DOI:
10.1039/D2QI00628F
(Research Article)
Inorg. Chem. Front., 2022,
9, 2862-2868
Assembling lanthanide–transition metal clusters on TiO2 for photocatalytic nitrogen fixation†
Received
24th March 2022
, Accepted 4th May 2022
First published on 5th May 2022
Abstract
Ammonia synthesis using light with low energy consumption offers an effective solution for energy saving and environmental protection. Herein, an abundant oxygen vacancy photocatalyst was synthesized via the integration of lanthanide–transition metal (4f–3d) clusters Ln52Ni56 on the TiO2 surface. The investigation of photocatalytic nitrogen fixation showed that Ln52Ni56 not only acts as a tool to improve charge separation but also enriches oxygen vacancies. Multiple synergies resulted in a photocatalytic nitrogen fixation efficiency of up to 800 μmol h−1 g−1 with the direct utilization of nitrogen and water without any sacrificial agents or co-catalysts. Electron paramagnetic resonance spectroscopy was conducted to investigate the mechanism of oxygen vacancy inactivation and recovery. This study provides a reference for the construction of a photochemical nitrogen fixation catalyst driven by defect engineering.
Introduction
Ammonia is an important raw material for synthetic fertilizers and chemical materials, particularly for agricultural and food production.1 Currently, the manufacture of ammonia mainly relies on the Haber–Bosch process, which consumes considerable energy.2–5 Therefore, the development of an efficient and sustainable method to synthesize ammonia, using nitrogen and water at normal temperature and pressure, seems promising. Many photocatalytic materials, such as BiOBr, LDHs, and g-C3N4, have been used since the first reported case of photocatalytic nitrogen fixation using TiO2.6–9 Different studies have reported that defect engineering is considered a powerful tool for providing multiple active sites and reducing energy activation barriers.10,11 Oxygen vacancies (OVs) have been extensively studied to construct catalytic sites, improve catalytic performance, and reduction of nitrogen.12–15 The participation of OVs can enhance the adsorption of substrates and adjust the energy band structure of semiconductors.16 However, the OVs produced via annealing by inert gas protection were unstable when exposed to air. Moreover, the control of the catalyst morphology affected the full utilization of OVs.17–21 The deactivation mechanism of OVs in photocatalytic nitrogen fixation is still unclear, and the regulation of OVs is very challenging.
TiO2 is regarded as a model catalyst owing to its low cost, non-toxicity, and stable structure.22,23 Many reports have shown that loading various metal nanoparticles or single atoms on the surface of TiO2 semiconductors can produce abundant OVs and improve charge separation, thus improving photocatalytic performance.16,18 Compared to polydisperse nanoparticles, metal clusters have a monodisperse size and well-defined crystal structures, which provide an ideal model catalyst for investigating the catalytic reaction mechanism.24–26 Herein, a Ln52Ni56/TiO2 composite material with abundant oxygen defects was obtained by loading well-defined heterometallic lanthanide–transition metal (4f–3d) clusters Ln52Ni56, (Ln = Eu, Pr, Nd, Gd) onto the TiO2 surface. The Ln52Ni56/TiO2 composite exhibited enhanced photocatalytic nitrogen fixation efficiency of up to 800 μmol h−1 g−1 under nitrogen and water conditions without any sacrificial agents or co-catalysts. The study of the mechanism suggests that the deactivation in solid–liquid phase reactions is attributed to the decrease in OVs caused by the occupation of reactive oxygen species.
