DOI:
10.1039/C0JA00153H
(Critical Review)
J. Anal. At. Spectrom., 2011,
26, 23-29
Direct speciation analysis of inorganic elements in single cells using X-ray absorption spectroscopy†
Received
9th September 2010
, Accepted 11th November 2010
First published on 24th November 2010
Abstract
Up to now, X-ray absorption spectroscopy (XAS), which includes XANES (X-ray absorption near edge structure) and EXAFS (Extended X-ray absorption fine structure), was mainly used with unfocused beams. The recent development of X-ray focusing optics, with spatial resolution down to the micrometre level while maintaining high photon fluxes, has opened the field of XAS application to single cell analysis. Micro-XAS enables the characterization of the local structure of the elements within subcellular structures in terms of oxidation state, site ligation, and coordination. It has been mainly applied to the determination of trace metal oxidation states using micro-XANES, and in some favorable cases to identify the molecules binding to the metals in cells. Due to the minute quantity of analytes in subcellular compartments, micro-EXAFS is still more challenging to perform than micro-XANES as it requires higher signal to noise ratios. Micro-XAS has proven to be useful in various fields of research such as metal-based neuro-degeneration, cellular pharmacology, trace element physiology and metal toxicology. Micro-XAS presents unique capabilities over other speciation methods because it can be performed in situ, directly in subcellular compartments, without cell fractionation which is prone to modify the chemical element species. However, the stability of chemical element species all along the analytical procedure, from sample preparation to storage and analysis should be tested systematically.
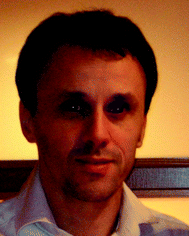 R. Ortega | Richard Ortega is research director at CNRS and Bordeaux University, France. His research focus is on the development of analytical methods based on ion beams and synchrotron radiation for the imaging and speciation of metals in cells and proteins. He obtained a Chemical engineer and Master Degree in Chemistry from the University of Marseille (1991), and a Ph.D. in Physics at the University of Bordeaux (1994). He was a Postdoctoral fellow with Pr. Bibudhendra Sarkar at the Research Institute of the Hospital for Sick Children, Canada (1995). He was an invited scientist at the European Synchrotron Radiation Facility (2001) and gained an award from the French Society of Chemistry in Analytical Chemistry (2004). |
Introduction
Mechanisms of metal bioavailability, homeostasis, toxicity or even pharmacology are governed by the chemical state of the elements within cells. A challenging issue in speciation analysis is the direct determination of chemical element species in single cells. Such information is needed to understand the biochemistry of trace metals in cells. The recent development of highly focused hard X-ray synchrotron beams has opened the application of X-ray absorption spectroscopy (XAS) to microscopic samples such as single cells. Since the development of synchrotron radiation sources XAS has been widely employed to unravel the local atomic geometry and electronic structure of chemical elements. XAS offers angstrom resolution to study the structural chemistry of the elements. When XAS is performed with a microbeam it allows the chemical speciation within subcellular compartments. Direct speciation analysis using micro-XAS in cells avoids cell fractionation and other preparation steps that might modify the chemical species present. This article presents data on the advantages and limitations of micro-XAS and is illustrated with recent achievements at the cellular level.
Principle of XAS
When the incident energy of X-rays is equal to the binding energy of a core electron (at layers K, L or M), the electron is ejected (photoelectric effect), thus creating a gap in the distribution of the electrons in the electronic shells, leaving the atom in an excited state. To stabilize the system, the excited atom relaxes by reorganization of the electronic shells according to a process of X-ray fluorescence or emission of Auger electron. XAS refers to the spectral features of the X-ray absorption coefficient (μ) around and above the absorption edge of a given element (Fig. 1).
