G. Laird and A. Rawat,
et al., Differential effects and potential adverse outcomes of ionic silver and silver nanoparticles in vivo and in vitro, Environ. Sci. Technol., 2014, 48, 4546–4555 CrossRef CAS PubMed.
- Y.-B. Ma, C.-J. Lu, M. Junaid, P.-P. Jia, L. Yang and J.-H. Zhang,
et al., Potential adverse outcome pathway (AOP) of silver nanoparticles mediated reproductive toxicity in zebrafish, Chemosphere, 2018, 207, 320–328 CrossRef CAS PubMed.
-
G. Hodges, S. Gutsell, N. Taylor, E. Brockmeier, E. Butler and C. Rendal, et al., in A systems biology approach to advancing adverse outcome pathways for risk assessment, Springer, 2018, pp. 75–106 Search PubMed.
- Y. Song, L. Xie, Y. Lee, D. A. Brede, F. Lyne and Y. Kassaye,
et al., Integrative assessment of low-dose gamma radiation effects on Daphnia magna reproduction: Toxicity pathway assembly and AOP development, Sci. Total Environ., 2020, 705, 135912 CrossRef CAS PubMed.
- R. C. Bicho, A. Faustino, A. Rêma, J. J. Scott-Fordsmand and M. J. Amorim, Confirmatory assays for transient changes of omics in soil invertebrates–copper materials in a multigenerational exposure, J. Hazard. Mater., 2021, 402, 123500 CrossRef CAS PubMed.
- J. W. Lee, E.-J. Won, S. Raisuddin and J.-S. Lee, Significance of adverse outcome pathways in biomarker-based environmental risk assessment in aquatic organisms, J. Environ. Sci., 2015, 35, 115–127 CrossRef CAS PubMed.
- J. Amorim, I. Abreu, P. Rodrigues, D. Peixoto, C. Pinheiro and A. Saraiva,
et al., Lymnaea stagnalis as a freshwater model invertebrate for ecotoxicological studies, Sci. Total Environ., 2019, 669, 11–28 CrossRef CAS PubMed.
- E. K. Brockmeier, G. Hodges, T. H. Hutchinson, E. Butler, M. Hecker and K. E. Tollefsen,
et al., The role of omics in the application of adverse outcome pathways for chemical risk assessment, Toxicol. Sci., 2017, 158, 252–262 CrossRef CAS PubMed.
- X. Zhang, P. Xia, P. Wang, J. Yang and D. J. Baird, Omics advances in ecotoxicology, Environ. Sci. Technol., 2018, 52, 3842–3851 CrossRef CAS PubMed.
- P. Antczak, T. A. White, A. Giri, F. Michelangeli, M. R. Viant and M. T. Cronin,
et al., Systems biology approach reveals a calcium-dependent mechanism for basal toxicity in Daphnia magna, Environ. Sci. Technol., 2015, 49, 11132–11140 CrossRef CAS PubMed.
- D. Batista, C. Pascoal and F. Cássio, How do physicochemical properties influence the toxicity of silver nanoparticles on freshwater decomposers of plant litter in streams?, Ecotoxicol. Environ. Saf., 2017, 140, 148–155 CrossRef CAS PubMed.
- B. Nowack, J. F. Ranville, S. Diamond, J. A. Gallego-Urrea, C. Metcalfe and J. Rose,
et al., Potential scenarios for nanomaterial release and subsequent alteration in the environment, Environ. Toxicol. Chem., 2012, 31, 50–59 CrossRef CAS PubMed.
- A. Arini, F. Pierron, S. Mornet and M. Baudrimont, Bioaccumulation dynamics and gene regulation in a freshwater bivalve after aqueous and dietary exposures to gold nanoparticles and ionic gold, Environ. Sci. Pollut. Res., 2019, 27(4), 3637–3650 CrossRef PubMed.
- M. Dellali, A. Khallouli, A. H. Harrath, F. Falodah, S. Alwasel and H. Beyrem,
et al., Effects of Au/TiO2 metallic nanoparticles on Unio ravoisieri: Assessment through an oxidative stress and toxicity biomarkers, Environ. Sci. Pollut. Res., 2021, 28, 18176–18185 CrossRef CAS PubMed.
- E. G. Canli and M. Canli, Antioxidant system biomarkers of freshwater mussel (Unio tigridis) respond to nanoparticle (Al2O3, Cuo, TiO2) exposures, Biomarkers, 2021, 5, 1–27 Search PubMed.