Results and discussion
Stable clusters formulated as [Ln52Ni56(IDA)48(OH)154(H2O)38]·(NO3)18 (abbreviated as Ln52Ni56, Ln = Eu, Pr, Nd, and Gd; iminodiacetic acid (H2IDA)) were obtained as described in our previous work.27 Ln52Ni56 cluster molecules were added to reaction solution during the formation of TiO2via in situ hydrothermal synthesis. Ln52Ni56 is a class of cationic cluster with four-shell, nesting doll-like structure.28 In the outermost layer of the structure, some NiII ions are coordinated by two aqua ligands. These coordinated waters are easily leaved and form empty coordination sites, forming Ni–O coordination bonds with oxygen atoms on TiO2 in hydrothermal reaction. In addition, the carboxyl oxygen atom of the IDA ligands outside the clusters can also be connected with Ti4+ ions through coordination bonds. Different amounts of Eu52Ni56 cluster additives (0, 0.1, 0.2, 0.5, 0.7, and 1 mL of 1 mM Eu52Ni56 aqueous solution) are denoted here as TiO2-blank, Eu52Ni56/TiO2-1, Eu52Ni56/TiO2-2, Eu52Ni56/TiO2-3, Eu52Ni56/TiO2-4, and Eu52Ni56/TiO2-5. As shown in Fig. 1a, powder X-ray diffraction (PXRD) patterns indicate that all diffraction peaks match well with anatase-phase TiO2 (JCPDS no. 21-1272), and no other phase is formed.16,18 The diffraction intensity of the (101) peak position is slightly reduced and gradually shifts to a lower angle with the participation of the Ln52Ni56 clusters (Fig. S1†). This result confirms the successful loading of clusters into TiO2 and the increase in lattice constants.16,29,30
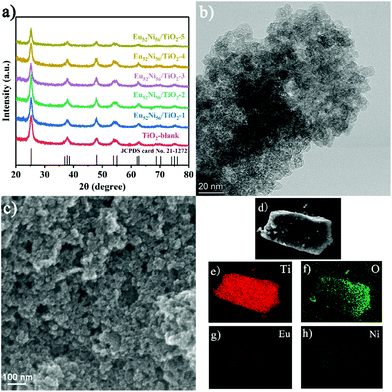 |
| Fig. 1 (a) PXRD patterns of TiO2-blank and Eu52Ni56/TiO2-1, 2, 3, 4, 5. (b) TEM profile of Eu52Ni56/TiO2-3. (c) SEM profile of Eu52Ni56/TiO2-3. (d–h) Selected area SEM profile of Eu52Ni56/TiO2-3, and corresponding EDX elemental mappings of Ti, O, Eu, and Ni. | |
The Transmission electron microscopy (TEM) profiles of TiO2-blank and Eu52Ni56/TiO2-3 show uniformly distributed tiny nanoparticles approximately 10 nm in diameter (Fig. 1b and S2a†). The high-resolution TEM (HRTEM) profiles of Eu52Ni56/TiO2-3 show a lattice spacing of 0.357 nm, which corresponds to 0.350 nm of the (101) lattice plane in TiO2 (Fig. S2c and d†). The increase in lattice spacing further confirms that the successful loading of clusters causes lattice distortion and a left shift of the XRD (101) peak.29,31 Selected area electron diffraction (SAED) profiles show that the catalyst mainly exposes (101) crystal planes (Fig. S3†). However, it is difficult to directly define the existence of clusters using HRTEM and high-angle annular dark-field scanning TEM (HAADF-STEM) (Fig. S4†). Scanning electron microscopy (SEM) shows that the catalyst has adequate size uniformity and does not significantly change the size of TiO2 particles (Fig. 1c and S5†). Elemental energy-dispersive X-ray spectroscopy (EDS) profiles (Fig. 1d–h) suggest that Eu52Ni56 is uniformly distributed throughout the catalyst.
The X-ray photoelectron spectroscopy (XPS) of TiO2 before and after loading shows that binding energies are located at 1155, 1126, and 867 eV in the full spectrum of the Eu52Ni56/TiO2-3 sample (Fig. S6†), which are attributed to Eu and Ni.32 In the XPS profile of Ti 2p (Fig. 2a), the two typical peaks at 458.7 and 464.5 eV are attributed to Ti 2p3/2 (Ti4+) and 2p1/2 (Ti4+), respectively. Notably, when compared to TiO2-blank, the Ti 2p3/2 and Ti 2p1/2 peaks of Eu52Ni56/TiO2-3 are shifted in the positive direction by 0.2 and 0.25 eV, respectively. This shift is attributed to the lattice distortions created by the interaction of TiO2 and Eu52Ni56 clusters.29,33 This observation confirms the XRD and TEM results, showing the successful loading of clusters. To confirm the presence of OVs, the XPS profile of O 1s was analyzed. As shown in Fig. 2b, the three peaks at 530.1, 513.5, and 532.7 eV are attributed to the three forms of internal lattice oxygen, OVs, and surface hydroxyl groups (OH), respectively.19 According to the integration of the areas of different samples (Fig. S9 and Table S1†), OV concentrations are in the order of Eu52Ni56/TiO2-3 (25.8%) > Eu52Ni56/TiO2-2 (23.2%) > Eu52Ni56/TiO2-1 (19.9%) > Eu52Ni56/TiO2-4 (19.1%) > TiO2-blank (18.6%) > Eu52Ni56/TiO2-5 (18.4%). The Eu52Ni56/TiO2-3 sample exhibits the highest OV content.