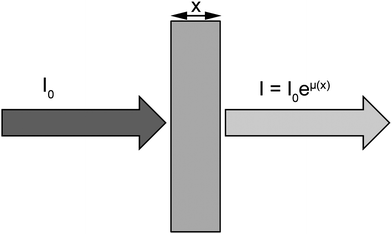 |
| Fig. 1
X-ray absorption spectroscopy refers to the variation of the absorption coefficient μ. I0 is the intensity of the incoming X-ray beam. After the X-rays have traversed a distance x into the sample, the intensity has been reduced to I = I0eμ(x), where μ is the absorption coefficient. | |
Each absorption edge is related to a specific atom present in the material and, more specifically, to a quantum-mechanical transition that excites a particular atomic core-orbital electron to the free or unoccupied continuum levels. The energies of the edges are unique to the type of atom that absorbs the x ray, and hence themselves are signatures of the atomic species present in a material. The excitation source to produce the photoelectric effect is usually provided by a synchrotron radiation X-ray beam. The energy of the incident X-rays is tuned around the absorption edge energy of the studied element, for example 7112 eV for Fe K-edge (Fig. 2). Local structural information on the absorber element can be deduced by analyzing oscillations in X-ray absorption versusphoton energy that are caused by the scattering of the X-ray excited photoelectrons. XAS can be divided into X-ray absorption near edge structure (XANES), which provides information primarily about geometry and oxidation state, and extended X-ray absorption fine structure (EXAFS), which provides information about metal site ligation. From the physical stand point, the EXAFS region relates to the scattering of the photoelectron with the neighboring atoms, while the XANES region is often dominated by strong multiple scattering processes as well as local atomic resonances in the X-ray absorption that cannot be generally interpreted by EXAFS. Indeed, the essential difference between EXAFS and XANES is found to lie in the strength of the scattering, with the crossover energy being typically about 30 eV or less. A detailed description of the theoretical bases of EXAFS and XANES can be found in a review from Rehr and Albers.1
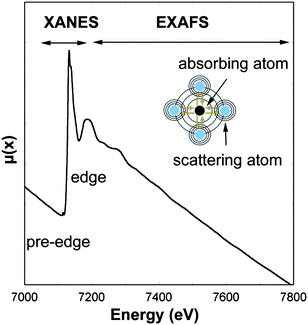 |
| Fig. 2 X-ray absorption spectra at Fe K-edge (7112 eV). The spectrum region around the absorption edge (XANES, X-ray absorption near edge structure) relates to the photoelectron multiple scattering regime and local atomic resonances, while the region above the absorption edge (EXAFS, extend X-ray absorption fine structure) relates both to the simple and multiple photoelectron scattering. | |
XAS is usually performed by measuring the photons transmitted through the sample; however for diluted analytes, such as trace metals in biological samples, XAS is performed in fluorescence mode which is much more sensitive than the absorption spectroscopy.
The development of micro-XAS on synchrotron radiation facilities is strongly related to the continuous improvements in hard X-ray focusing optics over the past decade. The focusing of high energy photons produced by synchrotron radiation sources ranging typically from 1 to several tens of keV is a difficult achievement. Five types of microfocusing optics can be used to obtain micrometre or even nanometre size X-ray beams: Kirkpatrick–Baez based mirrors, X-ray capillaries, waveguides, Fresnel zone plates, and compound refractive lenses. All these optics are intensively studied and are in constant development. The characteristics and use of these devices at synchrotron radiation facilities has been recently reviewed by Snigirev and Snigireva.2
Micro-XAS for speciation in single cells
XAS has been particularly useful for the bulk chemical speciation of dilute samples of biological concern.3,4 Micro-XAS is a more recent achievement but it can be anticipated that micro-XAS applications will increase in the near future because of the continuous development of highly focused X-ray beamlines on most synchrotron radiation facilities. Micro-XAS capabilities strongly depend on the characteristics of the X-ray beamline where it is carried out. It can be estimated that in order to perform chemical element speciation within single cells, the beam size should be at least in the micrometre range size, or less, while the intensity of the focused beam must be high enough to perform X-ray absorption spectroscopy, with about 109 ph/s, or more. For example, micro-XAS was performed on single cells at ESRF on ID22 beamline with an experimental setup based on Kirkpatrick-Baez X-ray focusing optics that maintains high flux of incoming radiation (>1011photons/s at 11.9 keV) at micrometric spatial resolution (1.5 × 4.0 μm2).5 With this experimental setup, the limit of detection, as evaluated from the standard deviation of eight blank measures, was 4.3 μg g−1. Similarly to the concept of “limit of quantification” used for quantitative analytical methods, a “limit of