- M. Garaud, J. Trapp, S. Devin, C. Cossu-Leguille, S. Pain-Devin and V. F. Lten,
et al., Multibiomarker assessment of cerium dioxide nanoparticle (nCeO2) sublethal effects on two freshwater invertebrates, Dreissena polymorpha and Gammarus roeseli, Aquat. Toxicol., 2015, 158, 63–74 CrossRef CAS PubMed.
- L. Gnatyshyna, H. Falfushynska, O. Horyn, V. Khoma and O. Stoliar, Biochemical responses of freshwater mussel Unio tumidus to titanium oxide nanoparticles, Bisphenol A, and their combination, Ecotoxicology, 2019, 28, 923–937 CrossRef CAS PubMed.
- M. A. Moustafa, H. S. Mossalem, R. M. Sarhan, A. A. Abdel-Rahman and E. M. Hassan, The potential effects of silver and gold nanoparticles as molluscicides and cercaricides on Schistosoma mansoni, Parasitol. Res., 2018, 117, 3867–3880 CrossRef CAS PubMed.
- S. N. Luoma, T. Stoiber, M. N. Croteau, I. Romer, R. Merrifeld and J. R. Lead, Effect of cysteine and humic acids on bioavailability of Ag from Ag nanoparticles to a freshwater snail, NanoImpact, 2016, 2, 61–69 CrossRef.
- C. Völker, T. Gräf, I. Schneider, M. Oetken and J. Oehlmann, Combined effects of silver nanoparticles and 17α-ethinylestradiol on the freshwater mudsnail Potamopyrgus antipodarum, Environ. Sci. Pollut. Res., 2014, 21, 10661–10670 CrossRef PubMed.
- T. Ramskov, V. E. Forbes, D. Gilliland and H. Selck, Accumulation and effects of sediment-associated silver nanoparticles to sediment-dwelling invertebrates, Aquat. Toxicol., 2015, 166, 96–105 CrossRef CAS PubMed.
- D. Ali, P. G. Yadav, S. Kumar, H. Ali, S. Alarifi and A. H. Harrath, Sensitivity of freshwater pulmonate snail Lymnaea luteola to silver nanoparticles, Chemosphere, 2014, 104, 134–140 CrossRef CAS PubMed.
- K. Afshinnia, M. Sikder, B. Cai and M. Baalousha, Effect of nanomaterial and media physicochemical properties on ag nm aggregation kinetics, J. Colloid Interface Sci., 2017, 487, 192–200 CrossRef CAS PubMed.
- P. Zhang, H. Xiao, Y. Ma, K. Lu, Y. Zhao and Z. Zhang, Distribution and bioavailability of ceria nanoparticles in an aquatic ecosystem model, Chemosphere, 2012, 89, 530–535 CrossRef CAS PubMed.
- M. Lima, M. Pereira, P. Filho, W. Siqueira, H. Silva and E. Frana,
et al., Studies on toxicity of suspensions of cdte quantum dots to Biomphalaria glabrata mollusks, Environ. Toxicol. Chem., 2019, 38, 2128–2136 CrossRef CAS PubMed.
- M. M. Attia, S. M. Soliman and M. A. Khalf, Hydrophilic nanosilica as a new larvicidal and molluscicidal agent for controlling of major infectious diseases in Egypt, Vet. World, 2017, 10, 1046–1051 CrossRef CAS PubMed.
- T. Ma, W. Meng, S. Gong and B. Tian, Impacts of sediment organic matter content and ph on ecotoxicity of coexposure of TiO2 nanoparticles and cadmium to freshwater snails Bellamya aeruginosa, Arch. Environ. Contam. Toxicol., 2017, 72, 1–13 CrossRef PubMed.
- M. K. Yeo and D. H. Nam, Influence of different types of nanomaterials on their bioaccumulation in a paddy microcosm: A comparison of TiO2 nanoparticles and nanotubes, Environ. Pollut., 2013, 178, 166–172 CrossRef CAS PubMed.
- J. I. Kim, H.-G. Park and K.-H. Chang,
et al., Trophic transfer of nano-TiO2 in a paddy microcosm: A comparison of single-dose versus sequential multi-dose exposures, Environ. Pollut., 2016, 212, 316–324 CrossRef CAS PubMed.
- D. Ali, H. Ali, S. Alarifi, S. Kumar, M. Serajuddin and A. P. Mashih,
et al. Impairment of DNA in a freshwater gastropod (Lymnea luteola) after exposure to titanium dioxide nanoparticles, Arch. Environ. Contam. Toxicol., 2015, 68, 543–552 CrossRef CAS PubMed.
- I. Blinova, J. Niskanen, P. Kajankari, L. Kanarbik, A. Käkinen and H. Tenhu,
et al., Toxicity of two types of silver nanoparticles to aquatic crustaceans Daphnia magna and Thamnocephalus platyurus, Environ. Sci. Pollut. Res., 2013, 20, 3456–3463 CrossRef CAS PubMed.