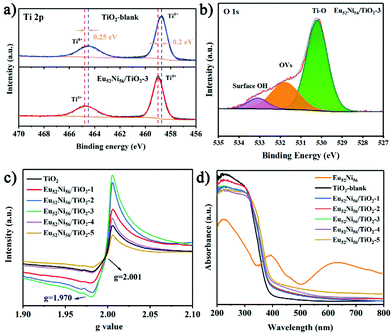 |
| Fig. 2 (a) Ti 2p XPS profiles of TiO2-blank and Eu52Ni56/TiO2-3. (b) O 1s XPS profile of Eu52Ni56/TiO2-3. (c) EPR profiles of TiO2-blank and Eu52Ni56/TiO2-1, 2, 3, 4, 5. (d) UV-vis DRS profiles of the Eu52Ni56 cluster, TiO2-blank, and Eu52Ni56/TiO2-1, 2, 3, 4, 5. | |
To further confirm the generation of OVs, Electron paramagnetic resonance (EPR) spectroscopy of Eu52Ni56/TiO2 was performed at 110 K without inert gas protection. As shown in Fig. 2c, a particularly distinct EPR peak is observed at a g value of 2.001, which is attributed to the OVs.19,34 Under the same experimental conditions, Eu52Ni56/TiO2-3 exhibited the highest concentration of OVs, which is consistent with the XPS results. It is worth noting that TiO2-blank still has a higher OV concentration because of the small and uniform nanoparticles. Based on these results, the loading of Ln52Ni56 clusters during in situ synthesis can indeed increase the concentration of OVs and have a stabilizing effect. The faint peak at g = 1.970 is attributed to Ti3+.35,36 The UV-vis diffuse reflectance spectroscopy (UV-vis DRS) profiles of the Eu52Ni56 cluster, TiO2-blank, and Eu52Ni56/TiO2-1, 2, 3, 4, 5 are shown in Fig. 2d. TiO2-blank almost exclusively absorbs ultraviolet light and exhibits only a weak response to visible light because of the charge transfer from the valence band (VB, O 2p orbitals) to the conduction band (CB, Ti 3d orbitals). The measured UV-vis DRS of the Eu52Ni56 shows two main peaks of d–d transition for octahedral Ni2+ at 400 and 632 nm, which are assigned to 3A2g → 3T1g (P) and 3A2g → 3T1g (F), respectively. The absorption of Eu52Ni56/TiO2-1, 2, 3, 4, and 5 improves because of the loading of clusters on TiO2.16,18,37 With the increase in Eu52Ni56 cluster loading, the apparent color of the catalyst slowly changes from white to yellowish (Fig. S10†), and this corresponds to the increase in light absorption.38
Owing to the abundant OVs, the photocatalytic NRR of small catalyst nanoparticles was performed under light irradiation (λ > 300 nm) using water and N2 (99.999%) as raw materials without any sacrificial reagents or co-catalysts. The NH4+ concentration was detected using Nessler's reagent and ion chromatography methods. As shown in Fig. 3a and S11,† TiO2-blank exhibits an activity of 228 μmol h−1 g−1, which is attributable to a large number of active OVs that enhance N2 adsorption and activation.7,39 As the concentration of OVs increases, the efficiency of Eu52Ni56/TiO2-3 increases to 803 μmol h−1 g−1, which is 3.5 times higher than that of TiO2-blank. The subsequent increase in loading clusters causes a decrease in NRR performance, which is attributed to the large number of clusters covering the active site and reducing the OV concentration.18 A contrast experiment was performed, under an argon atmosphere, to trace the source of ammonia (Fig. 3b and S12†). When argon was substituted with nitrogen as the feedstock gas, trace activity was observed, which confirmed that N2 was the origin of NH3. Another control photocatalytic NRR experiment was conducted with Eu3+, Ni2+, and iminodiacetic acid (H2IDA). The obtained response was even worse than that of TiO2-blank (Fig. 3b and S12†), which convincingly illustrated the key role of Eu52Ni56 cluster. Five cycles of testing (each run for 1 h, with washing and drying after each run) revealed that Eu52Ni56/TiO2-3 still maintained relatively adequate activity (Fig. 3c), and there was no distinct change in the morphology (Fig. S19 and S20†), indicating high stability. Composite catalysts with different lanthanide elements were studied to compare their performance, since different lanthanide elements may play different roles. Among the isostructural Ln52Ni56/TiO2 series, Eu52Ni56/TiO2 exhibits the best pNRR performance (Fig. 3d), indicating that Eu plays a key role in the entire catalytic system.