speciation” could be evaluated and was about 13 μg g−1. These values are only indicative because they strongly depend on the experimental setup (detection system, distance from sample to detector,…) and also on the element and the nature of the sample. However, the values are helpful for a rough estimate of the feasibility of micro-XANES experiments as similar detection levels are expected for elements of atomic number between 20 and 40, because the cross section for X-ray fluorescence and the detection efficiency do not vary much for these elements. In this experiment, speciation analysis was limited to the use of micro-XANES and not of micro-EXAFS. The limit of speciation for micro-EXAFS has not been measured exactly yet. The micro-XAS signal in single cells is low compared to macroscopic analysis first because the elements of interest, the trace metals, are usually diluted in the biological samples, and second because of the minute quantity of matter in a single cell. Therefore, XAS is often limited to the study of the absorption edge region (XANES) in single cells giving information about the oxidation state of the absorber element. The EXAFS data treatment requires high signal to noise acquisitions in order to fit the oscillations after the absorption edge which is difficult to obtain in most cases on single cells. Only a few examples of micro-EXAFS analysis in cells have been reported so far (Table 1). Another important limitation of micro-XAS is the influence of intense X-ray irradiation on element chemical state stability during analysis. Photo-oxidation and photo-reduction processes can occur during the irradiation. These processes are variable depending on the element and its initial oxidation state, and the analytical conditions: intensity of the X-ray beam, temperature of the sample, type of environment i.e., presence of oxygen in air,… Low temperature analysis at liquid nitrogen temperature is highly advocated but not necessarily sufficient. A systematic analysis of proper reference material must be performed, even when low temperature and neutral gas environment is used. The reference material, usually chemical compounds of known oxidation state, must be irradiated within exactly the same analytical conditions as the samples to verify that the element chemical state is not modified during analysis.5
Table 1 Applications of micro-XAS to element speciation in single cells
Element |
Chemical species identified |
Spectroscopy
|
References |
As |
As(III); As(V) |
μ-XANES |
5,19
|
Cr
|
Cr(III); Cr(VI) |
μ-XANES |
16,17
|
Chromium phosphate
|
μ-XANES |
27
|
Polynuclear Cr |
μ-EXAFS |
17
|
Cu
|
Cu(I); Cu(II) |
μ-XANES |
15,23,25
|
Fe
|
Fe(II); Fe(III) |
μ-XANES |
7,8,11,12,13,14,23,28,29
|
Fe in ferritin and magnetite |
μ-XANES |
14
|
Pt
|
Pt(II); Pt(IV) |
μ-XANES |
21
|
V |
V(III); V(IV) |
μ-XANES |
24
|
Zn
|
Zn(II)
|
μ-XANES |
22,23
|
Zn binding to S |
μ-XANES/EXAFS |
26
|
The main application of micro-XAS in single cells is the study of redox metals by micro-XANES. Oxidation state is an important parameter that defines the bioavailability and intracellular reactivity of biologically relevant metals. Micro-XANES is probably the unique analytical method available to probein situ the oxidation state of trace elements within subcellular compartments. One alternative method for oxidation state determination in subcellular compartments is EELS (Electron Energy Loss Spectroscopy) but very few examples of applications have been published,6 probably because sample preparation for EELS is very challenging. The size of the organelles that can be probed using micro-XANES depends on the size of the microbeam, typically with a 1 μm beam size it is possible to probe the nucleus, cytoplasm, lysosomes, vesicles, and the mitochondrial network. This is a real advantage over other speciation methods as it avoids cellular fractionation using differential centrifugation that can lead to changes of chemical species during sample preparation. The two first studies that demonstrated the feasibility of micro-XANES on single cells were both applied to the examination of Fe oxidation state.7,8 The redox cycling of Fe, Fe(II)/Fe(III), is known to participate to the oxidative stress through the Fenton reaction and could be involved in a number of pathological processes such as neurodegenerative diseases9 or cancer.10 Since these early studies, micro-XAS of single cells has been applied in studies of metal-based neuro-degeneration, metal carcinogenesis, cellular pharmacology of anticancer drugs, trace element physiology and environmental metals speciation in prokaryotes. The next section reviews the existing literature in order to illustrate with practical cases what type of structural information can be obtained with micro-XAS. The results are summarized in Table 1.
