DOI:
10.1039/D4QI00552J
(Research Article)
Inorg. Chem. Front., 2024,
11, 3418-3425
Magnifying the turn-on luminescence and electrical conductivity via the coupling effect of oxidation, metal ion adhesion and pressure within MnII-MOFs†
Received
1st March 2024
, Accepted 22nd April 2024
First published on 25th April 2024
Abstract
A metal–organic framework (MOF), MnII2-BTDB-MOF (Mn1, BTDB = 4,4′-(1,2,5-benzothiadiazole-4,7-diyl)bis-benzoic acid), exhibits enhancement of turn-on luminescence through the restriction of intramolecular vibration (RIV) mechanism, retaining its crystallinity and porosity. As expected, a 1.8-, 3.6-, 45.5-, and 164.4-fold emission enhancement respect to that of Mn1 by suffering from respective pressure, oxidation, the introduction of Hg2+, and the above three strategies totally, are observed, indicating their coupling effects on luminescence sensor. The results of density functional theory calculations reveal that the introduced metal ions trigger the RIV of BTDB by reducing the changes of the dihedral angle between the ground and excited states, suppressing nonradiative energy exhaustion, and thus magnifying turn-on luminescence. Furthermore, through stepwise fine-tuning the intricate physical and electronic structure associated with oxidation and the introduction of Zn2+ under 20 MPa, the electrical resistivity is dramatically improved from <10−17 S cm to 4.4 × 10−8 S cm. It is the first time to systematically magnify the luminescence, along with electrical resistivity, by employing the coupling effects of multiple external stimuli on MOFs, thereby highlighting their adaptabilities as sensors and electronics.
Introduction
Stimulus responsive materials (SRMs) respond to environmental changes through chemical and/or structural transformations, triggered by interactions at solid–gas or solid–liquid interfaces.1 SRMs span from organic polymers to porous inorganic solids, such as metal–organic frameworks (MOFs).2,3 On the one hand, MOFs can undergo post-synthetic modification (PSM) without compromising the overall framework integrity. On the other hand, the inorganic and the organic moieties, even some guest molecules, within the MOFs, can provide platforms to emit or induce luminescence.4,5 The recognition/binding events with guest substrates confined by tunable pore sizes and functional pore surfaces, which can be transduced into external optical signals, render MOFs as a new type of luminescent sensing material for the rapid detection of hazardous chemicals at trace concentrations.6,7 To date, most of the sensing procedures are based on fluorescence quenching, and strategies for the recognition of analytes through turn-on sensing behavior, especially using the coupling effect of more than two stimuli, are scarce.
The luminescence performance of MOFs can be affected by many environmental factors,8 including physical (pressure)9 and chemical stimuli (ions,10–12 solvents,13–15 pH,16 gases,17,18 and more19–23). However, unlike other luminescent materials, the intrinsic porosity, large surface area, and strong adsorption affinity of MOFs can effectively enrich guest species. Guest analytes can interact with the host framework through weak van der Waals interactions,24 hydrogen bonding,25 and coordination bonds, thus effectively altering the luminescent intensity and/or color of the host by changing its excited-state energy, non-radiation pathway and efficiency.26
Herein, we designed a porous MnII2-BTDB-MOF (Mn1, btdb = 4,4′-(1,2,5-benzothiadiazole-4,7-diyl)bis-benzoic acid) as an SRM based on the restriction of intramolecular vibration (RIV) mechanism using the following strategies (Scheme 1): (1) linkers, such as BTDB-containing sulfur or nitrogen atoms as soft bases, favor the anchoring of metal ions, increasing the steric hindrance to suppress the vibration of BTDB;27 (2) immobilization of the vibrator of BTDB via grinding or pressing prevents nonradiative excited energy dissipation;28 (3) through PSM such as oxidation without perturbing the parent framework;29 the interactions among metal ions (nodes) and the electron pairs of the adjacent N of BTDB within oxidized MOFs (Mn1′) are stronger than those of the original one, thereby magnifying the turn-on luminescence by RIV.30 As expected, the emission enhancement of Mn1 suffers from respective stimuli, such as pressure, oxidation, and addition of metal ions, and is much lower than that under multiple stimulus through the RIV mechanism. Density functional theory (DFT) calculations reveal that the introduced metal ions not only decrease the changes in the dihedral angles between the ground and excited states of BTDB, dramatically magnifying the luminescence intensity, but also reduce the band gaps of emissive transitions, red-shifting the emissive bands. Moreover, associated with oxidation, followed by the introduction of Zn2+ under 20 MPa, the electrical resistivity is dramatically improved from <10−17 S cm to 4.4 × 10−8 S cm. It firstly employs three strategies, synergistically magnifying turn-on luminescence and electrical conductivity, affording great prospects of MOFs as sensors and electronics for environmental applications.