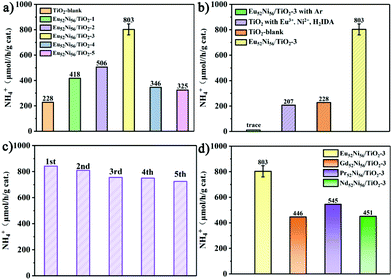 |
| Fig. 3 (a) N2 reduction activities of TiO2-blank and Eu52Ni56/TiO2-1, 2, 3, 4, 5. (b) Controlled experiments with TiO2-blank and Eu52Ni56/TiO2-3. (c) Five catalytic cycles of Eu52Ni56/TiO2-3. (d) N2 reduction activities of Eu52Ni56/TiO2-3, Gd52Ni56/TiO2-3, Pr52Ni56/TiO2-3, and Nd52Ni56/TiO2-3. | |
Catalytic efficiency gradually decreases during the photocatalytic reaction, and the ammonia yield reaches equilibrium at 5 h (Fig. S13†). Although this phenomenon has been reported in some photocatalytic NRR systems, none of studies discussed the reasons for this decrease in activity. A recent study suggested that a similar phenomenon occurred owing to the occupation of zinc vacancies.40 To further investigate the mechanism for the decrease in NRR activity and the changes in OV species under light excitation, in situ EPR was conducted at 293 K. As shown in Fig. S17 and S18,† the OV signal of the Eu52Ni56/TiO2-3 catalyst shows an obvious decrease after light irradiation. The decrease in OVs is caused by not only N2 capture but also some oxygen species present at the OV positions. For example, large amounts of water molecules in the reaction can act as competing species for N2 to occupy the OVs. If the combined water and oxygen species on the catalysts are removed, the OVs may be reexposed and reactivated. Following the NRR reaction, the EPR of the wet sample was performed at 110 K. As shown in Fig. 4a, the EPR spectrum of the wet sample shows a weak signal at g = 2.001 after the photoreaction. This suggests that most of the catalyst is deactivated. After drying at 80 °C for 24 h, the EPR results of the deactivated catalyst show enhanced OV signals, indicating that the OVs were restored after drying. The EPR results before and after drying not only illustrate the important role of OVs but also provide strong evidence for the mechanisms of catalyst deactivation and recovery.
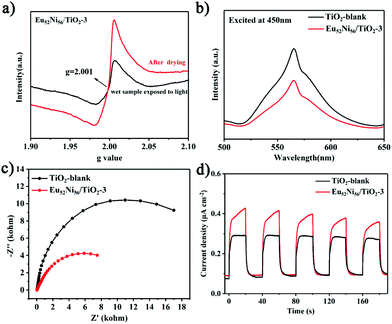 |
| Fig. 4 (a) EPR spectra of wet Eu52Ni56/TiO2-3 after light irradiation and after drying at 110 K. (b) Photoluminescence spectra of TiO2-blank and Eu52Ni56/TiO2-3 with excitation at 450 nm. (c) Electrochemical impedance spectroscopy of TiO2-blank and Eu52Ni56/TiO2-3. (d) Transient photocurrent response of TiO2-blank and Eu52Ni56/TiO2-3 under simulated irradiation and off. | |
To investigate the NRR mechanism of Eu52Ni56/TiO2, photoluminescence spectra were recorded. As shown in Fig. 4b, the emission spectrum of Eu52Ni56/TiO2-3 under excitation at 450 nm exhibits a lower signal than that of TiO2-blank. This indicates that the recombination of the excited electrons and holes of Eu52Ni56/TiO2-3 is significantly suppressed compared to that of TiO2-blank. Time-resolved steady state photoluminescence (PL) spectra show that the lifetimes of TiO2-blank and Eu52Ni56/TiO2-3 are 1.05 and 1.31 ns, respectively (Fig. S21†). The lifetime increase of Eu52Ni56/TiO2-3 indicates an increase in the lifetime of charge carriers.18,41 Electrochemical impedance spectroscopy (EIS) and transient photocurrent response (TPC) were performed to further investigate the transfer efficiency. As shown in Fig. 4c and d, Eu52Ni56/TiO2-3 has a smaller Nyquist radius and a higher photocurrent response. This confirms that Eu52Ni56/TiO2-3 has a lower interfacial charge transfer resistance and higher electron–hole separation and transfer efficiency compared to those of TiO2-blank. These results suggest that the rapid charge separation caused by assembling atomically precise clusters on semiconductors is also an important factor in improving photocatalytic performance.27,42
Mott–Schottky (MS) analyses show that the CB of Eu52Ni56/TiO2-3 is −0.34 V vs. the normal hydrogen electrode (NHE), which is positive than that of TiO2-blank (−0.47 V vs. NHE) (Fig. S22 and S23†). The UV-vis diffuse reflectance spectroscopy (UV-DRS) profile shows that the bandgaps for Eu52Ni56/TiO2-3 and TiO2-blank are 3.08 and 3.20 eV, respectively (Fig. S24†). From calculations, the VB of Eu52Ni56/TiO2-3 and TiO2-blank are located at 2.74 and 2.73 eV, respectively (Fig. 5 and S25†).43,44 A narrow band gap can promote the absorption of light energy and improve catalytic activity.45 According to Mott–Schottky spots and UV-vis DRS of Ln52Ni56, the LUMO energy levels of Eu52Ni56, Gd52Ni56, Pr52Ni56, and Nd52Ni56, which are −0.28, −0.26, −0.43, and −0.38 V. vs. NHE, respectively (Fig. S26–29†). Therefore, there is a natural driving force (potential difference) wherein the active electrons in the CB of TiO2-blank can be transferred to the LUMO energy of Ln52Ni56, improving the efficiency of charge separation (Fig. S30†). The energy level of the Eu3+/Eu2+, Nd3+/Nd2+, Gd3+/Gd, and Pr3+/Pr2+ reduction potentials are −0.35, −2.7, −2.28 and −3.1 V, respectively.46,47 The CB of TiO2-blank (−0.47 V) is negative than the energy level of the Eu3+/Eu2+ reduction potential (−0.35 V), indicating that the photoexcited electrons of TiO2-blank can transfer to Eu3+ (Fig. S31a†). However, the energy levels of Nd3+/Nd2+, Gd3+/Gd and Pr3+/Pr2+ reduction potentials are more negative than the CB of TiO2-blank, resulting in the photoexcited electrons of TiO2-blank cannot transfer to the Nd3+, Pr3+ and Gd3+ with only migrate to the LUMO energy level of Nd52Ni56, Gd52Ni56, and Pr52Ni56 with a single path (Fig. S31b†). So, the dual-channel charge transfer of Eu52Ni56/TiO2 to Eu3+ and LUMO energy level of Eu52Ni56 leads to efficient charge separation efficiency and high catalytic activity (Fig. 3d and 5).