Speciation of redox metals in neuronal cells
Redox metals are suspected to be involved in the etiology of number of neurodegenerative diseases such as Alzheimer's disease or Parkinson's disease. A hyperoxidation process due to the presence in excess of Fe(II) that exacerbates the production of free radicals has been suggested to play a critical role in neuronal cell death. Micro-XANES can be used to identify Fe(II) and Fe(III) from differences in their absorption edge energies and pre-edge features. For example Fe was shown to be present as Fe(III) in the nucleus and cytoplasm of dopaminergic cells cultured in vitro in normal conditions (Fig. 3).5 In pathological tissues, mixed valences of Fe(II)/Fe(III) have been observed in a single neuron from a Parkinson's disease patient.8 Mixed valences of Fe(II)/Fe(III) were also observed using micro-XANES on animal models of Parkinson's disease—monkeys injected with MPTP, a chemical that induces the specific degeneration of dopamine neurons.11 The presence of Fe(II) in pathological tissues of Parkinson's disease was hypothesized. However, some recent micro-XANES studies of human brains did not show any significant differences in iron oxidation state between Parkinson's disease patients and controls.12,13Iron was present only as Fe(III), presumably stored as ferritin. In a study of Alzheimer's disease patients, anomalous high iron concentrations were identified corresponding to mixed Fe(II)/Fe(III) valences in the Fe rich areas.14 By comparison with ferritin and magnetite reference compounds, micro-XANES spectra revealed that iron rich structures found in Alzheimer's disease were either mixtures of ferritin and magnetite, or purely magnetite, a magnetic iron oxide, potentially indicating disrupted brain-iron metabolism.
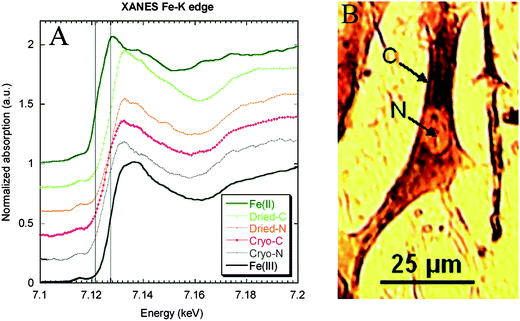 |
| Fig. 3
XANES at Fe K-edge on subcellular compartments (cytoplasm and nucleus) of single dopaminergic cells. Micro-XANES was performed at the subcellular level on a freeze-dried cell (cytoplasm: Dried-C and nucleus: Dried-N) and on a frozen-hydrated cell (cytoplasm: Cryo-C and nucleus: Cryo-N). Micro-XANES spectra were compared to Fe(II) and Fe(III) spectra of reference compounds. The vertical lines represent Fe(II) and Fe(III) first-derivative edge positions, respectively, at 7122.9 and 7128.7 eV. The spectra show that iron is present in its Fe(III) oxidation state in all cell compartments both in freeze-dried and frozen hydrated cells. Image reproduced by permission of the American Chemical Society (2007).5 | |
Copper is another candidate element that could be involved in the redox cycling, Cu(I)/Cu(II), leading to dopaminergic cell death in Parkinson's disease. Micro-XANES study of Cu chemical state has been performed in neurons from the substancia nigra of Parkinson's disease cases and control cases.15 Micro-XANES spectra were fitted with the use of least-square method. The comparison of the positions of white line, multiple scattering and pre-edge peak maximum at the energy scale did not reveal the existence of differences in Cu chemical state. Cu(II) was found in all cases.