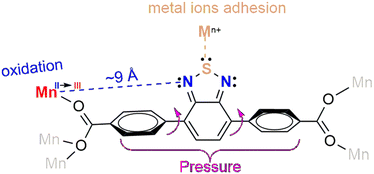 |
| Scheme 1 Strategies for designing metal-BTDB-MOFs with a turn-on sensing behaviour based on the coupling effect of oxidants, metal ions, and pressure. | |
Results and discussion
As shown in Table S1,† although several works based on using BTDB as the linker to construct M-BTDB-MOFs (M = Mn, Pd, Zr) have been reported, due to different components (auxiliary bridging ligand) inducing various coordination modes of metal ions and bridging modes of the linker, the versatile topological structure and porosity of the M-BTDB-MOFs could be provided. For instance, three-dimensional MnII4-BTDB2-MOF together with two-dimensional PbII-BTDB-MOF were previously employed as luminescent sensors for the detection of both highly toxic metal ions, even oxidizing anions with ppb detection limits in solution through luminescence quenching.31 Also, a luminescent ZrIV6-BTDB2-MOF exhibits an unprecedented detection limit in aqueous solutions for organic amines with ultralow detection limits, which was driven by hydrogen bonding interactions between the linker and the hosted amines. The selective recognition of different amines depends on their protonation in aqueous, inducing luminescence quenching for aromatic amines, enhancement for aliphatic amine, and negligible changes for pyridine.32 On the other hand, the in-depth exploration of turn-on sensing behavior through coupling effects within MOFs and its potential on electrical conductivity remain unexplored.
Crystal structure and characterization
The synthetic procedure (Fig. S1†), crystallographic data (Table S2†), selective bond lengths (Table S3†) and angles (Table S4†), together with the crystal structure of Mn1 (CCDC 2309896†), were analyzed in details. In the structure (Fig. 1a), the Mn2+ ion adopts a vacant octahedron coordination with five carboxylate oxygen from five btdb, bridged by carboxylate groups through μ2:η1:η1:η1 modes, formation of an 1D MnII chain along the b direction, in which the intra Mn⋯Mn distances are within 3.79–5.23 Å, and the intra-chain Mn–μ2-O–Mn angle is 120.92°. The 1D MnII chains are linked one to another via ligands and construct a 3D framework with various pores, leading to the formation of a wave-like shape along the a-axis with the smallest and largest apertures of 3.4 and 4.1 Å, respectively (Fig. S2†). The void volume that is generated after the removal of the coordinating DMF molecules is 25.6% of the total volume, as estimated by PLATON, accommodating lattice guest molecules (Fig. S4†).
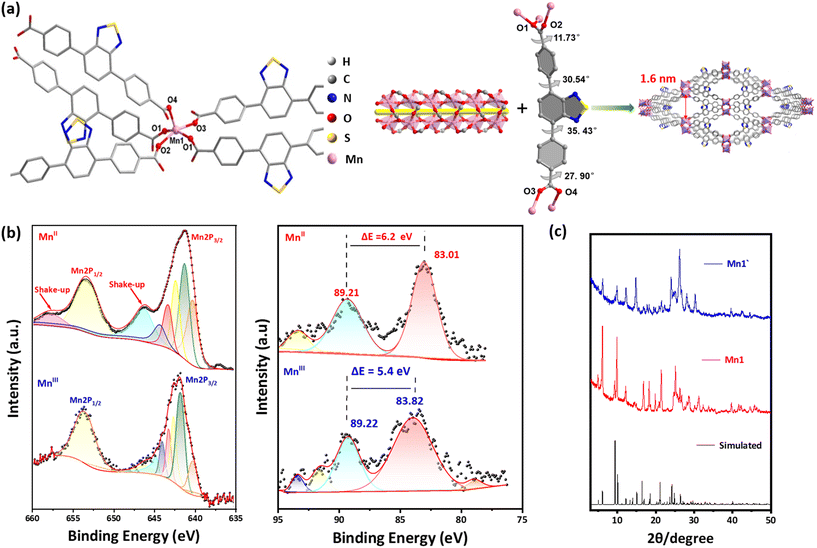 |
| Fig. 1 The asymmetric unit of Mn1 with the atomic numbering scheme (thermal ellipsoids at 50% probability) (a), and the perspective views of the 3D framework of Mn1 (b). The corresponding high-resolution XPS spectra of Mn 2p and Mn 3s signal deconvolution and PXRD patterns before and after oxidation (c). | |
After oxidation, the vibrating satellite peak of Mn 2p in the XPS spectrum disappears (Fig. 2b, left), and the binding energy fitted by the Mn 3s orbital shifts to 6.20 eV from 5.40 eV (Fig. 2b, right),33 confirming that the valence of MnII in Mn1 transfers into MnIII in Mn1′, broadening and strengthening the vibration in the range of 1300–1604 cm−1, which is attributed to the enhanced strength and symmetry of the coordination bonds between the carboxylate groups and Mn3+ (Fig. S3†). Similar PXRD patterns of Mn1 before and after oxidation, matching the simulated one of single crystal, confirm the chemical stability (Fig. 1c). Combining the results of TG, the desolvated frameworks were generated by heating Mn1 at 170 °C for 4.5 h and Mn1′ at 250 °C for 10 h (Fig. S4†); N2 absorption isotherm under 77 K was used to characterize the porosity of their networks (Fig. S5†), which shows a reversible type II isotherm, indicating the permanent micro-porosity. Gradually increasing the pressure, a little hysteresis between absorption/desorption curves suggests a small amount of meso-porosity in the desolvated material.34 The saturated uptake is 96.27 and 63.83 cm3 g−1, and the BET surface area is estimated as 184.66 and 13.07 m2 g−1 for Mn1 and Mn1′, respectively. Through the equation Vliq = Pa × Vads × Vm/RT, the total pore volume is calculated as 1.49 × 10−1 and 9.87 × 10−2 cc g−1, and the pore diameter is less than 1.79 and 1.62 nm at 0.99 atm for Mn1 and Mn1′, respectively. Additionally, the molecular formula of Mn1′ is deduced as MnIII2-BTDB·0.3H2O·0.4DMSO·I− based on the TGA (Fig. S4†) and titration measurement (Fig. S6†).
