Emerging investigator series: in-depth chemical profiling of tire and artificial turf crumb rubber: aging, transformation products, and transport pathways†
Received
31st May 2024
, Accepted 15th August 2024
First published on 16th August 2024
Abstract
Crumb rubber generated from end-of-life tires (ELTs) poses a threat to environmental and human health based on its widespread use. Of particular concern is the use of ELT crumb rubber as infill for artificial turf fields, as people are unknowingly exposed to complex mixtures of chemicals when playing on these fields. Additionally, there is concern regarding transport of rubber-related chemicals from artificial turf into the environment. However, existing knowledge does not fully elucidate the chemical profile, transformation products, and transport pathways of artificial turf crumb rubber across different ages. To address these knowledge gaps, we utilized a multi-faceted approach that consisted of targeted quantitation, chemical profiling, and suspect screening via ultra-high performance liquid chromatography-high-resolution mass spectrometry (UHPLC-HRMS). We collected and processed 3 tire and 11 artificial turf crumb rubber samples via solvent extraction, leaching, and a bioaccessibility-based extraction. Nineteen rubber-derived chemicals were quantified using parallel reaction monitoring and isotope dilution techniques. In solvent extracts, the most abundant analytes were 1,3-diphenylguanidine (0.18–1200 μg g−1), N-(1,3-dimethylbutyl)-N′-phenyl-p-phenylenediamine (6PPD, 0.16–720 μg g−1), 2-mercaptobenzothiazole (0.47–140 μg g−1), and benzothiazole (0.84–150 μg g−1). Chemical profiling assessed changes in sample diversity, abundance, polarity, and molecular mass. Suspect screening identified 81 compounds with different confidence levels (16 at level 1, 53 with level 2, 7 at level 3, and 5 at level 4). The formation rate of transformation products and clustering analysis results identified time-based trends in artificial turf field samples. We found that the first two years of aging may be critical for the potential environmental impact of artificial turf fields. Our analysis provides insight into the chemical complexity of artificial turf crumb rubber samples ranging from 0–14 years in age.
Environmental significance
Recycling end-of-life tires (ELTs) into crumb rubber is a global problem. The use of crumb rubber in artificial turf is concerning, as the chemical profile and transport pathways are not understood. Additionally, aging can result in the formation of transformation products, some of which can be more toxic than their precursor compounds. In this study we investigated the chemical profile and transport pathways of artificial turf and tire crumb rubber of varied ages using high-resolution mass spectrometry. The results showed that the aging of artificial turf crumb rubber affects the transformation and transport of rubber-derived chemicals. Our findings suggest that the first two years of aging is a critical time window for the environmental impact of artificial turf crumb rubber.
|
1 Introduction
Globally, around one billion tires reach the end of their useful lives every year.1 End-of-life tires (ELTs) are often recycled into crumb rubber that is subsequently repurposed into building materials, shoe soles, gym mats, or thrown away in landfills.2,3 One use of ELT crumb rubber is as infill for artificial turf fields. There are >26
000 artificial turf fields filled with crumb rubber in the US and EU combined.4,5 Despite their vast numbers, the environmental impacts and ecological exposures of ELTs are poorly understood. Specifically, their major transport pathways to surrounding environments and chemical similarity with tires is rarely investigated. Recent studies demonstrated that tire rubber-derived chemicals, such as the acute salmon toxicant 6PPD-quinone, are both ecotoxic and ubiquitous in the environment.6 However, the occurrence, transformation, and release of these chemicals from repurposed ELTs has not been well examined.7,8
Recent works on urban stormwater and tire wear particles (TWPs) revealed a diverse group of rubber-derived chemicals, including vulcanizing/crosslinking reagents, antioxidants, plasticizers, processing aids, and their respective transformation products (TPs).9–11 In addition to leaching with water, many rubber-derived chemicals may be lipophilic and more accessible via solvent extraction. These reflect a larger, more diverse suite of chemicals, whose transformation and transport behavior could differ from the leachable compounds from water-related studies.12,13 Another underexplored chemical area is the bioaccessible fraction from TWPs and ELTs.14 This is especially relevant for ELTs because they are direct sources of human exposure.15 TWPs mainly originate from tire tread, while ELTs include all tire components. Studies comparing TWPs and ELTs reveal significant differences in size and chemical composition16–19 especially in metal content, impacting their leaching and bioavailability as they age.12 Therefore, it is important to understand the chemical profiles of leachable, extractable, and bioaccessible fractions of TWP and ELT crumb rubber.