Speciation of carcinogenic metals
Several redox metals or metalloids are considered as carcinogens such as Cr and As. Their intracellular oxidation state is an important parameter to understand the mechanisms of carcinogenesis. The imaging of chromium oxidation states was compared in cells exposed to soluble and low solubility chromate compounds showing the intracellular reduction of Cr(VI) to Cr(III) in all cell compartments except in perinuclear structures from low solubility compounds (Fig. 4).16 The proposed mechanism for chromium differential carcinogenesis of chromium compounds according to their oxidation state and physical form (soluble and insoluble) is presented in Fig. 4. Cr(III) uptake is very limited explaining the low toxicity and carcinogenicity of Cr(III) compounds. Soluble Cr(VI) is readily transported into the cell through the sulfate transporters. Soluble Cr(VI) is immediately reduced in the cytoplasm into Cr(III) which can enter the nucleus and interact with genomic DNA. Insoluble Cr(VI) compounds are incorporated into the cell, through endocytosis like processes, then partially reduced to Cr(III) giving a similar effect to Cr(VI) soluble compounds. However, the persistence of Cr(VI) insoluble particles in the cytoplasm increases the oxidative stress and acts in synergy with Cr(III) binding to DNA.
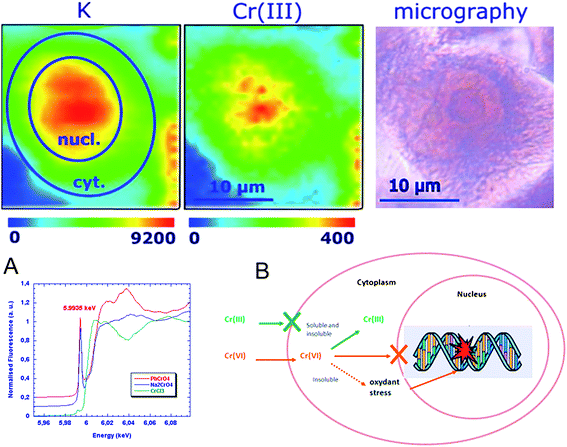 |
| Fig. 4
Chromium
oxidation state mapping. Potassium and chromium(III) distributions in single cell after exposure to soluble Cr(VI) compounds (top). X-ray absorption spectra of Cr(III) (green), soluble Cr(VI) (blue) and insoluble Cr(VI) red (A). Proposed mechanism for differential carcinogenesis of chromium compounds according to their oxidation state and physical form (B). Image reproduced by permission of the American Chemical Society (2005).16 | |
The predominance of Cr(III) in cells exposed to Cr(VI) soluble compounds was also verified in a micro-XANES study of human lung adenocarcinoma epithelial cells.17 In this study micro-EXAFS could also be performed in single cells. It is one of the very few examples of application of EXAFS analysis at the single cell level. Micro-EXAFS revealed that Cr hot spots in cells corresponded to polynuclear structures of Cr(III) formed during the intracellular reduction processes.
Arsenic is another important element involved in environmental toxicity and human carcinogenesis. The speciation of arsenic in human cells has been extensively studied.18 However the speciation analysis or arsenic in subcellular compartments is not possible with conventional techniques. Such speciation analysis is required to understand the cellular mechanisms of arsenic biotransformation. Using micro-XANES it has been shown that As(III) is the main form of arsenic in the cytosol, nucleus, and mitochondrial network of HepG2 cells exposed to As(OH)3.19 In some cases, oxidation to a pentavalent inorganic form is observed in nuclear structures of HepG2 cells (Fig. 5). This result is different from the EXAFS measurements performed on bulk samples which measured major contribution of As–S, probably as glutathione-arsenic compounds.20 The difference of chemical As states could come from the sample preparation and storage conditions as As–S binding was observed in freeze-dried cells but not in frozen hydrated cells.