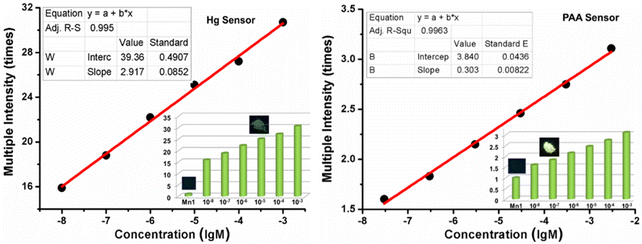 |
| Fig. 2 Rapid detection of PAA (a) and Hg(ClO4)2 (b) by Mn1 under UV irradiation with naked-eye limitation. | |
Optical property
Solid state BTDB and Mn1 exhibit similar absorption bands at ca. 257 nm, 307 nm, and 385 nm, assigned as the π–π* transitions. Upon irradiation within the corresponding absorption bands from 278 to 363 nm, Mn1 exhibits a green luminescence centered at ca. 523 nm, attributed to the π*–π transitions of the ligand (Fig. S7†).
Metal ion sensor.
Due to the potential coordination bonding of the free S or N atoms in BTDB, facilitating the sensor performance of metal ions, a series of parallel experiments were set up by immersing Mn1 crystals into DMF solutions of different metal salts (Ag+, Zn2+, Hg2+) with the same molar concentrations during the same period, and luminescent tests were conducted on dried solid samples under λex = 336 nm, inducing an obvious turn-on luminescence by RIV (Fig. S8†). On the one hand, the typical peaks of PXRD consistent with the simulated one (Fig. S9†) confirm a stable framework during metal ion sensing. On the other hand, since the pore diameters of Mn1 are much larger than the chosen metal ions, all the introduced metal ions could enter into the pore channel. Neglecting the subtle effect of anions on the luminescence intensity (Fig. S8d†), a more intensive enhancement of luminescent intensity with respect to that of original Mn1 was observed in perchlorate than that of nitrate (Fig. S8†) since one metal ion would link more than one N/S in different BTDB. Moreover, besides the 19- and 14-times emission enhancement by the introduction of AgClO4/AgNO3 into Mn1, the luminescent spectra red shift from 523 nm to 605 nm (Fig. S8a†).
Oxidation sensor.
According to a previous work, H2O2 was firstly considered as a candidate for Mn1 oxidation, but different PXRD before and after oxidation indicate the destruction of the original framework integrity (Fig. S10†). Then, the weaker oxidant I2 was selected to generate Mn′, confirmed by the valence of MnII to MnIII by XPS (Fig. 1b), with the original framework integrity by similar PXRD (Fig. 1c). The optimal approach came to Mn1 soaked in a solution of I2 in DMSO/H2O (v/v = 1
:
1) (Fig. S11†),35 where DMSO promotes the dissolution of I2, and the presence of water induces the reaction I2 + H2O = HI + HIO. It is the HIO actually as the oxidant that converts MnII into MnIII, confirmed by the increase in the acidity (Fig. S12†), which magnifies the luminescence intensity to 3.6-times compared with that of Mn1 (Fig. S13†) due to the stronger interactions between MnIII than MnII and electron pairs of nitrogen atoms in BTDB, triggering stronger emission intensity under UV irradiation by the RIV mechanism.
Pressure sensor.
The powders of Mn1 trigger turn-on luminescence by RIV under different degrees of pressure, and three things could be found: (i) increasing the pressure on powders up to 40 MPa, 1.8-times luminescence enhancement with respect to that of the original Mn1 could be achieved (Fig. S14a†); (ii) keeping the pressure at 40 MPa for 5 seconds or lasting or 2 h, the luminescence intensity remains almost unchanged (Fig. S14b†); (iii) the luminescence intensity of Mn1′ under 40 MPa significantly magnifies to 21.2 times compared with that of Mn1 (Fig. S14c†), inducing a bright blue emission under UV irradiation (Fig. S15†).
Two kinds of coupling sensor.
