DOI:
10.1039/D0QI00766H
(Review Article)
Inorg. Chem. Front., 2020,
7, 3837-3874
Noble-metal-free nanocatalysts for hydrogen generation from boron- and nitrogen-based hydrides
Received
28th June 2020
, Accepted 13th August 2020
First published on 14th August 2020
Abstract
Hydrogen has attracted much attention as a globally accepted clean energy carrier. Currently, the search for safe and efficient hydrogen storage materials is one of the most difficult challenges for the upcoming hydrogen economy. Boron- and nitrogen-based hydrides, such as metal borohydrides (e.g. NaBH4), ammonia borane (NH3BH3), ammonia (NH3), hydrous hydrazine (N2H4·H2O), and hydrazine borane (N2H4BH3), have received much attention as potential chemical hydrogen storage materials because of their high hydrogen contents and the advantage of CO-free H2 produced. In recent years, substantial efforts have been devoted to research highly efficient catalysts to significantly improve the kinetic properties for hydrogen evolution from the hydrolysis of sodium borohydride and ammonia borane, and selective decomposition of ammonia, hydrous hydrazine and hydrazine borane. Among them, non-noble metal catalysts have been widely considered as potential candidates due to their low cost, abundant reserves, and relatively high catalytic activities. In this review, we focus on the recent advances in non-noble metal catalyst design, synthesis and applications in hydrogen generation from boron- and nitrogen-based hydrides.
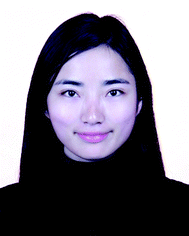 Qilu Yao | Qilu Yao received her PhD (2017) degree in chemistry from Jiangxi Normal University under the supervision of Prof. Zhang-Hui Lu. Since 2017, she has been working as a research associate at the Institute of Advanced Materials, Jiangxi Normal University. Her research is centered on the design and preparation of nanostructured materials and their applications in energy-/environment-related catalysis. |
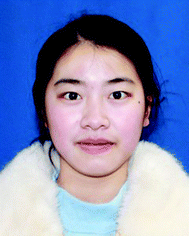 Yiyue Ding | Yiyue Ding was born in Hunan, P. R. China in 1999. She received her bachelor's degree in Chemistry from Changchun Normal University (2019), China. She is currently studying for her master's degree at Jiangxi Normal University under the supervision of Prof. Zhang-Hui Lu. Her research is mainly focused on the design and synthesis of nanocatalysts for hydrogen production from chemical hydrogen storage materials. |
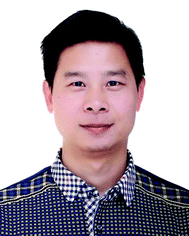 Zhang-Hui Lu | Zhang-Hui Lu is currently a professor at Jiangxi Normal University. He received his PhD degree in Chemical Science and Engineering in 2011 from Kobe University (Japan) under the supervision of Prof. Qiang Xu. He is the winner of the First Prize in the Natural Science Award of Jiangxi Province in 2019 and has published more than 100 papers with citations >4000. His current research interest is mainly focused on synthesizing micro/nanostructured materials and exploring their applications in the area of heterogeneous catalysis and electro-catalysis for clean energy and environmental clean-up. |
1. Introduction
The advancement of industrialization and economic growth means that human society is more heavily dependent on energy than ever before. The currently used fossil fuels (coal, crude oil, natural gas, etc.) are considered to be non-renewable energy and have limited reserves. In addition, the extraction, transport, and consumption of fossil fuels can also cause environmental pollution problems. Thus, it is urgent to find a clean and renewable alternative to fossil fuels. Hydrogen has been identified as a potential alternative energy carrier for future energy supplies because it is clean, renewable, and environment friendly, and has high energy density.1–4 The use of hydrogen fuel cells in portable electronic devices or vehicles requires lightweight hydrogen storage or on-board hydrogen evolution. In particular, for vehicular applications, the most important requirements are safety, ease of control and fast reaction kinetics as well as high hydrogen content. To this end, the search for secure and efficient hydrogen storage materials has become extremely important.
In the last decades, several materials for hydrogen storage such as metal hydrides,5 carbonaceous materials,6 zeolites,7 metal organic frameworks,8 and organic hydrides,9,10 have been extensively investigated, while big challenges still remain. Chemical hydrogen storage materials have high hydrogen contents and store hydrogen in the form of chemical bonds, and they are considered as highly promising hydrogen sources for fuel cells.11–14 Among them, boron- and nitrogen-based hydrides, such as metal borohydrides (e.g. NaBH4, 10.8 wt%), ammonia borane (NH3BH3, 19.6 wt%), ammonia (NH3, 17.7 wt%), hydrous hydrazine (N2H4·H2O, 8.0 wt%), and hydrazine borane (N2H4BH3, 15.4 wt%), have received much attention in recent years.15–21 These materials are not only high in hydrogen content, but are also easy to store and transport under mild conditions, making them highly potential hydrogen storage materials.
In order to achieve effective hydrogen evolution from boron- and nitrogen-based hydrides under ambient conditions, it is highly desired to develop economical, highly efficient and stable catalysts. To date, a lot of noble/non-noble metals and their composites have been developed as catalysts for hydrogen evolution from boron- and nitrogen-based hydrides.21–26 Among them, noble metal-based catalysts exhibit excellent catalytic activity, but they are unsuitable for large scale practical applications due to their high cost and insufficient reserves in the Earth's crust.27–30 Therefore, the development of cost-effective catalysts is of great importance for practical applications. Non-noble metal (e.g. Fe, Co, Ni, etc.) catalysts have been considered as potential candidates due to their low cost, abundant reserves, and relatively high catalytic performances.24,31,32 In this review, we summarize the recent developments in non-noble catalysts for hydrogen generation from these boron- and nitrogen-based chemical hydrogen storage materials.
2. Metal borohydrides
Metal borohydrides, such as LiBH4, NaBH4, KBH4 and Mg(BH4)2, have received considerable interest as promising hydrogen storage materials due to their high gravimetric hydrogen capacities.33–43 Compared to LiBH4, KBH4 and Mg(BH4)2, NaBH4 is more widely studied because it provides a safe and low-cost route to produce hydrogen.16,21,33 Thus, NaBH4 is taken as a representative for discussion.
Hydrogen stored in NaBH4 can be released by either thermolysis or hydrolysis.33,44–48 However, the thermolysis of NaBH4 is not conceivable because it requires high temperature (>500 °C), and it inevitably produces toxic species. Alternatively, hydrolysis can be used to release H2 from NaBH4 easily and in a controllable way under friendly conditions.33,47,48 As shown in eqn (1), half of the hydrogen produced originates from water, which is also an advantage of this reaction. In addition, the byproduct of this hydrolysis reaction (NaBO2) is nontoxic and can be recycled for regeneration, which facilitates its use in fuel cells. In general, NaBH4 is not stable in aqueous solution and can spontaneously release H2 without catalysts. However, the self-hydrolysis is too slow and uncontrollable, and only 7–8% of NaBH4 can be converted. In order to suppress the self-hydrolysis, NaOH is often added into the NaBH4 solution as a stabilizer. The alkaline NaBH4 solution can only be hydrolyzed by using suitable catalysts.
| NaBH4 + 2H2O → NaBO2 + 4H2 | (1) |
Unlike the simple hydrolysis reaction formula shown in eqn (1), the detailed mechanism of the hydrolysis of NaBH4 over transition metal catalysts is complicated and not fully understood.49–53 As early as in 1971, Holbrook and Twist proposed a plausible mechanism for metal (M) catalyzed hydrolysis of NaBH4.49 Firstly, BH4− ions are chemisorbed on a metal active site surface, forming M–BH3− and M–H intermediate species. Subsequently, M–BH3− reacts with OH− and H2O, possibly via a BH3 intermediate, to form BH3(OH)− and M–H intermediate species. Then, the intermediate BH3(OH)− finally converts into B(OH)4− by the replacement of B–H bonds with B–OH− bonds. Meanwhile, the intermediate M–H reacts with another M–H to generate H2 and to regenerate the metal active sites. Peña-Alonso et al. suggested that the intermediate M–H directly reacts with H2O to generate H2.50 Guella et al. proposed a mechanism based on 11B NMR measurements, and suggested that the rate-controlling step of the metal catalyzed hydrolysis of NaBH4 is the cleavage of the O–H bond in water.51,52 It is worth noting that the catalytic hydrolysis of NaBH4 is closely related to the metal active sites, as there is a charge transfer between the metal active sites and NaBH4 or intermediates. Therefore, the development of efficient metal catalysts is very important.
Schlesinger and coworker first found that adding an acid into aqueous NaBH4 solution can significantly accelerate the hydrolysis reaction.54 Since then, a number of catalysts have been investigated to accelerate the NaBH4 hydrolysis. Noble-metal-based catalysts such as Ru, Rh, Pt, Pd and relevant alloys display excellent catalytic performances, but the high cost and scarcity of noble metals limit their industrial applications.51,55–58 Low-cost and Earth-abundant transition metal (Co, Ni, and Fe), metal boride and metal phosphide catalysts are viable alternatives.31,32 Among them, Co-based catalysts have been proven to be highly effective at catalyzing NaBH4 hydrolysis (Table 1).31,59–61
Table 1 Catalytic activities for hydrogen evolution from the hydrolysis of NaBH4 by different catalysts
Catalyst |
Synthetic method |
Temp. (K) |
Activity (mL min−1 g−1) |
E
a (kJ mol−1) |
Ref. |
Intrazeolite Co nanoclusters |
Ion-exchange and chemical reduction |
298 |
— |
34 |
62
|
Co/CCs |
Hydrothermal and impregnation–reduction |
293 |
10 400 |
24.04 |
66
|
Co/ACs |
Hydrothermal and impregnation–reduction |
298 |
11 220 |
38.4 |
67
|
Co@C-700 |
Pyrolysis |
303 |
— |
56.9 |
68
|
Co@NMGC |
Pyrolysis |
298 |
3575 |
35.2 |
69
|
Co/IR-120 |
Wet-chemical reduction |
308 |
200 |
66.67 |
70
|
Co/Fe3O4@C |
Impregnation–reduction |
298 |
1403 |
49.2 |
71
|
Fe3O4@C–Co |
Solvothermal and impregnation–reduction |
298 |
1746 |
47.3 |
72
|
Co/SiO2 |
Impregnation–reduction |
313 |
8701 |
59 |
32
|
Cryogel p(AAm)–Co |
In situ chemical reduction |
303 |
1130.2 |
39.7 |
73
|
Cryogel p(AAm)–Ni |
In situ chemical reduction |
303 |
579.4 |
— |
73
|
Ni/graphitic layer |
Chemical vapor deposition |
298 |
600 |
— |
74
|
CoB |
Chemical reduction and post-annealing |
288 |
2970 |
— |
77
|
Co–B thin films |
Pulsed laser deposition |
298 |
3300 |
— |
78
|
Co–B hollow spheres |
Chemical reduction and post-annealing |
298 |
2720 |
45.5 |
79
|
CoB/SiO2 |
Impregnation–reduction |
298 |
10 586 |
— |
80
|
Mesoporous Co–B |
Chemical reduction |
303 |
3350 |
40 |
81
|
Co–Cr–B |
Chemical reduction |
298 |
3400 |
37 |
85
|
Co–Mo–B |
Chemical reduction |
298 |
2875 |
39 |
86
|
Co–Mo–B/carbon cloth |
Two-step electrodeposition |
303 |
1280.9 |
51 |
91
|
Co–Mn–B |
Chemical reduction and post-annealing |
293 |
1440 |
52.1 |
92
|
Co–Ti–B |
Chemical reduction |
323 |
7760 |
49.88 |
93
|
Co–Ce–B |
Chemical reduction and carbonization |
303 |
4760 |
33.1 |
94
|
Co–Zn–B |
In situ chemical reduction |
303 |
2180 |
35.92 |
95
|
Co–B/carbon |
Impregnation–reduction |
298 |
1127.2 |
57.8 |
96
|
CoB/o-CNTs |
Impregnation–reduction |
298 |
3041 |
37.63 |
98
|
CoB/TiO2 |
Impregnation–reduction |
293 |
6738 |
51 |
99
|
Co–B/nickel foam |
Electroless plating |
298 |
111 |
33 |
103
|
Ni–B–silica nanocomposite |
In situ chemical reduction |
298 |
1916 |
60.7 |
104
|
Ni–B/Ni foam |
Dipping–chemical reduction |
303 |
— |
61.841 |
105
|
Multi-shaped Ni–B |
Complexing–reduction |
303 |
— |
64.9 |
106
|
NiB/NiFe2O4 |
Impregnation–reduction |
298 |
299.88 |
72.52 |
107
|
Fe–B/C cloth |
Adsorption–chemical reduction |
298 |
813 |
— |
108
|
Fe–B/Ni foam |
Chemical reduction |
323 |
5487 |
64.26 |
109
|
Cu–B |
Complexing–reduction preparation |
303 |
6500 |
23.79 |
110
|
Co–P/Cu substrate |
Electroplating |
303 |
954 |
— |
111
|
Co–P |
Electroless deposition |
303 |
3300 |
60.2 |
112
|
Co–P/Cu sheet |
Electroless deposition |
303 |
1846 |
48.1 |
113
|
Co–P/Cu sheet |
Electroless plating |
323 |
2275.1 |
27.9 |
114
|
CoP/Ti mesh |
Phosphidation |
298 |
6100 |
42.01 |
115
|
CoP nanowire array/Ti |
Phosphidation |
293 |
6500 |
41 |
116
|
Co–W–P/Cu sheet |
Electroless deposition |
303 |
5000 |
22.8 |
117
|
Co–W–P/γ-Al2O3 |
Electroless deposition |
318 |
11 820 |
49.58 |
118
|
Co–W–P/carbon cloth |
Electroless deposition |
303 |
4379 |
27.18 |
119
|
Co–Ni–P/Cu substrates |
Electroless deposition |
303 |
2479 |
— |
120
|
Co–Ni–P/Cu sheet |
Electroless plating |
303 |
2172.4 |
53.5 |
121
|
Cu–Co–P/γ-Al2O3 |
Electroless deposition |
298 |
1115 |
47.8 |
122
|
Fe–CoP/Ti |
Phosphidation |
298 |
6060 |
39.6 |
123
|
2.1. Metal catalysts
Cobalt catalysts have been reported to be effective in catalytic hydrolysis of NaBH4.31,59 These are usually deposited on a support material in the form of Co2+ ions and then reduced to metal Co. Microporous and mesoporous materials are considered as suitable support materials because it can effectively limit the growth or sintering of metal nanoparticles (NPs). Özkar and coworkers reported the synthesis of intrazeolite Co nanoclusters by using ion-exchange of Co2+ ions with the extra-framework Na+ ions in zeolite-Y followed by reduction with NaBH4.62 The obtained intrazeolite Co nanoclusters provided 36
000 turnovers in the hydrolysis of NaBH4 and retained 59% of their initial catalytic activity after the fifth run. Later on, Kwon and coworkers developed a hydroxyapatite-supported Co (Co/HAP) pre-catalyst, which showed long-term stability, retaining 75% of its initial catalytic activity over 20 days of use.63 Auroux and coworkers chose various materials with different acid/base surface properties as supports (hydrotalcites, KF/Al2O3, and heteropolyanions) to immobilize Co NPs.64 Among them, a heteropolyanion supported Co pre-catalyst showed the highest hydrogen generation rate in NaBH4 hydrolysis.
Various carbon supported Co catalysts have been reported, which have the advantages of relatively low cost and excellent catalytic hydrogen production performance.65–69 Gou and coworker found that Co supported on colloidal carbon spheres (Co/CCS) obtained from glucose exhibited a high HGR (10
400 mL min−1 g−1) for NaBH4 hydrolysis at 293 K, whereas Co supported on carbon aerogels (Co/ACs) showed a higher HGR of 11
220 mL min−1 g−1.66,67 Zhang et al. fabricated a Co@C pre-catalyst by using Co-MOF as the starting precursor.68 The Co@C-700 pre-catalyst exhibited higher catalytic activity than Co@C-600 and retained 93.1% of its initial catalytic activity after five cycles in NaBH4 hydrolysis. Recently, Li et al. synthesized nitrogen-doped mesoporous graphitic carbon encapsulated Co NPs (Co@NMGC) by a simple one-step pyrolysis of a complex of Co(NO3)2·6H2O and ethylenediaminetetraacetic acid (EDTA).69 Co@NMGC annealed at 773 K showed high catalytic activity and remarkable durability, retaining 82.5% of its initial catalytic activity after 20 hydrolysis cycles.
Magnetic catalysts can be easily recycled with a permanent magnet from a spent NaBH4 system after hydrogen production.70–72 Chen et al. synthesized a magnetic Co/IR-120 pre-catalyst by a combination of ion-exchange and reduction methods.70 A stable generation rate of highly pure hydrogen near 200 mL min−1 g−1 was achieved over the Co/IR-120 pre-catalyst in 100 mL of 5 wt% NaBH4 solution. Kim and coworkers reported novel Co NPs supported on magnetic carbon (Co/Fe3O4@C) via a modified wetness impregnation–chemical reduction method.71 The abundant oxygen-containing-groups on the surface of the carbon layer can effectively immobilize and stabilize the Co NPs, thereby enhancing their catalytic activity for the hydrolysis of NaBH4. Ferromagnetic metals Co, Ni, and Fe were supported on porous SiO2via an incipient wetness impregnation method.32 The catalytic activities of ferromagnetic metal/SiO2 followed the order Co/SiO2 > Ni/SiO2 > Fe/SiO2. Furthermore, various other Ni-based catalysts have been reported, but Co-based catalysts have been found to be the most superior in terms of the maximum hydrogen generation rate.73–75
2.2. Metal boride catalysts
Schlesinger and co-workers first found that Co2B was active for hydrogen evolution from the hydrolysis of NaBH4.54 Afterwards, there have been many reports on the preparation and catalytic activity of Co–B catalysts.60,61,76–81 It has been reported that the structure and catalytic activity of Co–B catalysts were sensitive to their preparation conditions. Jeong and coworkers prepared a Co–B pre-catalyst by the chemical reduction method using NaBH4 as a reductant.76 The obtained Co–B catalyst was amorphous and showed excellent catalytic performance for hydrogen generation from an aqueous alkaline NaBH4 solution, which was comparable to that of a Ru catalyst. Wu and coworker found that amorphous Co–B after heat treatment showed much higher catalytic activity than the untreated sample, which is attributed to the formation of the crystalline state of Co–B.77 In addition, the Co–B catalyst treated at 500 °C exhibited the best crystallization and showed the highest hydrogen generation performances. When the Co–B catalyst was heated at 700 °C, the catalytic activity of the catalyst drastically decreased due to the decomposition of Co–B to form metal Co.
To further obtain efficient Co–B catalysts, the synthesis parameters such the cobalt salt, ratio of NaBH4/Co2+, calcination temperature and solvent type are investigated. Kim and coworkers examined the catalytic activity of a Co–B catalyst prepared by using different Co precursors (CoCl2 and CoSO4) and NaBH4/Co2+ molar ratios (0.67, 1.5, and 3) at different calcination temperatures (130, 250 and 450 °C).82 With CoCl2 as the precursor, a NaBH4/Co2+ molar ratio of 1.5, and a calcination temperature of 250 °C, the hydrogen evolution rate of the Co–B catalyst was the highest. Demirci and coworkers further confirmed that CoCl2 is the best precursor, which showed a four times higher hydrogen generation rate than those of other Co salts (Co(CH3COO)2, CoSO4, CoF2, and Co(NO3)2).83 The sizes, morphologies, and properties of the Co–B catalyst are also greatly influenced by the solvents used. Zhao and coworkers found that the Co–B catalyst was more likely to agglomerate as the viscosity of the solvent increased.84 The catalytic activities of Co–B catalysts prepared in different solvents are in the order of MeOH > H2O > EtOH > PrOH.
Although optimization of the synthesis parameters can improve the catalytic properties, the exothermic nature of the reduction reaction involves high surface energy and is easily prone to aggregation, leading to deteriorated activity. An efficient route to avoid agglomeration and increase the active surface area of Co–B particles is by doping with transition metals (Cr, Mo, W, etc.).85–91 These dopant metals, mainly in the form of oxides, act as atomic barriers that are able to significantly increase the surface area of the catalyst by avoiding agglomeration. Additionally, these dopant metals can also act as electron donors to increase the electron density on the active metal, which can further improve the catalytic activity of the Co–B catalyst. For instance, Patel et al. conducted a systematic and comparative study on transition metal (Cr, Mo, W, Cu, Ni and Fe) doped Co–B catalysts for hydrogen generation by hydrolysis of NaBH4.86 They found that the hydrogen generation rate of Co–B catalysts doped with Cr, W, Mo, and Cu is about 3–4 times higher than that of the undoped catalysts. Ni and Fe are only able to slightly enhance the catalytic activity of the Co–B catalyst. In recent years, Co–B catalysts doped with Mn,92 Ti,93 Ce94 and Zn95 have been investigated and they also showed positive promotion effects on the hydrogen evolution of NaBH4 hydrolysis. Another efficient route to enhance the catalytic performance is by supporting Co–B NPs in a support material, such as carbon,96–98 Al2O3,99 CeO2,99 TiO2,99–101 and Ni foam.102,103 The above supports can effectively inhibit the agglomeration of metal NPs and increase the active sites of the catalysts, and therefore can effectively improve the catalytic activity of the catalysts.
Other metal boride catalysts such as Ni–B, Cu–B, and Fe–B are also investigated for hydrogen evolution from the hydrolysis of NaBH4. Kim and co-workers reported a Ni–B–silica nanocomposite pre-catalyst via an in situ reduction method.104 The obtained amorphous pre-catalyst was active, providing a hydrogen generation of 1916 mL min−1 g−1. Later on, a stable and physically adhesive Ni–B on Ni foam was prepared by Lee and coworkers via a dipping-chemical reduction process and it exhibited high catalytic activity towards the hydrolysis of NaBH4.105 Zhang and coworkers prepared an amorphous Ni–B pre-catalyst via an ultrasonic complexing reduction route.106 They found that the chemical composition and phase state of the Ni–B pre-catalyst were greatly influenced by its complexing ability. The stronger the complexing ability of the complex, the smaller the size and the higher the dispersibility of the formed Ni–B, resulting in a higher catalytic activity of Ni–B. Recently, a Ni–B/NiFe2O4 magnetic pre-catalyst with a metal loading of 10 wt% retained 85% of its initial activity after the fifth run, and the Ni–B/NiFe2O4 can be easily separated from the reaction solution using an external magnet.107
Lee reported a Fe–B/C cloth pre-catalyst by electrochemical adsorption techniques.108 The hydrogen generation rate using the Fe–B/C cloth pre-catalyst can achieve 813 mL min−1 g−1 at room temperature. The Fe–B/Ni foam catalyst was also prepared by the same group for hydrogen generation from NaBH4 in a mixture solution of H2O and CH3OH.109 They found that using methanol as an additive to water can increase and stabilize the rate of hydrogen generation, but with lower gravimetric capacity. Bekirogullari compared the catalytic activities of Cu–B, Fe–B, and Ni–B pre-catalysts for hydrogen evolution from NaBH4.110 The results reveal that the catalytic activities of these metal borides followed an order of Cu–B > Ni–B > Fe–B.
2.3. Metal phosphide catalysts
Like Co–B, the other counterpart Co–P catalysts were also able to produce hydrogen from hydrolysis of NaBH4.111,112 Kwon and co-workers first reported a Co–P pre-catalyst electrodeposited on a Cu substrate in a sulfate based solution containing H2PO2− ions.111 They found that the amorphous Co–P pre-catalyst with 13 at% P showed the best hydrogen generation rate of 954 mL min−1 g−1 in a solution of 1 wt% NaOH and 10 wt% NaBH4 at 303 K, which is 18 times higher than that of a pure Co pre-catalyst. To further obtain efficient Co–P catalysts, the synthesis parameters such as the pH value, reactant concentration and deposition time are investigated.113,114 Chen's group found that the Co–P pre-catalyst formed at a pH value of 12.5, a NaH2PO2 concentration of 0.8 M, and a deposition time no more than 6 min showed the highest catalytic activity for the hydrolysis of NaBH4 solution.113 By tuning the deposition temperature, nanostructured Co–P/Cu sheets with different morphologies (nanoplatelets, nanospheres, pores and nanoclews) were selectively obtained.114 Notably, the nanostructured Co–P pre-catalyst deposited at 50 °C displayed novel hierarchical architectures and exhibited the highest catalytic properties with a high hydrogen release rate of 2275.1 mL min−1 g−1 and a low apparent activation energy of 27.9 kJ mol−1.
The Co–P based catalysts prepared by chemical and electrodeposition methods are usually formed in powder form. Compared to nanoparticle catalysts, monolithic catalysts have obvious advantages such as no aggregation, easy separation and reuse, and they can be utilized as an on/off switch for on-demand hydrogen generation.115,116 Sun's group designed 3D cobalt phosphide nanosheet arrays on Ti mesh (CoP/Ti mesh) via a topotactical conversion reaction.115 The obtained 3D monolithic pre-catalyst showed high catalytic activity, providing a maximum hydrogen generation rate of 6100 mL min−1 g−1 for NaBH4 hydrolysis in alkaline media and an activation energy of 42.01 kJ mol−1. Similarly, Liu and coworkers reported the synthesis of a CoP nanowire array integrated on a Ti mesh (CoP NA/Ti) for hydrolytic dehydrogenation of NaBH4 in basic solutions.116
Not only binary Co–P but also ternary Co–M–P (M = W, Ni, Cu, Fe, etc.) catalysts have been developed as robust catalysts for hydrogen generation from the hydrolysis of NaBH4 alkaline solution.117–123 Ma and coworkers found that the catalytic activity of the amorphous Co–P pre-catalyst could be markedly improved by incorporating W.117 However, the hydrogen generation rate of the Co–W–P pre-catalyst lost 49% of its activity after 5 cycles. Furthermore, by depositing Co–W–P on γ-Al2O3 and carbon cloth, the catalytic activity and stability of the pre-catalyst can be significantly improved.118,119 Kim et al. synthesized porous Co–Ni–P on Cu substrates by electrodeposition.120 The three-dimensional Co–Ni–P pre-catalyst formed at a high cathodic current density (>0.5 A cm−2) had a larger surface area than the two-dimensional pre-catalyst formed at a low cathodic current density (0.01 A cm−2), which significantly increased the rate of hydrogen generation in the alkaline NaBH4 solution. Diverse nanostructures of Co–Ni–P pre-catalysts have been prepared on Cu sheets via the electroless plating method by tuning the depositional pH value.121 Compared with football-, granular-, and shuttle-like Co–Ni–P, mockstrawberry-like Co–Ni–P exhibited the highest catalytic properties. It has been reported that the alloying of Cu into Co–Cu–P was also able to improve their catalytic activity in hydrogen production via NaBH4 hydrolysis.122 Lately, Fe-doped CoP nanoarrays on Ti foil (Fe-CoP/Ti) have been investigated by Sun's group as a robust catalyst for NaBH4 hydrolysis, resulting in a hydrogen generation rate of 6060 mL min−1 g−1 and an activation energy of about 39.6 kJ mol−1.123
Overall, Co-based catalysts are demonstrated to be cost-effective, active and stable catalysts for hydrogen generation from the hydrolysis of NaBH4. In addition, most of the reported metal borides/phosphides are in an amorphous state.61,124 The materials in the amorphous state have great structural distortion and high concentrations of unsaturated coordination sites, which make them show high catalytic activity. After heat treatment, the amorphous metal borides can be transformed into a crystalline state, because of which they usually exhibit higher catalytic performance. However, the material after heat treatment is often a mixture of the metal, borides and/or oxides. Thus, its composition and surface structures are complex, and it is difficult to identify the real active sites. Furthermore, the mechanism of their catalytic hydrogen production is not clear. Particularly, what is the exact active composition of Co and B or P to achieve high catalytic activity? Therefore, future research can be focused on mechanistic studies of the hydrolysis reaction at the surface of the Co-based catalysts.
3. Ammonia borane
Ammonia borane (NH3BH3, AB) is a stable solid at room temperature with a density of 0.780 g cm−3 and a melting point of 112–114 °C.125 Owing to its high hydrogen content (19.6 wt%), low molecular weight (30.9 g mol−1), and nontoxic and environmentally friendly nature, NH3BH3 has been considered as a promising hydrogen storage material.126–133 Hydrogen stored in NH3BH3 can be released by either thermal decomposition in the solid phase or catalytic solvolysis (hydrolysis and methanolysis) under mild conditions.126,134–138 There are considerable works involving hydrogen release from the thermal decomposition of NH3BH3.134–137 Although pure NH3BH3 possesses 3 equivalents of H2, only 2 equivalents could be released at a temperature of 200 °C. In order to maximize the efficacy of NH3BH3, higher temperatures are needed, which also results in the release of the by-product borazine. To decrease the thermolysis temperature and suppress the volatile byproducts, various approaches have been achieved, including nano-confinement, catalysis, dispersion in ionic liquids and organic liquids, and the synthesis of derivatives (e.g., metal amidoboranes).139–142 Generally speaking, thermal decomposition of NH3BH3 requires high temperature and the reaction is relatively difficult to control. In contrast, the hydrolytic (eqn (2)) or methanolytic (eqn (3)) dehydrogenation of NH3BH3 can be conducted at room temperature with a stoichiometric hydrogen release in the presence of suitable catalysts.25,126,138 The effective gravimetric hydrogen storage capacity (GHSC) of an NH3BH3 hydrolysis system (NH3BH3·2H2O) is about 8.9 wt%, which is higher than that from NH3BH3 methanolysis (NH3BH3·4MeOH, 3.9 wt%). | NH3BH3 + 2H2O → NH4BO2 + 3H2 | (2) |
| NH3BH3 + 4MeOH → NH4B(OMe)4 + 3H2 | (3) |
In 2006, Xu's research group firstly found that a stoichiometric amount of hydrogen could be released from the hydrolysis of NH3BH3 by using noble metal-based (Pt, Ru, and Pd) catalysts.126 Since then, numerous studies have been reported on hydrogen generation from the hydrolysis of NH3BH3.143–148 Also, several reviews have been reported on the research progress on the hydrolysis of NH3BH3.21,25,127,149–153 Here, we have mainly summarized the most active noble-metal-free nanocatalysts for the hydrolytic (Table 2) or methanolytic (Table 3) dehydrogenation of NH3BH3.