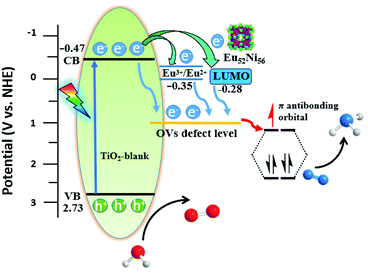 |
| Fig. 5 Charge separation and transfer in the Eu52Ni56/TiO2-3 composite under light irradiation. VB: valence band; CB: conduction band. | |
Combined with the above analysis, the entire process of the pNRR can be described as follows. Under light irradiation, the photogenerated electrons are quickly transferred to the CB of TiO2-blank. Most of them recombine with photogenerated cavities, and a small portion is quickly captured by the OV defect.16 Meanwhile, owing to the well-matched energy levels between TiO2 and Eu52Ni56, some of the electrons in the CB of TiO2 can be transferred to the LUMO energy level and Eu3+ of Eu52Ni56, which suppresses the recombination of photogenerated carriers.48 Because of the lower energy of defect levels, all transferred electrons eventually converge on the OVs that act as trapping sites.18,49 Subsequently, electrons are injected into the empty anti-bonding orbitals (π*) of adsorbed N2, which is accompanied by N
N triple-bond activation and hydrogenation, resulting in the generation of NH3.7,49 The photoinduced holes in the VB of TiO2-blank, which are oxidation of water into oxygen, and completion of the entire catalytic process. The Eu52Ni56 cluster plays an important synergistic role in improving catalytic activity.
Conclusions
In summary, we reported a method for enhanced photocatalytic nitrogen fixation by loading atomically precise 4f–3d clusters onto TiO2 semiconductors. A composite photocatalyst, Ln52Ni56/TiO2, with abundant OVs was prepared using a simple solvothermal method. The investigation of photocatalytic nitrogen fixation showed that Ln52Ni56, not only acts as a tool to improve charge separation but also serves as a loading species to increase the number of OVs. Eu52Ni56/TiO2-3 displayed the highest photocatalytic N2 activity of 800 μmol h−1 g−1 using nitrogen and water as raw materials without any sacrificial agents or co-catalysts. Notably, this study also revealed the mechanism of catalyst deactivation due to the occupation of OVs by reactive oxygen species in photocatalytic nitrogen fixation. This study suggests a new optimization method for defect and energy bond engineering by assembling atomically precise clusters on the surface of semiconductor materials.
Conflicts of interest
There are no conflicts to declare.
Acknowledgements
This work was supported by the National Natural Science Foundation of China (Grants 21871224, 92161104, 92161203, and 21721001) and Innovation Laboratory for Sciences and Technologies of Energy Materials of Fujian Province (IKKEM Grant RD2021040301).
Notes and references
- J. W. Erisman, M. A. Sutton, J. Galloway, Z. Klimont and W. Winiwarter, How a century of ammonia synthesis changed the world, Nat. Geosci., 2008, 1, 636–639 CrossRef CAS.
- S. Licht, B. Cui, B. Wang, F.-F. Li, J. Lau and S. Liu, Ammonia synthesis by N2 and steam electrolysis in molten hydroxide suspensions of nanoscale Fe2O3, Science, 2014, 345, 637–640 CrossRef CAS PubMed.
- J. A. Pool, E. Lobkovsky and P. J. Chirik, Hydrogenation and cleavage of dinitrogen to ammonia with a zirconium complex, Nature, 2004, 427, 527–530 CrossRef CAS PubMed.
- J. M. P. Martirez and E. A. Carter, Excited-state N2 dissociation pathway on Fe-functionalized Au, J. Am. Chem. Soc., 2017, 139, 4390–4398 CrossRef CAS PubMed.
- C. J. M. van der Ham, M. T. M. Koper and D. G. H. Hetterscheid, Challenges in reduction of dinitrogen by proton and electron transfer, Chem. Soc. Rev., 2014, 43, 5183–5191 RSC.