According to the above discussion, only 1.8-times luminescence magnification under 40 MPa pressure and a 3.6-fold intensity enhancement relative to that of Mn1 after oxidation was observed for Mn1, whereas the luminescence intensity significantly climbed to 21.2-times compared with those of original Mn1 under oxidation and 40 MPa pressure. Similarly, 65.7-times luminescence enhancement was obtained by the introduction of Zn(ClO4)2 into Mn1; further, under 40 MPa pressure, 73.3-folds luminescence magnification was observed (Fig. S16a†). Replacing Mn1 with Mn1′, 92.6-times enhancement of luminescence under Zn2+ adhesion and 103.7-folds further under 40 MPa pressure appeared (Fig. S16b†). All these facts suggest a coupling effect on the luminescent sensor. Using AgClO4 and Hg(ClO4)2 instead of Zn(ClO4)2 in the above experiments, the resultant coupling effect on turn-on luminescence is presented (Fig. S17†). Interestingly, as shown in Table 1, there were some differences dependent on the order. For instance, first oxidation Mn1 into Mn1′, then metal ion adhesion (MnOM, M = Ag+, Zn2+, Hg2+), or introduction of metal ion into Mn1, followed by further oxidation (MnMO), were studied, and it was found that the coupling effect on turn-on luminescence between MnOM and MnMO was different, where the latter was slightly more obvious than the former.
Table 1 Relative emission intensity and wavelength of Mn1 under oxidation, metal ions, pressure (40 MPa) and their coupling sensing at λex = 336 nm at room temperature
Factor |
RI/(λem/nm) |
DF |
RI/(λem/nm) |
TF |
RI/(λem/nm) |
RI = relative intensity; DF = double factors; TF = triple factors; O = oxidation by I2 in DMSO/H2O, P = pressure at 40 MPa, — = none. |
Blank |
1/523 |
— |
— |
— |
— |
P |
1.83/523 |
O + P |
21.2/520 |
— |
— |
O |
3.64/524 |
O + Ag+ |
22.2/606 |
O + Ag+ + P |
26.6/606 |
— |
— |
O + Hg2+ |
146.1/544 |
O + Hg2+ + P |
164.4/544 |
— |
— |
O + Zn2+ |
92.6/531 |
O + Zn2+ + P |
103.7/531 |
Ag+ |
18.7/605 |
Ag+ + O |
23.9/612 |
Ag+ + O + P |
26.7/613 |
Hg2+ |
47.2/544 |
Hg2+ + O |
152.5/544 |
Hg2+ + O + P |
158.9/544 |
Zn2+ |
65.7/531 |
Zn2+ + O |
94.1/531 |
Zn2+ + O + P |
104.6/532 |
Fe3+ |
8.4/521 |
Fe3+ + O |
32.9/515 |
Fe3+ + O + P |
59.4/514 |
Three kinds of coupling sensors.
As shown in Table 1, luminescence enhancement occurred from Mn1 to MnP, MnO and last MnOP of about 1 to 1.8-, 3.6- and finally to 21.2-times; if altering the order from MnZn to MnZnP, MnOZn, and MnOZnP, 65.7- to 73.3-, 92.6-, and total 103.7-times luminescence magnification was achieved. Anyway, three kinds of coupling sensors were presented in the above operations, and the total luminescent enhancement slightly depended on the order of the triggers (Fig. S18a†). Interestingly, even the introduction of the famous luminescent quencher of Fe3+ into Mn1,36 and further cooperation of oxidation and pressure led to at least 60-times luminescence enhancement (Fig. S18b†), confirming RIV as the dominant mechanism for luminescence enhancement within Mn1. For the coupling of oxidation and metal ions adhesion, we deduced that some of the free S in BTDB interacts with the metal ions; such a conclusion was also confirmed by the Raman spectroscopic study in a previous work.37
Rapid detection of hazardous chemicals with naked eyes
Fluorescence detection technology with high sensitivity and simple operation has been widely developed.38,39 Among common toxic heavy metal ions, Hg2+ easily causes various diseases, even death.40 The peroxidation value is usually used as a determination of the quality and deterioration of foods made of fat, such as swill-cooked dirty oil; the higher the peroxidation value, the more the rancidity.41 Thus, developing a rapid and accurate fluorescence detection approach with naked eyes is significant and important.
As shown in Table 1, luminescence enhancement from Mn to MnO, MnOHg and the last MnOHgP is 1- to 3.6-, 146.1-, and finally to 164.4-times; if altering the order from Mn1 to MnHg, MnHgO, and MnHgOP, 1- to 45.5-, 152.5-, and total 158.9-times luminescence magnification were also observed (Fig. S19a†). All these facts suggest that using Mn1 as a sensor for Hg2+ possesses superior sensitivity. Herein, to realize the quick detection of peroxyacetic acid (PAA) and Hg(ClO4)2, we carry out the sensing operation by the addition of a drop of hazardous chemicals of DMF solutions into the 10 mg powders. Then, the powders were laid and further dried through filter paper. Under 365 nm UV lamp irradiation, 10−7 M for PAA and 10−5 M for Hg2+ could be easily detected by a bright blue emission with naked eyes (Fig. 2). Also, the consistent PXRD patterns before and after detection (Fig. S9 and 20†) confirmed a stable framework during the sensing procedure. Although such turn-on luminescence for PAA and Hg(ClO4)2 detection was rapid and sensitivity, the selectivity was still to be solved. Since the introduced metal ions could enter into the channels of Mn1 and they have strong interactions with the electron pairs of adjacent N of BTDB, the cyclic performance of materials under various conditions is inferior (Fig. S21†). Anyway, the rapid detection with ultralow detection limits of Mn1 with naked eyes is promising for probing the local environment, such as pressure as well as toxic and oxidizing species, which has important environmental and humanitarian implications.