Table 2 Catalytic activities for hydrogen evolution from the hydrolysis of NH3BH3 by different catalysts
Catalyst |
Temp. (K) |
n
metal/nAB |
TOF (molH2 molmetal−1 min−1) |
E
a (kJ mol−1) |
Ref. |
The reaction was promoted with the addition of NaOH.
|
In situ Fe NPs |
RT |
0.12 |
3.12 |
— |
155
|
Co/γ-Al2O3 |
RT |
0.018 |
2.27 |
62 |
154
|
In situ Co NPs |
RT |
0.04 |
44.1 |
— |
156
|
G6-OH(Co60) |
298 |
0.013 |
10 |
50.2 |
158
|
Co/PEI-GO |
298 |
0.11 |
39.9 |
28.2 |
160
|
Co/MIL-101 |
298 |
0.02 |
51.4 |
31.3 |
167
|
Co/CTF |
298 |
0.05 |
42.3 |
42.7 |
169
|
Co NCs@PCC-2a |
298 |
0.07 |
90.1 |
— |
170
|
Co@N–C-700 |
298 |
0.057 |
5.6 |
31 |
171
|
Co/NPCNW |
298 |
0.075 |
7.29 |
25.4 |
172
|
Co/HPC |
323 |
0.11 |
2.94 |
32.8 |
174
|
Co-(CeOx)0.91/NGH |
298 |
0.04 |
79.5 |
31.82 |
161
|
Co@C–N@SiO2-800 |
298 |
— |
8.4 |
36.1 |
162
|
Ni/γ-Al2O3 |
RT |
0.018 |
2.5 |
— |
154
|
Ni/C |
298 |
0.0425 |
8.8 |
28 |
175
|
Ni/SiO2 |
298 |
0.0225 |
13.2 |
34 |
180
|
Ni@MSC-30 |
RT |
0.016 |
30.7 |
— |
181
|
Ni/ZIF-8 |
RT |
0.016 |
14.2 |
— |
182
|
Ni NPs/ZIF-8a |
298 |
0.03 |
85 |
42.7 |
183
|
Ni/CNT ALD |
298 |
— |
26.2 |
32.3 |
185
|
Ni@3D-(N)GFs |
RT |
0.009 |
41.7 |
— |
186
|
NiMo/graphene |
298 |
0.05 |
66.7 |
21.8 |
188
|
Ni-CeOx/graphene |
298 |
0.08 |
68.2 |
28.9 |
194
|
Ni/PDA-CoFe2O4 |
298 |
0.017 |
7.6 |
50.8 |
178
|
Ni/Ketjenblack |
298 |
0.13 |
7.5 |
66.6 |
179
|
Cu/γ-Al2O3 |
RT |
0.018 |
0.23 |
— |
154
|
p(AMPS)-Cu |
303 |
0.069 |
0.72 |
48.8 |
197
|
Zeolite confined Cu |
298 |
0.013 |
1.25 |
51.8 |
201
|
Cu/CoFe2O4@SiO2 |
298 |
0.0031 |
40 |
— |
202
|
Cu/RGO |
298 |
0.1 |
3.61 |
38.2 |
203
|
Cu@SiO2 |
298 |
0.08 |
3.24 |
36 |
204
|
Fe0.5Ni0.5 alloy |
293 |
0.12 |
11.1 |
— |
210
|
Fe0.3Co0.7 alloy |
293 |
0.12 |
13.9 |
16.3 |
216
|
Cu0.33Fe0.67 |
298 |
0.04 |
13.95 |
43.2 |
219
|
CuCo/graphene |
293 |
0.02 |
9.18 |
— |
220
|
Cu0.2Co0.8/PDA-rGO |
303 |
0.05 |
51.5 |
54.9 |
221
|
Cu0.2Co0.8/PDA-HNTs |
298 |
0.09 |
30.8 |
35.15 |
222
|
CuCo/C |
298 |
0.033 |
45 |
51.9 |
223
|
Cu0.5Co0.5@SiO2 |
298 |
0.08 |
4.26 |
24 |
230
|
Cu0.3Co0.7@MIL-101 |
RT |
0.034 |
19.6 |
— |
231
|
Cu0.72Co0.18Mo0.1 |
298 |
0.04 |
46.0 |
45 |
190
|
Cu0.72Co0.18Mo0.1a |
298 |
0.04 |
119.0 |
— |
190
|
CuNi/CMK-1 |
298 |
0.072 |
54.8 |
— |
224
|
Cu0.2Ni0.8/MCM-41 |
298 |
0.05 |
10.7 |
38 |
228
|
CuNi/47-SiO2 |
298 |
0.165 |
23.5 |
34.2 |
229
|
Cu0.8Co0.2O/GO |
298 |
0.024 |
70 |
45.5 |
243
|
Ni2P |
298 |
0.12 |
40.4 |
44.6 |
255
|
CoPa |
298 |
0.043 |
72.2 |
46.7 |
262
|
Ni0.7Co1.3P/GOa |
298 |
0.026 |
109.4 |
— |
263
|
Table 3 Catalytic activities for hydrogen evolution from the methanolysis of NH3BH3 by different catalysts
Catalyst |
Temp. (K) |
n
metal/nAB |
TOF (molH2 molmetal−1 min−1) |
E
a (kJ mol−1) |
Ref. |
Cu2O |
298 |
0.15 |
0.16 |
— |
198
|
Nano-Cu@Cu2O |
298 |
0.15 |
0.12 |
— |
198
|
Nano-Cu |
298 |
0.15 |
0.08 |
— |
198
|
PVP-stabilized Ni |
298 |
0.005 |
12.1 |
62 |
267
|
Co–Ni–B |
RT |
0.2 |
10 |
— |
276
|
Co–Co2B |
RT |
0.2 |
6 |
— |
276
|
Ni-Ni3B |
RT |
0.2 |
3.6 |
— |
276
|
Flower-like Cu |
298 |
0.15 |
2.41 |
34.2 |
277
|
Cu–Cu2O–CuO/C |
298 |
0.04 |
24 |
67.9 |
278
|
b-CuO NA/CF |
298 |
0.018 |
13.3 |
34.7 |
279
|
CuNi/graphene |
298 |
0.03 |
49.1 |
24.4 |
280
|
Cu/Co(OH)2 |
298 |
0.129 |
61.63 |
37.6 |
281
|
3.1. Monometallic catalysts
Xu's research group reported that non-noble-metals Co, Ni and Cu supported on different supports (Al2O3, SiO2 and C) were catalytically active, whereas supported Fe was inactive in hydrolytic dehydrogenation of NH3BH3 at room temperature.154 Unexpectedly, amorphous Fe NPs synthesized by in situ reduction (Fe/NH3BH3 = 0.12) with NH3BH3 and NaBH4 exhibited noble metal-like catalytic activity in the hydrolysis of AB (Fig. 1).155 The high activity of the amorphous metal NPs could be attributed to their amorphous structure, which has a much greater structural distortion and therefore a much higher concentration of active sites for the reaction than its crystalline counterpart. Then, the amorphous Co and Ni NPs were also found to exhibit enhanced catalytic performance in comparison with their crystalline counterparts.156,157 In particular, the amorphous Co NPs showed the highest catalytic activity with a TOF of 44.1 min−1 for hydrogen production from the hydrolysis of NH3BH3, which is the highest among the non-noble metal catalysts ever reported.156
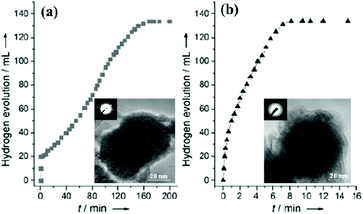 |
| Fig. 1 Hydrogen generation by hydrolysis of aqueous AB (0.16 m, 10 mL) in the presence of (a) the pre-synthesized and (b) in situ synthesized Fe catalysts (Fe/AB = 0.12) at room temperature under argon. The inset shows the corresponding TEM images and SAED patterns of the as-synthesized catalysts. Reprinted with permission from ref. 155. Copyright (2008) Wiley-VCH. | |
Co-Based catalysts have been widely studied in recent years due to their relatively higher catalytic activity and low cost.158–165 Xu's research group synthesized dendrimer-encapsulated Co NPs (G6-OH(Co60)) through the complexation of Co2+ cations with the internal tertiary amine of sixth-generation hydroxyl-terminated poly(amidoamine) dendrimers followed by reduction with NH3BH3 and NaBH4.158 The synthesized (G6-OH(Co60)) was highly dispersed (1.6 nm) and active for the hydrolysis of NH3BH3. Duan et al. constructed a Co@g-C3N4 core–shell nanostructure anchored onto the surface of rGO sheets (Co@g-C3N4-rGO), where g-C3N4 shells could protect Co cores from aggregating or leaching and rGO sheets could strengthen the magnetic momentum transfer of Co NPs from the external magnetic field.159 The self-stirring mode in the batch reactor and the magnetic fixing, stirring, and separating mode in the continuous-flow slurry-bed reactor provided an excellent catalytic process with Co@g-C3N4-rGO as the pre-catalyst. To further improve the activity of Co-based catalysts for NH3BH3 hydrolysis, post-modified supports have been used. Lu and coworkers reported well-dispersed Co NPs with an average size of 2.6 nm on PEI-decorated GO, and the resultant Co/PEI-GO nanocomposites showed excellent activity (39.9 min−1) due to the chelation effect of PEI with precursor metal ions.160
Due to their tunable porous structures, metal–organic frameworks (MOFs) have been used as supports to control the sizes of metal NPs.166–168 Gu and coworkers reported amorphous Co NPs with small particle size (1.6–2.6 nm) immobilized by MIL-101 through an ultrasound-assisted in situ method. The obtained Co/MIL-101 pre-catalyst showed excellent catalytic activity (51.4 min−1) for hydrogen evolution from the hydrolysis of NH3BH3.167 Chen and coworkers adopted a covalent triazine framework (CTF) as a support with the merits of high surface area, a well-defined porous structure and high nitrogen content (∼17%).169 Specifically, the Co/CTF with 3 wt% Co loading showed the highest activity towards NH3BH3 hydrolysis, providing a TOF value of 42.3 min−1 at room temperature. Zhou and coworkers developed a new type of metal–organic hybrid material, the porous coordination cage (PCC), which is efficient at stabilizing Co nanoclusters.170 The obtained Co@PCC-2a exhibited extraordinary catalytic activity (90.1 min−1) in the hydrolysis of NH3BH3 (Fig. 2).
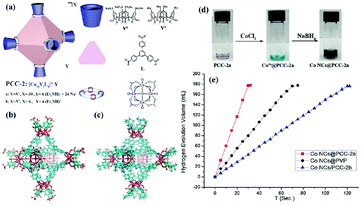 |
| Fig. 2 (a) Cartoon of octahedron cage PCC-2 and the cage components, (b) the crystal structure of PCC-2, and (c) the crystal structure of PCC-2b. (d) The preparation of Co NCs with PCC-2a. (e) Time course plots of H2 generation for the hydrolysis of AB by Co NCs@PCC-2a, Co NCs/PCC-2b, and Co NCs@PVP. Reprinted with permission from ref. 170. Copyright (2018) Wiley-VCH. | |
Recently, carbonization of inorganic/organic composites has been developed to prepared catalysts for the AB hydrolysis reaction due to their excellent chemical and mechanical stabilities, large specific surface areas and various functionalities.163,171–174 Chen and coworkers reported the preparation of Co@N–C pre-catalysts through one-step thermolysis of Co (salen, N,N′-bis(salicylidene)ethylenediamine) at selected temperatures (600–800 °C) under an Ar atmosphere.171 They found that Co@N–C obtained at 700 °C (Co@N–C-700) shows superior catalytic performance (5.6 min−1) and high sustainability among the synthesized pre-catalysts. After that, they also synthesized Co NPs (∼3.5 nm) supported on nitrogen-doped porous carbon nanowires (Co/NPCNW) by carbonization of a Co–metal organic framework (Co-MOF), which show a TOF of 7.29 min−1 and a relatively low activation energy of 25.4 kJ mol−1.172 Later on, Zhang et al. reported a series of highly active and stable nitrogen-doped mesoporous carbon embedded-Co NP pre-catalysts through a thermolysis and etching combined strategy.173 More recently, Yang and coworkers employed a selective atom evaporation-isolation strategy with bimetal Co/Zn-MOF-74 as a sacrificial template to obtain a metallic Co/HPC pre-catalyst by direct carbonization and in situ reduction under an Ar atmosphere.174 The as-synthesized Co/HPC pre-catalyst exerted higher catalytic activity and showed excellent reusability as compared to the Co/HPC derived from pure Co-MOF-74. The excellent catalytic performance can be attributed to the uniform dispersion and small particle size of metallic Co obtained under the assistance of the dopant Zn.
Ni catalysts are also widely studied as low cost non-noble metal catalysts owing to their exceptional activity in catalytic dehydrogenation of aqueous AB (Table 2).175–179 Monodisperse Ni NPs (3.2 nm) supported on Ketjen carbon black (Ni/C) showed high catalytic activity for the hydrolysis of AB with a TOF of 8.8 min−1.175 However, Ni/C was not stable during the reusability test due to the agglomeration of Ni NPs on the carbon support. In order to improve the stability of Ni NPs, they used various oxides including SiO2, Al2O3, and CeO2 as support materials.180 They found that 3.2 nm Ni NPs supported on SiO2 showed the highest activity (13.2 min−1) and durability. Subsequently, the Ni NPs (6.3 ± 1.7 nm) deposited into the nanoporous carbon (MSC-30) synthesized by Xu's research group showed excellent catalytic activity with a TOF value as high as 30.7 min−1, which is the highest one among all of the Ni catalysts ever reported for this hydrolysis reaction at room temperature.181 They also reported the synthesis of highly dispersed Ni NPs immobilized on the zeolitic metal–organic framework ZIF-8 (Ni/ZIF-8), which showed high catalytic activity and durability.182 They pointed out that this is the first example of water-stable MOF-supported metal NPs for hydrogen generation from hydrolysis of NH3BH3. Using the zeolitic metal–organic framework ZIF-8 as the support, Astruc and coworkers further developed highly dispersed Fe, Co, Ni and Cu NPs, and the highest catalytic performance with a TOF value of 85 min−1 was achieved in the presence of Ni/ZIF-8 with the assistance of an additive (NaOH).183
Zhong and coworkers reported a Ni/CNT hybrid pre-catalyst for the hydrolysis of NH3BH3.184 Combined with identical high-resolution TEM images, scanning transmission X-ray microscopy (STXM) clearly revealed the existence of a strong interaction between Ni NPs and thin CNTs (C–O–Ni bonds), which favored the tunable electronic structure of Ni NPs and facilitated the catalytic hydrolysis process. Later on, Qin and coworkers presented a facile atomic layer deposition approach (ALD) to synthesize highly dispersed Ni NPs on CNTs.185 The resultant Ni/CNT pre-catalyst produced with 200 ALD cycles showed the best catalytic activity (26.2 min−1) among all the Ni/CNT pre-catalysts. Mahyari et al. employed a three-dimensional nitrogen-doped graphene-based framework (3D-(N)GF) as a support to immobilized Ni NPs, which showed excellent catalytic activity (41.7 min−1) and high stability in NH3BH3 hydrolysis.186
Recently, our group reported a facile chemical reduction method to incorporate a small amount of inactive metals (Mo, Cr, and W) into Ni NPs.187 The characterization results revealed that the incorporation of Mo (mainly in the oxidation state) can not only reduce the metal particle size and crystallinity of the metal NPs, but also increase the electron density on the metal surface, which can effectively improve the catalytic activity of the catalysts. To further improve the dispersion and activity of the catalyst, Ni NPs modified with a Mo dopant have been synthesized on graphene (Ni–Mo/graphene), and they exhibit a high Pt-like catalytic activity (66.7 min−1) and robust durability in the hydrolysis of NH3BH3 at room temperature (Fig. 3).188 In addition, our synthesis is not limited only to Ni–M (M = Mo, Cr, W), but can also be extended to other transition metal systems (Cu–M, Co–W, etc.), providing a general method for the synthesis of highly efficient catalysts for the hydrogen evolution reaction.189–193
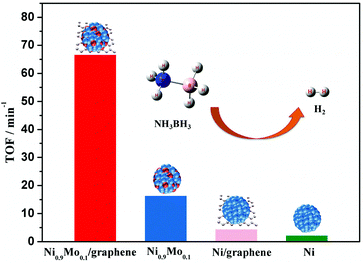 |
| Fig. 3 Catalytic activities for hydrogen generation from AB aqueous solution (5 mL, 0.2 M) over Ni0.9Mo0.1/graphene, Ni0.9Mo0.1, Ni/graphene, and Ni at 298 K (metal/AB = 0.05). Reprinted with permission from ref. 188. Copyright (2016) Royal Society of Chemistry. | |
Ni doped with CeOx and supported on graphene (Ni-CeOx/graphene) was also fabricated by our research group via a facile chemical reduction route.194 The combination of CeOx, metal, and graphene of the Ni-CeOx/graphene pre-catalyst confers remarkably enhanced catalytic activity (68.2 min−1) for the hydrolytic dehydrogenation of NH3BH3 (Fig. 4), as well as high stability. In addition, some other rare-earth metal oxide (ReOx, Re = La, Dy, Er, Yb, Gd, and Tb) doped Ni/graphene (Ni-ReOx/graphene) were also prepared and all showed higher catalytic activities than pure Ni/graphene. The excellent catalytic activities of Ni-ReOx/graphene might be attributed to the similar physical and chemical properties of the ReOx species, all of which promote the active sites of Ni to electron-rich states that are beneficial for the catalytic reaction. By using a similar approach, Co-CeOx/NGH and CuNi-CeOx/rGO were prepared and they showed synergistic and superior catalytic activities.161,195
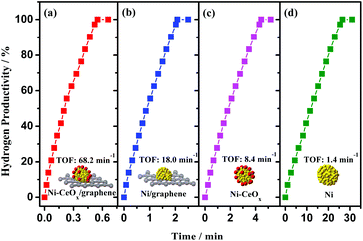 |
| Fig. 4 Hydrogen productivity vs. reaction time for hydrogen release from an aqueous AB solution (200 mM, 5 mL) catalyzed by (a) Ni-CeOx/graphene, (b) Ni/graphene, (c) Ni-CeOx, and (d) Ni at 298 K (nNi/nAB = 0.08). Reprinted with the permission from ref. 194. Copyright (2018) Springer Nature. | |
Comparing Co- and Ni-based catalysts, Cu-based catalysts have been reported to show a lower catalytic activity (Table 2).196,197 In 2006, Xu and coworkers found that Cu/γ-Al2O3 was catalytically active in NH3BH3 hydrolysis (0.78 min−1), but it takes nearly 600 minutes to release 2.83 equivalents of hydrogen (nCu/nAB = 0.018).154 Subsequently, nanostructured Cu, Cu2O, and Cu@Cu2O NPs were synthesized by Kalidindi and co-workers via the solvated metal atom dispersion (SMAD) method.198 They found that core–shell Cu@Cu2O and Cu2O NPs are more active than pure Cu NPs for this hydrolysis reaction. After that, Fukuzumi and coworker synthesized a series of Cu/Co3O4 composite pre-catalysts by a conventional impregnation method.199 The catalytic activities of Cu/Co3O4 were significantly dependent on the shape and size of nanosized Co3O4, and it was found that Co3O4 with a hexagonal sheet shape showed better catalytic activity than that with a cube or uncontrolled shape. They also found that capping of Cu2O with Co3O4 NPs was an effective way to suppress agglomerate formation, which made the Cu2O–Co3O4 composites exhibit high catalytic reactivity in hydrolytic dehydrogenation of NH3BH3.200
Özkar and co-worker synthesized zeolite confined Cu NPs by ion-exchange of Cu2+ with the extra framework Na+ in zeolite-Y followed by the reduction of the Cu2+ ions with NaBH4.201 The zeolite confined Cu NPs were found to be active in the hydrolysis of NH3BH3 with a TOF value of 0.78 min−1. Cu NPs supported on magnetic SiO2/CoFe2O4 (CuNPs@SCF) were reported to have an initial TOF of 40 min−1 for hydrolysis of NH3BH3 at 298 K.202 The authors claimed that this TOF value was higher than those of all the reported non-noble metal catalysts for the same hydrolysis reaction. It should be noted that the support of CoFe2O4 was not considered in the TOF calculation. The oxides of Fe and Co are difficult to be reduced by NH3BH3; however, they might be reduced by the Cu–H active species or hydrogen released in the hydrolysis reaction.
Our group reported the synthesis of reduced graphene oxide supported Cu NPs (Cu/RGO) via a facile in situ procedure using NH3BH3 as a reductant.203 The obtained Cu/RGO showed a higher catalytic activity than Cu/graphite powder, Cu/activated carbon and free Cu NPs, over which the NH3BH3 hydrolysis reaction is completed in 8.3 min, giving a TOF value of 3.61 min at 298 K. We also found that the ultrafine Cu NPs (∼2 nm) encapsulated in porous silica nanospheres can be simply fabricated via a one-pot synthetic route in a reverse micelle system.204 The outer shell of silica can effectively prevent the Cu NPs from aggregation. The Cu@SiO2 core–shell nanospheres showed superior activity as compared to Cu/commercial SiO2, Cu/SiO2 and free Cu NPs for hydrolysis of NH3BH3 (Fig. 5). Furthermore, the Cu@SiO2 core–shell nanospheres showed long-term stability, retaining 90% of their initial catalytic activity even after 10 runs. Recently, Zhang and coworkers fabricated Cu nanocrystals with different nanostructures (nanocubes, nanowires, nanotetrahedra, etc.) via simply adjusting the addition proportion of the reductant and orientation agent.205 In comparison with the ordinary Cu NPs with extremely low or hardly any catalytic performance activity, all of the regularly shaped Cu nanocrystals showed high catalytic activity, among which the nanocubed Cu nanocrystals exerted the best catalytic performance for hydrolyzing NH3BH3 with 3 equiv. of H2 extracted within 28 min at 298 K. However, the productivity of the hydrolysis reaction over a nanocubed Cu catalyst showed an obvious decrease after 5 runs.
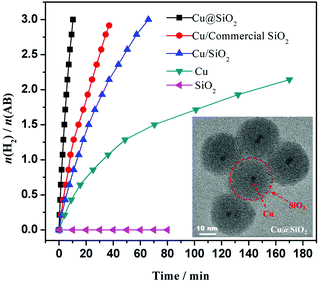 |
| Fig. 5 Hydrogen generation from the hydrolysis of AB (0.2 M, 5 mL) in the presence of different catalysts at 298 K (Cu/AB = 0.09). The inset shows the TEM image of the Cu@SiO2 catalyst. Reprinted with permission from ref. 204. Copyright (2014) Springer Nature. | |
3.2. Heterometallic catalysts
Heterometallic catalysts, including bi-metallic and multi-metallic catalysts, with unique structures may show higher catalytic performance than their monometallic counterparts due to the strong synergistic effects/electronic effects between the metals.23,206–209 A number of heterometallic catalysts, such as Fe–Ni,210–213 Fe–Co,214–216 Ni–Co,217,218 Cu–Fe,219 Cu–Co,220–223 and Cu–Ni,224–228 have been reported toward the hydrolysis of NH3BH3. For example, our group prepared a series of Cu1−xFex alloy NPs by a very simple in situ reduction method, and the optimal Cu0.33Fe0.67 alloy NPs exhibited superior catalytic activity with a TOF value of 13.95 min−1 (Fig. 6).219 Moreover, these in situ synthesized CuFe alloy NPs can be easily separated from the solution with an external magnet, and they showed good recycling stability.
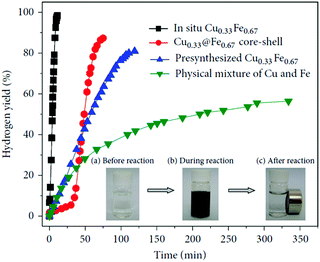 |
| Fig. 6 Hydrogen generation from the hydrolysis of AB in the presence of different catalysts (metal/AB = 0.04). The inset shows photographs of the catalytic hydrolysis of AB via in situ synthesized Cu0.33Fe0.67 alloys. Reprinted with permission from Ref. 219. Copyright (2013) Elsevier. | |
In subsequent research, various distinct support materials, such as carbon materials, nanotubes, nanofibers, silica spheres, metal–organic frameworks (MOFs), and so on, have been used to fabricate well-dispersed and stable bimetallic NP nanocatalysts.220–224,229–231 Co decorated Cu NPs on graphene (graphene–CuCo) have been synthesized by Yan et al. via a one-step self-catalytic method, which showed high efficiency toward the hydrolytic dehydrogenation of NH3BH3.220 Xu's research group employed diamine-functionalized reduced graphene oxide as a support for CuCo NPs (CuCo/PDA-rGO), which showed higher catalytic performance (41 min−1) as compared to CuCo/rGO (20.6 min−1).221 Later on, Zhang and coworkers prepared poly(diallyldimethylammonium chloride) functionalized halloysite nanotube supported Cu–Co NPs (CuCo/PDDA-HNTs), in which CuCo NPs were highly dispersed on the surface of halloysite nanotubes and they showed an extraordinary catalytic property (30.8 min−1) in NH3BH3 hydrolysis reaction.222 Zahmakiran and coworkers reported copper–cobalt alloy NPs supported on activated carbon (CuCo/C) by a surfactant-free deposition–reduction technique. The obtained CuCo/C showed excellent catalytic performance with an initial TOF of 45 min−1.223
Kleitz and coworkers selected mesoporous carbons (CMK-1 and MCNS) and mesoporous silica (MCM-48) as supports for CuNi alloy NPs.224 The catalytic performances of carbon-supported Cu0.5Ni0.5/CMK-1 and Cu0.5Ni0.5/MCNS are comparable in the hydrolysis of NH3BH3 and are 3-fold higher than that of silica-supported Cu0.5Ni0.5/MCM-48. Yu and coworkers successfully immobilized CuNi NPs on six differently sized SiO2 spheres (47, 97, 195, 333, 391 and 485 nm).229 The results showed that the catalytic activity of CuNi/SiO2 increases with the decrease of SiO2 particle size, due to which the CuNi NPs supported on the smallest SiO2 (CuNi/47-SiO2) exhibit the highest catalytic activity in the hydrolysis of NH3BH3 at 298 K. However, the stability of CuNi/47-SiO2 showed obvious degradation after seven cycles. Our group successfully encapsulated ultrafine Cu–Co NPs (∼2 nm) inside SiO2 nanospheres (CuCo@SiO2) (Fig. 7), which can effectively prevent metal NP aggregation and enhance the pre-catalyst stability.230 The optimized Cu0.5Co0.5@SiO2 pre-catalyst showed excellent catalytic activity in the hydrolysis of NH3BH3 and preserved 93% of its initial catalytic activity even after 10 runs. CuCo alloy NPs confined in the pores of MIL-101 (CuCo@MIL-101) via a double-solvent method combined with the excellent reduction approach also showed high activity and long durability for hydrolysis of NH3BH3.231
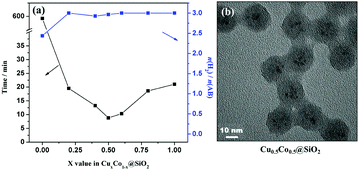 |
| Fig. 7 (a) CuxCo1−x@SiO2 core–shell nanospheres with different x values under an ambient atmosphere at 298 K; (b) TEM image of Cu0.5Co0.5@SiO2. Reprinted with permission from Ref. 209. Copyright (2015) American Chemical Society. | |
Trimetallic CuCoMo NPs without any surfactant or support have been fabricated by our group through a facile co-reduction method.190 Compared to their bi-/monometallic counterparts, the obtained optimized trimetallic Cu0.72Co0.18Mo0.1 pre-catalyst exhibits the highest catalytic activity for AB hydrolysis with a TOF value of 46.0 min−1 at 298 K (Fig. 8). Moreover, the catalytic performance of Cu0.72Co0.18Mo0.1 can be further significantly improved by introducing NaOH as a promoter, providing a TOF value as high as 119.0 min−1 at 298 K, which is among the highest values of all the reported non-noble metal catalysts (Table 2) and even higher than that of the commercial Pt/C pre-catalyst for the same reaction.126 However, introducing the NH4+ species (e.g., NH3·H2O, NH4Cl, etc.) into the reaction system is unfavorable for the hydrolysis of AB.
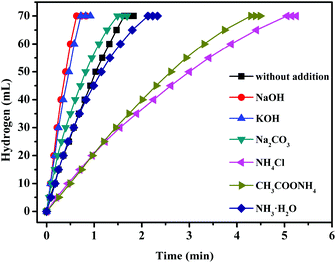 |
| Fig. 8 Hydrogen generation from the hydrolysis of AB (0.20 M, 5 mL) with the addition of 1 M NaOH, KOH, Na2CO3, NH4Cl, CH3COONH4 and NH3·H2O (2 mL) catalyzed by Cu0.72Co0.18Mo0.1 NPs at 298 K (nmetal = 0.04 mmol). Reprinted with permission from ref. 190. Copyright (2018) Royal Society of Chemistry. | |
In addition to alloy NPs, core–shell NPs have also attracted considerable attention due to their unique physical and chemical properties, and they exhibited excellent catalytic performance in hydrolytic dehydrogenation of NH3BH3.232–238 Among them, catalysts with a Cu core are the most attractive. In 2011, Xu's research group first reported the synthesis of Cu@M (M = Co, Fe, Ni) NPs via a simple one-step seeding-growth route using NH3BH3 as a weak reducing agent, which could selectively reduce Cu2+ first to form the core, and successively reduce M2+ (M = Co, Fe, Ni) to form the shell.233 A relatively stronger reductant NaBH4, instead of NH3BH3, resulted in the formation of CuM alloys. Compared to monometallic and alloy counterparts, the core–shell Cu@M (M = Co, Fe, Ni) NPs showed synergistic and superior catalytic activity for hydrogen evolution from the hydrolysis of NH3BH3. In addition, H2 generation over Cu1@Co4 NPs is the most active among that over all the Cu@M (M = Co, Fe, Ni) NPs, with which the hydrolysis reaction is completed within 10 min at room temperature. By following a similar approach, various core–shell catalysts based on Cu as a core, such as bimetallic Cu@Co,234 trimetallic Cu@FeNi,236 Cu@FeCo,237 Cu@CoNi,238,239 Cu@CoMo240 and even tetrametallic Cu@FeCoNi,225 have been synthesized and they showed higher activities than the corresponding monometallic and alloy counterparts toward the hydrolysis of ammonia borane for hydrogen production.
3.3. Other catalysts
Since non-noble metal NPs are very easy to oxidize, the direct use of non-noble metal oxides as catalysts for hydrolytic dehydrogenation of NH3BH3 has attracted much attention in recent years.241–244 Li and coworkers prepared CuCo2O4 pre-catalysts with different shapes (nanoplates, nanosheets, and nanoparticles).241 Among them, the nanoplate-shaped CuCo2O4 pre-catalyst showed the best catalytic activity for hydrogen production from the hydrolysis of NH3BH3, giving a TOF value of 44 min−1 at room temperature. Moreover, the CuCo2O4 nanoplate pre-catalyst almost retained its initial catalytic activity after eight cycles, indicative of its excellent durability and stability. Later on, they developed a series of non-noble metal oxides such as NiCo2O4,242 MnCo2O4,245 CuCoMoO4,246 CuNiCo2O4,247 Co3O4/CuMoO4,248 CuO–NiO/Co3O4249 and CoxCu1−xCo2O4@ CoyCu1−yCo2O4,250 which are active in NH3BH3 hydrolysis reaction. Zhong and coworker reported the synthesis of bimetallic oxide NPs CuxCo1−xO deposited on grapheme oxide (GO) via a facile route.243 The obtained Cu0.8Co0.2O/GO pre-catalyst exhibits an initial TOF value as high as 70.0 min−1 for the hydrolysis of NH3BH3, owing to the interfacial interaction between CuCoO NPs and GO. Moreover, they performed the first in situ XAS experiments to study the electronic structure changes of the pre-catalyst during hydrolysis. Recently, Liu and coworkers reported a graphene oxide supported Cu@CuCoOx pre-catalyst, with which superior catalytic activity was achieved toward the hydrolysis of NH3BH3 with a TOF of 44.6 min−1 at room temperature.244 With a metal oxide as a pre-catalyst, the catalytically active component of the catalyst is the zero-valent metal because a part of the metal oxide can be reduced by NH3BH3. Therefore, such metal oxide catalysts are mainly Cu-based oxides, because Cu oxides can be easily reduced to Cu(0) by NH3BH3 at room temperature.