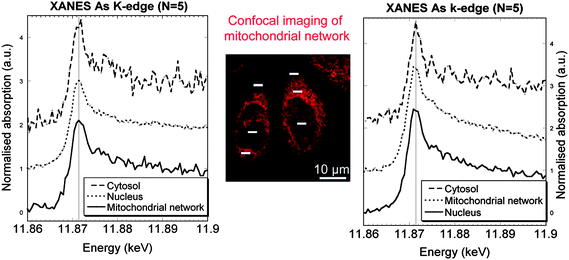 |
| Fig. 5 Micro-XANES at arsenic K-edge obtained in the cytosol, mitochondrial network, and nucleus of cells exposed to As2O3, and labelled with Rhodamine 123, a probe for mitochondrial network imaging (center image obtained by confocal microscopy). Left and right spectra correspond, respectively, to the zones analysed on the left and right cells (white bars). Image reproduced by permission of Elsevier (2010).19 | |
Speciation of anticancer drugs in tumor cells
In one of the very first experiments of micro-XANES at the single cell level, the chemical state of iron in cancer cells exposed to doxorubicin was investigated.7Doxorubicin is one of the most important anticancer agents in use. Doxorubicin is part of anthracycline antibiotics family which are known to be DNA intercalating agents and to concentrate in the cell nucleus. Doxorubicin can complex ironin vitro. Doxorubicin-iron complexes if formed in tumor cells could participate to the overall toxicity of the drug by redox cycling of Fe-doxorubicin complexes, and generation of highly reactive hydroxyl radicals. It was found that iron was present as Fe(III) in the nucleus of cultured cancer cells demonstrating the feasibility of micro-XANES in subcellular compartments. However, changes in Fe K-edge absorption spectra of cell exposed to doxorubicin compared to control cells were not observed. Micro-XANES did not allow identifying which compounds were binding to Fe. This is one limitation of the technique, XAS spectra are the mean of the chemical compounds present in the sample, therefore the most concentrated species will contribute to the essential absorption features and the more diluted compounds, such as doxorubicin-Fe complexes, may not appear in the XAS spectra.
Micro-XANES was also applied to the chemical speciation of platinum anticancer drugs.21Platinum-based anticancer agents are widely used in cancer chemotherapy, especially cisplatin, a Pt(II) oxidation state compound. Despite its high therapeutic efficacy, cisplatin presents high secondary toxicity to normal tissues. New platinum Pt(IV) compounds have been synthesized to overcome this secondary toxicity because Pt(IV) complexes are more inert than Pt(II) compounds. Micro-XANES revealed to be a very convenient analytical tool to follow the Pt oxidation states in cancer cells. The maxima of the first derivatives of the XANES spectra of the Pt(IV) oxidation states is 2.2 eV higher than that for Pt(II) at Pt L3-edge. It was shown that therapeutically relevant Pt(IV) compounds are readily reduced to Pt(II) in cells to form more reactive compounds.
Also related to the physiopathology of cancer, the accumulation of Zn in prostate cancer tissues and cultured cells was investigated using micro-XANES.22Zinc was present in tissues at Zn(II) oxidation state, and the position of Zn K-edge in XANES spectrum did not dependent on histological status of the tissue. In the case of cultured cells, differences in chemical neighbourhood zinc atoms were occasionally observed as demonstrated by the shift of absorption K-edge of zinc. In a recent study, micro-XANES was used to determine zinc, copper and iron oxidation state in invasive ductal carcinoma of breast tissue and normal surrounding tissue with a 5 × 5 μm beam size.23 Normal and cancer tissues did not differ in their Cu or Zn oxidation state but normal tissue regions were found to have a higher fraction of Fe(II) compared to the cancer tissues.
Speciation of essential trace elements
Micro-XANES can be used not only to study pathological processes but also for the examination of physiological mechanisms involving redox metals. For instance, micro-XANES revealed that the oxidation state of vanadium in vanadocytes from ascidians was +3 and +4.24 Also, in the field of trace element physiology of eukaryotic cells, micro-XANES has been performed on a copper-loaded NIH 3T3 cell revealing a near-edge feature that is characteristic for low-coordinate monovalent copper both in the cytoplasm and the nucleus.25 This result suggests that Cu binds mainly to biomolecules different from those generally expected to bind Cu in cells, namely glutathione and metallothionein. The nature of the Cu-binding molecules is still elusive but this example illustrates the great potential for in situ metal speciation to better understand the biochemistry of essential metals in cells. Another interesting example concerns zinc homeostasis in macrophage cells. In these cells Zn is stored in vesicles, so-called zincosomes, but the chemical speciation of zincosomal zinc has remained enigmatic. Micro-EXAFS showed that zinc is not free, but present in a complexed form involving S bounding.26
Micro-XANES is probably the unique analytical tool available to study the interaction of metals with bacteria at the single-cell level. Only EELS has been reported to be able to probeCr oxidation states in micro-organisms.6 This capability may be of particular use at the time to investigate the spatial heterogeneity of microbial communities. Micro-XANES was used to probe the chemical state of chromium in Pseudomonas fluorescens, showing spectral features consistent with association of Cr with a phosphoryl functional group.27 In Shewanella putrefaciens, intracellular granules containing iron have been observed. Micro-XANES revealed that both ferric and ferrous iron were present in those granules.28 The authors suggested that the relationship of the internal particles to Fe(III) reduction may indicate a respiratory role. The speciation analysis of metals in prokaryotes has important applications such as the investigation of microbiologically influenced corrosion processes. The spatial distribution of iron species throughout Pseudomonas aeruginosa biofilms was analysed to assess the influence of chemical heterogeneity on biomineralization.29Fe(III) was mainly associated with cell surfaces, while small amounts of Fe(II) was found in the extracellular space revealing that biofilm microbes may generate the observed multiple iron valence states through conditioning their localized extracellular microenvironments to a degree where nonenzymatic iron reduction is possible.