- G. Dong, W. Ho and C. Wang, Selective photocatalytic N2 fixation dependent on g-C3N4 induced by nitrogen vacancies, J. Mater. Chem. A, 2015, 3, 23435–23441 RSC.
- H. Li, J. Shang, Z. Ai and L. Z. Zhang, Efficient visible light nitrogen fixation with BiOBr nanosheets of oxygen vacancies on the exposed {001} facets, J. Am. Chem. Soc., 2015, 137, 6393–6399 CrossRef CAS PubMed.
- G. N. Schrauzer and T. D. Guth, Photolysis of water and photoreduction of nitrogen on titanium dioxide, J. Am. Chem. Soc., 1977, 99, 7189–7193 CrossRef CAS.
- Y. F. Zhao, Y. X. Zhao, G. I. N. Waterhouse, L. R. Zheng, X. Z. Cao, F. Teng, L. Z. Wu, C. H. Tung, D. O'Hare and T. R. Zhang, Layered-Double-Hydroxide nanosheets as efficient visible-light-driven photocatalysts for dinitrogen fixation, Adv. Mater., 2017, 29, 1703828 CrossRef PubMed.
- B. M. Comer, Y.-H. Liu, M. B. Dixit, K. B. Hatzell, Y. Ye, E. J. Crumlin, M. C. Hatzell and A. J. Medford, The role of adventitious carbon in photo-catalytic nitrogen fixation by titania, J. Am. Chem. Soc., 2018, 140, 15157–15160 CrossRef CAS PubMed.
- H. Li, C. Mao, H. Shang, Z. Yang, Z. Ai and L. Z. Zhang, New opportunities for efficient N2 fixation by nanosheet photocatalysts, Nanoscale, 2018, 10, 15429–15435 RSC.
- C. Lv, Y. Qian, C. Yan, Y. Ding, Y. Liu, G. Chen and G. Yu, Defect engineering metal-free polymeric carbon nitride electrocatalyst for effective nitrogen fixation under ambient conditions, Angew. Chem., Int. Ed., 2018, 57, 10246–10250 CrossRef CAS PubMed.
- J. Qian, S. Zhao, W. Dang, Y. Liao, W. Zhang, H. Wang, L. Lv, L. Luo, H.-Y. Jiang and J. Tang, Photocatalytic nitrogen reduction by Ti3C2 MXene derived oxygen vacancy-rich C/TiO2, Adv. Sustainable Syst., 2021, 5, 2000282 CrossRef CAS.
- Y. Tong, H. Guo, D. Liu, X. Yan, P. Su, J. Liang, S. Zhou, J. Liu, G. Q. Lu and S. X. Dou, Vacancy engineering of Iron-Doped W18O49 nanoreactors for Low-Barrier electrochemical nitrogen reduction, Angew. Chem., Int. Ed., 2020, 59, 7356–7361 CrossRef CAS PubMed.
- N. Zhang, A. Jalil, D. Wu, S. Chen, Y. Liu, C. Gao, W. Ye, Z. Qi, H. Ju, C. Wang, X. Wu, L. Song, J. Zhu and Y. Xiong, Refining defect states in W18O49 by Mo doping: A strategy for tuning N2 activation towards solar-driven nitrogen Fixation, J. Am. Chem. Soc., 2018, 140, 9434–9443 CrossRef CAS PubMed.
- J. Li, D. Wang, R. Guan, Y. Zhang, Z. Zhao, H. Zhai and Z. Sun, Vacancy-Enabled mesoporous TiO2 modulated by nickel doping with enhanced photocatalytic nitrogen fixation performance, ACS Sustainable Chem. Eng., 2020, 8, 18258–18265 CrossRef CAS.
- N. Zhang, L. Li, Q. Shao, T. Zhu, X. Huang and X. Xiao, Fe-Doped BiOCl nanosheets with light-switchable oxygen vacancies for photocatalytic nitrogen fixation, ACS Appl. Energy Mater., 2019, 2, 8394–8398 CrossRef CAS.
- Y. Zhao, Y. Zhao, R. Shi, B. Wang, G. I. N. Waterhouse, L.-Z. Wu, C.-H. Tung and T. R. Zhang, Tuning oxygen vacancies in ultrathin TiO2 nanosheets to boost photocatalytic nitrogen fixation up to 700 nm, Adv. Mater., 2019, 31, 1806482 CrossRef PubMed.
- P. Li, Z. Zhou, Q. Wang, M. Guo, S. Chen, J. Low, R. Long, W. Liu, P. Ding, Y. Wu and Y. J. Xiong, Visible-Light-Driven nitrogen fixation catalyzed by Bi5O7Br nanostructures: Enhanced performance by oxygen vacancies, J. Am. Chem. Soc., 2020, 142, 12430–12439 CrossRef CAS PubMed.