Non-noble-metal catalysts are also easily prone to aggregation in air and under the catalytic reaction conditions, resulting in loss or deactivation of catalytic activity. Recent studies have shown that the catalytic activity and stability of non-noble-metal catalysts could be improved by the introduction of metalloid elements (e.g. B and P).60,61,116,251–257 A series of Co–B catalysts have been reported to show high catalytic activity and durability for hydrogen generation from NH3BH3.251,252,258–261 For example, Figen and Coşkuner synthesized a Co–B pre-catalyst by the sol–gel reaction of boron oxide with cobalt chloride hexahydrate in the presence of citric acid.252 The obtained amorphous Co–B pre-catalyst showed higher catalytic activity than a crystalline Co–B catalyst due to its high specific surface area and it retained 90% of its initial activity after 4 runs. They also found that Co–B supported on mesoporous silica (SBA-15) and a Co–B thin film pre-catalyst deposited by Pulsed Laser Deposition (PLD) were able to decrease the activation energy, thus improving the catalytic activity in NH3BH3 hydrolysis.258,259
Fu and coworkers reported the synthesis of Ni2P NPs by reacting Ni(OH)2 with solid NaH2PO2 in argon at 543 K.254 The obtained Ni2P NPs (<12 nm) showed excellent catalytic activity and high sustainability toward hydrogen evolution from the hydrolysis of NH3BH3 with an initial TOF of 40.4 min−1 at 298 K. In addition, they investigated the reaction mechanism via DFT calculations (Fig. 9), suggesting that the combination of the Ni2P surface and substrate molecules significantly enhances the hydrolytic dehydrogenation activity by reducing the reaction barrier, making it easy to overcome at room temperature. They also found that the introduction of anions (OH−, F−, and Cl−) during NH3BH3 hydrolysis could significantly promote the catalytic activity in NH3BH3 hydrolysis reaction over a Co–P pre-catalyst at room temperature.262 Moreover, the effect of OH− was higher than that of F− and Cl− on the TOF, and a high TOF of 72.2 min−1 was achieved at a CoP/NH3BH3 molar ratio of 0.041 at 298 K. Recently, a series of Co-doped Ni2P NPs and their nanohybrid with graphene oxide were also fabricated by Fu and coworkers.263 The optimal Ni0.7Co1.3P/GO pre-catalyst shows a high initial TOF of up to 109.4 min−1 in the presence of NaOH at 298 K. The incorporation of Co into Ni2P can effectively optimize the electronic structures of Ni2−xCoxP pre-catalysts, enhance their interaction with NH3BH3, and facilitate the hydroxyl activation of NH3BH3, thus resulting in a decrease of the reaction energy barrier and improvement of the hydrogen generation rate.
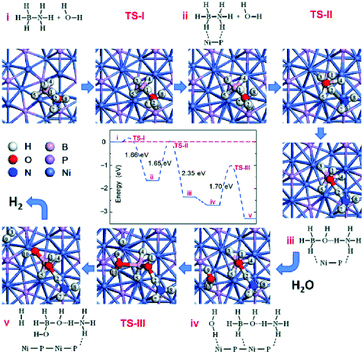 |
| Fig. 9 Plot of energy changes versus reaction coordinates calculated for Ni2P-catalyzed hydrolysis of AB. Reprinted with permission from ref. 254. Copyright (2016) Wiley-VCH. | |
For the transition metal catalyzed hydrolysis of NH3BH3, some plausible mechanisms have been proposed. In 2006, Xu's research group suggested that the formation of an activated complex species between a metal surface and NH3BH3 molecules is the rate-determining step.154 Then, the activated complex species is attacked by H2O, leading to concerted dissociation of the B–N bond and hydrolysis of the resulting BH3 intermediate to produce the borate ion along with H2 release. Jagirdar and Chen assumed that the release of H2 is due to the attack of H2O on a transient M–H.198,264 Fu et al. proposed an almost self-powered process based on DFT calculations, which involves the formation of BH3OH− and NH4+ species, and then attacking adjacent H2O to produce H2.254 Recently, He and Duan proposed that the rate-determining step for NH3BH3 is the breaking of the O–H bond in H2O based on the kinetic isotopic effect measurements.169,265
3.4. Catalysts for NH3BH3 methanolysis
As for NH3BH3 methanolysis, despite the GHSC of the NH3BH3–4MeOH system (3.9 wt%) being much lower than that from the hydrolysis system, NH3BH3 methanolysis has some advantages that merit its potential applications as a portable hydrogen source.25,138,266–273 Firstly, addition of suitable catalysts can initiate the NH3BH3 methanolysis reaction below 0 °C, thereby satisfying the applications in cold weather. Secondly, pure H2 can be released by methanolysis of NH3BH3 without the production of NH3. More importantly, the byproduct of NH3BH3 methanolysis (i.e., NH4B(OMe)4) can be easily reconverted back to NH3BH3 by reaction with NH4Cl and LiAlH4 at room temperature.138
Compared with the extensive studies of NH3BH3 hydrolysis, there are only a few studies regarding NH3BH3 methanolysis (Table 3).138,267,274–276 In 2007, RuCl3, RhCl3, PdCl2, CoCl2, NiCl2, Pd/C, and RANEY® Ni were firstly reported for the methanolysis of NH3BH3.138 Since then, non-noble metal pre-catalysts such as PVP-stabilized Ni, Co-Co2B, Ni-Ni3B, Co–Ni–B, and Co–Ni–P pre-catalysts have been reported for dehydrogenation of NH3BH3 in methanol.276 Later on, mesoporous Cu with diverse morphologies (flower-, nanosheet-, bundle- and dandelion-like) have been prepared by our group via a simple wet-chemical method combined with a reduction strategy.277 Among the four Cu nanostructures, the flower-like Cu nanostructure was the most active with a TOF of 2.41 min−1 toward the methanolysis of NH3BH3. Moreover, the flower-like Cu nanostructures also showed excellent recyclability in the methanolysis of NH3BH3. Subsequently, Zahmakiran and coworkers reported Cu–Cu2O–CuO/C composites to catalyze hydrogen generation from the methanolysis of NH3BH3, which showed high activity (24 min−1) in this methanolysis reaction.278 After that, bunch-like copper oxide nanowire arrays on copper foam (b-CuO NA/CF) were prepared via a simple oxidation process and they behaved as efficient pre-catalysts (13.3 min−1) for methanolytic dehydrogenation of NH3BH3.279 Recently, Sun's research group found that CuNi alloy NPs deposited on graphene (CuNi/G) showed excellent catalytic activity (49.1 min−1) and recyclability toward the methanolysis of NH3BH3.280 More recently, a noble-metal-free Cu/Co(OH)2 nanohybrid pre-catalyst was fabricated by Chen and coworkers via a feasible in situ method.281 By varying the metal/support ratio, a highly efficient catalytic performance (61.63 min−1) for the methanolysis of NH3BH3 and long-term stability at ambient temperature were observed. By DFT calculations, they revealed the role of charge transfer in promoting the methanolysis reactions and the metal/support ratio in manipulating the catalytic activity via tuning electrostatic interactions.
In short, tremendous progress has been achieved in catalytic dehydrogenation of NH3BH3 by noble-metal-free catalysts (Table 2). However, further efforts are still needed to develop efficient and stable catalysts or some new methods (e.g. photocatalytic assisted technology) to promote the hydrolysis of NH3BH3. It is worth noting that the convenience of conducting AB hydrolysis reaction enables its use not only in chemical storage/hydrogen production, but also as a model reaction (similar to the CO oxidation reaction) for testing the activity of new catalysts, which will attract the attention of more and more researchers.
4. Ammonia
Ammonia is a second largest chemical in the world (after sulphuric acid) with over 170 megatonne per year being synthesized via the Haber–Bosch process, bulk of which is used as fertilizers.282 Ammonia is also considered as a potential hydrogen carrier because of its high gravimetric capacity (17.7 wt% H), low price, and production of inherently COx-free H2.24,26,283–286 In particular, it can be readily liquefied under mild conditions (−33.4 °C at 1 atm or 20 °C at 8.46 atm), which makes its storage and delivery relatively easy.287–289 NH3 can be decomposed to release H2 along with N2 by the following reaction (eqn (4)):
The NH3 decomposition is an endothermic reaction (ΔH = 92 kJ mol−1) and a high temperature is required for efficient hydrogen generation.290 Thermal decomposition of NH3 into H2 and N2 has been extensively studied in the past decades.24,26,284,286 The works before the 1990s on NH3 decomposition were mainly studied to gain insight into the reaction kinetics of NH3 synthesis.291 Nowadays, the research on NH3 decomposition is mainly focused on the generation of high quality H2. Generally, N2 adsorption is thought to be rate-limiting in NH3 synthesis, while the rate determining step for NH3 decomposition varies. For noble metal (e.g. Ru, Rh, Ir, Pt, or Pd) catalysts, N–H cleavage is the rate-determining step, while, for non-noble metal (e.g. Fe, Co, Ni, etc.) catalysts, N2 desorption is the rate-determining step.24,26,292,293
Up to now, Ru-based catalysts are known to be the most active in catalytic decomposition of NH3.294–298 However, the high cost and limited availability inhibit the wide scale use of these catalysts. Therefore, much attention has been recently been paid to non-noble metal catalysts, such as Fe, Co, and Ni as well as a series of bimetallic systems, metal carbides, and metal nitrides.24
4.1. Monometallic catalysts
Fe-Based catalysts have been extensively studied in the NH3 decomposition reaction owing to its industrial use in the NH3 synthesis reaction.299–304 Fe promoted by K2O, CaO, SiO2 and Al2O3 is active for NH3 synthesis at temperatures above 400 °C and was initially considered as a potential pre-catalyst for NH3 decomposition.299 The overall activity of Fe-based pre-catalysts toward NH3 decomposition is relatively low. Great efforts have been made to enhance the catalytic performances of Fe-based catalysts by depositing Fe onto supports such as carbon nanotubes, zeolites, and metal oxides, since their properties such as reducibility, particle size and dispersion, thermal stability and electronic structures would be improved after Fe particles are supported.301–303,305 For example, Duan et al. synthesized a novel Fe-CNF/mica pre-catalyst via a catalytic CVD method and it showed excellent catalytic activity for NH3 decomposition.305 They also found that the size and shape of Fe particles on the top of CNFs depended on the Fe particle reconstruction and CNF morphology. In addition, the pre-catalyst of Fe–CNFs/mica showed a higher catalytic activity and stability than the Fe/CNF pre-catalyst, which could be attributed to the Fe particles isolated by mica and CNFs and the higher degree of graphitization of CNFs. Recently, a series of Fe-based catalysts supported on two-dimensional mica nanosheets (Fe/MS) were synthesized by Yuan and coworkers via three different methods namely homogeneous precipitation (HP), impregnation (IM), and deposition precipitation (DP) methods.306 The catalytic results show that the Fe/MS prepared by the HP method showed the highest catalytic performance among all the synthesized pre-catalysts with different methods. The excellent catalytic performance of the Fe/MS-HP pre-catalyst is attributed to the highly dispersed Fe species, layered structure of mica, and strong metal–support interactions between Fe and mica.
High temperatures easily lead to Fe sintering, but that can be prevented by confining Fe in porous materials or forming a core–shell structure.307–309 Highly dispersed γ-Fe2O3 NPs (∼6 nm) confined within the porous systems of CMK-5 carbons and a carbon-SBA-15 composite were synthesized by Lu and coworker via a facile wet impregnation method.307 The obtained γ-Fe2O3/CMK-5 pre-catalyst showed the high catalytic activity toward the decomposition of NH3 at 600 °C. The Fe2O3/carbon-SBA-15 pre-catalysts were much more stable over a long reaction time. The excellent catalytic performance is attributed to the space limitation in the pores and the strong interaction with the composite support, thus preventing the migration and subsequent sintering of nanoparticles. The group of Ji and Au has embedded Fe NPs in microporous and mesoporous silica shells via a sonication-assisted Stöber process.308 The core–shell structure of Fe NPs showed higher catalytic activity and more stability than that of naked Fe NPs owing to the stable silica shells that effectively prevent aggregation of Fe NPs. Recently, Varisli et al. synthesized a robust Fe@mesoporous carbon pre-catalyst by a traditional impregnation procedure, which was very active for microwave-assisted NH3 decomposition.309
Ni-Based catalysts have also been used as alternative catalysts for NH3 decomposition due to their substantially lower costs compared to Ru and the higher activity compared to Fe.310–314 Previously, Ganley et al. reported an order of activity as Ru > Ni > Rh > Co > Ir > Fe for NH3 decomposition.310 Zhang et al. reported a co-precipitation method to prepare a series of supported pre-catalysts by depositing Ni NPs on Al2O3.311 The catalytic activity of Ni/Al2O3 increased with increasing Ni loading and reached a maximum at a Ni/Al ratio of 1.2. Interestingly, the conversion of NH3 was further increased after the addition of a La promoter into the Ni/Al2O3 pre-catalyst. For example, when the La/Ni molar ratio increased from 0 to 0.2, the conversion of NH3 progressively increased from 38.2 to 63.9% at 773 K. The characterization results indicated that the Ni/La–Al2O3 pre-catalyst with an appropriate amount of La possessed a more open mesoporous structure and higher dispersion of Ni as compared to Ni/Al2O3. Later on, Xu and coworker reported that the addition of CeO2 obviously improved the catalytic activity and stability of Ni/Al2O3 for NH3 decomposition to COx-free H2.315 The characterization results showed that the addition of CeO2 could enlarge the pre-catalyst pores, moderate the interaction between the metal and support, suppress Ni NPs from sintering, and improve the recombinative desorption of N adatoms from the Ni NP surface. After that, the group of Liu has also proved that adding an appropriate amount of Ce and La to Ni/SBA-15 can improve the NH3 decomposition activity, and the highest activity could be achieved when the Ce(La)/Ni ratio was around 0.3.316 Rare-earth oxides can not only act as an additive but can also be employed as an efficient support for the synthesis of high performance Ni-based catalysts. Okura and coworkers investigated the catalytic performances over Ni supported on various rare-earth oxides (Y2O3, CeO2, La2O3, Sm2O3 and Gd2O3) for NH3 decomposition.317 Among the samples investigated, the Ni/Y2O3 pre-catalyst showed the highest catalytic activity. The kinetics studies revealed that most of the rare-earth oxides could effectively alleviate the inhibition of hydrogen in the decomposition reaction. In addition, the desorption behavior of hydrogen showed that the amount of hydrogen atoms adsorbed on the Ni NP surface of Ni/Y2O3 was relatively small at high temperatures. Furthermore, this group also found that the catalytic activity of Ni/Y2O3 can be remarkably improved by modifying with a small amount of Sr or Ba species, while the addition of Mg and Ca species was not effective.318 Recently, ceria catalytic structures with a woodpile geometry of micro-channels have been prepared by 3D printing and used as a support to disperse Ni NPs.319 The obtained 3D-printed Ni/CeO2 pre-catalyst showed higher catalytic performance than the Ni/CeO2 powder pre-catalyst and the conventional cordierite honeycomb wash coated with Ni/CeO2 under the same reaction conditions.
Compared with Fe-based and Ni-based catalysts, Co-based catalysts are less studied in NH3 decomposition reactions.320,321 The roles of supports such as carbon materials and mesoporous silica and various promoters were also investigated on Co as well. Zhang et al. reported that fresh commercial carbon nanotubes (CNTs) containing residual Co or Fe NPs are highly active for NH3 decomposition, and the microstructure of the CNTs remained unchanged after the decomposition reaction.320 After that, Co supported on CNTs was synthesized and studied.321 The conversion of NH3 over Co/CNTs was 60.8%, which was higher as compared to 14.8% for Fe/CNTs and 25.4% for Ni/CNTs at 500 °C. Moreover, the effect of metal–support interactions between Co and CNTs was investigated by varying the pretreatment temperatures (230–700 °C) and gas (N2 and H2).322 It is found that the catalytic activity of Co/CNT pre-catalyst treatment in N2 was higher than that of Co/CNT in H2, and the pretreatment of Co/CNTs at 600 °C showed the highest NH3 decomposition activity and the lowest activation energy (68.6 kJ mol−1). Furthermore, Li and coworkers found that the Co@C-700-N sample calcined under limited air atmosphere showed a better activity for NH3 decomposition as compared to the calcined sample in pure N2 flow.323 Podila and coworkers have reported that Co supported on a Mg–La mixed oxide showed high activity for NH3 decomposition.324,325 Recently, Jia and coworkers synthesized a high surface area Co–SiO2 pre-catalyst by a simple two-step procedure with activated carbon as the template for NH3 decomposition reaction.326 The presence of SiO2 can effectively protect active Co species from agglomeration during the calcination and NH3 decomposition reaction. The obtained Co–SiO2 exerted superior activity to other reported catalysts.
4.2. Bimetallic catalysts
Bimetallic catalysts with unique structures may achieve excellent catalytic activity in comparison with their individual monometallic components. To date, a series of bimetallic catalysts, such as Co–Mo,327–329 Fe–Mo,330 Fe–Co,331–334 Fe–Ni335–337 and Co–Ni,338 have been prepared and employed as catalysts for NH3 decomposition. For example, Duan et al. reported that the bimetallic Co–Mo/MCM-41 pre-catalyst shows a higher activity in the NH3 decomposition reaction than a monometallic Co/MCM-41 or Mo/MCM-41 pre-catalyst under the same conditions.327 In addition, Co–Mo/MCM-41 with a Co/Mo molar ratio of 7/3 shows the highest NH3 conversion and exhibits good thermal stability. Later on, they studied the effects of Co–Mo precursors on the catalytic activity.328 The results showed that the CoMo-I/γ-Al2O3 pre-catalyst prepared using monocomponent metal amine metallate (Co(en)3MoO4) as the active phase precursor exhibited higher activity and stability for ammonia decomposition than the CoMo-II/γ-Al2O3 pre-catalyst prepared using bicomponent Co(NO3)2 and (NH4)6Mo7O24 as the active phase precursors. Moreover, they found that the textural and chemical properties of the Co–Mo pre-catalyst were significantly affected by the calcination atmosphere (i.e., Ar and Air).329 The Co–Mo pre-catalyst calcined in air showed higher catalytic activity, and Co3Mo3N is suggested as the dominant active phase of Co–Mo. In addition, FeMo-based pre-catalysts were also investigated and they were found to be active in NH3 decomposition reaction.330
Zhang et al. successfully encapsulated Fe–Co alloy NPs inside CNTs (FeCo-in-CNTs) on the basis of the capillary phenomenon in the channel of CNTs, which effectively prevented the metal NPs from aggregating even at high temperature.331 Thus, the resulting FeCo-in-CNT showed remarkable thermal stability in NH3 decomposition reaction. Recently, 2D ultrathin Co–Fe spinel oxide nanosheets confined in mesoporous silica shells (CoxFe3−xO4@mSiO2) have been fabricated by Zhang and coworkers.334 By tuning the chemical stoichiometry of CoxFe3−xO4 nanosheets, the strength of the M–N bond can reasonably adjust and subsequently greatly optimize the catalytic performance. The optimized Co0.89Fe2.11O4@mSiO2 pre-catalyst attained 88% conversion of NH3 at 600 °C and a space velocity of 60
000 cm3 g−1 h−1, even maintained for 48 h without attenuation. Similarly, Ni–Fe/Al2O3,335,336 Ni–Fe alloys337 and NiCo/Ce0.6Zr0.3Y0.1O2 solid solutions338 were found to show higher catalytic performance than the single metal pre-catalyst toward the decomposition of NH3.
4.3. Metal carbides/nitrides
Transition metal carbides (WCx, MoCx FeCx, VCx and TaCx) and nitrides (MoNx, VNx and WNx) have also been investigated for NH3 decomposition reaction.339–344 Sourabh and coworkers reported that WC can attain complete decomposition of NH3 at 550 °C; however, the WC samples need to be pretreated in a gas mixture (H2/CO) before the decomposition reaction.339 Subsequently, Shi and coworkers synthesized a mesoporous WC pre-catalyst via an impregnation–compaction route, which showed high and stable catalytic activity, and complete NH3 decomposition was achieved at a low temperature (500 °C).340 Kraupner and coworkers reported mesoporous Fe3C with high crystallinity and high surface area by a combination of a hard-templating approach and carbothermal reduction.341 The obtained Fe3C pre-catalyst showed good catalytic activity in NH3 decomposition with conversion above 95% at 700 °C. Choi et al. investigated VC and TaC as pre-catalysts for NH3 decomposition, which showed excellent catalytic performance, higher than that of a Pt/C pre-catalyst.342,343 Zheng et al. synthesized high-surface area Mo2C from a h-MoO3 precursor via a temperature-programmed reduction–carburization under a flowing atmosphere of H2 and CH4.344 The result showed that Mo2C undergoes a phase transformation during the NH3 decomposition reaction, and the active phase is actually MoN.
Mo-Based nitrides for NH3 decomposition are the most studied among the metal carbides and nitrides due to their low cost and high activity. Li et al. reported that MoNx/α-Al2O3 and NiMoNy/α-Al2O3 exhibited excellent catalytic properties for NH3 decomposition with conversions reaching as high as 98.7% and 99.8%, respectively, at 650 °C.345 Moreover, the increasing nitride phase content of Ni2Mo3N up to 37 wt% doubles the NH3 conversion at 550 °C.346 Podila et al. found that the addition of Co into γ-Mo2N can further improve the conversion of NH3 decomposition, which is due to the formation of the Co3Mo3N phase in Co-containing samples.347 After that, Srifa et al. synthesized pure phase Co3Mo3N from CoMoO4via a temperature-programmed reaction method, which showed almost 100% of NH3 conversion at 600 °C.289 The catalytic activity of the Co3Mo3N pre-catalyst for NH3 decomposition can be significantly improved by the addition of a small amount of Cs species.348 Furthermore, Mo-based nitrides such as Mo2N, Ni2Mo3N, Ni3Mo3N and Fe3Mo3N also have been suggested as the highly active species for the NH3 decomposition reaction.348–350
5. Hydrous hydrazine
Anhydrous hydrazine (N2H4), a colorless flammable liquid at room temperature, has a high hydrogen capacity as high as 12.5 wt%. Hydrogen stored in N2H4 can be catalytically decomposed over supported metals, metal carbides, and metal nitrides in two pathways (eqn (5) and eqn (6)).351–358 The reaction decomposition routes are determined by the catalysts used and the reaction conditions. However, most of the reports on hydrazine decomposition showed that ammonia is present as a product, while reports on the selective decomposition of hydrazine exclusively to hydrogen are rare, and high temperatures (>300 °C) are usually required due to the decomposition of NH3. Even worse, the anhydrous hydrazine (>98%) is highly toxic and explosive when exposed to metal catalyst surfaces, making it difficult to use it safely.
Hydrous hydrazine, such as hydrazine monohydrate (N2H4·H2O) which has a hydrogen capacity of 8.0 wt%, is believed to be relatively safe.19,20,22,359–361 Notably, N2H4·H2O is a liquid over a wide range of temperatures (213–392 K), and therefore it is easy to recharge using the current equipment for liquid fuels. Furthermore, the complete decomposition of N2H4 generates only a by-product of N2 in addition to H2, which does not need on-board collection for recycling. These merits have made N2H4·H2O a promising hydrogen carrier for storage and transportation. Thereby, it is crucial to develop effective and selective catalysts for complete hydrogen generation from N2H4·H2O.361–367 Xu and co-workers initially investigated various metal (Rh, Co, Ru, Ir, Cu, Ni, Fe, Pt and Pd) NPs as catalysts for N2H4 decomposition.368 Among all the synthesized metal NPs, Rh showed the highest H2 selectivity (43.8%) for hydrogen evolution from N2H4·H2O decomposition. Other metal NPs, such as Co, Ru, and Ir, exhibited poor H2 selectivity (7%), and Fe, Cu, Ni, Pd, and Pt were totally inactive under the same reaction conditions. Up to now, Ni,369–372 Ni–Pt,373–377 Ni–Rh,378–383 Ni–Ir,384,385 Ni–Pd,386,387 Co–Pt,388,389 Co–Ir,390 Rh–Mo,191 Rh–P,391 Ni–Fe,392–394 Ni–Cu,224,395,396 and Ni–Co397 have been reported to be efficient in the decomposition of N2H4·H2O to H2. Since noble metals (Pt, Rh, Ir, and Pd) are expensive, noble-metal-free catalysts (Ni, Fe, and Cu) were developed for the economic advantage, which is essential for promoting the potential applications of N2H4·H2O as a hydrogen storage material. Herein, we categorize noble-metal-free catalysts into three major groups: (a) Ni-based metal NPs, (b) complex oxide supported catalysts, and (c) other supported catalysts for catalytic decomposition of N2H4·H2O to H2 under various reaction conditions (Table 4).
Table 4 Catalytic activities for hydrogen evolution from N2H4·H2O catalyzed by different catalysts
Catalyst |
Temp. (K) |
n
metal/nN2H4·H2O |
H2 selectivity (%) |
TOF (molH2 molmetal−1 h−1) |
E
a (kJ mol−1) |
Ref. |
Ni0.5Fe0.5 NPs |
343 |
0.1 |
100 |
6.3 |
— |
392
|
NiFe/Cu |
343 |
0.2 |
100 |
35.3 |
44 |
398
|
Cu@Fe5Ni5 |
353 |
0.11 |
100 |
18.2 |
79.2 |
399
|
NiMoB–La(OH)3 |
323 |
0.3 |
100 |
13.3 |
55.1 |
400
|
Ni0.6Fe0.4Mo |
323 |
0.1 |
100 |
28.8 |
50.7 |
401
|
Cu0.4Ni0.6Mo |
323 |
0.2 |
100 |
38.7 |
56.6 |
402
|
Ni–Al2O3-HT |
303 |
0.4 |
93 |
2.2 |
49.3 |
403
|
RANEY® Ni |
303 |
— |
>99 |
162 |
44.4 |
369
|
Ni-0.080CeO2 |
303 |
0.45 |
99 |
51.6 |
47 |
370
|
Ni/CeO2 |
323 |
0.1 |
100 |
34.0 |
56.2 |
404
|
Ni0.5Cu0.5/CeO2 |
323 |
0.2 |
100 |
111.7 |
63.0 |
395
|
Ni0.5Cu0.5/CeO2 |
343 |
0.2 |
100 |
371.1 |
— |
395
|
Ni–CeO2@SiO2 |
343 |
0.1 |
100 |
219.5 |
59.26 |
405
|
2D Ni0.6Fe0.4/CeO2 |
323 |
0.1 |
99 |
5.76 |
44.06 |
406
|
NiFe/CeZrO2 |
343 |
0.1 |
100 |
119.2 |
50.4 |
407
|
NiFe–La(OH)3 |
343 |
0.2 |
100 |
100.6 |
57.8 |
408
|
Ni1.5Fe1.0/(MgO)3.5 |
299 |
0.21 |
99 |
10.3 |
— |
393
|
Ni0.9Fe0.1–Cr2O3 |
343 |
0.2 |
100 |
893.5 |
86.3 |
394
|
NiCo/NiO–CoOx |
298 |
0.2 |
99 |
12.8 |
45.15 |
397
|
Ni3Fe-(CeOx)0.15/rGO |
343 |
0.1 |
100 |
126.2 |
34.3 |
409
|
Ni3Fe-(CeOx)0.15/rGO |
328 |
0.1 |
100 |
56.8 |
— |
409
|
Ni nanofiber |
333 |
0.5 |
100 |
6.9 |
52.07 |
371
|
Ni–CNTs-OH |
333 |
— |
100 |
19.4 |
51.05 |
410
|
Ni@TNTs |
333 |
0.125 |
100 |
96 |
53.2 |
411
|
Ni0.5Cu0.5/MCNS |
333 |
0.28 |
100 |
21.8 |
— |
224
|
Ni10Mo/Ni–Mo–O |
323 |
0.167 |
100 |
54.5 |
— |
412
|
Ni250 NPs |
343 |
0.5 |
100 |
11.0 |
56.3 |
366
|
5.1. Metal NP catalysts
Xu and co-workers synthesized bimetallic Ni–Fe NPs by co-reduction of an aqueous solution of NiCl2 and FeSO4 in the presence of CTAB.392 Although all the synthesized Ni–Fe NPs are inactive in the decomposition reaction of N2H4·H2O at 298 K, a hydrogen selectivity of 81% can be achieved with Ni0.5Fe0.5 NPs with elevation of the reaction temperature at 343 K (Fig. 10). Furthermore, it was found that the hydrogen selectivity could be significantly enhanced by the addition of NaOH into the reaction mixture. The Ni0.5Fe0.5 NPs released gases in a stoichiometric amount (3.0 equiv.) with 100% H2 selectivity from the decomposition of N2H4·H2O in 190 min with NaOH (0.5 M) at 343 K. However, the addition of a weaker base such as NH3 and CH3COONa had no effect on the catalytic performance of the Ni–Fe NPs. The possible reason is that the highly alkaline reaction conditions make the catalyst surface highly basic, which may be unfavorable for the formation of basic NH3, therefore hindering the decomposition of N2H4 to NH3.
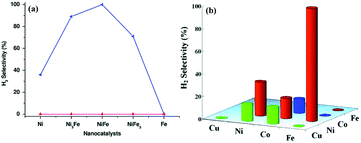 |
| Fig. 10 (a) Comparison of hydrogen selectivity over Ni, Ni3Fe, NiFe and Fe nanocatalysts (catalyst/N2H4 = 0.1) with NaOH (0.5 M) at (red) 298 K and (blue) 343 K. (b) Comparative hydrogen selectivity in the decomposition of N2H4·H2O (0.5 M) to hydrogen in the presence of different nanocatalysts. Reprinted with permission from ref. 392. Copyright (2011) American Chemical Society. | |
It has been reported that the catalytic activity and H2 selectivity of Ni–Fe NPs could be improved by introducing a Cu core.398 The Cu core is not selective for hydrogen evolution from the decomposition of N2H4·H2O, while the NiFe/Cu NPs exhibited high activity, stability, and ∼100% H2 selectivity for hydrogen evolution at 330–340 K. After that, Zhang and co-workers synthesized core–shell Cu@Fe5Ni5 NPs via an in situ seeding-growth approach and investigated their catalytic performances.399 The core–shell Cu@Fe5Ni5 NPs have a small size of about 8.5 nm. The core–shell Cu@Fe5Ni5 pre-catalyst showed high activity and 100% H2 selectivity within 70 min toward the complete decomposition of N2H4·H2O in the presence of NaOH at 343 K (Fig. 11). In contrast, for the CuFe5Ni5 alloy NPs, only 2.3 equivalents of gas (H2 selectivity = 74%) were generated even after 125 min under the same reaction conditions.