Density functional theory calculations
To further explore the RIV mechanism and the abnormal luminescence enhancement of the quencher Fe3+ ions as well as the red-shift phenomenon after introducing Ag+ ions, we adopted a methodology inspired by previous literature, wherein density functional theory (DFT) calculations were performed on H2BTDB (H2L) and H2L@Ag+/H2L@Zn2+/H2L@Fe3+, respectively (Fig. S22 and Tables S5–12†).32 The results show that before introducing Zn2+, the dihedral angle between the benzene ring and the thiadiazole group is 38.20° in the ground state and 18.04° in the excited state of H2L, with a change of 20.16° between the ground and excited states. After introducing Zn2+, the dihedral angle of the ground states decreases to 23.84° and one of the excited states is 21.57°, with a significant reduction of 2.27° (Tables S9 and 10†). This demonstrates that the introduction of Zn2+ significantly suppresses the dihedral vibration of H2L, thus supporting the rationality of the RIV mechanism. As for H2L@Fe, the dihedral angle between the benzene ring and the thiadiazole group is 29.43° in the ground state and 30.17° in the excited state, inducing a change in the dihedral angle that is 0.74° (Tables S11 and 12†) smaller than that in H2L@Zn (2.27°) (Fig. 3a). On the other hand, the low-lying excited states with similar energy gaps of Fe3+ facilitate internal conversion and vibrational relaxation, thus quenching the luminescence. Thus, the cooperation between efficiently preventing RIV and luminescence quenching makes the luminescence enhancement of Fe3+@Mn1 (8.4 times) lower than that of Zn2+@Mn1 (65.7 times, Table 1).
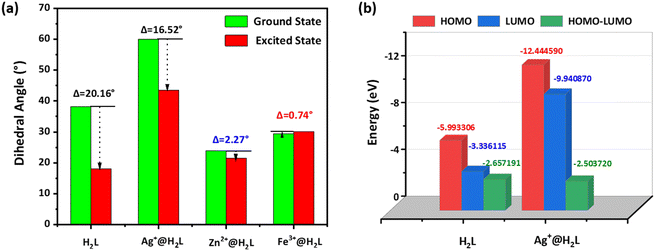 |
| Fig. 3 The angle change (Δ) between the ground state and excited state benzene-thiazole in H2L (a); the lowest level energy value of the HOMO and LUMO of H2L and Ag+@H2L (b). | |
Furthermore, the DFT calculations on the frontier molecular orbitals (MOs) of H2L (Tables S5 and 6†) and H2L@Ag+ (Tables S7 and 8†) show that the HOMO and LUMO energies are −5.99 eV and −3.34 eV before the addition of Ag+, giving a band gap of −2.66 eV between the MOs. After the introduction of Ag+, the HOMO and LUMO energies become −12.44 eV and −9.94 eV, reducing the band gap between MOs to −2.50 eV (Fig. 3b), thus red-shifting the emission bands.
Electrical conductivity modulation
Unlike the previous concept of introducing unpaired electrons into the redox hopping strategy, we emphasize the synergy strategies of both oxidation and guest-promoted transport.44 Through stepwise fine-tuning the intricate physical and electronic structure,42,43 a pronounced enhancement of the electric conductivity of Mn1 upon oxidation and subsequent introduction of metal ions was observed. On the one hand, the d-electron configuration of Mn2+ in Mn1 is fully occupied, without unpaired electrons. After oxidation, the Mn3+ in Mn1′ creates an unpaired electron, which participates in electron conduction along with the c-axis of the lattice, thus magnifying the electrical conductivity (Fig. 4a). On the other hand, according to the hard–soft acid–base theory, Zn2+ being a relatively soft metal ion easily experiences π-d conjugated bonds with the softer S or N atoms in BTDB, further optimizing the electrical conductivity.