- J. Yang, Y. Guo, R. Jiang, F. Qin, H. Zhang, W. Lu, J. Wang and J. C. Yu, High-Efficiency “Working-in-Tandem” nitrogen photofixation achieved by assembling plasmonic gold nanocrystals on ultrathin titania nanosheets, J. Am. Chem. Soc., 2018, 140, 8497–8508 CrossRef CAS PubMed.
- Q. Wu, Q. Zheng and R. van de Krol, Creating oxygen vacancies as a novel strategy to form tetrahedrally coordinated Ti4+ in Fe/TiO2 nanoparticles, J. Phys. Chem. C, 2012, 116, 7219–7226 CrossRef CAS.
- R. Guan, H. Zhai, J. Li, Y. Qi, M. Li, M. Song, Z. Zhao, J. Zhang, D. Wang and H. Tan, Reduced mesoporous TiO2 with Cu2S heterojunction and enhanced hydrogen production without noble metal cocatalyst, Appl. Surf. Sci., 2020, 507, 144772 CrossRef CAS.
- T. Hisatomi, J. Kubota and K. Domen, Recent advances in semiconductors for photocatalytic and photoelectrochemical water splitting, Chem. Soc. Rev., 2014, 43, 7520–7535 RSC.
- R. Chen, Z. H. Yan and X. J. Kong, Recent advances in First-Row transition metal clusters for photocatalytic water splitting, ChemPhotoChem, 2020, 4, 157–167 CrossRef CAS.
- Z.-H. Pan, Z.-Z. Weng, X.-J. Kong, L.-S. Long and L.-S. Zheng, Lanthanide-containing clusters for catalytic water splitting and CO2 conversion, Coord. Chem. Rev., 2022, 457, 214419 CrossRef CAS.
- Z. H. Yan, M. H. Du, J. X. Liu, S. Y. Jin, C. Wang, G. L. Zhuang, X. J. Kong, L. S. Long and L. S. Zheng, Photo-generated dinuclear {Eu(II)}2 active sites for selective CO2 reduction in a photosensitizing metal-organic framework, Nat. Commun., 2018, 9, 3353 CrossRef PubMed.
- R. Chen, Z. H. Yan, X. J. Kong, L. S. Long and L. S. Zheng, Integration of Lanthanide-Transition-Metal clusters onto CdS surfaces for photocatalytic hydrogen evolution, Angew. Chem., Int. Ed., 2018, 57, 16796–16800 CrossRef CAS PubMed.
- X. J. Kong, Y. P. Ren, W. X. Chen, L. S. Long, Z. Zheng, R. B. Huang and L. S. Zheng, A four-shell, nesting doll-like 3d–4f cluster containing 108 metal ions, Angew. Chem., Int. Ed., 2008, 47, 2398–2401 CrossRef CAS PubMed.
- T. Wu, X. Zhu, Z. Xing, S. Mou, C. Li, Y. Qiao, Q. Liu, Y. Luo, X. Shi, Y. Zhang and X. Sun, Greatly improving electrochemical N2 reduction over TiO2 nanoparticles by Iron doping, Angew. Chem., Int. Ed., 2019, 58, 18449–18453 CrossRef CAS PubMed.
- C.-Y. Wang, C. Böttcher, D. W. Bahnemann and J. K. Dohrmann, A comparative study of nanometer sized Fe(iii)-doped TiO2 photocatalysts: synthesis, characterization and activity, J. Mater. Chem., 2003, 13, 2322–2329 RSC.
- J. Yang, H. Bai, Y. Guo, H. Zhang, R. Jiang, B. Yang, J. Wang and J. C. Yu, Photodriven disproportionation of nitrogen and its change to reductive nitrogen photofixation, Angew. Chem., Int. Ed., 2021, 60, 927–936 CrossRef CAS PubMed.
- Z. Yao, F. Jia, S. Tian, C. Li, Z. Jiang and X. Bai, Microporous Ni-Doped TiO2 film photocatalyst by Plasma electrolytic oxidation, ACS Appl. Mater. Interfaces, 2010, 2, 2617–2622 CrossRef CAS PubMed.
- E. M. Neville, M. J. Mattle, D. Loughrey, B. Rajesh, M. Rahman, J. M. D. MacElroy, J. A. Sullivan and K. R. Thampi, Carbon-Doped TiO2 and carbon, Tungsten-Codoped TiO2 through Sol–Gel processes in the presence of melamine borate: Reflections through photocatalysis, J. Phys. Chem. C, 2012, 116, 16511–16521 CrossRef CAS.
- S. Wang, X. Hai, X. Ding, K. Chang, Y. Xiang, X. Meng, Z. Yang, H. Chen and J. Ye, Light-Switchable oxygen vacancies in ultrafine Bi5O7Br nanotubes for boosting solar-driven nitrogen fixation in pure water, Adv. Mater., 2017, 29, 1701774 CrossRef PubMed.