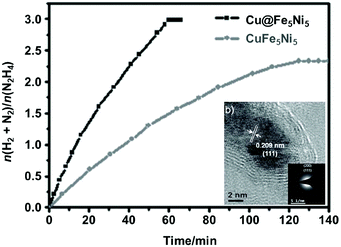 |
| Fig. 11 Time-course plots for the decomposition of N2H4H2O toward H2 over Cu@Fe5Ni5 and CuFe5Ni5NCs in the presence of NaOH at 70 °C. The inset shows an HR-TEM image of the as-synthesized Cu@Fe5Ni5 NCs. Reprinted with permission from ref. 399. Copyright (2014) Wiley-VCH. | |
Wang and coworkers found that the NiB NP pre-catalyst showed poor catalytic activity for N2H4·H2O decomposition.400 But upon incorporation of Mo and La elements into NiB NPs, the catalytic properties of the NiMoB–La(OH)3 pre-catalyst was obviously enhanced for both catalytic activity (13.3 h−1) and H2 selectivity. Later on, noble-metal-free NiFeMo NPs have been synthesized via a simple one-step synthetic route at room temperature.401 The optimized Ni0.6Fe0.4Mo NPs lead to the complete decomposition of N2H4·H2O and superior catalytic activity (28.8 h−1) within 15 min at 323 K. Mo acted as an electron donor for Ni and Fe atoms, and it has the potential to endow itself with high catalytic activity for hydrogen generation from the decomposition of N2H4·H2O. Recently, our group reported the synthesis of a CuNiMo pre-catalyst, which also showed excellent catalytic activity toward N2H4·H2O decomposition for hydrogen production.402
5.2. Complex oxide supported catalysts
Using a Ni–Al hydrotalcite-like compound (Ni–Al-HT) as the precursor, He et al. obtained a highly dispersed Ni–Al2O3-HT pre-catalyst after reduction of Ni–Al-HT under a H2 atmosphere at 773 K.403 The obtained Ni–Al2O3-HT pre-catalyst showed a high catalytic activity and 93% H2 selectivity towards H2 for N2H4·H2O decomposition within 70 min at 303 K. In contrast, the impregnated counterpart Ni/Al2O3–IMP pre-catalyst exhibited a much lower H2 selectivity (67%) and catalytic activity with a total reaction time of 440 min. The high catalytic performance of Ni–Al2O3-HT could be attributed to a much stronger interaction of the Ni component with Al2O3 and the strongly basic sites. This is the first report that supported a base metal pre-catalyst showing such high H2 selectivity towards the decomposition of N2H4 aqueous solution. They also found that RANEY® Ni was active and exhibited >99% selectivity towards H2 for the decomposition of N2H4·H2O in the presence of NaOH at 303 K.369 Compared with RANEY® Ni-40, RANEY® Ni-300 showed higher catalytic and H2 selectivity in this decomposition reaction, which was probably due to a relatively low content of remaining aluminum. In addition, RANEY® Ni-300 could be easily collected and reused after the catalytic reaction, as RANEY® Ni has excellent magnetic properties. This convenient route provides great potential for industrial applications.
He et al. developed a facile coprecipitation approach to synthesize CeO2-modified Ni RANEY® Ni-300. The obtained Ni–0.08CeO2 pre-catalyst (Fig. 12) showed a 99% H2 selectivity and a 3-fold higher TOF value than bare Ni NPs and the impregnated counterpart Ni/CeO2–IMP for N2H4·H2O decomposition reaction.370 This improvement was caused by the modification of Ni with CeO2 nearby through strong metal–support interactions (e.g., Ni–O–Ce structure). Although the Ni–O–Ce structure itself is inactive for N2H4·H2O decomposition, it could alter the chemical properties of surface Ni and make it both active and selective for N2H4·H2O. Furthermore, this promoting effect could be extended to other oxides which can also form strong metal–support interactions with Ni, such as ZrO2, MgO, and La2O3. Later on, a series of Ni/CeO2 were prepared by solution combustion synthesis (SCS) in a one-step process and used as efficient catalysts for decomposition of N2H4·H2O.404 It was found that the catalytic activity and H2 selectivity for hydrogen evolution from N2H4·H2O significantly depended on the SCS synthesis parameters (ratio of precursor oxidizers, fuel-to-oxidizer ratio and fuel type). The tailored 6 wt% Ni/CeO2 pre-catalyst prepared with a fuel-to-oxidizer ratio of 2 and N2H4 fuel showed the highest catalytic and 100% hydrogen selectivity, for which the decomposition reaction took 17.7 min for 50% conversion of N2H4·H2O in the presence of NaOH, corresponding to a TOF value of 34.0 h−1 at 323 K. The characterization results confirmed the interaction between Ni and CeO2, namely the existence of Ni–O–Ce solid solution. In addition, the oxygen vacancy in the Ni–O–Ce solid solution of the Ni/CeO2 pre-catalyst modifies the electronic ability of Ni as an electron donor and alters the interaction between Ni and N2H4, which promotes N–H bond dissociation rather than N–N bond dissociation and makes the H2 generation easier. Inspired by this effective method, they also found that the addition of Cu to Ni/CeO2 exhibited a synergistic effect to enhance the catalytic activity for the reaction.395
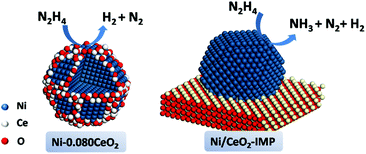 |
| Fig. 12 Structure model of Ni-0.080CeO2 and Ni/CeO2–IMPcatalysts and the scheme of N2H4·H2O decomposition. Reprinted with permission from ref. 370. Copyright (2015) American Chemical Society. | |
The above results showed that the catalytic properties of Ni-based catalysts can be significantly enhanced by introducing a certain amount of CeO2. To further maximize the active interface and the stability of catalysts, our group designed and synthesized ultrafine Ni NPs self-assembled on CeO2 nanowires embedded in a microporous silica shell (Ni–CeO2@SiO2) via a one-pot facile strategy (Fig. 13).405 The resulting wormlike core–shell-structured Ni–CeO2@SiO2 pre-catalyst showed high performance and robust durability with 100% hydrogen selectivity for H2 production from N2H4 aqueous solution. The excellent catalytic properties of Ni–CeO2@SiO2 can be attributed to the synergistic electronic effect and strong interactions between Ni NPs and CeO2 NWs with plenty of oxygen vacancies, as well as the unique structure effect.
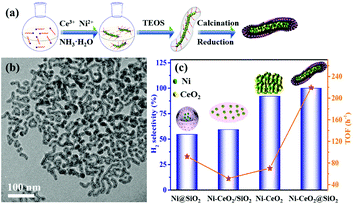 |
| Fig. 13 (a) Synthetic scheme for the preparation of Ni–CeO2@SiO2. (b) The TEM image of the Ni–CeO2@SiO2 catalyst. (c) The hydrogen selectivity and TOF value over different catalysts (nNi/nHH = 0.1) for gas generation from N2H4·H2O (200 mM, 5 mL) at 343 K. Reprinted with permission from ref. 405. Copyright (2020) American Chemical Society. | |
Wen and co-workers synthesized a two-dimensional NiFe/CeO2 pre-catalyst via a dynamics controlling coprecipitation reduction (DCCR) process followed by calcination.406 Small NiFe NPs (∼5 nm) were uniformly anchored on the surface of CeO2 nanosheets. The optimal Ni0.6Fe0.4/CeO2 pre-catalyst displayed over 99% selectivity towards H2 evolution from N2H4·H2O without using an alkali additive at 323 K. Besides CeO2, recently we adopted nano CeZrO2 solid solution as a support to disperse NiFe NPs well, leading to the complete decomposition of N2H4 to H2.407 Later, we prepared a NiFe–La(OH)3 pre-catalyst for N2H4 dehydrogenation, in which metal NPs were highly dispersed with smaller particle size and low crystallinity.408 Importantly, 100% hydrogen selectivity from N2H4 aqueous solution was achieved in 6.5 min, providing a rather high TOF value of 100.6 h−1 at 343 K, which is about 35-fold higher than that of pure NiFe NPs (2.8 h−1).
Using the DCCR method, Wen and co-workers synthesized NiCo/NiO–CoOx ultrathin layered nanocomposites with NiCo NPs (∼4 nm) uniformly anchored on the NiO–CoOx ultrathin layered nanosheets.397 The obtained Ni70Co30/NiO–CoOx pre-catalyst exhibited optimal catalytic performance and 99% hydrogen selectivity for H2 evolution from the decomposition of N2H4·H2O without the assistance of NaOH at 298 K. Wu et al. fabricated a bifunctional NiFe/MgO pre-catalyst containing both the NiFe-alloy active center and a solid base via a calcination–reduction of a NiFeMg-layered double hydroxide (LDH) precursor.393 Moreover, the basicity of Ni1.5Fe1.0/(MgO)z can be easily tuned by changing the amount of the Mg precursor. The optimized Ni1.5Fe1.0/(MgO)3.5 pre-catalyst showed a high catalytic performance with 100% conversion and 99% H2 selectivity for N2H4·H2O decomposition at 299 K. Notably, there was no obvious correlation between the catalytic performance and surface area, which further confirms that the basicity of the MgO support has a more pronounced effect on the catalytic behavior. Recently, our group reported the synthesis of a NiFe–Cr2O3 pre-catalyst, which displayed an extraordinary catalytic activity (893.5 h−1) for the complete decomposition of N2H4·H2O at 343 K.394
5.3. Other supported metal catalysts
Various support materials, such as graphene, carbon nanotubes, titanate nanotubes, mesoporous carbons, and so on, have also been used to fabricate well-dispersed noble-metal NPs with controllable size and morphology. Luo and coworkers reported the synthesis of CeOx-modified NiFe nanodendrites supported on reduced graphene oxide (NiFe–CeOx/rGO).409 The optimal Ni3Fe-(CeOx)0.15/rGO exhibited superior catalytic activity toward H2 evolution from N2H4·H2O under alkaline conditions with a TOF value of 126.2 h−1 at 343 K. Zhao and coworkers prepared Ni nanofibers via an electrospinning and vacuum thermal reduction method.371 The catalytic performance of the prepared Ni nanofibers significantly depended on the morphologies and Ni grain sizes. The Ni nanofibers with PVP
:
EC mass ratios of 7
:
3, and having a high specific surface area and small crystal size, showed nearly 100% hydrogen selectivity to H2 generation and a TOF of 6.9 h−1 in NaOH solution at 333 K. Recently, they have grown carbon nanotubes (CNTs) on Ni nanofibers (Ni–CNTs) and then subjected them to a hydroxylation treatment (Ni–CNTs–OH).410 They found that the introduced CNTs and the hydroxyl groups on the CNTs significantly improved the catalytic performance of the active Ni nanofibers. The Ni–CNTs–OH showed excellent catalytic performance with a TOF of 19.4 h−1 toward the N2H4·H2O decomposition reaction in the presence of NaOH at 333 K.
Wang and co-workers successfully confined Ni NPs inside a titanate nanotube (Ni@TNT) channel by using the capillary force under ultrasonic treatment.411 The obtained Ni@TNT pre-catalyst exhibited high activity and 100% hydrogen selectivity, completing the N2H4·H2O decomposition in only 5 min in the presence of NaOH at 333 K, which is higher than that of Ni/TNTs. In addition, owing to the good stability of the Ni@TNT pre-catalyst, no significant decrease in catalytic performance was observed, while the Ni/TNTs showed an obvious decrease during the reuse (Fig. 14). The excellent catalytic performance of the Ni@TNT pre-catalyst is attributed to the small size and high dispersion of Ni NPs after encapsulation in the channel of TNTs. The Kleitz group prepared highly dispersed CuNi alloy NPs supported on mesoporous carbons (CuNi/MCNS) by a simple incipient wetness method.224 Interestingly, all the bimetallic CuNi/MCNS pre-catalysts showed 100% selectivity to H2 from the N2H4·H2O decomposition and the reaction was complete within 50 min over Cu0.5Ni0.5/MCNS in the presence of NaOH at 333 K.
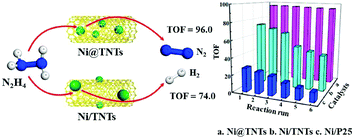 |
| Fig. 14 (Left) Schematic illustration of the formation processes of Ni@TNTs and Ni/TNTs and N2H4·H2O decomposition. (Right) The tests of re-usability of (a) Ni@TNTs, (b) Ni/TNTs and (c) Ni/P25 for hydrogen generation from N2H4·H2O. Reprinted with permission from ref. 411. Copyright (2018) Elsevier. | |
Recently, Wang and coworkers reported the synthesis of Ni10Mo NPs on a Ni–W–O matrix (Ni10Mo NPs/Ni–W–O) through a simple hydrothermal method followed by annealing treatment under a H2 atmosphere.412 The obtained nanocomposite enabled the complete decomposition of N2H4·H2O in 7 min at 323 K, providing a TOF value of 54.5 h−1. They also synthesized a monolithic Ni10Mo/Ni–Mo–O/Ni foam pre-catalyst and showed a high hydrogen generation rate with 98% H2 selectivity and rapid dynamic response after 5 start/stop cycles.412 Using a similar method, they also synthesized a series of Ni–W–O-derived nanocomposites.413 It was found that the catalytic properties of the resulting pre-catalyst depended significantly upon the annealing temperature. On the basis of a combination of experimental and DFT theoretical calculations, the observed changes in catalytic properties are related to the changes in the phase structure and microstructural features with temperature during the reductive annealing process. Specifically, the as-synthesized Ni4W/WO2/NiWO4 exhibited remarkably distinct catalytic performance, nearly 100% selectivity, and high stability in catalyzing N2H4·H2O decomposition for hydrogen production. Furthermore, they also reported a first-principles study of the elementary steps of N2H4 decomposition over a Ni pre-catalyst.372 The calculation results indicated that the decomposition behaviors of N2H4 strongly depend on the surface coverage. At a lower coverage, the cleavage of the N–N bond is dominant, resulting in the formation of NH3. In contrast, at a higher coverage, the cleavage of the N–H bond is in competition with that of the N–N bond, and N2 and H2 are finally released.
Overall, Ni and Ni-based noble-metal-free catalysts can efficiently catalyze the complete decomposition of N2H4·H2O to produce hydrogen, but the catalytic activity is still not satisfactory at room temperature or without additives (e.g. NaOH). Therefore, for the practical use of N2H4·H2O as a safe and effective hydrogen storage material, it is necessary to further develop more effective noble-metal-free catalysts for complete conversion of N2H4·H2O to H2 at low temperature without additives.
6. Hydrazine borane
Hydrazine borane (N2H4BH3, HB, 15.4 wt%), a derivative of NH3BH3 where an N2H4 group substituted the NH3 group, has been regarded as a competitive candidate for chemical hydrogen storage.22,150,151,414–420 N2H4BH3 can be easily synthesized by the reaction of (N2H5)2SO4 with NaBH4 in dioxane at room temperature.421 The hydrogen in N2H4BH3 can be released through either thermolysis or solvolysis.421–428 The thermal decomposition of N2H4BH3 was firstly reported by Goubeau and Ricker.421 N2H4BH3 begins to decompose slowly at around 60 °C. It was found that N2H4BH3 released 6.5 wt% H2 within 16 h at 140 °C, while more than 11 wt% H2 can be released from N2H4BH3 in the presence of LiH at 150 °C in less than an hour.422
Like for NH3BH3, the BH3 group in N2H4BH3 is readily hydrolyzed in the presence of a suitable catalyst (eqn (7)) at room temperature.427–432 The hydrolysis of N2H4BH3 over noble-metal-free catalysts was firstly reported by Özkar and co-workers.430 They found that highly dispersed Ni(0) and Co(0) NPs stabilized by poly(4-styrenesulfonic acid-co-maleic acid (PSSMA-Ni and PSSMA-Co) could be facilely prepared by in situ reduction of NiCl2 and CoCl2 during the hydrolytic dehydrogenation of N2H4BH3.430,431 The in situ formed PSSMA-Ni and PSSMA-Co NPs were highly active in the hydrolysis of N2H4BH3 with the release of nearly 3 equiv. H2 per N2H4BH3. Moreover, the obtained PSSMA-Co (TOF = 370 h−1) showed much higher catalytic performance than PSSMA-Ni (TOF = 183 h−1) for this hydrolysis reaction at 298 K.430,431 The Cu@SiO2 core–shell pre-catalyst developed by our research group also showed excellent catalytic activity (TOF = 454.8 h−1) in the hydrolytic dehydrogenation of N2H4BH3.204 Due to the protection of the porous silica shell, the stability of the pre-catalyst is effectively improved, because of which the Cu@SiO2 nanospheres preserved 85% of their initial catalytic activity even in the tenth run. Recently, we found that transition metal (Cr, Mo, and W) modified Ni NPs showed high activity toward hydrogen generation from the hydrolysis of N2H4BH3 at 298 K.187
| N2H4BH3 + 2H2O → N2H5BO2 + 3H2 | (7) |
By the hydrolysis of the BH3 group of N2H4BH3, the liberation of H2 with good kinetics can be achieved with the catalysts reported above. However, the effective GHSC of the N2H4BH3·3H2O system (6.0 wt%) is not high because H in N2H4 is not released. Similar to hydrolysis, 1 mol N2H4BH3 can also produce 3 mol of H2 by methanolysis in a suitable catalyst (eqn (8)).267,366,425In situ formed bulk Ni and PVP-stabilized Ni NPs were developed by Özkar and co-worker for hydrogen evolution from the methanolysis of N2H4BH3.267,425 Since the weight of methanol is much higher than that of water, the GHSC of an N2H4BH3·4MeOH (3.5 wt%) system is much lower than that of an N2H4BH3·2H2O (7.4 wt%) system. However, the methanolysis reaction of N2H4BH3 can be initiated even below 0 °C, thereby satisfying the cold start requirements of vehicular and portable applications in cold weather.
| N2H4BH3 + 4MeOH → N2H5B(OMe)4 + 3H2 | (8) |
Unlike the NH3 moiety of NH3BH3, the N2H4 moiety in N2H4BH3 can also be dehydrogenated to H2 and N2 over a selective catalyst (eqn (5)).433–442 Theoretically, 1 mol N2H4BH3 can be completely dehydrogenated into 5 mol H2 and 1 mol N2 (eqn (9)). This corresponds to an effective GHSC of 10.0 wt% for the N2H4BH3·3H2O system, which is much higher than those of NaBH4·4H2O (7.3 wt%), NH3BH3·2H2O (8.9 wt%) and N2H4·H2O (8.0 wt%).22,416 However, the dehydrogenation reaction is in competition with NH3 release (eqn (10)). In 2014, Demirci and co-worker synthesized a series of Ni-based bimetallic pre-catalysts for the hydrolysis of the BH3 group and then decomposition of the N2H4 group.443 The optimized Ni0.7Fe0.3 NPs can release 3.9 equiv. (H2 + N2) per N2H4BH3 (21% H2 selectivity) in 180 min at 323 K, indicating an activity in the decomposition of the N2H4 moiety NH3BH3. However, the conversion of N2H4BH3 is incomplete and the reaction kinetics is slow. Therefore, great efforts were devoted to synthesize a highly selective catalyst that could achieve complete dehydrogenation of NH3BH3 to H2.
| N2H4BH3 + 3H2O → B(OH)3 + 5H2 + N2 | (9) |
| N2H4BH3 + 3H2O → B(OH)3 + (3 + 2α)H2 + (2α + 1)/3N2 + 4(1 − α)/3NH3 | (10) |
In 2018, our group reported highly active and selective noble-metal-free CuNiMo NPs by using a facile chemical reduction approach.402 Among all the synthesized pre-catalysts, the optimized Cu0.4Ni0.6Mo exhibited the highest catalytic activity and 100% H2 selectivity toward hydrogen generation from N2H4BH3 in an alkaline solution, with which 6 equiv. (H2 + N2) per N2H4BH3 (Fig. 15) can be released within 13.9 min. To the best of our knowledge, this is the first report that a noble-metal-free pre-catalyst can achieve a complete conversion of N2H4BH3 to H2 (Table 5). Later on, Yan and co-workers reported the synthesis of a boron nitride (BN) supported Ni-MoOx (Ni-MoOx/BN) pre-catalyst without the help of a surfactant by a sequential impregnation reduction approach.444 The resultant Ni-MoOx/BN showed excellent catalytic performance with 100% H2 selectivity for hydrogen generation from N2H4BH3 alkaline conditions at 323 K, giving a TOF value of 600.0 h−1. They also developed a facile and universal methodology for the synthesis of amorphous/poorly crystallized noble-metal-free NiFe–CeOx NPs supported on a MOF substrate, and the obtained NiFe–CeOx/MOF pre-catalyst can also show 100% H2 selectivity and record good catalytic performance for N2H4BH3 decomposition at 343 K.445
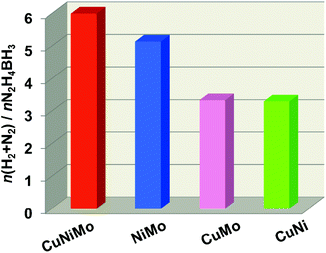 |
| Fig. 15
n(H2 + N2)/n(N2H4BH3) for dehydrogenation of N2H4BH3 catalyzed by CuNiMo, NiMo, CuMo, and CuNi nanocatalysts in the presence of NaOH (2.0 M) at 323 K. Reprinted with permission from ref. 402. Copyright (2018) Royal Society of Chemistry. | |
Table 5 Catalytic activities for hydrogen evolution from N2H4BH3 by different catalysts
Catalyst |
Temp. (K) |
n
metal/nN2H4BH3 |
n(H2 + N2)/nN2H4BH3 |
TOF (molH2 molmetal−1 h−1) |
Ref. |
Hydrogen release from the hydrolysis of the BH3 group of HB only.
Hydrogen release from the methanolysis of the BH3 group of HB only.
The TOF reported here was calculated based on the surface Ni atoms in the catalyst.
|
Ni/PSSMAa |
298 |
0.01 |
3 |
183 |
430
|
Co/PSSMAa |
298 |
0.01 |
3 |
370 |
431
|
Cu@SiO2a |
298 |
0.09 |
3 |
454.8 |
204
|
Ni0.9Mo0.1a |
298 |
0.05 |
3 |
2400 |
187
|
Bulk Ni NPsb |
298 |
0.006 |
3 |
1440 |
425
|
PVP-stabilized Nib |
298 |
0.005 |
3 |
2136 |
267
|
Ni0.7Fe0.3 NPs |
323 |
0.35 |
3.9 |
3.3 |
443
|
Cu0.4Ni0.6Mo |
323 |
0.1 |
6 |
108 |
402
|
Ni-MoOx/BN |
323 |
0.1 |
6 |
600 |
444
|
Ni0.5Fe0.5-CeOx/MIL-101 |
343 |
0.2 |
6 |
351.3 |
445
|
Ni0.5Fe0.5-CeOx/ZIF-67 |
343 |
0.2 |
6 |
361.5 |
445
|
Ni–CeO2@SiO2 |
343 |
0.1 |
6 |
442.5 |
405
|
NiFe–La(OH)3 |
343 |
0.2 |
6 |
251.4 |
408
|
RANEY® Nic |
298 |
— |
6 |
892 |
446
|
Recently, our group reported a wormlike Ni–CeO2@SiO2 core–shell pre-catalyst with high performance (442.5 h−1) and 100% hydrogen selectivity for hydrogen evolution from N2H4BH3 aqueous solution at 343 K.405 In addition, we proposed a plausible mechanism for metal catalyzed dehydrogenation of N2H4BH3, which involves the chemisorption of N2H4BH3 molecules on the metal active surface and generates the activated intermediate species.405 Subsequently, the activated intermediate species is attacked by H2O molecules, leading to the dissociation of the B–N bond in N2H4BH3. Then, the BH3 moiety of N2H4BH3 is catalytically hydrolyzed by metal active sites; meanwhile, the resulting N2H4 group is decomposed to produce H2 and N2.
Despite much progress having been made in recent years, the noble-metal-free catalysts reported above for complete hydrogen evolution from NH3BH3 required a relatively high reaction temperature (≥323 K). Therefore, the development of non-noble-metal catalysts for the complete dehydrogenation of N2H4BH3 at room temperature is highly desired. We found that the RANEY® Ni pre-catalyst exhibited an outstanding catalytic performance with 6.0 equiv. (H2 + N2) per N2H4BH3 being released at 298 K, which is the first report of a noble-metal-free pre-catalyst achieving a complete dehydrogenation of N2H4BH3 at room temperature (Table 5).446 The TOF value over RANEY® Ni for the complete dehydrogenation of N2H4BH3 at room temperature was calculated to be 892 h−1 based on surface Ni atoms.
Up to now, preliminary progress has been made in noble-metal-free catalysts for complete hydrogen generation from N2H4BH3. However, there are still only a handful of catalysts that can catalyze the complete hydrogen evolution from N2H4BH3 (Table 5). Moreover, the kinetics of the N2H4 moiety decomposition is much sluggish than that of the BH3 group hydrolysis. Therefore, the current challenges include finding a catalyst that can catalyze both BH3 group hydrolysis and N2H4 moiety decomposition reactions with similar kinetics.
7. Summary
Safe and efficient storage and delivery of hydrogen are essential for the development of a hydrogen-based energy infrastructure. Boron- and nitrogen-based hydrogen storage materials reviewed above have a relatively high hydrogen content and have tremendous potential to be used as hydrogen sources for portable fuel cells. Overall, each of these materials has its own merits and drawbacks. The catalytic performances for hydrogen evolution from these systems have been greatly improved. Non-noble metal catalysts with low cost and relatively high catalytic activity can make boron- and nitrogen-based hydrides potential candidates for portable fuel cells. However, there is still a certain gap in the catalytic activity between non-noble metal catalysts and noble metal catalysts. In addition, the stability of non-noble metal catalysts is generally low, mainly because of their easy oxidation and agglomeration. Therefore, the development of high-activity and high-stability non-noble metal catalysts still requires further efforts.
Besides, the mechanism of nucleation and growth of metal NPs and the active sites of multi-component catalysts are not clear. Theoretical calculations and modern characterization technologies (e.g., in situ X-ray absorption spectroscopy (XAS), transmission electron microscopy (TEM), Raman spectroscopy, etc.) could be helpful in understanding the structure–catalysis relationship, thus providing an effective method to guide the design of metal catalysts at the molecular level. Furthermore, some new methods (e.g. photocatalysis assisted technology) can be developed to promote the hydrogen generation rate from boron- and nitrogen-based chemical hydrides. Additionally, to maximize the use of metal atoms, highly dispersed catalysts (e.g. metal single-atom) can be designed and synthesized for further improving the catalytic activity of the catalyst. We are looking forward to new breakthroughs in noble-metal-free catalysts for hydrogen generation from boron- and nitrogen-based chemical hydrides and their practical applications.
Conflicts of interest
There are no conflicts to declare.
Acknowledgements
This work was financially supported by the National Natural Science Foundation of China (No. 21763012 and 21802056), the Natural Science Foundation of Jiangxi Province of China (No. 20192BAB203009), and the Sponsored Program for Cultivating Youths of Outstanding Ability in Jiangxi Normal University.
References
- L. Schlapbach and A. Zuttel, Hydrogen-storage materials for mobile applications, Nature, 2001, 414, 353–358 CrossRef CAS.
- U. Eberle, M. Felderhoff and F. Schueth, Chemical and physical solutions for hydrogen storage, Angew. Chem., Int. Ed., 2009, 48, 6608–6630 CrossRef CAS.
- J. H. Shi, F. Qiu, W. B. Yuan, M. M. Guo, C. L. Yuan and Z. H. Lu, Novel electrocatalyst of nanoporous FeP cubes prepared by fast electrodeposition coupling with acid-etching for efficient hydrogen evolution, Electrochim. Acta, 2020, 329, 135185 CrossRef CAS.
- J. H. Shi, F. Qiu, W. B. Yuan, M. M. Guo and Z. H. Lu, Nitrogen-doped carbon-decorated yolk-shell CoP@FeCoP micro-polyhedra derived from MOF for efficient overall water splitting, Chem. Eng. J., 2021, 403, 126312 CrossRef CAS.
- S. I. Orimo, Y. Nakamori, J. R. Eliseo, A. Zuettel and C. M. Jensen, Complex hydrides for hydrogen storage, Chem. Rev., 2007, 107, 4111–4132 CrossRef CAS.
- H. M. Cheng, Q. H. Yang and C. Liu, Hydrogen storage in carbon nanotubes, Carbon, 2001, 39, 1447–1454 CrossRef CAS.
- H. W. Langmi, D. Book, A. Walton, S. R. Johnson, M. M. Al-Mamouri, J. D. Speight, P. P. Edwards, I. R. Harris and P. A. Anderson, Hydrogen storage in ion-exchanged zeolites, J. Alloys Compd., 2005, 404, 637–642 CrossRef.
- N. L. Rosi, J. Eckert, M. Eddaoudi, D. T. Vodak, J. Kim, M. O'Keeffe and O. M. Yaghi, Hydrogen storage in microporous metal-organic frameworks, Science, 2003, 300, 1127–1129 CrossRef CAS.
- Y. G. Wang, N. Shah and G. P. Huffman, Simultaneous production of hydrogen and carbon nanostructures by decomposition of propane and cyclohexane over alumina supported binary catalysts, Catal. Today, 2005, 99, 359–364 CrossRef CAS.
- P. Makowski, A. Thomas, P. Kuhn and F. Goettmann, Organic materials for hydrogen storage applications: from physisorption on organic solids to chemisorption in organic molecules, Energy Environ. Sci., 2009, 2, 480–490 RSC.
- U. Eberle, M. Felderhoff and F. Schuth, Chemical and physical solutions for hydrogen storage, Angew. Chem., Int. Ed., 2009, 48, 6608–6630 CrossRef CAS.
- J. Chen, Z. H. Lu, Q. L. Yao, G. Feng and Y. Luo, Complete dehydrogenation of N2H4BH3 with NiM-Cr2O3 (M = Pt, Rh, and Ir) hybrid nanoparticles, J. Mater. Chem. A, 2018, 6, 20746–20752 RSC.
- Z. Zhang, Y. Luo, S. Liu, Q. Yao, S. Qing and Z. H. Lu, A PdAg-CeO2 nanocomposite anchored on mesoporous carbon: a highly efficient catalyst for hydrogen production from formic acid at room temperature, J. Mater. Chem. A, 2019, 7, 21438–21446 RSC.
- Y. Luo, Q. Yang, W. Nie, Q. L. Yao, Z. Zhang and Z. H. Lu, Anchoring IrPdAu nanoparticles on NH2-SBA-15 for fast hydrogen production from formic acid at room temperature, ACS Appl. Mater. Interfaces, 2020, 12, 8082–8090 CrossRef CAS.
- C. W. Hamilton, R. T. Baker, A. Staubitz and I. Manners, B-N compounds for chemical hydrogen storage, Chem. Soc. Rev., 2009, 38, 279–293 RSC.
- H. L. Jiang, S. K. Singh, J. M. Yan, X. B. Zhang and Q. Xu, Liquid-phase chemical hydrogen storage: catalytic hydrogen generation under ambient conditions, ChemSusChem, 2010, 3, 541–549 CrossRef CAS.