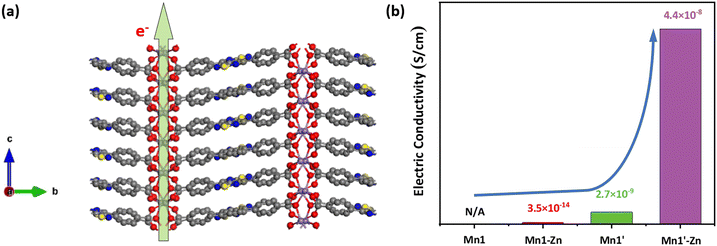 |
| Fig. 4 A-axis-aligned 2D structure of Mn1 (a), and conductivity of Mn1 under Zn2+ adhesion/oxidation spectra (b). | |
As shown in Fig. 4b and Tables S13–15,† the value of resistance of Mn1 exceeds the maximum range of our high-impedance meter with a maximum detection limit of 1017 Ω cm. Excluding the effect of adsorbed I2 for electrical conductivity (Fig. S23†), after the oxidation of Mn1 into Mn1′ under a pressure of 20 MPa, the electrical conductivity reaches 2.68 × 10−9 S cm−1. On the further introduction of Zn2+ into Mn1′ then under 20 MPa, it climbs up to 4.4 × 10−8 S cm−1 (Fig. S24–26†). The above findings highlight the remarkably tunable effect of Mn-MOF on the conductivity under different chemical environments, bolstering their potential applications in sensing circuits and other electronic domains.45
Conclusions
We designed a porous MnII2-BTDB-MOFs (Mn1) as SRMs based on the RIV mechanism with its original crystallinity and porosity to realize turn-on luminescence through the coupling effect among oxidation, metal ions adhesion and pressure. Since magnifying the luminescence by the coupled stimulus is much higher than that by the respective one, the rapid detection of Hg2+ by naked eyes with ultralow detection limits is operative and practicable. Benefiting from the smallest changes in the dihedral angle, which effectively prevents nonradiative energy exhaustion by RIV, an obvious enhancement of turn-on luminescence still could be observed by the introduction of the luminescence quencher of Fe3+. Also, the introduced metal ions lower the band gaps of emissive transitions, red-shifting the emission band by the introduction of Ag+. Beside magnifying the turn-on luminescence, we also dramatically improved the electrical conductivity of Mn1 by the above coupled stimulus, highlighting the adaptability of MOFs in diverse external stimulus, which is beneficial for applications of sensors and electronics.
Conflicts of interest
There are no conflicts to declare.
Acknowledgements
We thank the financial support from BAGUI talent program in Guangxi Province (no. 2019AC26001), the NSFC (no. 22273019, 22171075, U23A2080); National Natural Science Foundation of China for Distinguished Young Scholars (no. 22101077), the Youth Foundation of Hubei Province (no. 2021CFB092), the “Chu-Tian Scholar” Program of Hubei Province, and Wuhan Science and Technology (2023010201010136).
References
- A. Muñoz-Bonilla, M. Fernández-García and J. Rodríguez-Hernández, Towards hierarchically ordered functional porous polymeric surfaces prepared by the breath figures approach, Prog. Polym. Sci., 2014, 39, 510–554 CrossRef.
- S. Mandal, S. Natarajan, P. Mani and A. Pankajakshan, Post–Synthetic Modification of Metal–Organic Frameworks Toward Applications, Adv. Funct. Mater., 2020, 31, 2006291 CrossRef.
- K. Müller-Buschbaum, F. Beuerle and C. Feldmann, MOF based luminescence tuning and chemical/physical sensing, Microporous Mesoporous Mater., 2015, 216, 171–199 CrossRef.
- L. Chen, R. Luque and Y. Li, Controllable design of tunable nanostructures inside metal-organic frameworks, Chem. Soc. Rev., 2017, 46, 4614–4630 RSC.
- Y. Liu, X. Y. Xie, C. Cheng, Z. S. Shao and H. S. Wang, Strategies to fabricate metal–organic framework (MOF)-based luminescent sensing platforms, J. Mater. Chem. C, 2019, 7, 10743–10763 RSC.
- M. Woellner, S. Hausdorf, N. Klein, P. Mueller, M. W. Smith and S. Kaskel, Adsorption and Detection of Hazardous Trace Gases by Metal-Organic Frameworks, Adv. Mater., 2018, 30, e1704679 CrossRef PubMed.
- G. L. Yang, X. L. Jiang, H. Xu and B. Zhao, Applications of MOFs as Luminescent Sensors for Environmental Pollutants, Small, 2021, 17, e2005327 CrossRef PubMed.
- X. D. Zhu, K. Zhang, Y. Wang, W. W. Long, R. J. Sa, T. F. Liu and J. Lu, Fluorescent Metal-Organic Framework (MOF) as a Highly Sensitive and Quickly Responsive Chemical Sensor for the Detection of Antibiotics in Simulated Wastewater, Inorg. Chem., 2018, 57, 1060–1065 CrossRef CAS PubMed.
- I. E. Collings and A. L. Goodwin, Metal–organic frameworks under pressure, J. Appl. Phys., 2019, 126, 181101 CrossRef.
- S. S. Zhao, J. Yang, Y. Y. Liu and J. F. Ma, Fluorescent Aromatic Tag-Functionalized MOFs for Highly Selective Sensing of Metal Ions and Small Organic Molecules, Inorg. Chem., 2016, 55, 2261–2273 CrossRef CAS PubMed.
- X. Song, T. K. Kim, H. Kim, D. Kim, S. Jeong, H. R. Moon and M. S. Lah, Post-Synthetic Modifications of Framework Metal Ions in Isostructural Metal–Organic Frameworks: Core–Shell Heterostructures via Selective Transmetalations, Chem. Mater., 2012, 24, 3065–3073 CrossRef CAS.
- J. Zhao, Y. N. Wang, W. W. Dong, Y. P. Wu, D. S. Li and Q. C. Zhang, A Robust Luminescent Tb(III)-MOF with Lewis Basic Pyridyl Sites for the Highly Sensitive Detection of Metal Ions and Small Molecules, Inorg. Chem., 2016, 55, 3265–3271 CrossRef CAS PubMed.