Conclusion
The chemical speciation of trace inorganic elements at the subcellular level is crucial to understanding their toxic properties or physiologic functions in cells. X-ray absorption spectroscopy performed with synchrotron radiation micro-beams is probably the unique method available to probe the chemical state of inorganic elements directly within subcellular compartments. Up to now, micro-XAS has been essentially applied to measure metal oxidation states in singles cells from biological tissues, and in subcellular organelles of cultured cells. It can be anticipated that micro-XAS applications will increase in the near future following the development of highly focused X-ray beamlines on several synchrotron radiation facilities worldwide. The expected gain in spatial resolution of these future beamlines will enable to probe smaller cellular organelles. However, there are still some improvements to make on the detection systems to increase the intensity of the X-ray fluorescence signal in order to facilitate micro-EXAFS speciation. Also special attention should be given to the sample preparation, storage and analysis methods. Cryogenic methods should be advocated at all stages of the analytical process in order to keep biological tissues in their (frozen) hydrated state avoiding modifications of chemical species. Also, the stability of the chemical species under beam irradiation should be tested systematically with standard reference material.
References
- J. J. Rehr and R. C. Albers, Rev. Mod. Phys., 2000, 72, 624–654.
- A. Snigirev and I. Snigireva, C. R. Phys., 2008, 9, 507–516 Search PubMed.
- K. K. Gunter, L. M. Miller, M. Aschner, R. Eliseev, D. Dupuis, C. E. Gavin and T. H. Gunter, NeuroToxicol., 2002, 23, 127–146 CrossRef CAS.
- J. E. Penner-Hahn, Coord. Chem. Rev., 2005, 249, 161–177 CrossRef CAS.
- T. Bacquart, G. Devès, A. Carmona, R. Tucoulou, S. Bohic and R. Ortega, Anal. Chem., 2007, 79, 7353–7359 CrossRef CAS.
- T. L. Daulton, B. J. Little, K. Lowe and J. Jones-Meehan, J. Microbiol. Methods, 2002, 50, 39–54 CrossRef CAS.
- R. Ortega, G. Devès, S. Bohic, A. Simionovici, B. Ménez and M. Bonnin-Mosbah, Nucl. Instrum. Methods Phys. Res., Sect. B, 2001, 181, 480–484 CrossRef CAS.
- S. Yoshida, A. Ektessabi and S. Fujisawa, J. Synchrotron Radiat., 2001, 8, 998–1000 CrossRef CAS.
- S. Rivera-Mancía, I. Pérez-Neri, C. Ríos, L. Tristán-López, L. Rivera-Espinosa and S. Montes, Chem.-Biol. Interact., 2010, 186, 184–199 CrossRef CAS.
- M. Valko, C. J. Rhodes, J. Moncol, M. Izakovic and M. Mazur, Chem.-Biol. Interact., 2006, 160, 1–40 CrossRef CAS.
- A. Ide-Ektessabi and M. Rabionnet, Anal. Sci., 2005, 21, 885–892 CrossRef CAS.