- T. He, H. Wu, G. Wu, J. Wang, W. Zhou, Z. Xiong, J. Chen, T. Zhang and P. Chen, Borohydride hydrazinates: high hydrogen content materials for hydrogen storage, Energy Environ. Sci., 2012, 5, 5686–5689 RSC.
- G. Moussa, R. Moury, U. B. Demirci, T. Şener and P. Miele, Boron-based hydrides for chemical hydrogen storage, Int. J. Energy Res., 2013, 37, 825–842 CrossRef CAS.
- L. He, B. Liang, Y. Huang and T. Zhang, Design strategies of highly selective nickel catalysts for H2 production via hydrous hydrazine decomposition: a review, Natl. Sci. Rev., 2018, 5, 356–364 CrossRef CAS.
- Y. Cheng, X. Wu and H. Xu, Catalytic decomposition of hydrous hydrazine for hydrogen production, Sustainable Energy Fuels, 2019, 3, 343–365 RSC.
- C. Lang, Y. Jia and X. Yao, Recent advances in liquid-phase chemical hydrogen storage, Energy Storage Mater., 2020, 26, 290–312 CrossRef.
- M. Yadav and Q. Xu, Liquid-phase chemical hydrogen storage materials, Energy Environ. Sci., 2012, 5, 9698–9725 RSC.
- A. K. Singh and Q. Xu, Synergistic Catalysis over Bimetallic Alloy Nanoparticles, ChemCatChem, 2013, 5, 652–676 CrossRef CAS.
- T. E. Bell and L. Torrente-Murciano, H2 production via ammonia decomposition using non-noble metal catalysts: A review, Top. Catal., 2016, 59, 1438–1457 CrossRef CAS.
- W. W. Zhan, Q. L. Zhu and Q. Xu, Dehydrogenation of ammonia aorane by metal nanoparticle catalysts, ACS Catal., 2016, 6, 6892–6905 CrossRef CAS.
- S. Mukherjee, S. V. Devaguptapu, A. Sviripa, C. R. F. Lund and G. Wu, Low-temperature ammonia decomposition catalysts for hydrogen generation, Appl. Catal., B, 2018, 226, 162–181 CrossRef CAS.
- Y. Hu, Y. Wang, Z. H. Lu, X. Chen and L. Xiong, Core–shell nanospheres Pt@SiO2 for catalytic hydrogen production, Appl. Surf. Sci., 2015, 341, 185–189 CrossRef CAS.
- Q. L. Yao, Z. H. Lu, Y. Jia, X. Chen and X. Liu, In situ facile synthesis of Rh nanoparticles supported on carbon nanotubes as highly active catalysts for H2 generation from NH3BH3 hydrolysis, Int. J. Hydrogen Energy, 2015, 40, 2207–2215 CrossRef CAS.
- W. Wang, Z. H. Lu, Y. Luo, A. Zou, Q. L. Yao and X. Chen, Mesoporous carbon nitride supported Pd and Pd-Ni nanoparticles as highly efficient catalyst for catalytic hydrolysis of NH3BH3, ChemCatChem, 2018, 10, 1620–1626 CrossRef CAS.
- W. Wang, X. Hong, Q. L. Yao and Z. H. Lu, Bimetallic Ni–Pt nanoparticles immobilized on mesoporous N-doped carbon as a highly efficient catalyst for complete hydrogen evolution from hydrazine, J. Mater. Chem. A, 2020, 8, 13694–13701 RSC.
- U. B. Demirci and P. Miele, Cobalt-based catalysts for the hydrolysis of NaBH4 and NH3BH3, Phys. Chem. Chem. Phys., 2014, 16, 6872–6885 RSC.
- Y. J. Shih, C. C. Su, Y. H. Huang and M. C. Lu, SiO2-supported ferromagnetic catalysts for hydrogen generation from alkaline NaBH4 (sodium borohydride) solution, Energy, 2013, 54, 263–270 CrossRef CAS.
- B. H. Liu and Z. P. Li, A review: Hydrogen generation from borohydride hydrolysis reaction, J. Power Sources, 2009, 187, 527–534 CrossRef CAS.
- U. B. Demirci and P. Miele, Sodium borohydride versus ammonia borane, in hydrogen storage and direct fuel cell applications, Energy Environ. Sci., 2009, 2, 627–637 RSC.
- L. Lu, H. Zhang, S. Zhang and F. Li, A family of high-efficiency hydrogen-generation catalysts based on ammonium species, Angew. Chem., Int. Ed., 2015, 54, 9328–9332 CrossRef CAS.
- J. Wang, Z. Huang, L. Lu, Q. Jia, L. Huang, S. Chang, M. Zhang, Z. Zhang, S. Li, D. He, W. Wu, S. Zhang, N. Toshima and H. Zhang, Colloidal Co single-atom catalyst: a facile synthesis strategy and high catalytic activity for hydrogen generation, Green Chem., 2020, 22, 1269–1274 RSC.
- O. Sahin, H. Dolas and M. Ozdemir, The effect of various factors on the hydrogen generation by hydrolysis reaction of potassium borohydride, Int. J. Hydrogen Energy, 2007, 32, 2330–2336 CrossRef CAS.
- D. Xu, H. Wang, Q. Guo and S. Ji, Catalytic behavior of carbon supported Ni–B, Co–B and Co–Ni–B in hydrogen generation by hydrolysis of KBH4, Fuel Process. Technol., 2011, 92, 1606–1610 CrossRef CAS.
- X. Wang, S. Sun, Z. Huang, H. Zhang and S. Zhang, Preparation and catalytic activity of PVP-protected Au/Ni bimetallic nanoparticles for hydrogen generation from hydrolysis of basic NaBH4 solution, Int. J. Hydrogen Energy, 2014, 39, 905–916 CrossRef CAS.
- X. Wang, Z. Huang, L. Lu, H. Zhang, Y. Cao, Y. Gu, Z. Cheng and S. Zhang, Preparation and catalytic activities of Au/Co bimetallic nanoparticles for hydrogen generation from NaBH4 solution, J. Nanosci. Nanotechnol., 2015, 15, 2770–2276 CrossRef CAS.
- L. Wang, L. Huang, C. Jiao, Z. Huang, F. Liang, S. Liu, Y. Wang and H. Zhang, Preparation of Rh/Ni bimetallic nanoparticles and their catalytic activities for hydrogen generation from hydrolysis of KBH4, Catalysts, 2017, 7, 125 CrossRef.
- L. Huang, C. Jiao, L. Wang, Z. Huang, F. Liang, S. Liu, Y. Wang, H. Zhang and S. Zhang, Preparation of Rh/Ag bimetallic nanoparticles as effective catalyst for hydrogen generation from hydrolysis of KBH4, Nanotechnology, 2018, 29, 044002 CrossRef.
- Y. Pei, L. Wang, L. Huang, Y. Hu, Q. Jia, H. Zhang and S. Zhang, ISOBAM-stabilized Ni2+ colloidal catalysts: high catalytic activities for hydrogen generation from hydrolysis of KBH4, Nanotechnology, 2020, 31, 134003 CrossRef CAS.
- J. Urgnani, F. J. Torres, M. Palumbo and M. Baricco, Hydrogen release from solid state NaBH4, Int. J. Hydrogen Energy, 2008, 33, 3111–3115 CrossRef CAS.
- P. Martelli, R. Caputo, A. Remhof, P. Mauron, A. Borgschulte and A. Züttel, Stability and decomposition of NaBH4, J. Phys. Chem. C, 2010, 114, 7173–7177 CrossRef CAS.
- P. Ngene, R. van den Berg, M. H. W. Verkuijlen, K. P. de Jong and P. E. de Jongh, Reversibility of the hydrogen desorption from NaBH4 by confinement in nanoporous carbon, Energy Environ. Sci., 2011, 4, 4108–4115 RSC.
- S. S. Muir and X. Yao, Progress in sodium borohydride as a hydrogen storage material: Development of hydrolysis catalysts and reaction systems, Int. J. Hydrogen Energy, 2011, 36, 5983–5997 CrossRef CAS.
- P. Brack, S. E. Dann and K. G. U. Wijayantha, Heterogeneous and homogenous catalysts for hydrogen generation by hydrolysis of aqueous sodium borohydride (NaBH4) solutions, Energy Sci. Eng., 2015, 3, 174–188 CrossRef CAS.
- K. A. Holbrook and P. J. Twist, Hydrolysis of the borohydride ion catalysed by metal-boron alloys, J. Chem. Soc. A, 1971, 15, 890–894 RSC.
- R. Peña-Alonso, A. Sicurelli, E. Callone, G. Carturan and R. Raj, A picoscale catalyst for hydrogen generation from NaBH4 for fuel cells, J. Power Sources, 2007, 165, 315–323 CrossRef.
- G. Guella, C. Zanchetta, B. Patton and A. Miotello, New insights on the mechanism of palladium-catalyzed hydrolysis of sodium borohydride from 11B NMR measurements, J. Phys. Chem. B, 2006, 110, 17024–17033 CrossRef CAS.
- G. Guella, B. Patton and A. Miotello, Kinetic features of the platinum catalyzed hydrolysis of sodium borohydride from 11B NMR measurements, J. Phys. Chem. C, 2007, 111, 18744–18750 CrossRef CAS.
- P. Wang and X. D. Kang, Hydrogen-rich boron-containing materials for hydrogen storage, Dalton Trans., 2008, 40, 5400–5413 RSC.
- H. I. Schlesinger, H. C. Brown, A. E. Finholt, J. R. Gilbreath, H. R. Hoekstra and E. K. Hyde, Sodium borohydride, its hydrolysis and its use as a reducing agent and in the generation of hydrogen, J. Am. Chem. Soc., 1953, 75, 215–219 CrossRef CAS.
- M. Zahmakiran and S. Özkar, Zeolite-confined ruthenium(0) nanoclusters catalyst: record catalytic activity, reusability, and lifetime in hydrogen generation from the hydrolysis of sodium borohydride, Langmuir, 2009, 25, 2667–2678 CrossRef CAS.
- Y. V. Larichev, O. V. Netskina, O. V. Komova and V. I. Simagina, comparative XPS study of Rh/Al2O3 and Rh/TiO2 as catalysts for NaBH4 hydrolysis, Int. J. Hydrogen Energy, 2010, 35, 6501–6507 CrossRef CAS.
- D. Xu, H. Zhang and W. Ye, Hydrogen generation from hydrolysis of alkaline sodium borohydride solution using Pt/C catalyst, Catal. Commun., 2007, 8, 1767–1771 CrossRef CAS.
- P. Krishnan, T.-H. Yang, W.-Y. Lee and C.-S. Kim, PtRu-LiCoO2—an efficient catalyst for hydrogen generation from sodium borohydride solutions, J. Power Sources, 2005, 143, 17–23 CrossRef CAS.
- U. B. Demirci and P. Miele, Cobalt in NaBH4 hydrolysis, Phys. Chem. Chem. Phys., 2010, 12, 14651–14665 RSC.
- N. Patel and A. Miotello, Progress in Co–B related catalyst for hydrogen production by hydrolysis of boron-hydrides: A review and the perspectives to substitute noble metals, Int. J. Hydrogen Energy, 2015, 40, 1429–1464 CrossRef CAS.
- H. Sun, J. Meng, L. Jiao, F. Cheng and J. Chen, A review of transition-metal boride/phosphide-based materials for catalytic hydrogen generation from hydrolysis of boron-hydrides, Inorg. Chem. Front., 2018, 5, 760–772 RSC.
- M. Rakap and S. Özkar, Intrazeolite cobalt(0) nanoclusters as low-cost and reusable catalyst for hydrogen generation from the hydrolysis of sodium borohydride, Appl. Catal., B, 2009, 91, 21–29 CrossRef CAS.
- J. W. Jaworski, S. Cho, Y. Kim, J. H. Jung, H. S. Jeon, B. K. Min and K. Y. Kwon, Hydroxyapatite supported cobalt catalysts for hydrogen generation, J. Colloid Interface Sci., 2013, 394, 401–408 CrossRef CAS.
- S. Bennici, H. Yu, E. Obeid and A. Auroux, Highly active heteropolyanions supported Co catalysts for fast hydrogen generation in NaBH4 hydrolysis, Int. J. Hydrogen Energy, 2011, 36, 7431–7442 CrossRef CAS.
- D. Xu, P. Dai, X. Liu, C. Cao and Q. Guo, Carbon-supported cobalt catalyst for hydrogen generation from alkaline sodium borohydride solution, J. Power Sources, 2008, 182, 616–620 CrossRef CAS.
- J. Zhu, R. Li, W. Niu, Y. Wu and X. Gou, Facile hydrogen generation using colloidal carbon supported cobalt to catalyze hydrolysis of sodium borohydride, J. Power Sources, 2012, 211, 33–39 CrossRef CAS.
- J. Zhu, R. Li, W. Niu, Y. Wu and X. Gou, Fast hydrogen generation from NaBH4 hydrolysis catalyzed by carbon aerogels supported cobalt nanoparticles, Int. J. Hydrogen Energy, 2013, 38, 10864–10870 CrossRef CAS.
- X. Zhang, X. Sun, D. Xu, X. Tao, P. Dai, Q. Guo and X. Liu, Synthesis of MOF-derived Co@C composites and application for efficient hydrolysis of sodium borohydride, Appl. Surf. Sci., 2019, 469, 764–769 CrossRef CAS.
- J. Li, X. Hong, Y. Wang, Y. Luo, P. Huang, B. Li, K. Zhang, Y. Zou, L. Sun, F. Xu, F. Rosei, S. P. Verevkin and A. A. Pimerzin, Encapsulated cobalt nanoparticles as a recoverable catalyst for the hydrolysis of sodium borohydride, Energy Storage Mater., 2020, 27, 187–197 CrossRef.
- C. H. Liu, B. H. Chen, C. L. Hsueh, J. R. Ku, F. Tsau and K. J. Hwang, Preparation of magnetic cobalt-based catalyst for hydrogen generation from alkaline NaBH4 solution, Appl. Catal., B, 2009, 91, 368–379 CrossRef CAS.
- B. Chen, S. Chen, H. A. Bandal, R. Appiah-Ntiamoah, A. R. Jadhav and H. Kim, Cobalt nanoparticles supported on magnetic core-shell structured carbon as a highly efficient catalyst for hydrogen generation from NaBH4 hydrolysis, Int. J. Hydrogen Energy, 2018, 43, 9296–9306 CrossRef CAS.
- A. F. Baye, M. W. Abebe, R. Appiah-Ntiamoah and H. Kim, Engineered iron-carbon-cobalt (Fe3O4@C-Co) core-shell composite with synergistic catalytic properties towards hydrogen generation via NaBH4 hydrolysis, J. Colloid Interface Sci., 2019, 543, 273–284 CrossRef CAS.
- F. Seven and N. Sahiner, Enhanced catalytic performance in hydrogen generation from NaBH4 hydrolysis by super porous cryogel supported Co and Ni catalysts, J. Power Sources, 2014, 272, 128–136 CrossRef CAS.
- J. Lee, H. Shin, K. S. Choi, J. Lee, J.-Y. Choi and H. K. Yu, Carbon layer supported nickel catalyst for sodium borohydride (NaBH4) dehydrogenation, Int. J. Hydrogen Energy, 2019, 44, 2943–2950 CrossRef CAS.
- R. Barrios-Francisco and J. J. García, Semihydrogenation of alkynes in the presence of Ni(0) catalyst using ammonia-borane and sodium borohydride as hydrogen sources, Appl. Catal., A, 2010, 385, 108–113 CrossRef CAS.
- S. U. Jeong, R. K. Kim, E. A. Cho, H. J. Kim, S. W. Nam, I. H. Oh, S. A. Hong and S. H. Kim, A study on hydrogen generation from NaBH4 solution using the high-performance Co-B catalyst, J. Power Sources, 2005, 144, 129–134 CrossRef CAS.
- C. Wu, F. Wu, Y. Bai, B. Yi and H. Zhang, Cobalt boride catalysts for hydrogen generation from alkaline NaBH4 solution, Mater. Lett., 2005, 59, 1748–1751 CrossRef CAS.
- N. Patel, G. Guella, A. Kale, A. Miotello, B. Patton, C. Zanchetta, L. Mirenghi and P. Rotolo, Thin films of Co–B prepared by pulsed laser deposition as efficient catalysts in hydrogen producing reactions, Appl. Catal., A, 2007, 323, 18–24 CrossRef CAS.
- H. Ma, W. Ji, J. Zhao, J. Liang and J. Chen, Preparation, characterization and catalytic NaBH4 hydrolysis of Co-B hollow spheres, J. Alloys Compd., 2009, 474, 584–589 CrossRef CAS.
- C. C. Yang, M. S. Chen and Y. W. Chen, Hydrogen generation by hydrolysis of sodium borohydride on CoB/SiO2 catalyst, Int. J. Hydrogen Energy, 2011, 36, 1418–1423 CrossRef CAS.
- S. Gupta, N. Patel, R. Fernandes, D. C. Kothari and A. Miotello, Mesoporous Co–B nanocatalyst for efficient hydrogen production by hydrolysis of sodium borohydride, Int. J. Hydrogen Energy, 2013, 38, 14685–14692 CrossRef CAS.
- S. U. Jeong, E. A. Cho, S. W. Nam, I. H. Oh, U. H. Jung and S. H. Kim, Effect of preparation method on Co–B catalytic activity for hydrogen generation from alkali NaBH4 solution, Int. J. Hydrogen Energy, 2007, 32, 1749–1754 CrossRef CAS.
- O. Akdim, U. B. Demirci, D. Muller and P. Miele, Cobalt(II) salts, performing materials for generating hydrogen from sodium borohydride, Int. J. Hydrogen Energy, 2009, 34, 2631–2637 CrossRef CAS.
- X. Shen, M. Dai, M. Gao, B. Zhao and W. Ding, Solvent effects in the synthesis of CoB catalysts on hydrogen generation from hydrolysis of sodium borohydride, Chin. J. Catal., 2013, 34, 979–985 CrossRef CAS.
- R. Fernandes, N. Patel and A. Miotello, Hydrogen generation by hydrolysis of alkaline NaBH4 solution with Cr-promoted Co–B amorphous catalyst, Appl. Catal., B, 2009, 92, 68–74 CrossRef CAS.
- N. Patel, R. Fernandes and A. Miotello, Promoting effect of transition metal-doped Co–B alloy catalysts for hydrogen production by hydrolysis of alkaline NaBH4 solution, J. Catal., 2010, 271, 315–324 CrossRef CAS.
- H. B. Dai, Y. Liang and P. Wang, Effect of trapped hydrogen on the induction period of cobalt–tungsten–boron/nickel foam catalyst in catalytic hydrolysis reaction of sodium borohydride, Catal. Today, 2011, 170, 27–32 CrossRef CAS.
- A. Ekinci, Ö. Şahin, C. Saka and T. Avci, The effects of plasma treatment on electrochemical activity of Co–W–B catalyst for hydrogen production by hydrolysis of NaBH4, Int. J. Hydrogen Energy, 2013, 38, 15295–15301 CrossRef CAS.
- D. Ke, Y. Tao, Y. Li, X. Zhao, L. Zhang, J. Wang and S. Han, Kinetics study on hydrolytic dehydrogenation of alkaline sodium borohydride catalyzed by Mo-modified Co–B nanoparticles, Int. J. Hydrogen Energy, 2015, 40, 7308–7317 CrossRef CAS.
- X. Shen, Q. Wang, S. Guo, B. Liu, Z. Sun, Z. Zhang, Z. Wang, B. Zhao and W. Ding, W-modified CoB supported on Ag-activated TiO 2 for hydrogen generation from alkaline NaBH 4 solution, Int. J. Hydrogen Energy, 2015, 40, 6346–6357 CrossRef CAS.
- Y. Wei, R. Wang, L. Meng, Y. Wang, G. Li, S. Xin, X. Zhao and K. Zhang, Hydrogen generation from alkaline NaBH4 solution using a dandelion-like Co–Mo–B catalyst supported on carbon cloth, Int. J. Hydrogen Energy, 2017, 42, 9945–9951 CrossRef CAS.
- X. Yuan, C. Jia, X. L. Ding and Z. F. Ma, Effects of heat-treatment temperature on properties of Cobalt–manganese–Boride as efficient catalyst toward hydrolysis of alkaline sodium borohydride solution, Int. J. Hydrogen Energy, 2012, 37, 995–1001 CrossRef CAS.
- M. Aydin, A. Hasimoglu and O. K. Ozdemir, Kinetic properties of Cobalt–Titanium–Boride (Co–Ti–B) catalysts for sodium borohydride hydrolysis reaction, Int. J. Hydrogen Energy, 2016, 41, 239–248 CrossRef CAS.
- Y. Zou, Y. Yin, Y. Gao, C. Xiang, H. Chu, S. Qiu, E. Yan, F. Xu and L. Sun, Chitosan-mediated Co–Ce–B nanoparticles for catalyzing the hydrolysis of sodium borohydride, Int. J. Hydrogen Energy, 2018, 43, 4912–4921 CrossRef CAS.
- C. Xiang, D. Jiang, Z. She, Y. Zou, H. Chu, S. Qiu, H. Zhang, F. Xu, C. Tang and L. Sun, Hydrogen generation by hydrolysis of alkaline sodium borohydride using a cobalt–zinc–boron/graphene nanocomposite treated with sodium hydroxide, Int. J. Hydrogen Energy, 2015, 40, 4111–4118 CrossRef CAS.
- J. Zhao, H. Ma and J. Chen, Improved hydrogen generation from alkaline NaBH4 solution using carbon-supported Co–B as catalysts, Int. J. Hydrogen Energy, 2007, 32, 4711–4716 CrossRef CAS.
- Y. Huang, Y. Wang, R. Zhao, P. Shen and Z. Wei, Accurately measuring the hydrogen generation rate for hydrolysis of sodium borohydride on multiwalled carbon nanotubes/Co–B catalysts, Int. J. Hydrogen Energy, 2008, 33, 7110–7115 CrossRef CAS.
- F. Li, Q. Li and H. Kim, CoB/open-CNTs catalysts for hydrogen generation from alkaline NaBH4 solution, Chem. Eng. J., 2012, 210, 316–324 CrossRef CAS.
- Y. C. Lu, M. S. Chen and Y. W. Chen, Hydrogen generation by sodium borohydride hydrolysis on nanosized CoB catalysts supported on TiO2, Al2O3 and CeO2, Int. J. Hydrogen Energy, 2012, 37, 4254–4258 CrossRef CAS.
- J. Cheng, C. Xiang, Y. Zou, H. Chu, S. Qiu, H. Zhang, L. Sun and F. Xu, Highly active nanoporous Co–B–TiO2 framework for hydrolysis of NaBH4, Ceram. Int., 2015, 41, 899–905 CrossRef CAS.
- X. Shen, Q. Wang, Q. Wu, S. Guo, Z. Zhang, Z. Sun, B. Liu, Z. Wang, B. Zhao and W. Ding, CoB supported on Ag-activated TiO2 as a highly active catalyst for hydrolysis of alkaline NaBH4 solution, Energy, 2015, 90, 464–474 CrossRef CAS.
- H. B. Dai, Y. Liang, P. Wang and H. M. Cheng, Amorphous cobalt–boron/nickel foam as an effective catalyst for hydrogen generation from alkaline sodium borohydride solution, J. Power Sources, 2008, 177, 17–23 CrossRef CAS.
- S. Guo, Q. Wu, J. Sun, T. Chen, M. Feng, Q. Wang, Z. Wang, B. Zhao and W. Ding, Highly stable and controllable CoB/Ni-foam catalysts for hydrogen generation from alkaline NaBH4 solution, Int. J. Hydrogen Energy, 2017, 42, 21063–21072 CrossRef CAS.
- Y. Chen and H. Kim, Use of a nickel-boride–silica nanocomposite catalyst prepared by in situ reduction for hydrogen production from hydrolysis of sodium borohydride, Fuel Process. Technol., 2008, 89, 966–972 CrossRef CAS.
- J. K. Lee, H. H. Ann, Y. Yi, K. W. Lee, S. Uhm and J. Lee, A stable Ni–B catalyst in hydrogen generation via NaBH4 hydrolysis, Catal. Commun., 2011, 16, 120–123 CrossRef CAS.
- J. Zhang, C. Li, L. Li, X. Du, B. Song and H. Xu, Multi-shaped amorphous alloy Ni-B: Ultrasonically aided complexing-reduction preparation, catalytic ability for NaBH4 hydrolysis yielding H2 gas, Z. Anorg. Allg. Chem., 2014, 640, 456–461 CrossRef CAS.
- Z. Liang, Q. Li, F. Li, S. Zhao and X. Xia, Hydrogen generation from hydrolysis of NaBH4 based on high stable NiB/NiFe2O4 catalyst, Int. J. Hydrogen Energy, 2017, 42, 3971–3980 CrossRef CAS.
- T. N. Tuan, Y. Yi, J. K. Lee and J. Lee, Fe–B catalyst fabricated by hybrid capacitive adsorption–chemical reduction method and its application for hydrogen production from NaBH4 solution, Catal. Today, 2013, 216, 240–245 CrossRef CAS.
- J. D. Ocon, T. N. Tuan, Y. Yi, R. L. de Leon, J. K. Lee and J. Lee, Ultrafast and stable hydrogen generation from sodium borohydride in methanol and water over Fe–B nanoparticles, J. Power Sources, 2013, 243, 444–450 CrossRef CAS.
- M. Bekirogullari, Catalytic activities of non-noble metal catalysts (Cu-B, Fe-B, and Ni-B) with C.Vulgaris microalgal strain support modified by using phosphoric acid for hydrogen generation from sodium borohydride methanolysis, Int. J. Hydrogen Energy, 2019, 44, 14981–14991 CrossRef CAS.
- K. W. Cho and H. S. Kwon, Effects of electrodeposited Co and Co–P catalysts on the hydrogen generation properties from hydrolysis of alkaline sodium borohydride solution, Catal. Today, 2007, 120, 298–304 CrossRef CAS.
- K. Eom, K. Cho and H. Kwon, Effects of electroless deposition conditions on microstructures of cobalt–phosphorous catalysts and their hydrogen generation properties in alkaline sodium borohydride solution, J. Power Sources, 2008, 180, 484–490 CrossRef CAS.
- X. Zhang, J. Zhao, F. Cheng, J. Liang, Z. Tao and J. Chen, Electroless-deposited Co–P catalysts for hydrogen generation from alkaline NaBH4 solution, Int. J. Hydrogen Energy, 2010, 35, 8363–8369 CrossRef CAS.
- Y. Wang, Y. Shen, K. Qi, Z. Cao, K. Zhang and S. Wu, Nanostructured cobalt–phosphorous catalysts for hydrogen generation from hydrolysis of sodium borohydride solution, Renewable Energy, 2016, 89, 285–294 CrossRef CAS.
- T. Liu, K. Wang, G. Du, A. M. Asiri and X. Sun, Self-supported CoP nanosheet arrays: a non-precious metal catalyst for efficient hydrogen generation from alkaline NaBH4 solution, J. Mater. Chem. A, 2016, 4, 13053–13057 RSC.
- L. Cui, Y. Xu, L. Niu, W. Yang and J. Liu, Monolithically integrated CoP nanowire array: An on/off switch for effective on-demand hydrogen generation via hydrolysis of NaBH4 and NH3BH3, Nano Res., 2016, 10, 595–604 CrossRef.
- Y. Guo, Z. Dong, Z. Cui, X. Zhang and J. Ma, Promoting effect of W doped in electrodeposited Co–P catalysts for hydrogen generation from alkaline NaBH4 solution, Int. J. Hydrogen Energy, 2012, 37, 1577–1583 CrossRef CAS.
- L. Wang, Z. Li, X. Liu, P. Zhang and G. Xie, Hydrogen generation from alkaline NaBH 4 solution using electroless-deposited Co–W–P supported on γ-Al2O3, Int. J. Hydrogen Energy, 2015, 40, 7965–7973 CrossRef CAS.
- Y. Wei, X. Huang, J. Wang, H. Yu, X. Zhao and D. Cheng, Synthesis of bifunctional non-noble monolithic catalyst Co-W-P/carbon cloth for sodium borohydride hydrolysis and reduction of 4-nitrophenol, Int. J. Hydrogen Energy, 2017, 42, 25860–25868 CrossRef CAS.
- D. R. Kim, K. W. Cho, Y. I. Choi and C. J. Park, Fabrication of porous Co–Ni–P catalysts by electrodeposition and their catalytic characteristics for the generation of hydrogen from an alkaline NaBH4 solution, Int. J. Hydrogen Energy, 2009, 34, 2622–2630 CrossRef CAS.
- Y. Wang, G. Li, S. Wu, Y. Wei, W. Meng, Y. Xie, Y. Cui, X. Lian, Y. Chen and X. Zhang, Hydrogen generation from alkaline NaBH4 solution using nanostructured Co–Ni–P catalysts, Int. J. Hydrogen Energy, 2017, 42, 16529–16537 CrossRef CAS.
- Z. Li, L. Wang, Y. Zhang and G. Xie, Properties of Cu Co P/γ-Al2O3 catalysts for efficient hydrogen generation by hydrolysis of alkaline NaBH4 solution, Int. J. Hydrogen Energy, 2017, 42, 5749–5757 CrossRef CAS.
- C. Tang, R. Zhang, W. Lu, L. He, X. Jiang, A. M. Asiri and X. Sun, Fe-Doped CoP nanoarray: A monolithic multifunctional catalyst for highly efficient hydrogen generation, Adv. Mater., 2017, 29, 1602441 CrossRef.
- S. Carenco, D. Portehault, C. Boissiere, N. Mezailles and C. Sanchez, Nanoscaled metal borides and phosphides: Recent developments and perspectives, Chem. Rev., 2013, 113, 7981–8065 CrossRef CAS.
- A. Staubitz, A. P. Robertson and I. Manners, Ammonia-borane and related compounds as dihydrogen sources, Chem. Rev., 2010, 110, 4079–4124 CrossRef CAS.
- M. Chandra and Q. Xu, A high-performance hydrogen generation system: Transition metal-catalyzed dissociation and hydrolysis of ammonia–borane, J. Power Sources, 2006, 156, 190–194 CrossRef CAS.
- H. L. Jiang and Q. Xu, Catalytic hydrolysis of ammonia borane for chemical hydrogen storage, Catal. Today, 2011, 170, 56–63 CrossRef CAS.
- O. T. Summerscales and J. C. Gordon, Regeneration of ammonia borane from spent fuel materials, Dalton Trans., 2013, 42, 10075–10084 RSC.
- H. L. Jiang, T. Umegaki, T. Akita, X. B. Zhang, M. Haruta and Q. Xu, Bimetallic Au-Ni nanoparticles embedded in SiO2 nanospheres: Synergetic catalysis in hydrolytic dehydrogenation of ammonia borane, Chem. – Eur. J., 2010, 16, 3132–3137 CrossRef CAS.
- Y. Z. Chen, L. Liang, Q. Yang, M. Hong, Q. Xu, S. H. Yu and H. L. Jiang, A seed-mediated approach to the general and mild synthesis of non-noble metal nanoparticles stabilized by a metal–organic framework for highly efficient catalysis, Mater. Horiz., 2015, 2, 606–612 RSC.