- Y. Horiuchi, T. Toyao, M. Saito, K. Mochizuki, M. Iwata, H. Higashimura, M. Anpo and M. Matsuoka, Visible-Light-Promoted photocatalytic hydrogen production by using an amino-functionalized Ti(IV) metal–organic framework, J. Phys. Chem. C, 2012, 116, 20848–20853 CrossRef CAS.
- Z. Li, J.-D. Xiao and H.-L. Jiang, Encapsulating a Co(II) molecular photocatalyst in Metal–Organic Framework for visible-light-driven H2 Production: Boosting catalytic efficiency via spatial charge separation, ACS Catal., 2016, 6, 5359–5365 CrossRef CAS.
- G. Liu, Y. Zhao, C. Sun, F. Li, G. Q. Lu and H.-M. Cheng, Synergistic effects of B/N doping on the visible-light photocatalytic activity of mesoporous TiO2, Angew. Chem., Int. Ed., 2008, 47, 4516–4520 CrossRef CAS PubMed.
- G. Colón, M. Maicu, M. C. Hidalgo and J. A. Navío, Cu-doped TiO2 systems with improved photocatalytic activity, Appl. Catal., B, 2006, 67, 41–51 CrossRef.
- H. Hirakawa, M. Hashimoto, Y. Shiraishi and T. Hirai, Photocatalytic conversion of nitrogen to ammonia with water on surface oxygen vacancies of titanium dioxide, J. Am. Chem. Soc., 2017, 139, 10929–10936 CrossRef CAS PubMed.
- H. Han, Y. Yang, J. Liu, X. Zheng, X. Wang, S. Meng, S. Zhang, X. Fu and S. Chen, Effect of Zn vacancies in Zn3In2S6 nanosheets on boosting photocatalytic N2 fixation, ACS Appl. Energy Mater., 2020, 3, 11275–11284 CrossRef CAS.
- Y. Bo, H. Wang, Y. Lin, T. Yang, R. Ye, Y. Li, C. Hu, P. Du, Y. Hu, Z. Liu, R. Long, C. Gao, B. Ye, L. Song, X. Wu and Y. J. Xiong, Altering hydrogenation pathways in photocatalytic nitrogen fixation by tuning local electronic structure of oxygen vacancy with dopant, Angew. Chem., Int. Ed., 2021, 60, 16085–16092 CrossRef CAS PubMed.
- R. Chen, G.-L. Zhuang, Z.-Y. Wang, Y.-J. Gao, Z. Li, C. Wang, Y. Zhou, M.-H. Du, S. Zeng, L.-S. Long, X.-J. Kong and L.-S. Zheng, Integration of bio-inspired lanthanide-transition metal cluster and P-doped carbon nitride for efficient photocatalytic overall water splitting, Natl. Sci. Rev., 2021, 8, nwaa234 CrossRef CAS PubMed.
- M. K. Nowotny, L. R. Sheppard, T. Bak and J. Nowotny, Defect chemistry of titanium dioxide. Application of defect engineering in processing of TiO2-Based photocatalysts, J. Phys. Chem. C, 2008, 112, 5275–5300 CrossRef CAS.
- M. Xing, J. Zhang, F. Chen and B. Tian, An economic method to prepare vacuum activated photocatalysts with high photo-activities and photosensitivities, Chem. Commun., 2011, 47, 4947–4949 RSC.
- X. Chen, N. Li, Z. Kong, W.-J. Ong and X. Zhao, Photocatalytic fixation of nitrogen to ammonia: state-of-the-art advancements and future prospects, Mater. Horiz., 2018, 5, 9–27 RSC.
-
A. J. Bard and L. R. Faulkner, Electrochemical Methods: Fundamentals and Applications, 2nd edn, Wiley, 2000 Search PubMed.
-
D. R. Lide, G. Baysinger and L. Berger, CRC Handbook of Chemistry and Physics, 87th edn, CRC, 2007, p. 1438 Search PubMed.
- L. Shi, Z. Li, L. Ju, A. Carrasco-Pena, N. Orlovskaya, H. Zhou and Y. Yang, Promoting nitrogen photofixation over a periodic WS2@TiO2 nanoporous film, J. Mater. Chem. A, 2020, 8, 1059–1065 RSC.
- Y. Li, X. Chen, M. Zhang, Y. Zhu, W. Ren, Z. Mei, M. Gu and F. Pan, Oxygen vacancy-rich MoO3−x nanobelts for photocatalytic N2 reduction to NH3 in pure water, Catal. Sci. Technol., 2019, 9, 803–810 RSC.
Footnote |
† Electronic supplementary information (ESI) available: Sample preparation; characterization; including Fig. S1–S31 and Table S1. See DOI: https://doi.org/10.1039/d2qi00628f |
|
This journal is © the Partner Organisations 2022 |