- K. Mehennaoui, S. Cambier, T. Serchi, J. Ziebel, E. Lentzen and N. Valle,
et al., Do the pristine physico-chemical properties of silver and gold nanoparticles influence uptake and molecular effects on Gammarus fossarum (crustacea amphipoda)?, Sci. Total Environ., 2018, 643, 1200–1215 CrossRef CAS PubMed.
- T. T. Thai, P. T. Luu, N. X. Quang and D. T. Son, Chronic effects of silver nanoparticles on micro-crustacean Daphnia lumholtzi, Natural Science and Technology, 2020, 36(2), 54–61 Search PubMed.
- R. Ishwarya, B. Vaseeharan, S. Shanthi, S. Ramesh, P. Manogari and K. Dhanalakshmi,
et al., Green synthesized silver nanoparticles: Toxicity against poecilia reticulata fishes and Ceriodaphnia cornuta crustaceans, J. Cluster Sci., 2017, 28, 519–527 CrossRef CAS.
- H. C. Poynton, J. M. Lazorchak, C. A. Impellitteri, B. J. Blalock, K. Rogers and H. J. Allen,
et al., Toxicogenomic responses of nanotoxicity in Daphnia magna exposed to silver nitrate and coated silver nanoparticles, Environ. Sci. Technol., 2012, 46, 6288–6296 CrossRef CAS PubMed.
- A. Shokry, M. Khalil, H. Ibrahim, M. Soliman and S. Ebrahim, Acute toxicity assessment of polyaniline/ag nanoparticles/graphene oxide quantum dots on Cypridopsis vidua and Artemia salina, Sci. Rep., 2021, 11, 1–9 CrossRef PubMed.
- B. Janani, D. A. Al Farraj, L. L. Raju, M. S. Elshikh, N. A. Alkubaisi and A. M. Thomas,
et al., Cytotoxicological evaluation of copper oxide nanoparticles on green algae, bacteria and crustacean systems, J. Environ. Health Sci. Eng., 2020, 18, 1465–1472 CrossRef CAS PubMed.
- F. Wu, B. J. Harper, L. E. Crandon and S. L. Harper, Assessment of cu and cuo nanoparticle ecological responses using laboratory small-scale microcosms, Environ. Sci.: Nano, 2020, 7, 105–115 RSC.
- F. Arratia, P. Olivares-Ferretti, A. García-Rodríguez, R. Marcos and E. R. Carmona, Comparative toxic effects of copper-based nanoparticles and their microparticles in Daphnia magna by using natural freshwater media, N. Z. J. Mar. Freshwater Res., 2019, 53, 460–469 CrossRef CAS.
- F. Shariati, T. Poordeljoo and P. Zanjanchi, The acute toxicity of SiO2 and Fe3O4 nano-particles on Daphnia magna, Silicon, 2020, 12, 1–6 CrossRef.
- C. Tan and W. X. Wang, Modification of metal bioaccumulation and toxicity in Daphnia magna by titanium dioxide nanoparticles, Environ. Pollut., 2014, 186, 36–42 CrossRef CAS PubMed.
- V. Iswarya, M. Bhuvaneshwari, N. Chandrasekaran and A. Mukherjee, Individual and binary toxicity of anatase and rutile nanoparticles towards Ceriodaphnia dubia, Aquat. Toxicol., 2016, 178, 209–221 CrossRef CAS PubMed.
- N. Adam, C. Schmitt, J. Galceran, E. Companys, A. Vakurov and R. Wallace,
et al., The chronic toxicity of zno nanoparticles and ZnCl2 to Daphnia magna and the use of different methods to assess nanoparticle aggregation and dissolution, Nanotoxicology, 2014, 8, 709–717 CAS.
- L. Lin, M. Xu, H. Mu, W. Wang, J. Sun and J. He,
et al., Quantitative proteomic analysis to understand the mechanisms of zinc oxide nanoparticle toxicity to Daphnia pulex (crustacea: Daphniidae): Comparing with bulk Zinc oxide and Zinc salt, Environ. Sci. Technol., 2019, 53, 5436–5444 CrossRef CAS PubMed.
- R. Bacchetta, N. Santo, M. Marelli, G. Nosengo and P. Tremolada, Chronic toxicity effects of ZnSO4 and ZnO nanoparticles in Daphnia magna, Environ. Res., 2017, 152, 128–140 CrossRef CAS PubMed.
|
This journal is © The Royal Society of Chemistry 2022 |
Click here to see how this site uses Cookies. View our privacy policy here.