- Q. Yang, Y. Z. Chen, Z. U. Wang, Q. Xu and H. L. Jiang, One-pot tandem catalysis over Pd@MIL-101: boosting the efficiency of nitro compound hydrogenation by coupling with ammonia borane dehydrogenation, Chem. Commun., 2015, 51, 10419–10422 RSC.
- X. Ma, Y. X. Zhou, H. Liu, Y. Li and H. L. Jiang, A MOF-derived Co-CoO@N-doped porous carbon for efficient tandem catalysis: dehydrogenation of ammonia borane and hydrogenation of nitro compounds, Chem. Commun., 2016, 52, 7719–7722 RSC.
- Y. H. Zhou, Q. Yang, Y. Z. Chen and H. L. Jiang, Low-cost CuNi@MIL-101 as an excellent catalyst toward cascade reaction: integration of ammonia borane dehydrogenation with nitroarene hydrogenation, Chem. Commun., 2017, 53, 12361–12364 RSC.
- A. Boddien, B. Loges, F. Gartner, C. Torborg, K. Fumino, H. Junge, R. Ludwig and M. Beller, Iron-catalyzed hydrogen production from formic acid, J. Am. Chem. Soc., 2010, 132, 8924–8934 CrossRef CAS.
- M. C. Denney, V. Pons, T. J. Hebden, D. M. Heinekey and K. I. Goldberg, Efficient catalysis of ammonia borane dehydrogenation, J. Am. Chem. Soc., 2006, 128, 12048–12049 CrossRef CAS.
- A. Paul and C. B. Musgrave, Catalyzed dehydrogenation of ammonia-borane by iridium dihydrogen pincer complex differs from ethane dehydrogenation, Angew. Chem., Int. Ed., 2007, 46, 8153–8156 CrossRef CAS.
- N. Blaquiere, S. Diallo-Garcia, S. I. Gorelsky, D. A. Black and K. Fagnou, Ruthenium-catalyzed dehydrogenation of ammonia boranes, J. Am. Chem. Soc., 2008, 130, 14034–14035 CrossRef CAS.
- P. V. Ramachandran and P. D. Gagare, Preparation of ammonia borane in high yield and purity, methanolysis, and regeneration, Inorg. Chem., 2007, 46, 7810–7817 CrossRef CAS.
- A. Gutowska, L. Y. Li, Y. S. Shin, C. M. M. Wang, X. H. S. Li, J. C. Linehan, R. S. Smith, B. D. Kay, B. Schmid, W. Shaw, M. Gutowski and T. Autrey, Nanoscaffold mediates hydrogen release and the reactivity of ammonia borane, Angew. Chem., Int. Ed., 2005, 44, 3578–3582 CrossRef CAS.
- M. E. Bluhm, M. G. Bradley, R. Butterick III, U. Kusari and L. G. Sneddon, Amineborane-based chemical hydrogen storage: Enhanced ammonia borane dehydrogenation in ionic liquids, J. Am. Chem. Soc., 2006, 128, 7748–7749 CrossRef CAS.
- L. Li, X. Yao, C. Sun, A. Du, L. Cheng, Z. Zhu, C. Yu, J. Zou, S. C. Smith, P. Wang, H. M. Cheng, R. L. Frost and G. Q. Lu, Lithium-catalyzed dehydrogenation of ammonia borane within mesoporous carbon framework for chemical hydrogen storage, Adv. Funct. Mater., 2009, 19, 265–271 CrossRef CAS.
- J. Huang, F. Zhu, W. He, F. Zhang, W. Wang and H. Li, Periodic mesoporous organometallic silicas with unary or binary organometals inside the channel walls as active and reusable catalysts in aqueous organic reactions, J. Am. Chem. Soc., 2010, 132, 1492–1493 CrossRef CAS.
- L. Wang, H. Li, W. Zhang, X. Zhao, J. Qiu, A. Li, X. Zheng, Z. Hu, R. Si and J. Zeng, Supported rhodium catalysts for ammonia-borane hydrolysis: Dependence of the catalytic activity on the highest occupied state of the single rhodium atoms, Angew. Chem., Int. Ed., 2017, 56, 4712–4718 CrossRef CAS.
- H. Yan, Y. Lin, H. Wu, W. Zhang, Z. Sun, H. Cheng, W. Liu, C. Wang, J. Li, X. Huang, T. Yao, J. Yang, S. Wei and J. Lu, Bottom-up precise synthesis of stable platinum dimers on graphene, Nat. Commun., 2017, 8, 1070 CrossRef.
- Q. Sun, N. Wang, T. Zhang, R. Bai, A. Mayoral, P. Zhang, Q. Zhang, O. Terasaki and J. Yu, Zeolite-encaged single-atom rhodium catalysts: Highly-efficient hydrogen generation and shape-selective tandem hydrogenation of nitroarenes, Angew. Chem., Int. Ed., 2019, 58, 18570–18576 CrossRef CAS.
- Q. L. Yao, W. Shi, G. Feng, Z. H. Lu, X. Zhang, D. Tao, D. Kong and X. Chen, Ultrafine Ru nanoparticles embedded in SiO2 nanospheres: Highly efficient catalysts for hydrolytic dehydrogenation of ammonia borane, J. Power Sources, 2014, 257, 293–299 CrossRef CAS.
- Q. L. Yao, Z. H. Lu, Y. Hu and X. Chen, Core–shell Co@SiO2 nanosphere immobilized Ag nanoparticles for hydrogen evolution from ammonia borane, RSC Adv., 2016, 6, 89450–89456 RSC.
- Q. L. Yao, Y. Shi, X. Zhang, X. Chen and Z. H. Lu, Facile Synthesis of Platinum-cerium(IV) oxide hybrids arched on reduced graphene oxide catalyst in reverse micelles with high activity and durability for hydrolysis of ammonia borane, Chem. – Asian J., 2016, 11, 3251–3257 CrossRef CAS.
- Q. L. Zhu and Q. Xu, Liquid organic and inorganic chemical hydrides for high-capacity hydrogen storage, Energy Environ. Sci., 2015, 8, 478–512 RSC.
- Z. H. Lu, Q. L. Yao, Z. Zhang, Y. Yang and X. Chen, Nanocatalysts for hydrogen generation from ammonia borane and hydrazine borane, J. Nanomater., 2014, 729027 Search PubMed.
- Q. L. Yao, X. Chen and Z. H. Lu, Catalytic dehydrogenation of NH3BH3, N2H4, and N2H4BH3 for chemical hydrogen storage, Energy Environ. Focus, 2014, 3, 236–245 CrossRef.
- C. Y. Alpaydın, S. K. Gülbay and C. O. Colpan, A review on the catalysts used for hydrogen production from ammonia borane, Int. J. Hydrogen Energy, 2020, 45, 3414–3434 CrossRef.
- M. Zahmakiran and S. Özkar, Transition Metal nanoparticles in catalysis for the hydrogen generation from the hydrolysis of ammonia-borane, Top. Catal., 2013, 56, 1171–1183 CrossRef CAS.
- Q. Xu and M. Chandra, Catalytic activities of non-noble metals for hydrogen generation from aqueous ammonia–borane at room temperature, J. Power Sources, 2006, 163, 364–370 CrossRef CAS.
- J. M. Yan, X. B. Zhang, S. Han, H. Shioyama and Q. Xu, Iron-nanoparticle-catalyzed hydrolytic dehydrogenation of ammonia borane for chemical hydrogen storage, Angew. Chem., Int. Ed., 2008, 47, 2287–2289 CrossRef CAS.
- J. M. Yan, X. B. Zhang, H. Shioyama and Q. Xu, Room temperature hydrolytic dehydrogenation of ammonia borane catalyzed by Co nanoparticles, J. Power Sources, 2010, 195, 1091–1094 CrossRef CAS.
- T. Umegaki, J. M. Yan, X. B. Zhang, H. Shioyama, N. Kuriyama and Q. Xu, Preparation and catalysis of poly(N-vinyl-2-pyrrolidone) (PVP) stabilized nickel catalyst for hydrolytic dehydrogenation of ammonia borane, Int. J. Hydrogen Energy, 2009, 34, 3816–3822 CrossRef CAS.
- K. Aranishi, Q. L. Zhu and Q. Xu, Dendrimer-encapsulated cobalt nanoparticles as high-performance catalysts for the hydrolysis of ammonia aorane, ChemCatChem, 2014, 6, 1375–1379 CAS.
- S. Duan, G. Han, Y. Su, X. Zhang, Y. Liu, X. Wu and B. Li, Magnetic Co@g-C3N4 Core-shells on rGO sheets for momentum transfer with catalytic activity toward continuous-flow hydrogen generation, Langmuir, 2016, 32, 6272–6281 CrossRef CAS.
- J. Hu, Z. Chen, M. Li, X. Zhou and H. Lu, Amine-capped Co nanoparticles for highly efficient dehydrogenation of ammonia borane, ACS Appl. Mater. Interfaces, 2014, 6, 13191–13200 CrossRef CAS.
- Y. Men, J. Su, C. Huang, L. Liang, P. Cai, G. Cheng and W. Luo, Three-dimensional nitrogen-doped graphene hydrogel supported Co-CeOx nanoclusters as efficient catalysts for hydrogen generation from hydrolysis of ammonia borane, Chin. Chem. Lett., 2018, 29, 1671–1674 CrossRef CAS.
- M. Chen, R. Xiong, X. Cui, Q. Wang and X. Liu, SiO2-encompassed Co@N-doped porous carbon assemblies as recyclable catalysts for efficient hydrolysis of ammonia borane, Langmuir, 2019, 35, 671–677 CrossRef CAS.
- S. L. Zacho, J. Mielby and S. Kegnaes, Hydrolytic dehydrogenation of ammonia borane over ZIF-67 derived Co nanoparticle catalysts, Catal. Sci. Technol., 2018, 8, 4741–4746 RSC.
- X. Zhao, D. Ke, S. Han, Y. Li, H. Zhang and Y. Cai, Reduced graphene oxide sheets supported waxberry-like Co catalysts for improved hydrolytic dehydrogenation of ammonia borane, ChemistrySelect, 2019, 4, 2513–2518 CrossRef CAS.
- Y. W. Yang, G. Feng, Z. H. Lu, H. Na, F. Zhang and X. S. Chen, In situ synthesis of reduced graphene oxide supported Co nanoparticles as efficient catalysts for hydrogen generation from NH3BH3, Acta Phys. – Chim. Sin., 2014, 30, 1180–1186 CAS.
- A. Aijaz, A. Karkamkar, Y. J. Choi, N. Tsumori, E. Ronnebro, T. Autrey, H. Shioyama and Q. Xu, Immobilizing highly catalytically active Pt nanoparticles inside the pores of metal-organic framework: a double solvents approach, J. Am. Chem. Soc., 2012, 134, 13926–13929 CrossRef CAS.
- P. Liu, X. Gu, K. Kang, H. Zhang, J. Cheng and H. Su, Highly efficient catalytic hydrogen evolution from ammonia borane using the synergistic effect of crystallinity and size of noble-metal-free nanoparticles supported by porous metal-organic frameworks, ACS Appl. Mater. Interfaces, 2017, 9, 10759–10767 CrossRef CAS.
- Y. Z. Chen, Q. Xu, S. H. Yu and H. L. Jiang, Tiny Pd@Co core-shell nanoparticles confined inside a metal-organic framework for highly efficient catalysis, Small, 2015, 11, 71–76 CrossRef CAS.
- Z. Li, T. He, L. Liu, W. Chen, M. Zhang, G. Wu and P. Chen, Covalent triazine framework supported non-noble metal nanoparticles with superior activity for catalytic hydrolysis of ammonia borane: from mechanistic study to catalyst design, Chem. Sci., 2017, 8, 781–788 RSC.
- Y. Fang, Z. Xiao, J. Li, C. Lollar, L. Liu, X. Lian, S. Yuan, S. Banerjee, P. Zhang and H. C. Zhou, Formation of a highly reactive cobalt nanocluster crystal within a highly negatively charged porous coordination cage, Angew. Chem., Int. Ed., 2018, 57, 5283–5287 CrossRef CAS.
- H. Wang, Y. Zhao, F. Cheng, Z. Tao and J. Chen, Cobalt nanoparticles embedded in porous N-doped carbon as long-life catalysts for hydrolysis of ammonia borane, Catal. Sci. Technol., 2016, 6, 3443–3448 RSC.
- L. Zhou, J. Meng, P. Li, Z. Tao, L. Mai and J. Chen, Ultrasmall cobalt nanoparticles supported on nitrogen-doped porous carbon nanowires for hydrogen evolution from ammonia borane, Mater. Horiz., 2017, 4, 268–273 RSC.
- F. Zhang, C. Ma, Y. Zhang, H. Li, D. Fu, X. Du and X.-M. Zhang, N-doped mesoporous carbon embedded Co nanoparticles for highly efficient and stable H2 generation from hydrolysis of ammonia borane, J. Power Sources, 2018, 399, 89–97 CrossRef CAS.
- X. L. Zhang, D. X. Zhang, G. G. Chang, X. C. Ma, J. Wu, Y. Wang, H. Z. Yu, G. Tian, J. Chen and X. Y. Yang, Bimetallic (Zn/Co) MOFs-derived highly dispersed metallic Co/HPC for completely hydrolytic dehydrogenation of ammonia–borane, Ind. Eng. Chem. Res., 2019, 58, 7209–7216 CrossRef CAS.
- Ö. Metin, V. Mazumder, S. Özkar and S. Sun, Monodisperse nickel nanoparticles and their catalysis in hydrolytic dehydrogenation of ammonia borane, J. Am. Chem. Soc., 2010, 132, 1468–1469 CrossRef.
- Y. Li, L. Xie, Y. Li, J. Zheng and X. Li, Metal-organic-framework-based catalyst for highly efficient H2 generation from aqueous NH3BH3 solution, Chemistry, 2009, 15, 8951–8954 CrossRef CAS.
- A. Nozaki, Y. Tanihara, Y. Kuwahara, T. Ohmichi, K. Mori, T. Nagase, H. Y. Yasuda and H. Yamashita, Skeletal Ni catalysts prepared from amorphous Ni-Zr alloys: Enhanced catalytic performance for hydrogen generation from ammonia borane, ChemPhysChem, 2016, 17, 412–417 CrossRef CAS.
- J. Manna, S. Akbayrak and S. Özkar, Nickel(0) nanoparticles supported on bare or coated cobalt ferrite as highly active, magnetically isolable and reusable catalyst for hydrolytic dehydrogenation of ammonia borane, J. Colloid Interface Sci., 2017, 508, 359–368 CrossRef CAS.
- K. Guo, H. Li and Z. Yu, Size-dependent catalytic activity of monodispersed nickel nanoparticles for the hydrolytic dehydrogenation of ammonia borane, ACS Appl. Mater. Interfaces, 2018, 10, 517–525 CrossRef CAS.
- Ö. Metin, S. Özkar and S. Sun, Monodisperse nickel nanoparticles supported on SiO2 as an effective catalyst for the hydrolysis of ammonia-borane, Nano Res., 2010, 3, 676–684 CrossRef.
- P. Z. Li, A. Aijaz and Q. Xu, Highly dispersed surfactant-free nickel nanoparticles and their remarkable catalytic activity in the hydrolysis of ammonia borane for hydrogen generation, Angew. Chem., Int. Ed., 2012, 51, 6753–6756 CrossRef CAS.
- P. Z. Li, K. Aranishi and Q. Xu, ZIF-8 immobilized nickel nanoparticles: highly effective catalysts for hydrogen generation from hydrolysis of ammonia borane, Chem. Commun., 2012, 48, 3173–3175 RSC.
- C. Wang, J. Tuninetti, Z. Wang, C. Zhang, R. Ciganda, L. Salmon, S. Moya, J. Ruiz and D. Astruc, Hydrolysis of Ammonia-borane over Ni/ZIF-8 nanocatalyst: High efficiency, mechanism, and controlled hydrogen release, J. Am. Chem. Soc., 2017, 139, 11610–11615 CrossRef CAS.
- G. Zhao, J. Zhong, J. Wang, T. K. Sham, X. Sun and S. T. Lee, Revealing the synergetic effects in Ni nanoparticle-carbon nanotube hybrids by scanning transmission X-ray microscopy and their application in the hydrolysis of ammonia borane, Nanoscale, 2015, 7, 9715–9722 RSC.
- J. Zhang, C. Chen, W. Yan, F. Duan, B. Zhang, Z. Gao and Y. Qin, Ni nanoparticles supported on CNTs with excellent activity produced by atomic layer deposition for hydrogen generation from the hydrolysis of ammonia borane, Catal. Sci. Technol., 2016, 6, 2112–2119 RSC.
- M. Mahyari and A. Shaabani, Nickel nanoparticles immobilized on three-dimensional nitrogen-doped graphene as a superb catalyst for the generation of hydrogen from the hydrolysis of ammonia borane, J. Mater. Chem. A, 2014, 2, 16652–16659 RSC.
- K. Yang, Q. L. Yao, W. Huang, X. Chen and Z. H. Lu, Enhanced catalytic activity of NiM (M=Cr, Mo, W) nanoparticles for hydrogen evolution from ammonia borane and hydrazine borane, Int. J. Hydrogen Energy, 2017, 42, 6840–6850 CrossRef CAS.
- Q. L. Yao, Z. H. Lu, W. Huang, X. Chen and J. Zhu, High Pt-like activity of the Ni–Mo/graphene catalyst for hydrogen evolution from hydrolysis of ammonia borane, J. Mater. Chem. A, 2016, 4, 8579–8583 RSC.
- K. Yang, Q. L. Yao, Z. H. Lu, Z. B. Kang and X. S. Chen, Facile synthesis of CuMo nanoparticles as highly active and cost-effective catalysts for the hydrolysis of ammonia borane, Acta Phys. – Chim. Sin., 2017, 33, 993–1000 CAS.
- Q. L. Yao, K. Yang, X. Hong, X. Chen and Z. H. Lu, Base-promoted hydrolytic dehydrogenation of ammonia borane catalyzed by noble-metal-free nanoparticles, Catal. Sci. Technol., 2018, 8, 870–877 RSC.
- Q. L. Yao, M. He, X. Hong, X. Chen, G. Feng and Z. H. Lu, Hydrogen production via selective dehydrogenation of hydrazine borane and hydrous hydrazine over MoOx-promoted Rh catalyst, Int. J. Hydrogen Energy, 2019, 44, 28430–28440 CrossRef CAS.
- Q. L. Yao, M. He, X. Hong, X. Zhang and Z. H. Lu, MoOx-modified bimetallic alloy nanoparticles for highly efficient hydrogen production from hydrous hydrazine, Inorg. Chem. Front., 2019, 6, 1546–1552 RSC.
- Q. L. Yao, K. Yang, W. Nie, Y. Li and Z. H. Lu, Highly efficient hydrogen generation from hydrazine borane via a MoOx-promoted NiPd nanocatalyst, Renewable Energy, 2020, 147, 2024–2031 CrossRef CAS.
- Q. L. Yao, Z. H. Lu, Y. Yang, Y. Chen, X. Chen and H. L. Jiang, Facile synthesis of graphene-supported Ni-CeOx nanocomposites as highly efficient catalysts for hydrolytic dehydrogenation of ammonia borane, Nano Res., 2018, 11, 4412–4422 CrossRef CAS.
- Y. H. Zhou, S. Wang, Y. Wan, J. Liang, Y. Chen, S. Luo and C. Yong, Low-cost CuNi-CeO2/rGO as an efficient catalyst for hydrolysis of ammonia borane and tandem reduction of 4-nitrophenol, J. Alloys Compd., 2017, 728, 902–909 CrossRef CAS.
- Y. W. Yang, Z. H. Lu and X. S. Chen, Cu-based nanocatalysts for hydrogen generation via hydrolysis and methanolysis of ammonia borane, Mater. Technol., 2015, 30, A89–A93 CrossRef CAS.
- O. Ozay, E. Inger, N. Aktas and N. Sahiner, Hydrogen production from ammonia borane via hydrogel template synthesized Cu, Ni, Co composites, Int. J. Hydrogen Energy, 2011, 36, 8209–8216 CrossRef CAS.
- S. B. Kalidindi, U. Sanyal and B. R. Jagirdar, Nanostructured Cu and Cu@Cu2O core shell catalysts for hydrogen generation from ammonia-borane, Phys. Chem. Chem. Phys., 2008, 10, 5870–5874 RSC.
- Y. Yamada, K. Yano, Q. Xu and S. Fukuzumi, Cu/Co3O4 nanoparticles as catalysts for hydrogen evolution from ammonia borane by hydrolysis, J. Phys. Chem. C, 2010, 114, 16456–16462 CrossRef CAS.
- Y. Yamada, K. Yano and S. Fukuzumi, Catalytic application of shape-controlled Cu2O particles protected by Co3O4 nanoparticles for hydrogen evolution from ammonia borane, Energy Environ. Sci., 2012, 5, 5356–5363 RSC.
- M. Zahmakıran, F. Durap and S. Özkar, Zeolite confined copper(0) nanoclusters as cost-effective and reusable catalyst in hydrogen generation from the hydrolysis of ammonia-borane, Int. J. Hydrogen Energy, 2010, 35, 187–197 CrossRef.
- M. Kaya, M. Zahmakiran, S. Özkar and M. Volkan, Copper(0) nanoparticles supported on silica-coated cobalt ferrite magnetic particles: cost effective catalyst in the hydrolysis of ammonia-borane with an exceptional reusability performance, ACS Appl. Mater. Interfaces, 2012, 4, 3866–3873 CrossRef CAS.
- Y. Yang, Z. H. Lu, Y. Hu, Z. Zhang, W. Shi, X. Chen and T. Wang, Facile in situ synthesis of copper nanoparticles supported on reduced graphene oxide for hydrolytic dehydrogenation of ammonia borane, RSC Adv., 2014, 4, 13749–13752 RSC.
- Q. L. Yao, Z. H. Lu, Z. Zhang, X. Chen and Y. Lan, One-pot synthesis of core-shell Cu@SiO2 nanospheres and their catalysis for hydrolytic dehydrogenation of ammonia borane and hydrazine borane, Sci. Rep., 2014, 4, 7597 CrossRef CAS.
- J. Zhang, Y. Wang, Y. Zhu, G. Mi, X. Du and Y. Dong, Shape-selective fabrication of Cu nanostructures: Contrastive study of catalytic ability for hydrolytically releasing H2 from ammonia borane, Renewable Energy, 2018, 118, 146–151 CrossRef CAS.
- J. X. Kang, T. W. Chen, D. F. Zhang and L. Guo, PtNiAu trimetallic nanoalloys enabled by a digestive-assisted process as highly efficient catalyst for hydrogen generation, Nano Energy, 2016, 23, 145–152 CrossRef CAS.
- K. Yang, L. Zhou, X. Xiong, M. Ye, L. Li and Q. Xia, RuCuCo nanoparticles supported on MIL-101 as a novel highly efficient catalysts for the hydrolysis of ammonia borane, Microporous Mesoporous Mater., 2016, 225, 1–8 CrossRef CAS.
- D. Ke, Y. Li, J. Wang, L. Zhang, J. Wang, X. Zhao, S. Yang and S. Han, Facile fabrication of poly(amidoamine) (PAMAM) dendrimers-encapsulated Ag–Co bimetallic nanoparticles for highly efficient dehydrogenation of ammonia borane, Int. J. Hydrogen Energy, 2016, 41, 2564–2574 CrossRef CAS.
- Q. Wang, F. Fu, S. Yang, M. M. Moro, M. d. l. A. Ramirez, S. Moya, L. Salmon, J. Ruiz and D. Astruc, Dramatic synergy in CoPt nanocatalysts stabilized by “Click” dendrimers for evolution of hydrogen from hydrolysis of ammonia borane, ACS Catal., 2018, 9, 1110–1119 CrossRef.
- J. M. Yan, X. B. Zhang, S. Han, H. Shioyama and Q. Xu, Magnetically recyclable Fe–Ni alloy catalyzed dehydrogenation of ammonia borane in aqueous solution under ambient atmosphere, J. Power Sources, 2009, 194, 478–481 CrossRef CAS.
- X. Zhou, Z. Chen, D. Yan and H. Lu, Deposition of Fe–Ni nanoparticles on polyethyleneimine-decorated graphene oxide and application in catalytic dehydrogenation of ammonia borane, J. Mater. Chem., 2012, 22, 13506–13516 RSC.
- S. W. Lai, H. L. Lin, Y. P. Lin and T. L. Yu, Hydrolysis of ammonia–borane catalyzed by an iron–nickel alloy on an SBA-15 support, Int. J. Hydrogen Energy, 2013, 38, 4636–4647 CrossRef CAS.
- C. Cui, Y. Liu, S. Mehdi, H. Wen, B. Zhou, J. Li and B. Li, Enhancing effect of Fe-doping on the activity of nano Ni catalyst towards hydrogen evolution from NH3BH3, Appl. Catal., B, 2020, 265, 118612 CrossRef.
- F. Qiu, L. Li, G. Liu, Y. Wang, C. An, C. Xu, Y. Xu, Y. Wang, L. Jiao and H. Yuan, Synthesis of Fe0.3Co0.7/rGO nanoparticles as a high performance catalyst for the hydrolytic dehydrogenation of ammonia borane, Int. J. Hydrogen Energy, 2013, 38, 7291–7297 CrossRef CAS.
- F. Qiu, L. Li, G. Liu, Y. Wang, Y. Wang, C. An, Y. Xu, C. Xu, Y. Wang, L. Jiao and H. Yuan, In situ synthesized Fe–Co/C nano-alloys as catalysts for the hydrolysis of ammonia borane, Int. J. Hydrogen Energy, 2013, 38, 3241–3249 CrossRef CAS.
- F. Y. Qiu, Y. J. Wang, Y. P. Wang, L. Li, G. Liu, C. Yan, L. F. Jiao and H. T. Yuan, Dehydrogenation of ammonia borane catalyzed by in situ synthesized Fe–Co nano-alloy in aqueous solution, Catal. Today, 2011, 170, 64–68 CrossRef CAS.
- S. Zhou, M. Wen, N. Wang, Q. Wu, Q. Wu and L. Cheng, Highly active NiCo alloy hexagonal nanoplates with crystal plane selective dehydrogenation and visible-light photocatalysis, J. Mater. Chem., 2012, 22, 16858–16864 RSC.
- Q. Wang, Z. Zhang, J. Liu, R. Liu and T. Liu, Bimetallic non-noble CoNi nanoparticles monodispersed on multiwall carbon nanotubes: Highly efficient hydrolysis of ammonia borane, Mater. Chem. Phys., 2018, 204, 58–61 CrossRef CAS.
- Z. H. Lu, J. Li, A. Zhu, Q. L. Yao, W. Huang, R. Zhou, R. Zhou and X. Chen, Catalytic hydrolysis of ammonia borane via magnetically recyclable copper iron nanoparticles for chemical hydrogen storage, Int. J. Hydrogen Energy, 2013, 38, 5330–5337 CrossRef CAS.
- J. M. Yan, Z. L. Wang, H. L. Wang and Q. Jiang, Rapid and energy-efficient synthesis of a graphene–CuCo hybrid as a high performance catalyst, J. Mater. Chem., 2012, 22, 10990–10993 RSC.
- F. Z. Song, Q. L. Zhu, X. C. Yang and Q. Xu, Monodispersed CuCo Nanoparticles supported on diamine-functionalized graphene as a non-noble metal catalyst for hydrolytic dehydrogenation of ammonia borane, ChemNanoMat, 2016, 2, 942–945 CrossRef CAS.
- Y. Liu, J. Zhang, H. Guan, Y. Zhao, J. H. Yang and B. Zhang, Preparation of bimetallic Cu-Co nanocatalysts on poly (diallyldimethylammonium chloride) functionalized halloysite nanotubes for hydrolytic dehydrogenation of ammonia borane, Appl. Surf. Sci., 2018, 427, 106–113 CrossRef CAS.
- A. Bulut, M. Yurderi, İ. E. Ertas, M. Celebi, M. Kaya and M. Zahmakiran, Carbon dispersed copper-cobalt alloy nanoparticles: A cost-effective heterogeneous catalyst with exceptional performance in the hydrolytic dehydrogenation of ammonia-borane, Appl. Catal., B, 2016, 180, 121–129 CrossRef CAS.
- H. Yen, Y. Seo, S. Kaliaguine and F. Kleitz, Role of Metal–support interactions, particle size, and metal–metal synergy in CuNi nanocatalysts for H2 generation, ACS Catal., 2015, 5, 5505–5511 CrossRef CAS.
- X. Meng, S. Li, B. Xia, L. Yang, N. Cao, J. Su, M. He, W. Luo and G. Cheng, Decoration of graphene with tetrametallic Cu@FeCoNi core–shell nanoparticles for catalytic hydrolysis of amine boranes, RSC Adv., 2014, 4, 32817–32825 RSC.
- D. Gao, Y. Zhang, L. Zhou and K. Yang, CuNi NPs supported on MIL-101 as highly active catalysts for the hydrolysis of ammonia borane, Appl. Surf. Sci., 2018, 427, 114–122 CrossRef CAS.
- S. J. Li, H. L. Wang, J. M. Yan and Q. Jiang, Oleylamine-stabilized Cu0.9Ni0.1 nanoparticles as efficient catalyst for ammonia borane dehydrogenation, Int. J. Hydrogen Energy, 2017, 42, 25251–25257 CrossRef CAS.
- Z. H. Lu, J. Li, G. Feng, Q. L. Yao, F. Zhang, R. Zhou, D. Tao, X. Chen and Z. Yu, Synergistic catalysis of MCM-41 immobilized Cu–Ni nanoparticles in hydrolytic dehydrogeneration of ammonia borane, Int. J. Hydrogen Energy, 2014, 39, 13389–13395 CrossRef CAS.
- K. Guo, Y. Ding, J. Luo, M. Gu and Z. Yu, NiCu Bimetallic Nanoparticles on silica support for catalytic hydrolysis of ammonia borane: Composition-dependent activity and support size effect, ACS Appl. Energy Mater., 2019, 2, 5851–5861 CrossRef CAS.
- Q. L. Yao, Z. H. Lu, Y. Wang, X. Chen and G. Feng, Synergetic catalysis of Non-noble bimetallic Cu–Co nanoparticles embedded in SiO2 nanospheres in hydrolytic dehydrogenation of ammonia borane, J. Phys. Chem. C, 2015, 119, 14167–14174 CrossRef CAS.
- J. Li, Q. L. Zhu and Q. Xu, Non-noble bimetallic CuCo nanoparticles encapsulated in the pores of metal–organic frameworks: synergetic catalysis in the hydrolysis of ammonia borane for hydrogen generation, Catal. Sci. Technol., 2015, 5, 525–530 RSC.
- J. M. Yan, X. B. Zhang, T. Akita, M. Haruta and Q. Xu, One-step seeding growth of magnetically recyclable Au@Co core-shell nanoparticles: highly efficient catalyst for hydrolytic dehydrogenation of ammonia borane, J. Am. Chem. Soc., 2010, 132, 5326–5327 CrossRef CAS.
- H. L. Jiang, T. Akita and Q. Xu, A one-pot protocol for synthesis of non-noble metal-based core-shell nanoparticles under ambient conditions: toward highly active and cost-effective catalysts for hydrolytic dehydrogenation of NH3BH3, Chem. Commun., 2011, 47, 10999–11001 RSC.