- F. Millange, C. Serre, N. Guillou, G. Férey and R. I. Walton, Structural Effects of Solvents on the Breathing of Metal–Organic Frameworks: An In Situ Diffraction Study, Angew. Chem., 2008, 120, 4168–4173 CrossRef.
- B. Chen, X. Wang, Q. Zhang, X. Xi, J. Cai, H. Qi, S. Shi, J. Wang, D. Yuan and M. Fang, Synthesis and characterization of the interpenetrated MOF-5, J. Mater. Chem. A, 2010, 20, 3758–3767 RSC.
- Y. Wu, G. P. Yang, Y. Zhao, W. P. Wu, B. Liu and Y. Y. Wang, Three new solvent-directed Cd(II)-based MOFs with unique luminescent properties and highly selective sensors for Cu2+ cations and nitrobenzene, Dalton Trans., 2015, 44, 3271–3277 RSC.
- M. Ding, X. Cai and H. L. Jiang, Improving MOF stability: approaches and applications, Chem. Sci., 2019, 10, 10209–10230 RSC.
- M. Alhamami, H. Doan and C. H. Cheng, A Review on Breathing Behaviors of Metal-Organic-Frameworks (MOFs) for Gas Adsorption, Materials, 2014, 7, 3198–3250 CrossRef PubMed.
- J. R. Li, R. J. Kuppler and H. C. Zhou, Selective gas adsorption and separation in metal-organic frameworks, Chem. Soc. Rev., 2009, 38, 1477–1504 RSC.
- W. Fan, X. Zhang, Z. Kang, X. Liu and D. Sun, Iso-reticular chemistry within metal–organic frameworks for gas storage and separation, Coord. Chem. Rev., 2021, 443, 213968 CrossRef CAS.
- K. I. Hadjiivanov, D. A. Panayotov, M. Y. Mihaylov, E. Z. Ivanova, K. K. Chakarova, S. M. Andonova and N. L. Drenchev, Power of Infrared and Raman Spectroscopies to Characterize Metal-Organic Frameworks and Investigate Their Interaction with Guest Molecules, Chem. Rev., 2021, 121, 1286–1424 CrossRef CAS PubMed.
- H. Li, L. Li, R.-B. Lin, W. Zhou, Z. Zhang, S. Xiang and B. Chen, Porous metal-organic frameworks for gas storage and separation: Status and challenges, J. Energy Chem., 2019, 1, 100006 Search PubMed.
- E. V. Perez, K. J. Balkus, J. P. Ferraris and I. H. Musselman, Mixed-matrix membranes containing MOF-5 for gas separations, J. Membr. Sci., 2009, 328, 165–173 CrossRef CAS.
- J.-H. Wang, M. Li and D. Li, A dynamic, luminescent and entangled MOF as a qualitative sensor for volatile organic solvents and a quantitative monitor for acetonitrile vapour, Chem. Sci., 2013, 4, 1793–1801 RSC.
- R. Poloni, B. Smit and J. B. Neaton, CO2 capture by metal-organic frameworks with van der Waals density functionals, J. Phys. Chem. A, 2012, 116, 4957–4964 CrossRef CAS PubMed.
- I. Ahmed and S. H. Jhung, Applications of metal-organic frameworks in adsorption/separation processes via hydrogen bonding interactions, Chem. Eng. J., 2017, 310, 197–215 CrossRef CAS.
- P. Deria, J. Yu, T. Smith and R. P. Balaraman, Ground-State versus Excited-State Interchromophoric Interaction: Topology Dependent Excimer Contribution in Metal-Organic Framework Photophysics, J. Am. Chem. Soc., 2017, 139, 5973–5983 CrossRef CAS PubMed.
- L. Zhu, B. Zhu, J. Luo and B. Liu, Design and Property Modulation of Metal–Organic Frameworks with Aggregation-Induced Emission, ACS Mater. Lett., 2020, 3, 77–89 CrossRef.
- C. X. Chen, S. Y. Yin, Z. W. Wei, Q. F. Qiu, N. X. Zhu, Y. N. Fan, M. Pan and C. Y. Su, Pressure-Induced Multiphoton Excited Fluorochromic Metal-Organic Frameworks for Improving MPEF Properties, Angew. Chem., Int. Ed., 2019, 58, 14379–14385 CrossRef CAS PubMed.
- Z. Yin, S. Wan, J. Yang, M. Kurmoo and M. H. Zeng, Recent advances in post-synthetic modification of metal–organic frameworks: New types and tandem reactions, Coord. Chem. Rev., 2019, 378, 500–512 CrossRef CAS.
- Y. Y. Liu, X. Zhang, K. Li, Q. C. Peng, Y. J. Qin, H. W. Hou, S. Q. Zang and B. Z. Tang, Restriction of Intramolecular Vibration in Aggregation-Induced Emission Luminogens: Applications in Multifunctional Luminescent Metal-Organic Frameworks, Angew. Chem., Int. Ed., 2021, 60, 22417–22423 CrossRef CAS PubMed.