- J. Chwiej, D. Adamek, M. Szczerbowska-Boruchowska, A. Krygowska-Wajs, S. Wojcik, G. Falkenberg, A. Manka and M. Lankosz, JBIC, J. Biol. Inorg. Chem., 2007, 12, 204–211 CrossRef CAS.
- S. Bohic, K. Murphy, W. Paulus, P. Cloetens, M. Salome, J. Susini and K. Double, Anal. Chem., 2008, 80, 9557–9566 CrossRef CAS.
- J. F. Collingwood, A. Mikhaylova, M. Davidson, C. Batich, W. J. Streit, J. Terry and J. Dobsona, J. Alzheimer's Dis., 2005, 7, 267–272 CAS.
- J. Chwiej, D. Adamek, M. Szczerbowska-Boruchowska, A. Krygowska-Wajs, S. Bohic and M. Lankosz, J. Trace Elem. Med. Biol., 2008, 22, 183–188 CrossRef CAS.
- R. Ortega, B. Fayard, M. Salomé, G. Devès and J. Susini, Chem. Res. Toxicol., 2005, 18, 1512–1519 CrossRef CAS.
- H. H. Harris, A. Levina, C. T. Dillon, I. Mulyani, B. Lai, Z. Cai and P. A. Lay, JBIC, J. Biol. Inorg. Chem., 2005, 10, 105–118 CrossRef CAS.
- E. Dopp, U. von Recklinghausen, R. Diaz-Bone, A. V. Hirner and A. W. Rettenmeier, Environ. Res., 2010, 110, 435–442 CrossRef CAS.
- T. Bacquart, G. Devès and R. Ortega, Environ. Res., 2010, 110, 413–416 CrossRef CAS.
- K. L. Munro, A. Mariana, A. I. Klavins, A. J. Foster, B. Lai, S. Vogt, Z. Cai, H. H. Harris and C. T. Dillon, Chem. Res. Toxicol., 2008, 21, 1760–1769 CrossRef CAS.
- M. D. Hall, C. T. Dillon, M. Zhang, P. Beale, Z. Cai, B. Lai, A. P. Stampfl and T. W. Hambley, JBIC, J. Biol. Inorg. Chem., 2003, 8, 726–732 CrossRef CAS.
- M. Podgorczyk, W. M. Kwiatek, W. M. Zajac, J. Dulinska-Litewka, E. Welter and D. Grolimund, X-Ray Spectrom., 2009, 38, 557–562 CrossRef CAS.
- A. Al-Ebraheem, J. Goettlicher, K. Geraki, S. Ralph and M. J. Farquharson, X-Ray Spectrom., 2010, 39, 332–337 CrossRef CAS.
- K. Takemoto, T. Ueki, B. Fayard, A. Yanamoto, M. Salomé, S. Scippa, J. Susini, T. Uyama, H. Michibata and H. Kihara, J. Phys. IV, 2003, 104, 333–336 Search PubMed.
- L. Yang, R. McRae, M. M. Henary, R. Patel, B. Lai, S. Vogt and C. J. Fahrni, Proc. Natl. Acad. Sci. U. S. A., 2005, 102, 11179 CrossRef CAS , 11184.
- G. Wellenreuther, M. Cianci, R. Tucoulou, W. Meyer-Klaucke and H. Haase, Biochem. Biophys. Res. Commun., 2009, 380, 198–203 CrossRef CAS.
- K. M. Kemner, S. D. Kelly, B. Lai, J. Maser, E. J. O'Loughlin, D. Sholto-Douglas, Z. Cai, M. A. Schneegurt, C. F. Kulpa Jr. and K. H. Nealson, Science, 2004, 306, 686–687 CrossRef CAS.
- S. Glasauer, S. Langley, M. Boyanov, B. Lai, K. Kemner and T. J. Beveridge, Appl. Environ. Microbiol., 2007, 73, 993–996 CrossRef CAS.
- R. C. Hunter, A. P. Hitchcock, J. J. Dynes, M. Obst and T. J. Beveridge, Environ. Sci. Technol., 2008, 42, 8766–8772 CrossRef CAS.
Footnote |
† This article is part of a themed issue highlighting outstanding and emerging work in the area of speciation. |
|
This journal is © The Royal Society of Chemistry 2011 |