- Y. Du, N. Cao, L. Yang, W. Luo and G. Cheng, One-step synthesis of magnetically recyclable rGO supported Cu@Co core–shell nanoparticles: highly efficient catalysts for hydrolytic dehydrogenation of ammonia borane and methylamine borane, New J. Chem., 2013, 37, 3035–3042 RSC.
- J. Zhang, H. Li, H. Zhang, Y. Zhu and G. Mi, Porously hierarchical Cu@Ni cubic-cage microstructure: Very active and durable catalyst for hydrolytically liberating H2 gas from ammonia borane, Renewable Energy, 2016, 99, 1038–1045 CrossRef CAS.
- H. L. Wang, J. M. Yan, Z. L. Wang and Q. Jiang, One-step synthesis of Cu@FeNi core–shell nanoparticles: Highly active catalyst for hydrolytic dehydrogenation of ammonia borane, Int. J. Hydrogen Energy, 2012, 37, 10229–10235 CrossRef CAS.
- F. Qiu, Y. Dai, L. Li, C. Xu, Y. Huang, C. Chen, Y. Wang, L. Jiao and H. Yuan, Synthesis of Cu@FeCo core–shell nanoparticles for the catalytic hydrolysis of ammonia borane, Int. J. Hydrogen Energy, 2014, 39, 436–441 CrossRef CAS.
- X. Meng, L. Yang, N. Cao, C. Du, K. Hu, J. Su, W. Luo and G. Cheng, Graphene-supported trimetallic core-shell Cu@CoNi nanoparticles for catalytic hydrolysis of amine borane, ChemPlusChem, 2014, 79, 325–332 CrossRef CAS.
- H. Zhang, X. Wang, C. Chen, C. An, Y. Xu, Y. Huang, Q. Zhang, Y. Wang, L. Jiao and H. Yuan, Facile synthesis of Cu@CoNi core-shell nanoparticles composites for the catalytic hydrolysis of ammonia borane, Int. J. Hydrogen Energy, 2015, 40, 12253–12261 CrossRef CAS.
- C. Wang, H. Wang, Z. Wang, X. Li, Y. Chi, M. Wang, D. Gao and Z. Zhao, Mo remarkably enhances catalytic activity of Cu@MoCo core-shell nanoparticles for hydrolytic dehydrogenation of ammonia borane, Int. J. Hydrogen Energy, 2018, 43, 7347–7355 CrossRef CAS.
- Q. Liu, S. Zhang, J. Liao, K. Feng, Y. Zheng, B. G. Pollet and H. Li, CuCo2O4 nanoplate film as a low-cost, highly active and durable catalyst towards the hydrolytic dehydrogenation of ammonia borane for hydrogen production, J. Power Sources, 2017, 355, 191–198 CrossRef CAS.
- J. Liao, H. Li, X. Zhang, K. Feng and Y. Yao, Fabrication of a Ti-supported NiCo2O4 nanosheet array and its superior catalytic performance in the hydrolysis of ammonia borane for hydrogen generation, Catal. Sci. Technol., 2016, 6, 3893–3899 RSC.
- K. Feng, J. Zhong, B. Zhao, H. Zhang, L. Xu, X. Sun and S. T. Lee, CuxCo1−xO nanoparticles on graphene oxide as a aynergistic catalyst for high-efficiency hydrolysis of ammonia-borane, Angew. Chem., Int. Ed., 2016, 55, 11950–11954 CrossRef CAS.
- J. Li, X. Ren, H. Lv, Y. Wang, Y. Li and B. Liu, Highly efficient hydrogen production from hydrolysis of ammonia borane over nanostructured Cu@CuCoOx supported on graphene oxide, J. Hazard. Mater., 2020, 391, 122199 CrossRef CAS.
- Q. Liu, S. Zhang, J. Liao, X. Huang, Y. Zheng and H. Li, MnCo2O4 film composed of nanoplates: synthesis, characterization and its superior catalytic performance in the hydrolytic dehydrogenation of ammonia borane, Catal. Sci. Technol., 2017, 7, 3573–3579 RSC.
- J. Liao, D. Lu, G. Diao, X. Zhang, M. Zhao and H. Li, Co0.8Cu0.2MoO4 microspheres composed of nanoplatelets as a robust catalyst for the hydrolysis of ammonia borane, ACS Sustainable Chem. Eng., 2018, 6, 5843–5851 CrossRef CAS.
- D. Lu, J. Liao, Y. Leng, S. Zhong, J. He, H. Wang, R. Wang and H. Li, Mo-doped Cu0.5Ni0.5Co2O4 nanowires, a strong substitute for noble-metal-based catalysts towards the hydrolysis of ammonia borane for hydrogen production, Catal. Commun., 2018, 114, 89–92 CrossRef CAS.
- D. Lu, J. Liao, H. Li, S. Ji and B. G. Pollet, Co3O4/CuMoO4 hybrid microflowers composed of nanorods with rich particle boundaries as a highly active catalyst for ammonia borane Hydrolysis, ACS Sustainable Chem. Eng., 2019, 7, 16474–16482 CrossRef CAS.
- J. Liao, Y. Feng, W. Lin, X. Su, S. Ji, L. Li, W. Zhang, B. G. Pollet and H. Li, CuO–NiO/Co3O4 hybrid nanoplates as highly active catalyst for ammonia borane hydrolysis, Int. J. Hydrogen Energy, 2020, 45, 8168–8176 CrossRef CAS.
- D. Lu, J. Li, C. Lin, J. Liao, Y. Feng, Z. Ding, Z. Li, Q. Liu and H. Li, A Simple and scalable route to synthesize CoxCu1−xCo2O4@CoyCu1−yCo2O4 yolk-shell microspheres, A high-performance catalyst to hydrolyze ammonia borane for hydrogen production, Small, 2019, 15, e1805460 CrossRef.
- R. Fernandes, N. Patel, A. Miotello, R. Jaiswal and D. C. Kothari, Dehydrogenation of ammonia borane with transition metal-doped Co–B alloy catalysts, Int. J. Hydrogen Energy, 2012, 37, 2397–2406 CrossRef CAS.
- A. K. Figen and B. Coşkuner, A novel perspective for hydrogen generation from ammonia borane (NH3BH3) with Co–B catalysts: “Ultrasonic hydrolysis”, Int. J. Hydrogen Energy, 2013, 38, 2824–2835 CrossRef.
- K. Eom, K. Cho and H. Kwon, Hydrogen generation from hydrolysis of NH3BH3 by an electroplated Co–P catalyst, Int. J. Hydrogen Energy, 2010, 35, 181–186 CrossRef CAS.
- C. Y. Peng, L. Kang, S. Cao, Y. Chen, Z. S. Lin and W. F. Fu, Nanostructured Ni2P as a robust catalyst for the hydrolytic dehydrogenation of ammonia-borane, Angew. Chem., Int. Ed., 2015, 54, 15725–15729 CrossRef CAS.
- C. Tang, L. Xie, K. Wang, G. Du, A. M. Asiri, Y. Luo and X. Sun, A Ni2P nanosheet array integrated on 3D Ni foam: an efficient, robust and reusable monolithic catalyst for the hydrolytic dehydrogenation of ammonia borane toward on-demand hydrogen generation, J. Mater. Chem. A, 2016, 4, 12407–12410 RSC.
- C. Tang, F. Qu, A. M. Asiri, Y. Luo and X. Sun, CoP nanoarray: a robust non-noble-metal hydrogen-generating catalyst toward effective hydrolysis of ammonia borane, Inorg. Chem. Front., 2017, 4, 659–662 RSC.
- X. Qu, R. Jiang, Q. Li, F. Zeng, X. Zheng, Z. Xu, C. Chen and J. Peng, The hydrolysis of ammonia borane catalyzed by NiCoP/OPC-300 nanocatalysts: high selectivity and efficiency, and mechanism, Green Chem., 2019, 21, 850–860 RSC.
- N. Patel, R. Fernandes, G. Guella and A. Miotello, Nanoparticle-assembled Co-B thin film for the hydrolysis of ammonia borane: A highly active catalyst for hydrogen production, Appl. Catal., B, 2010, 95, 137–143 CrossRef CAS.
- N. Patel, R. Fernandes, S. Gupta, R. Edla, D. C. Kothari and A. Miotello, Co-B catalyst supported over mesoporous silica for hydrogen production by catalytic hydrolysis of Ammonia Borane: A study on influence of pore structure, Appl. Catal., B, 2013, 140–141, 125–132 CrossRef CAS.
- Y. Zou, J. Cheng, Q. Wang, C. Xiang, H. Chu, S. Qiu, H. Zhang, F. Xu, S. Liu, C. Tang and L. Sun, Cobalt–boron/nickel–boron nanocomposite with improved catalytic performance for the hydrolysis of ammonia borane, Int. J. Hydrogen Energy, 2015, 40, 13423–13430 CrossRef CAS.
- H. Wang, D. Gao, L. Wang, Y. Chi, M. Wang, Y. Gu, C. Wang and Z. Zhao, Highly dispersed surfactant-free amorphous NiCoB nanoparticles and their remarkable catalytic activity for hydrogen generation from ammonia borane dehydrogenation, Catal. Lett., 2018, 148, 1739–1749 CrossRef CAS.
- Z. C. Fu, Y. Xu, S. L. Chan, W. W. Wang, F. Li, F. Liang, Y. Chen, Z. S. Lin, W. F. Fu and C. M. Che, Highly efficient hydrolysis of ammonia borane by anion OH−, F−, Cl−-tuned interactions between reactant molecules and CoP nanoparticles, Chem. Commun., 2017, 53, 705–708 RSC.
- C. C. Hou, Q. Li, C. J. Wang, C. Y. Peng, Q. Q. Chen, H. F. Ye, W. F. Fu, C. M. Che, N. López and Y. Chen, Ternary Ni–Co–P nanoparticles as noble-metal-free catalysts to boost the hydrolytic dehydrogenation of ammonia-borane, Energy Environ. Sci., 2017, 10, 1770–1776 RSC.
- X. Yang, F. Cheng, Z. Tao and J. Chen, Hydrolytic dehydrogenation of ammonia borane catalyzed by carbon supported Co core–Pt shell nanoparticles, J. Power Sources, 2011, 196, 2785–2789 CrossRef CAS.
- W. Y. Chen, D. L. Li, Z. J. Wang, G. Qian, Z. J. Sui, X. Z. Duan, X. G. Zhou, I. Yeboah and D. Chen, Reaction mechanism and kinetics for hydrolytic dehydrogenation of ammonia borane on a Pt/CNT catalyst, AIChE J., 2017, 63, 60–65 CrossRef CAS.
- D. Sun, V. Mazumder, Ö. Metin and S. Sun, Methanolysis of ammonia baorane by CoPd nanoparticles, ACS Catal., 2012, 2, 1290–1295 CrossRef CAS.
- D. Özhava, N. Z. Kılıçaslan and S. Özkar, PVP-stabilized nickel(0) nanoparticles as catalyst in hydrogen generation from the methanolysis of hydrazine borane or ammonia borane, Appl. Catal., B, 2015, 162, 573–582 CrossRef.
- D. Özhava and S. Özkar, Rhodium(0) nanoparticles supported on nanosilica: Highly active and long lived catalyst in hydrogen generation from the methanolysis of ammonia borane, Appl. Catal., B, 2016, 181, 716–726 CrossRef.
- Y. Fang, J. Li, T. Togo, F. Jin, Z. Xiao, L. Liu, H. Drake, X. Lian and H. C. Zhou, Ultra-small face-centered-cubic Ru nanoparticles confined within a porous coordination cage for dehydrogenation, Chem, 2018, 4, 555–563 CAS.
- A. K. Figen, Improved catalytic performance of metal oxide catalysts fabricated with electrospinning in ammonia borane methanolysis for hydrogen production, Int. J. Hydrogen Energy, 2019, 44, 28451–28462 CrossRef.
- E. G. Sogut, H. Acidereli, E. Kuyuldar, Y. Karatas, M. Gulcan and F. Sen, Single-walled carbon nanotube supported Pt-Ru bimetallic superb nanocatalyst for the hydrogen generation from the methanolysis of methylamine-borane at mild conditions, Sci. Rep., 2019, 9, 15724 CrossRef.
- S. E. Korkut, H. Kucukkececi and Ö. Metin, Mesoporous graphitic carbon nitride/black phosphorus/AgPd alloy nanoparticles ternary nanocomposite: A highly efficient catalyst for the methanolysis of ammonia borane, ACS Appl. Mater. Interfaces, 2020, 12, 8130–8139 CrossRef.
- X. Li, C. Zhang, M. Luo, Q. L. Yao and Z. H. Lu, Ultrafine Rh nanoparticles confined by nitrogen-rich covalent organic frameworks for methanolysis of ammonia borane, Inorg. Chem. Front., 2020, 7, 1298–1306 RSC.
- K. O. Amoo, E. N. Onyeozili, E. E. Kalu, J. A. Omoleye and V. E. Efeovbokhan, Activity of varying compositions of Co–Ni–P catalysts for the methanolysis of ammonia borane, Int. J. Hydrogen Energy, 2016, 41, 21221–21235 CrossRef CAS.
- J. Zhang, Y. Duan, Y. Zhu, Y. Wang, H. Yao and G. Mi, Evenly dispersed microspherical amorphous alloy Co x B 1-x : Robust and magnetically recyclable catalyst for alcoholyzing ammonia borane to release H2, Mater. Chem. Phys., 2017, 201, 297–301 CrossRef CAS.
- S. B. Kalidindi, A. A. Vernekar and B. R. Jagirdar, Co-Co2B, Ni-Ni3B and Co-Ni-B nanocomposites catalyzed ammonia-borane methanolysis for hydrogen generation, Phys. Chem. Chem. Phys., 2009, 11, 770–775 RSC.
- Q. L. Yao, M. Huang, Z. H. Lu, Y. Yang, Y. Zhang, X. Chen and Z. Yang, Methanolysis of ammonia borane by shape-controlled mesoporous copper nanostructures for hydrogen generation, Dalton Trans., 2015, 44, 1070–1076 RSC.
- M. Yurderi, A. Bulut, I. E. Ertas, M. Zahmakiran and M. Kaya, Supported copper-copper oxide nanoparticles as active, stable and low-cost catalyst in the methanolysis of ammonia-borane for chemical hydrogen storage, Appl. Catal., B, 2015, 165, 169–175 CrossRef CAS.
- L. Cui, X. Cao, X. Sun, W. Yang and J. Liu, A Bunch-like copper oxide nanowire array as an efficient, durable, and economical catalyst for the methanolysis of ammonia borane, ChemCatChem, 2018, 10, 710–715 CrossRef CAS.
- C. Yu, J. Fu, M. Muzzio, T. Shen, D. Su, J. Zhu and S. Sun, CuNi nanoparticles
assembled on graphene for catalytic methanolysis of ammonia borane and hydrogenation of nitro/nitrile compounds, Chem. Mater., 2017, 29, 1413–1418 CrossRef CAS.
- Q. Q. Chen, Q. Li, C. C. Hou, C. J. Wang, C. Y. Peng, N. López and Y. Chen, Enhancing electrostatic interactions to activate polar molecules: ammonia borane methanolysis on a Cu/Co(OH)2 nanohybrid, Catal. Sci. Technol., 2019, 9, 2828–2835 RSC.
- “Nitrogen (Fixed)–Ammonia”, U.S. Geological Survey, Mineral Commodity Summaries, 2017.
- L. Greenjr, An ammonia energy vector for the hydrogen economy, Int. J. Hydrogen Energy, 1982, 7, 355–359 CrossRef.
- S. F. Yin, B. Q. Xu, X. P. Zhou and C. T. Au, A mini-review on ammonia decomposition catalysts for on-site generation of hydrogen for fuel cell applications, Appl. Catal., A, 2004, 277, 1–9 CrossRef CAS.
- A. Klerke, C. H. Christensen, J. K. Nørskov and T. Vegge, Ammonia for hydrogen storage: challenges and opportunities, J. Mater. Chem., 2008, 18, 2304–2310 RSC.
- F. Schüth, R. Palkovits, R. Schlögl and D. S. Su, Ammonia as a possible element in an energy infrastructure: catalysts for ammonia decomposition, Energy Environ. Sci., 2012, 5, 6278–6289 RSC.
- C. H. Christensen, T. Johannessen, R. Z. Sørensen and J. K. Nørskov, Towards an ammonia-mediated hydrogen economy?, Catal. Today, 2006, 111, 140–144 CrossRef CAS.
- D. A. Hansgen, D. G. Vlachos and J. G. Chen, Using first principles to predict bimetallic catalysts for the ammonia decomposition reaction, Nat. Chem., 2010, 2, 484–489 CrossRef CAS.
- A. Srifa, K. Okura, T. Okanishi, H. Muroyama, T. Matsui and K. Eguchi, COx-free hydrogen production via ammonia decomposition over molybdenum nitride-based catalysts, Catal. Sci. Technol., 2016, 6, 7495–7504 RSC.
- T. Umegaki, J. M. Yan, X. B. Zhang, H. Shioyama, N. Kuriyama and Q. Xu, Boron- and nitrogen-based chemical hydrogen storage materials, Int. J. Hydrogen Energy, 2009, 34, 2303–2311 CrossRef CAS.
- K. Hashimoto and N. Toukai, Decomposition of ammonia over a catalyst consisting of ruthenium metal and cerium oxides supported on Y-form zeolite, J. Mol. Catal. A: Chem., 2000, 161, 171–178 CrossRef CAS.
- A. Boisen, S. Dahl, J. Norskov and C. Christensen, Why the optimal ammonia synthesis catalyst is not the optimal ammonia decomposition catalyst, J. Catal., 2005, 230, 309–312 CrossRef CAS.
- C. J. Jacobsen, S. Dahl, B. S. Clausen, S. Bahn, A. Logadottir and J. K. Norskov, Catalyst design by interpolation in the periodic table: Bimetallic ammonia synthesis catalysts, J. Am. Chem. Soc., 2001, 123, 8404–8405 CrossRef CAS.
- W. Raróg-Pilecka, Ammonia decomposition over the carbon-based ruthenium catalyst promoted with barium or cesium, J. Catal., 2003, 218, 465–469 CrossRef.
- S. F. Yin, B. Q. Xu, C. F. Ng and C. T. Au, Nano Ru/CNTs: a highly active and stable catalyst for the generation of CO -free hydrogen in ammonia decomposition, Appl. Catal., B, 2004, 48, 237–241 CrossRef CAS.
- L. Li, Z. H. Zhu, Z. F. Yan, G. Q. Lu and L. Rintoul, Catalytic ammonia decomposition over Ru/carbon catalysts: The importance of the structure of carbon support, Appl. Catal., A, 2007, 320, 166–172 CrossRef CAS.
- Z. Wang, Z. Cai and Z. Wei, Highly Active Ruthenium catalyst supported on barium hexaaluminate for ammonia decomposition to COx-free hydrogen, ACS Sustainable Chem. Eng., 2019, 7, 8226–8235 CrossRef CAS.
- X. C. Hu, X. P. Fu, W. W. Wang, X. Wang, K. Wu, R. Si, C. Ma, C. J. Jia and C. H. Yan, Ceria-supported ruthenium clusters transforming from isolated single atoms for hydrogen production via decomposition of ammonia, Appl. Catal., B, 2020, 268, 118424 CrossRef.
- Z. Kowalczyk, J. Sentek, S. Jodzis, M. Muhler and O. Hinrichsen, Effect of potassium on the kinetics of ammonia synthesis and decomposition over fused iron catalyst at atmospheric pressure, J. Catal., 1997, 169, 407–414 CrossRef CAS.
- X. Duan, J. Ji, G. Qian, C. Fan, Y. Zhu, X. Zhou, D. Chen and W. Yuan, Ammonia decomposition on Fe(110), Co(111) and Ni(111) surfaces: A density functional theory study, J. Mol. Catal. A: Chem., 2012, 357, 81–86 CrossRef CAS.
- D. Rein, K. F. Ortega, C. Weidenthaler, E. Bill and M. Behrens, The roles of Co-precipitation pH, phase-purity and alloy formation for the ammonia decomposition activity of Ga-promoted Fe/MgO catalysts, Appl. Catal., A, 2017, 548, 52–61 CrossRef CAS.
- Z. P. Hu, L. Chen, C. Chen and Z. Y. Yuan, Fe/ZSM-5 catalysts for ammonia decomposition to COx-free hydrogen: effect of SiO2/Al2O3 ratio, Mol. Catal., 2018, 455, 14–22 CrossRef CAS.
- Z. P. Hu, L. Chen, C. C. Weng and Z. Y. Yuan, Fe nanocatalysts supported on dealuminated ZSM-5 for efficient decomposition of ammonia to COx-free Hydrogen, ChemistrySelect, 2018, 3, 4439–4447 CrossRef CAS.
- Y. Q. Gu, Z. Jin, H. Zhang, R. J. Xu, M. J. Zheng, Y. M. Guo, Q. S. Song and C. J. Jia, Transition metal nanoparticles dispersed in an alumina matrix as active and stable catalysts for COx-free hydrogen production from ammonia, J. Mater. Chem. A, 2015, 3, 17172–17180 RSC.
- X. Duan, G. Qian, X. Zhou, Z. Sui, D. Chen and W. Yuan, Tuning the size and shape of Fe nanoparticles on carbon nanofibers for catalytic ammonia decomposition, Appl. Catal., B, 2011, 101, 189–196 CrossRef CAS.
- Z. P. Hu, C. C. Weng, C. Chen and Z. Y. Yuan, Two-dimensional mica nanosheets supported Fe nanoparticles for NH3 decomposition to hydrogen, Mol. Catal., 2018, 448, 162–170 CrossRef CAS.
- A. H. Lu, J. J. Nitz, M. Comotti, C. Weidenthaler, K. Schlichte, C. W. Lehmann, O. Terasaki and F. Schuth, Spatially and size selective synthesis of Fe-based nanoparticles on ordered mesoporous supports as highly active and stable catalysts for ammonia decomposition, J. Am. Chem. Soc., 2010, 132, 14152–14162 CrossRef CAS.
- Y. Li, S. Liu, L. Yao, W. Ji and C. T. Au, Core-shell structured iron nanoparticles for the generation of COx-free hydrogen via ammonia decomposition, Catal. Commun., 2010, 11, 368–372 CrossRef CAS.
- D. Varisli, C. Korkusuz and T. Dogu, Microwave-assisted ammonia decomposition reaction over iron incorporated mesoporous carbon catalysts, Appl. Catal., B, 2017, 201, 370–380 CrossRef CAS.
- J. C. Ganley, F. S. Thomas, E. G. Seebauer and R. I. Masel, A priori catalytic activity correlations: the difficult case of hydrogen production from ammonia, Catal. Lett., 2004, 96, 117–122 CrossRef CAS.
- J. Zhang, H. Xu, X. Jin, Q. Ge and W. Li, Characterizations and activities of the nano-sized Ni/Al2O3 and Ni/La–Al2O3 catalysts for NH3 decomposition, Appl. Catal., A, 2005, 290, 87–96 CrossRef CAS.
- Z. P. Hu, C. C. Weng, C. Chen and Z. Y. Yuan, Catalytic decomposition of ammonia to COx-free hydrogen over Ni/ZSM-5 catalysts: A comparative study of the preparation methods, Appl. Catal., A, 2018, 562, 49–57 CrossRef CAS.
- D. Sima, H. Wu, K. Tian, S. Xie, J. J. Foo, S. Li, D. Wang, Y. Ye, Z. Zheng and Y.-Q. Liu, Enhanced low temperature catalytic activity of Ni/Al–Ce0.8Zr0.2O2 for hydrogen production from ammonia decomposition, Int. J. Hydrogen Energy, 2020, 45, 9342–9352 CrossRef CAS.
- Y. Yu, Y.-M. Gan, C. Huang, Z. H. Lu, X. Wang, R. Zhang and G. Feng, Ni/La2O3 and Ni/MgO–La2O3 catalysts for the decomposition of NH3 into hydrogen, Int. J. Hydrogen Energy, 2020, 45, 16528–16539 CrossRef CAS.
- W. Zheng, J. Zhang, Q. Ge, H. Xu and W. Li, Effects of CeO2 addition on Ni/Al2O3 catalysts for the reaction of ammonia decomposition to hydrogen, Appl. Catal., B, 2008, 80, 98–105 CrossRef CAS.
- H. Liu, H. Wang, J. Shen, Y. Sun and Z. Liu, Promotion effect of cerium and lanthanum oxides on Ni/SBA-15 catalyst for ammonia decomposition, Catal. Today, 2008, 131, 444–449 CrossRef CAS.
- K. Okura, T. Okanishi, H. Muroyama, T. Matsui and K. Eguchi, Ammonia decomposition over nickel catalysts supported on rare-earth oxides for the on-site generation of hydrogen, ChemCatChem, 2016, 8, 2988–2995 CrossRef CAS.
- K. Okura, T. Okanishi, H. Muroyama, T. Matsui and K. Eguchi, Additive effect of alkaline earth metals on ammonia decomposition reaction over Ni/Y2O3 catalysts, RSC Adv., 2016, 6, 85142–85148 RSC.
- I. Lucentini, I. Serrano, L. Soler, N. J. Divins and J. Llorca, Ammonia decomposition over 3D-printed CeO2 structures loaded with Ni, Appl. Catal., A, 2020, 591, 117382 CrossRef.
- J. Zhang, M. Comotti, F. Schüth, R. Schlögl and D. S. Su, Commercial Fe- or Co-containing carbon nanotubes as catalysts for NH3 decomposition, Chem. Commun., 2007, 1916–1918 RSC.
- H. Zhang, Y. A. Alhamed, Y. Kojima, A. A. Al-Zahrani and L. A. Petrov, Cobalt supported on carbon nanotubes. An efficient catalyst for ammonia decomposition, C. R. Acad. Bulg. Sci., 2013, 66, 519–524 CAS.
- H. Zhang, Y. A. Alhamed, W. Chu, Z. Ye, A. AlZahrani and L. Petrov, Controlling Co-support interaction in Co/MWCNTs catalysts and catalytic performance for hydrogen production via NH3 decomposition, Appl. Catal., A, 2013, 464–465, 156–164 CrossRef CAS.
- L. Li, R. Jiang, W. Chu, H. Cang, H. Chen and J. Yan, Cobalt nanoparticles embedded in a porous carbon matrix as an efficient catalyst for ammonia decomposition, Catal. Sci. Technol., 2017, 7, 1363–1371 RSC.
- S. Podila, Y. A. Alhamed, A. A. AlZahrani and L. A. Petrov, Hydrogen production by ammonia decomposition using Co catalyst supported on Mg mixed oxide systems, Int. J. Hydrogen Energy, 2015, 40, 15411–15422 CrossRef CAS.
- S. Podila, H. Driss, S. F. Zaman, A. M. Ali, A. A. Al-Zahrani, M. A. Daous and L. A. Petrov, Effect of preparation methods on the catalyst performance of Co/Mg La mixed oxide catalyst for COx-free hydrogen production by ammonia decomposition, Int. J. Hydrogen Energy, 2017, 42, 24213–24221 CrossRef CAS.
- X. C. Hu, W. W. Wang, Y. Q. Gu, Z. Jin, Q. S. Song and C. J. Jia, Ammonia decomposition for COx-free hydrogen production over Co-SiO2 nanocomposite catalysts, ChemPlusChem, 2017, 82, 368–375 CrossRef CAS.
- X. Duan, G. Qian, X. Zhou, D. Chen and W. Yuan, MCM-41 supported CoMo bimetallic catalysts for enhanced hydrogen production by ammonia decomposition, Chem. Eng. J., 2012, 207–208, 103–108 CrossRef CAS.
- J. Ji, X. Duan, G. Qian, X. Zhou, G. Tong and W. Yuan, Towards an efficient CoMo/γ-Al2O3 catalyst using metal amine metallate as an active phase precursor: Enhanced hydrogen production by ammonia decomposition, Int. J. Hydrogen Energy, 2014, 39, 12490–12498 CrossRef CAS.
- X. Duan, J. Ji, X. Yan, G. Qian, D. Chen and X. Zhou, Understanding Co-Mo catalyzed ammonia decomposition: influence of calcination atmosphere and identification of active phase, ChemCatChem, 2016, 8, 938–945 CrossRef CAS.
- B. Lorenzut, T. Montini, M. Bevilacqua and P. Fornasiero, FeMo-based catalysts for H2 production by NH3 decomposition, Appl. Catal., B, 2012, 125, 409–417 CrossRef CAS.
- J. Zhang, J. O. Muller, W. Zheng, D. Wang, D. Su and R. Schlogl, Individual Fe-Co alloy nanoparticles on carbon nanotubes: structural and catalytic properties, Nano Lett., 2008, 8, 2738–2743 CrossRef CAS.
- Z. Lendzion-Bieluń and W. Arabczyk, Fused FeCo catalysts for hydrogen production by means of the ammonia decomposition reaction, Catal. Today, 2013, 212, 215–219 CrossRef.
- Z. Lendzion-Bieluń, R. Pelka and Ł. Czekajło, Characterization of FeCo based catalyst for ammonia decomposition. The effect of potassium oxide, Pol. J. Chem. Technol., 2014, 16, 111–116 Search PubMed.
- L. Huo, B. Liu, H. Li, B. Cao, X. C. Hu, X. P. Fu, C. Jia and J. Zhang, Component synergy and armor protection induced superior catalytic activity and stability of ultrathin Co-Fe spinel nanosheets confined in mesoporous silica shells for ammonia decomposition reaction, Appl. Catal., B, 2019, 253, 121–130 CrossRef CAS.
- S. B. Simonsen, D. Chakraborty, I. Chorkendorff and S. Dahl, Alloyed Ni-Fe nanoparticles as catalysts for NH3 decomposition, Appl. Catal., A, 2012, 447–448, 22–31 CrossRef CAS.
- H. Silva, M. G. Nielsen, E. M. Fiordaliso, C. D. Damsgaard, C. Gundlach, T. Kasama, I. B. Chorkendorff and D. Chakraborty, Synthesis and characterization of Fe–Ni/É£-Al2O3 egg-shell catalyst for H2 generation by ammonia decomposition, Appl. Catal., A, 2015, 505, 548–556 CrossRef CAS.
- Y. Yi, L. Wang, Y. Guo, S. Sun and H. Guo, Plasma–assisted ammonia decomposition over Fe–Ni alloy catalysts for COx–free hydrogen, AIChE J., 2019, 65, 691–701 CAS.
- C. Huang, H. Li, J. Yang, C. Wang, F. Hu, X. Wang, Z. H. Lu, G. Feng and R. Zhang, Ce0.6Zr0.3Y0.1O2 solid solutions-supported Ni Co bimetal nanocatalysts for NH3 decomposition, Appl. Surf. Sci., 2019, 478, 708–716 CrossRef CAS.
- S. S. Pansare, W. Torres and J. G. Goodwin, Ammonia decomposition on tungsten carbide, Catal. Commun., 2007, 8, 649–654 CrossRef CAS.
- X. Cui, H. Li, L. Guo, D. He, H. Chen and J. Shi, Synthesis of mesoporous tungsten carbide by an impregnation-compaction route, and its NH3 decomposition catalytic activity, Dalton Trans., 2008, 6435–6440 RSC.