- A. K. Jana and S. Natarajan, Fluorescent Metal–Organic Frameworks for Selective Sensing of Toxic Cations (Tl3+, Hg2+) and Highly Oxidizing Anions ((CrO4)2−, (Cr2O7)2−, (MnO4)−), ChemPlusChem, 2017, 82, 1153–1163 CrossRef PubMed.
- A. Mallick, A. M. El-Zohry, O. Shekhah, J. Yin, J. Jia, H. Aggarwal, A.-H. Emwas, O. F. Mohammed and M. Eddaoudi, Unprecedented Ultralow Detection Limit of Amines using a Thiadiazole-Functionalized Zr(IV)-Based Metal–Organic Framework, J. Am. Chem. Soc., 2019, 141, 7245–7249 CrossRef CAS PubMed.
- M. C. Biesinger, B. P. Payne, A. P. Grosvenor, L. W. M. Lau, A. R. Gerson and R. S. C. Smart, Resolving surface chemical states in XPS analysis of first row transition metals, oxides and hydroxides: Cr, Mn, Fe, Co and Ni, Appl. Surf. Sci., 2011, 257, 2717–2730 CrossRef CAS.
- X. Y. Tian, H. L. Zhou, X. W. Zhang, C. Wang, Z. H. Qiu, D. D. Zhou and J. P. Zhang, Two Isostructural Flexible Porous Coordination Polymers Showing Contrasting Single-Component and Mixture Adsorption Properties for Propylene/Propane, Inorg. Chem. Commun., 2020, 59, 6047–6052 CrossRef CAS PubMed.
- M. H. Zeng, Z. Yin, Y. X. Tan, W. X. Zhang, Y. P. He and M. Kurmoo, Nanoporous cobalt(II) MOF exhibiting four magnetic ground states and changes in gas sorption upon post-synthetic modification, J. Am. Chem. Soc., 2014, 136, 4680–4688 CrossRef CAS PubMed.
- R. R. Srivastava, V. K. Singh and A. Srivastava, Facile synthesis of highly fluorescent water-soluble SnS2 QDs for effective detection of Fe3+ and unveiling its fluorescence quenching mechanism, Opt. Mater. Express, 2020, 109, 110337 CrossRef CAS.
- P. Rodríguez-Zamora, C. A. Cordero-Silis, J. Fabila, J. C. Luque-Ceballos, F. Buendía, A. Heredia-Barbero and I. L. Garzón, Interaction Mechanisms and Interface Configuration of Cysteine Adsorbed on Gold, Silver, and Copper Nanoparticles, Langmuir, 2022, 38, 5418–5427 CrossRef PubMed.
- A. Karmakar, P. Samanta, S. Dutta and S. K. Ghosh, Fluorescent “Turn-on” Sensing Based on Metal-Organic Frameworks (MOFs), Chem. – Asian J., 2019, 14, 4506–4519 CrossRef CAS PubMed.
- D. Zhao, S. Yu, W. J. Jiang, Z. H. Cai, D. L. Li, Y. L. Liu and Z. Z. Chen, Recent Progress in Metal-Organic Framework Based Fluorescent Sensors for Hazardous Materials Detection, Molecules, 2022, 27, 2226 CrossRef CAS PubMed.
- R. A. Bernhoft, Mercury toxicity and treatment: a review of the literature, J. Environ. Public Health, 2012, 2012, 460508 Search PubMed.
- J. Kanner, Oxidative processes in meat and meat products: Quality implications, Meat Sci., 1994, 36, 169–189 CrossRef CAS PubMed.
- A. Gładysiak, T. N. Nguyen, M. Spodaryk, J. H. Lee, J. B. Neaton, A. Züttel and K. C. Stylianou, Incarceration of Iodine in a Pyrene–Based Metal–Organic Framework, Chem. – Eur. J., 2018, 25, 501–506 CrossRef PubMed.
- X. Zhang, I. da Silva, R. Fazzi, A. M. Sheveleva, X. Han, B. F. Spencer, S. A. Sapchenko, F. Tuna, E. J. L. McInnes, M. Li, S. Yang and M. Schröder, Iodine Adsorption in a Redox-Active Metal–Organic Framework: Electrical Conductivity Induced by Host−Guest Charge-Transfer, Inorg. Chem., 2019, 58, 14145–14150 CrossRef CAS PubMed.
- L. S. Xie, G. Skorupskii and M. Dinca, Electrically Conductive Metal-Organic Frameworks, Chem. Rev., 2020, 120, 8536–8580 CrossRef CAS PubMed.
- E. M. Johnson, S. Ilic and A. J. Morris, Design Strategies for Enhanced Conductivity in Metal-Organic Frameworks, ACS Cent. Sci., 2021, 7, 445–453 CrossRef CAS PubMed.
Footnotes |
† Electronic supplementary information (ESI) available: Experimental details, elemental analyses, IR, X-ray crystallography information, UV-Vis spectra, fluorescence spectra. CCDC 2309896. For ESI and crystallographic data in CIF or other electronic format see DOI: https://doi.org/10.1039/d4qi00552j |
‡ These authors contributed equally to this work. |
|
This journal is © the Partner Organisations 2024 |