- A. Kraupner, A. Markus, R. Palkovits, K. Schlicht and C. Giordano, Mesoporous Fe3C sponges as magnetic supports and as heterogeneous catalyst, J. Mater. Chem., 2010, 20, 6019–6022 RSC.
- J. C. Ingersoll, N. Mani, J. C. Thenmozhiyal and A. Muthaiah, Catalytic hydrolysis of sodium borohydride by a novel nickel–cobalt–boride catalyst, J. Power Sources, 2007, 173, 450–457 CrossRef CAS.
- J. G. Choi, Synthesis and catalytic properties of porous Ta carbide crystallites for hydrogen production from the decomposition of ammonia, J. Porous Mater., 2013, 20, 1059–1068 CrossRef CAS.
- W. Zheng, T. P. Cotter, P. Kaghazchi, T. Jacob, B. Frank, K. Schlichte, W. Zhang, D. S. Su, F. Schuth and R. Schlogl, Experimental and theoretical investigation of molybdenum carbide and nitride as catalysts for ammonia decomposition, J. Am. Chem. Soc., 2013, 135, 3458–3464 CrossRef CAS.
- C. Liang, W. Li, Z. Wei, Q. Xin and C. Li, Catalytic Decomposition of ammonia over nitrided MoNx/α-Al2O3 and NiMoNy/α-Al2O3 catalysts, Ind. Eng. Chem. Res., 2000, 39, 3694–3697 CrossRef CAS.
- D. V. Leybo, A. N. Baiguzhina, D. S. Muratov, D. I. Arkhipov, E. A. Kolesnikov, V. V. Levina, N. I. Kosova and D. V. Kuznetsov, Effects of composition and production route on structure and catalytic activity for ammonia decomposition reaction of ternary Ni–Mo nitride catalysts, Int. J. Hydrogen Energy, 2016, 41, 3854–3860 CrossRef CAS.
- S. Podila, S. F. Zaman, H. Driss, A. A. Al-Zahrani, M. A. Daous and L. A. Petrov, High performance of bulk Mo2N and Co3Mo3N catalysts for hydrogen production from ammonia: Role of citric acid to Mo molar ratio in preparation of high surface area nitride catalysts, Int. J. Hydrogen Energy, 2017, 42, 8006–8020 CrossRef CAS.
- A. Srifa, K. Okura, T. Okanishi, H. Muroyama, T. Matsui and K. Eguchi, Hydrogen production by ammonia decomposition over Cs-modified Co3Mo3N catalysts, Appl. Catal., B, 2017, 218, 1–8 CrossRef CAS.
- L. A. Jolaoso, S. F. Zaman, S. Podila, H. Driss, A. A. Al-Zahrani, M. A. Daous and L. Petrov, Ammonia decomposition over citric acid induced γ-Mo2N and Co3Mo3N catalysts, Int. J. Hydrogen Energy, 2018, 43, 4839–4844 CrossRef CAS.
- S. F. Zaman, L. A. Jolaoso, S. Podila, A. A. Al-Zahrani, Y. A. Alhamed, H. Driss, M. M. Daous and L. Petrov, Ammonia decomposition over citric acid chelated γ-Mo2N and Ni2Mo3N catalysts, Int. J. Hydrogen Energy, 2018, 43, 17252–17258 CrossRef CAS.
- P. X. Zhang, Y. G. Wang, Y. Q. Huang, T. Zhang, G. S. Wu and J. Li, Density functional theory investigations on the catalytic mechanisms of hydrazine decompositions on Ir(111), Catal. Today, 2011, 165, 80–88 CrossRef CAS.
- H. Gu, R. Ran, W. Zhou, Z. Shao, W. Jin, N. Xu and J. Ahn, Solid-oxide fuel cell operated on in situ catalytic decomposition products of liquid hydrazine, J. Power Sources, 2008, 177, 323–329 CrossRef CAS.
- M. Zheng, X. Chen, R. Cheng, N. Li, J. Sun, X. Wang and T. Zhang, Catalytic decomposition of hydrazine on iron nitride catalysts, Catal. Commun., 2006, 7, 187–191 CrossRef CAS.
- M. Zheng, R. Cheng, X. Chen, N. Li, L. Li, X. Wang and T. Zhang, A novel approach for CO-free H production via catalytic decomposition of hydrazine, Int. J. Hydrogen Energy, 2005, 30, 1081–1089 CrossRef CAS.
- J. Santos, Catalytic Decomposition of hydrazine on tungsten carbide: The Influence of adsorbed oxygen, J. Catal., 2002, 210, 1–6 CrossRef CAS.
- X. Chen, T. Zhang, P. Ying, M. Zheng, W. Wu, L. Xia, T. Li, X. Wang and C. Li, A novel catalyst for hydrazine decomposition: molybdenum carbide support on γ-Al2O3, Chem. Commun., 2002, 288–289 RSC.
- X. W. Chen, T. Zhang, L. G. Xia, T. Li, M. Y. Zheng, Z. L. Wu, X. D. Wang, Z. B. Wei, Q. Xin and C. Li, Catalytic decomposition of hydrazine over supported molybdenum nitride catalysts in a monopropellant thruster, Catal. Lett., 2002, 79, 21–25 CrossRef CAS.
- L. Ding, Y. Shu, A. Wang, M. Zheng, L. Li, X. Wang and T. Zhang, Preparation and catalytic performances of ternary phosphides NiCoP for hydrazine decomposition, Appl. Catal., A, 2010, 385, 232–237 CrossRef CAS.
- S. K. Singh and Q. Xu, Nanocatalysts for hydrogen generation from hydrazine, Catal. Sci. Technol., 2013, 3, 1889 RSC.
- D. Bhattacharjee and S. Dasgupta, Trimetallic NiFePd nanoalloy catalysed hydrogen generation from alkaline hydrous hydrazine and sodium borohydride at room temperature, J. Mater. Chem. A, 2015, 3, 24371–24378 RSC.
- Y. P. Qiu, Q. Shi, L. L. Zhou, M. H. Chen, C. Chen, P. P. Tang, G. S. Walker and P. Wang, NiPt nanoparticles anchored onto hierarchical nanoporous N-doped carbon as an efficient catalyst for hydrogen generation from hydrazine monohydrate, ACS Appl. Mater. Interfaces, 2020, 12, 18617–18624 CrossRef CAS.
- A. Kumar, X. Yang and Q. Xu, Ultrafine bimetallic Pt–Ni nanoparticles immobilized on 3-dimensional N-doped graphene networks: a highly efficient catalyst for dehydrogenation of hydrous hydrazine, J. Mater. Chem. A, 2019, 7, 112–115 RSC.
- F. Z. Song, X. Yang and Q. Xu, Ultrafine bimetallic Pt–Ni nanoparticles achieved by metal–organic framework templated zirconia/porous carbon/reduced graphene oxide: remarkable catalytic activity in dehydrogenation of hydrous hydrazine, Small Methods, 2019, 4, 1900707 CrossRef.
- H. L. Wang, J. M. Yan, Z. L. Wang, O. Song-Il and Q. Jiang, Highly efficient hydrogen generation from hydrous hydrazine over amorphous Ni0.9Pt0.1/Ce2O3 nanocatalyst at room temperature, J. Mater. Chem. A, 2013, 1, 14957–14962 RSC.
- X. Lu, S. Francis, D. Motta, N. Dimitratos and A. Roldan, Mechanistic study of hydrazine decomposition on Ir(111), Phys. Chem. Chem. Phys., 2020, 22, 3883–3896 RSC.
- H. Wang, L. Wu, Y. Wang, X. Li and Y. Wang, Facile synthesis of Ni nanoparticles from triangular Ni(HCO3)2 nanosheets as catalysts for hydrogen generation from hydrous hydrazine, Catal. Commun., 2017, 100, 33–37 CrossRef CAS.
- T. Liu, J. Yu, H. Bie and Z. Hao, Highly efficient hydrogen generation from hydrous hydrazine using a reduced graphene oxide-supported NiPtP nanoparticle catalyst, J. Alloys Compd., 2017, 690, 783–790 CrossRef CAS.
- S. K. Singh, X. B. Zhang and Q. Xu, Room-temperature hydrogen generation from hydrous hydrazine for chemical hydrogen storage, J. Am. Chem. Soc., 2009, 131, 9894–9895 CrossRef CAS.
- L. He, Y. Huang, A. Wang, X. Wang and T. Zhang, H2 production by selective decomposition of hydrous hydrazine over Raney Ni catalyst under ambient conditions, AIChE J., 2013, 59, 4297–4302 CrossRef.
- L. He, B. Liang, L. Li, X. Yang, Y. Huang, A. Wang, X. Wang and T. Zhang, Cerium-oxide-modified nickel as a non-noble metal catalyst for selective decomposition of hydrous hydrazine to hydrogen, ACS Catal., 2015, 5, 1623–1628 CrossRef CAS.
- Q. Fu, P. Yang, J. Wang, H. Wang, L. Yang and X. Zhao, In situ synthesis of Ni nanofibers via vacuum thermal reduction and their efficient catalytic properties for hydrogen generation, J. Mater. Chem. A, 2018, 6, 11370–11376 RSC.
- H. Yin, Y. P. Qiu, H. Dai, L. Y. Gan, H. B. Dai and P. Wang, Understanding of selective H2 generation from hydrazine decomposition on Ni(111) surface, J. Phys. Chem. C, 2018, 122, 5443–5451 CrossRef CAS.
- N. Cao, L. Yang, C. Du, J. Su, W. Luo and G. Cheng, Highly efficient dehydrogenation of hydrazine over graphene supported flower-like Ni–Pt nanoclusters at room temperature, J. Mater. Chem. A, 2014, 2, 14344–14347 RSC.
- H. Ma, H. Wang and C. Na, Microwave-assisted optimization of platinum-nickel nanoalloys for catalytic water treatment, Appl. Catal., B, 2015, 163, 198–204 CrossRef CAS.
- C. Wan, L. Sun, L. Xu, D. G. Cheng, F. Chen, X. Zhan and Y. Yang, Novel NiPt alloy nanoparticle decorated 2D layered g-C3N4 nanosheets: a highly efficient catalyst for hydrogen generation from hydrous hydrazine, J. Mater. Chem. A, 2019, 7, 8798–8804 RSC.
- M. Zhang, L. Liu, S. Lu, L. Xu, Y. An and C. Wan, Facile fabrication of NiPt/CNTs as an efficient catalyst for hydrogen production from hydrous hydrazine, ChemistrySelect, 2019, 4, 10494–10500 CrossRef CAS.
- F. Guo, H. Zou, Q. L. Yao, B. Huang and Z. H. Lu, Monodispersed bimetallic nanoparticles anchored on TiO2-decorated titanium carbide MXene for efficient hydrogen production from hydrazine in aqueous solution, Renewable Energy, 2020, 155, 1293–1301 CrossRef CAS.
- J. Wang, X. B. Zhang, Z. L. Wang, L. M. Wang and Y. Zhang, Rhodium–nickel nanoparticles grown on graphene as highly efficient catalyst for complete decomposition of hydrous hydrazine at room temperature for chemical hydrogen storage, Energy Environ. Sci., 2012, 5, 6885–6888 RSC.
- J. Wang, W. Li, Y. Wen, L. Gu and Y. Zhang, Rh-Ni-B Nanoparticles as highly efficient catalysts for hydrogen generation from hydrous hydrazine, Adv. Energy Mater., 2015, 5, 1401879 CrossRef.
- J. B. Yoo, H. S. Kim, S. H. Kang, B. Lee and N. H. Hur, Hollow nickel-coated silica microspheres containing rhodium nanoparticles for highly selective production of hydrogen from hydrous hydrazine, J. Mater. Chem. A, 2014, 2, 18929–18937 RSC.
- P. Zhao, N. Cao, W. Luo and G. Cheng, Nanoscale MIL-101 supported RhNi nanoparticles: an efficient catalyst for hydrogen generation from hydrous hydrazine, J. Mater. Chem. A, 2015, 3, 12468–12475 RSC.
- J. Chen, Q. L. Yao, J. Zhu, X. Chen and Z. H. Lu, Rh–Ni nanoparticles immobilized on Ce(OH)CO3 nanorods as highly efficient catalysts for hydrogen generation from alkaline solution of hydrazine, Int. J. Hydrogen Energy, 2016, 41, 3946–3954 CrossRef CAS.
- R. Jiang, X. Qu, F. Zeng, Q. Li, X. Zheng, Z. Xu and J. Peng, MOF-74-immobilized ternary Rh-Ni-P nanoparticles as highly efficient hydrous hydrazine dehydrogenation catalysts in alkaline solutions, Int. J. Hydrogen Energy, 2019, 44, 6383–6391 CrossRef CAS.
- L. He, Y. Huang, X. Y. Liu, L. Li, A. Wang, X. Wang, C.-Y. Mou and T. Zhang, Structural and catalytic properties of supported Ni–Ir alloy catalysts for H2 generation via hydrous hydrazine decomposition, Appl. Catal., B, 2014, 147, 779–788 CrossRef CAS.
- Y. P. Qiu, H. Yin, H. Dai, L. Y. Gan, H. B. Dai and P. Wang, Tuning the surface composition of Ni/meso-CeO2 with iridium as an efficient catalyst for hydrogen generation from hydrous hydrazine, Chemistry, 2018, 24, 4902–4908 CrossRef CAS.
- D. Bhattacharjee, K. Mandal and S. Dasgupta, High performance nickel–palladium nanocatalyst for hydrogen generation from alkaline hydrous hydrazine at room temperature, J. Power Sources, 2015, 287, 96–99 CrossRef CAS.
- Y. Chen, L. Wang, Y. Zhai, H. Chen, Y. Dou, J. Li, H. Zheng and R. Cao, Pd–Ni nanoparticles supported on reduced graphene oxides as catalysts for hydrogen generation from hydrazine, RSC Adv., 2017, 7, 32310–32315 RSC.
- O. Song-Il, J. M. Yan, H. L. Wang, Z. L. Wang and Q. Jiang, High catalytic kinetic performance of amorphous CoPt NPs induced on CeOx for H2 generation from hydrous hydrazine, Int. J. Hydrogen Energy, 2014, 39, 3755–3761 CrossRef CAS.
- K. Wang, Q. L. Yao, S. Qing and Z. H. Lu, La(OH)3 nanosheet-supported CoPt nanoparticles: a highly efficient and magnetically recyclable catalyst for hydrogen production from hydrazine in aqueous solution, J. Mater. Chem. A, 2019, 7, 9903–9911 RSC.
- N. Firdous, N. K. Janjua, I. Qazi and M. H. S. Wattoo, Optimal
Co–Ir bimetallic catalysts supported on γ-Al2O3 for hydrogen generation from hydrous hydrazine, Int. J. Hydrogen Energy, 2016, 41, 984–995 CrossRef CAS.
- X. Du, P. Cai, W. Luo and G. Cheng, Facile synthesis of P-doped Rh nanoparticles with superior catalytic activity toward dehydrogenation of hydrous hydrazine, Int. J. Hydrogen Energy, 2017, 42, 6137–6143 CrossRef CAS.
- S. K. Singh, A. K. Singh, K. Aranishi and Q. Xu, Noble-metal-free bimetallic nanoparticle-catalyzed selective hydrogen generation from hydrous hydrazine for chemical hydrogen storage, J. Am. Chem. Soc., 2011, 133, 19638–19641 CrossRef CAS.
- W. Gao, C. Li, H. Chen, M. Wu, S. He, M. Wei, D. G. Evans and X. Duan, Supported nickel–iron nanocomposites as a bifunctional catalyst towards hydrogen generation from N2H4·H2O, Green Chem., 2014, 16, 1560–1568 RSC.
- J. Chen, H. Zou, Q. L. Yao, M. Luo, X. Li and Z. H. Lu, Cr2O3-modified NiFe nanoparticles as a noble-metal-free catalyst for complete dehydrogenation of hydrazine in aqueous solution, Appl. Surf. Sci., 2020, 501, 144247 CrossRef CAS.
- W. Kang, H. Guo and A. Varma, Noble-metal-free NiCu/CeO2 catalysts for H2 generation from hydrous hydrazine, Appl. Catal., B, 2019, 249, 54–62 CrossRef CAS.
- H. Wang, Q. Fu, G. Zhang and Y. Sun, The synthesis of Ni–Cu alloy nanofibers via vacuum thermal Co-reduction toward hydrogen generation from hydrazine decomposition, Catal. Lett., 2018, 149, 77–83 CrossRef.
- D. Wu, M. Wen, X. Lin, Q. Wu, C. Gu and H. Chen, A NiCo/NiO–CoOx ultrathin layered catalyst with strong basic sites for high-performance H2 generation from hydrous hydrazine, J. Mater. Chem. A, 2016, 4, 6595–6602 RSC.
- K. V. Manukyan, A. Cross, S. Rouvimov, J. Miller, A. S. Mukasyan and E. E. Wolf, Low temperature decomposition of hydrous hydrazine over FeNi/Cu nanoparticles, Appl. Catal., A, 2014, 476, 47–53 CrossRef CAS.
- J. Wang, Y. Li and Y. Zhang, Precious-metal-free nanocatalysts for highly efficient hydrogen production from hydrous hydrazine, Adv. Funct. Mater., 2014, 24, 7073–7077 CAS.
- J. Zhang, Q. Kang, Z. Yang, H. Dai, D. Zhuang and P. Wang, A cost-effective NiMoB–La(OH)3 catalyst for hydrogen generation from decomposition of alkaline hydrous hydrazine solution, J. Mater. Chem. A, 2013, 1, 11623–11628 RSC.
- H. L. Wang, J. M. Yan, S. J. Li, X. W. Zhang and Q. Jiang, Noble-metal-free NiFeMo nanocatalyst for hydrogen generation from the decomposition of hydrous hydrazine, J. Mater. Chem. A, 2015, 3, 121–124 RSC.
- Q. L. Yao, Z. H. Lu, R. Zhang, S. Zhang, X. Chen and H. L. Jiang, A noble-metal-free nanocatalyst for highly efficient and complete hydrogen evolution from N2H4BH3, J. Mater. Chem. A, 2018, 6, 4386–4393 RSC.
- L. He, Y. Huang, A. Wang, X. Wang, X. Chen, J. J. Delgado and T. Zhang, A noble-metal-free catalyst derived from Ni-Al hydrotalcite for hydrogen generation from N2H4·H2O decomposition, Angew. Chem., Int. Ed., 2012, 51, 6191–6194 CrossRef CAS.
- W. Kang and A. Varma, Hydrogen generation from hydrous hydrazine over Ni/CeO2 catalysts prepared by solution combustion synthesis, Appl. Catal., B, 2018, 220, 409–416 CrossRef CAS.
- M. Huang, Q. L. Yao, G. Feng, H. Zou and Z. H. Lu, Nickel-ceria nanowires embedded in microporous silica: controllable synthesis, formation mechanism, and catalytic applications, Inorg. Chem., 2020, 59, 5781–5790 CrossRef CAS.
- D. Wu, M. Wen, C. Gu and Q. Wu, 2D NiFe/CeO2 basic-site-enhanced catalyst via in situ topotactic reduction for selectively catalyzing the H2 generation from N2H4·H2O, ACS Appl. Mater. Interfaces, 2017, 9, 16103–16108 CrossRef CAS.
- H. Zou, Q. L. Yao, M. Huang, M. Zhu, F. Zhang and Z. H. Lu, Noble-metal-free NiFe nanoparticles immobilized on nano CeZrO2 solid solutions for highly efficient hydrogen production from hydrous hydrazine, Sustainable Energy Fuels, 2019, 3, 3071–3077 RSC.
- H. Zou, F. Guo, M. Luo, Q. L. Yao and Z. H. Lu, La(OH)3-decorated NiFe nanoparticles as efficient catalyst for hydrogen evolution from hydrous hydrazine and hydrazine borane, Int. J. Hydrogen Energy, 2020, 45, 11641–11650 CrossRef CAS.
- Y. Men, X. Du, G. Cheng and W. Luo, CeOx-modified NiFe nanodendrits grown on rGO for efficient catalytic hydrogen generation from alkaline solution of hydrazine, Int. J. Hydrogen Energy, 2017, 42, 27165–27173 CrossRef CAS.
- P. Yang, L. Yang, Q. Gao, Q. Luo, X. Zhao, X. Mai, Q. Fu, M. Dong, J. Wang, Y. Hao, R. Yang, X. Lai, S. Wu, Q. Shao, T. Ding, J. Lin and Z. Guo, Anchoring carbon nanotubes and post-hydroxylation treatment enhanced Ni nanofiber catalysts towards efficient hydrous hydrazine decomposition for effective hydrogen generation, Chem. Commun., 2019, 55, 9011–9014 RSC.
- H. Wang, L. Wu, A. Jia, X. Li, Z. Shi, M. Duan and Y. Wang, Ni nanoparticles encapsulated in the channel of titanate nanotubes: Efficient noble-metal-free catalysts for selective hydrogen generation from hydrous hydrazine, Chem. Eng. J., 2018, 332, 637–646 CrossRef CAS.
- Y. P. Qiu, G. X. Cao, H. Wen, Q. Shi, H. Dai and P. Wang, High-capacity hydrogen generation from hydrazine monohydrate using a noble-metal-free Ni10Mo/Ni–Mo–O nanocatalyst, Int. J. Hydrogen Energy, 2019, 44, 15110–15117 CrossRef CAS.
- Q. Shi, D. X. Zhang, H. Yin, Y. P. Qiu, L. L. Zhou, C. Chen, H. Wu and P. Wang, Noble-metal-free Ni–W–O-derived catalysts for high-capacity hydrogen production from hydrazine monohydrate, ACS Sustainable Chem. Eng., 2020, 8, 5595–5603 CrossRef CAS.
- R. Moury and U. Demirci, Hydrazine borane and Hydrazinidoboranes as chemical hydrogen storage materials, Energies, 2015, 8, 3118–3141 CrossRef CAS.
- Y. Zhu and N. S. Hosmane, Nanocatalysis: Recent advances and applications in boron chemistry, Coord. Chem. Rev., 2015, 293–294, 357–367 CrossRef CAS.
- S. Zhang, Q. L. Yao and Z. H. Lu, Synthesis and dehydrogenation of hydrazine borane, Prog. Chem., 2017, 29, 426–434 Search PubMed.
- J. Chen, Z. H. Lu, W. Huang, Z. Kang and X. Chen, Galvanic replacement synthesis of NiPt/graphene as highly efficient catalysts for hydrogen release from hydrazine and hydrazine borane, J. Alloys Compd., 2017, 695, 3036–3043 CrossRef CAS.
- Z. Zhang, S. Zhang, Q. L. Yao, X. Chen and Z. H. Lu, Controlled synthesis of MOF-encapsulated NiPt nanoparticles toward efficient and complete hydrogen evolution from hydrazine borane and hydrazine, Inorg. Chem., 2017, 56, 11938–11945 CrossRef CAS.
- K. Yang, K. Yang, S. Zhang, Y. Luo, Q. L. Yao and Z. H. Lu, Complete dehydrogenation of hydrazine borane and hydrazine catalyzed by MIL-101 supported NiFePd nanoparticles, J. Alloys Compd., 2018, 732, 363–371 CrossRef CAS.
- Z. Zhang, S. Zhang, Q. L. Yao, G. Feng, M. Zhu and Z. H. Lu, Metal–organic framework immobilized RhNi alloy nanoparticles for complete H2 evolution from hydrazine borane and hydrous hydrazine, Inorg. Chem. Front., 2018, 5, 370–377 RSC.
- J. Goubeau and E. Ricker, Borinhydrazin und seine Pyrolyseprodukte, Z. Anorg. Allg. Chem., 1961, 310, 123–142 CrossRef CAS.
- T. Hugle, M. F. Kuhnel and D. Lentz, Hydrazine borane: a promising hydrogen storage material, J. Am. Chem. Soc., 2009, 131, 7444–7446 CrossRef.
- Y. S. Chua, Q. Pei, X. Ju, W. Zhou, T. J. Udovic, G. Wu, Z. Xiong, P. Chen and H. Wu, Alkali metal hydride modification on hydrazine borane for improved dehydrogenation, J. Phys. Chem. C, 2014, 118, 11244–11251 CrossRef CAS.
- R. Moury, K. Robeyns, Y. Filinchuk, P. Miele and U. B. Demirci, In situ thermodiffraction to monitor synthesis and thermolysis of hydrazine borane-based materials, J. Alloys Compd., 2016, 659, 210–216 CrossRef CAS.
- S. Karahan, M. Zahmakiran and S. Özkar, Catalytic methanolysis of hydrazine borane: a new and efficient hydrogen generation system under mild conditions, Dalton Trans., 2012, 41, 4912–4918 RSC.
- Y. Karatas, M. Gülcan and F. Sen, Catalytic methanolysis and hydrolysis of hydrazine-borane with monodisperse Ru NPs@nano-CeO2 catalyst for hydrogen generation at room temperature, Int. J. Hydrogen Energy, 2019, 44, 13432–13442 CrossRef CAS.
- D. Çelik, S. Karahan, M. Zahmakıran and S. Özkar, Hydrogen generation from the hydrolysis of hydrazine-borane catalyzed by rhodium(0) nanoparticles supported on hydroxyapatite, Int. J. Hydrogen Energy, 2012, 37, 5143–5151 CrossRef.
- Q. L. Yao, Z. H. Lu, K. Yang, X. Chen and M. Zhu, Ruthenium nanoparticles confined in SBA-15 as highly efficient catalyst for hydrolytic dehydrogenation of ammonia borane and hydrazine borane, Sci. Rep., 2015, 5, 15186 CrossRef CAS.
- S. Karahan, M. Zahmakıran and S. Özkar, Catalytic hydrolysis of hydrazine borane for chemical hydrogen storage: Highly efficient and fast hydrogen generation system at room temperature, Int. J. Hydrogen Energy, 2011, 36, 4958–4966 CrossRef CAS.
- S. Şencanlı, S. Karahan and S. Özkar, Poly(4-styrenesulfonic acid-co-maleic acid) stabilized nickel(0) nanoparticles: Highly active and cost effective catalyst in hydrogen generation from the hydrolysis of hydrazine borane, Int. J. Hydrogen Energy, 2013, 38, 14693–14703 CrossRef.
- S. Karahan and S. Özkar, Poly(4-styrenesulfonic acid-co-maleic acid) stabilized cobalt(0) nanoparticles: A cost-effective and magnetically recoverable catalyst in hydrogen generation from the hydrolysis of hydrazine borane, Int. J. Hydrogen Energy, 2015, 40, 2255–2265 CrossRef CAS.
- N. Tunç, B. Abay and M. Rakap, Hydrogen generation from hydrolytic dehydrogenation of hydrazine borane by poly(N-vinyl-2-pyrrolidone)-stabilized palladium nanoparticles, J. Power Sources, 2015, 299, 403–407 CrossRef.
- J. Hannauer, O. Akdim, U. B. Demirci, C. Geantet, J. M. Herrmann, P. Miele and Q. Xu, High-extent dehydrogenation of hydrazine borane N2H4BH3 by hydrolysis of BH3 and decomposition of N2H4, Energy Environ. Sci., 2011, 4, 3355–3358 RSC.
- Ç. Çakanyıldırım, U. B. Demirci, T. Şener, Q. Xu and P. Miele, Nickel-based bimetallic nanocatalysts in high-extent dehydrogenation of hydrazine borane, Int. J. Hydrogen Energy, 2012, 37, 9722–9729 CrossRef.
- J. Hannauer, U. B. Demirci, C. Geantet, J. M. Herrmann and P. Miele, Transition metal-catalyzed dehydrogenation of hydrazine borane N2H4BH3 via the hydrolysis of BH3 and the decomposition of N2H4, Int. J. Hydrogen Energy, 2012, 37, 10758–10767 CrossRef CAS.
- D. C. Zhong, K. Aranishi, A. K. Singh, U. B. Demirci and Q. Xu, The synergistic effect of Rh-Ni catalysts on the highly-efficient dehydrogenation of aqueous hydrazine borane for chemical hydrogen storage, Chem. Commun., 2012, 48, 11945–11947 RSC.
- C. Li, Y. Dou, J. Liu, Y. Chen, S. He, M. Wei, D. G. Evans and X. Duan, Synthesis of supported Ni@(RhNi-alloy) nanocomposites as an efficient catalyst towards hydrogen generation from N2H4BH3, Chem. Commun., 2013, 49, 9992–9994 RSC.
- Q. L. Zhu, D. C. Zhong, U. B. Demirci and Q. Xu, Controlled synthesis of ultrafine surfactant-free NiPt nanocatalysts toward efficient and complete hydrogen generation from hydrazine borane at room temperature, ACS Catal., 2014, 4, 4261–4268 CrossRef CAS.
- Z. Zhang, Z. H. Lu and X. Chen, Ultrafine Ni–Pt alloy nanoparticles grown on graphene as highly efficient catalyst for complete hydrogen generation from hydrazine borane, ACS Sustainable Chem. Eng., 2015, 3, 1255–1261 CrossRef CAS.
- Z. Zhang, Z. H. Lu, H. Tan, X. Chen and Q. L. Yao, CeOx-modified RhNi nanoparticles grown on rGO as highly efficient catalysts for complete hydrogen generation from hydrazine borane and hydrazine, J. Mater. Chem. A, 2015, 3, 23520–23529 RSC.
- Z. Zhang, Y. Wang, X. Chen and Z. H. Lu, Facile synthesis of NiPt–CeO2 nanocomposite as an efficient catalyst for hydrogen generation from hydrazine borane, J. Power Sources, 2015, 291, 14–19 CrossRef CAS.
- X. Hong, Q. L. Yao, M. Huang, H. Du and Z. H. Lu, Bimetallic NiIr nanoparticles supported on lanthanum oxy-carbonate as highly efficient catalysts for hydrogen evolution from hydrazine borane and hydrazine, Inorg. Chem. Front., 2019, 6, 2271–2278 RSC.
- W. Ben Aziza, J. F. Petit, U. B. Demirci, Q. Xu and P. Miele, Bimetallic nickel-based nanocatalysts for hydrogen generation from aqueous hydrazine borane: Investigation of iron, cobalt and palladium as the second metal, Int. J. Hydrogen Energy, 2014, 39, 16919–16926 CrossRef CAS.
- S. J. Li, X. Kang, B. R. Wulan, X. L. Qu, K. Zheng, X. D. Han and J. M. Yan, Noble–metal–free Ni–MoOx nanoparticles supported on BN as a highly efficient catalyst toward complete decomposition of Hydrazine Borane, Small Methods, 2018, 2, 1800250 CrossRef.
- S. J. Li, H. L. Wang, B. R. Wulan, X. B. Zhang, J. M. Yan and Q. Jiang, Complete dehydrogenation of N2H4BH3 over noble-metal-free Ni0.5Fe0.5-CeOx/MIL-101 with high activity and 100% H2 selectivity, Adv. Energy Mater., 2018, 8, 1800625 CrossRef.
- S. Zhang, Q. L. Yao, Q. Li, G. Feng and Z. H. Lu, Complete hydrogen production from hydrazine Bborane over raney Ni catalyst at room temperature, Energy Technol., 2019, 7, 1800533 CrossRef.
|
This journal is © the Partner Organisations 2020 |