Environmental aging and chemical transformation of rubber has been another research focus because many additives (e.g., antioxidants, UV absorbers) are designed to function by going through transformations. These reactions can form TPs which can lead to unexpected environmental and toxicological consequences, such as 6PPD-quinone.6,20,21 Increased toxicity of TPs has been recorded for other contaminants such as triclosan22 and organophosphites.23 A few very recent studies investigated the occurrence of TPs and the aging process. Fohet et al.24 investigated the accelerated thermal, photochemical, and natural outdoor aging of tire rubber using cryomilled tire tread particles. They presented time-concentration profiles of 23 rubber-derived chemicals, and calculated time constants. Concentration profiles showed different aging behaviors among chemicals, though most analyzed compounds have a half-life <50 days. Wayrauch et al.25 explored the accelerated aging of TWPs by elevated temperature, artificial sunlight, and mechanical stress. This study suggested that sunlight exposure was the most influential factor in changing the chemical composition of tire extracts/leachates, and various TPs were observed in the aging process. Zhao et al.26 examined the occurrence and oxidation of p-phenylenediamines (PPDs) and their quinones in artificial turf fields, and found most PPDs decreased >50% within 4 days. These studies revealed the importance of environmental aging of tire rubber, and implied that more investigations on aging/aged rubber samples are needed.
To understand the rubber-derived chemicals from different sample preparation methods and ages, we performed targeted quantification, chemical profiling, and suspect screening on 3 tire and 11 artificial turf crumb rubber samples. Our study used three sample preparation methods: water leaching, organic solvent extraction, and a bioaccessibility-based extraction mimicking gastric and gastrointestinal fluids. This comprehensive approach will provide insight into the overall composition of the samples, as well as which compounds are leachable/bioaccessible. Through a multi-faceted approach, we aim to better understand the potential health and environmental concerns associated with artificial turf fields.
2 Materials and methods
2.1 Chemicals
Chemical standards of rubber-derived chemicals and isotopically labeled internal standards were purchased commercially (see Table S1†). OPTIMA grade water, methanol, acetone, formic acid, and ammonium fluoride were purchased from Fisher Scientific (Pittsburgh, PA, USA).
2.2 Collection of crumb rubber samples
Artificial turf crumb rubbers (n = 11) were collected in dry weather (>48 h prior dry weather period) from public park (n = 3) or school (n = 8) artificial turf fields in Massachusetts and New Hampshire (USA, see Table S2†). When known, the approximate date of the field installation was recorded, however, for two of the samples (Unk1, 2), the ages are unknown. Samples for each field were collected from 3 locations, corresponding to the two goals and center line, in addition to field blanks. To reduce the possibility of only sampling the top layer of artificial turf, which may be replaced over time by new crumb rubber, samples were collected by scooping rubber from all layers until the artificial turf backing was reached. Samples were mixed prior to weighing and stored at 4 °C in the dark until extraction. TWP samples (n = 3) were collected with different generation methods: physical abrasion (n = 1, TU&N; Tire Used & New) and cryo-milled (n = 2, TU; Tire Used, TN; Tire New, see Table S3†).17,27
2.3 Organic solvent extraction of crumb rubber samples
Identifiable debris from the artificial turf samples (e.g., rocks, sand, artificial grass) were manually removed during the weighing process. 50 mg of crumb rubber were weighed into vials in triplicate and spiked with 100 μL of a 1000 ng mL−1 isotopically labeled internal standard (ISTD) mixture (see Table S1†). After spiking, the vials were loosely covered with clean foil and allowed to evaporate overnight (ambient air and room temperature). The samples were extracted in 10 mL of 1
:
1 (v/v) methanol
:
acetone. The vials were vortexed for 1 minute, then shaken for 24 h at room temperature. After extraction, samples were centrifuged for supernatant collection (2500 rpm, 20 min), which was subsequently concentrated to ∼0.75 mL under nitrogen, and gravimetrically brought to 1 mL. Samples were stored at −20 °C overnight, then syringe filtered through 0.2 μm PTFE filters into 2 mL LC vials. This resulted in a final ISTD concentration of 100 ng mL−1. Samples were stored at −20 °C until analysis. Method blanks (n = 3, empty 15 mL glass vials without crumb rubber) were included for each extraction batch.
2.4 Leachables sample preparation
Leachables sample preparation was adapted from a reported protocol.25 Triplicates of 12 mg of crumb rubber were weighed into vials and spiked with 120 μL of a 10
000 ng mL−1 ISTD mixture (see Table S1†). 12 mL of water was added to each vial. The vials were then vortexed for 1 minute, and shaken for 72 h at room temperature. Samples were centrifuged for supernatant collection (2500 rpm, 20 min). Samples were stored at 4 °C overnight, then syringe filtered through 0.2 μm PTFE filters into 2 mL LC vials. This resulted in a final ISTD concentration of 100 ng mL−1. Samples were stored at 4 °C until analysis. Method blanks (n = 3, empty 15 mL glass vials without crumb rubber) were included.
2.5 Bioaccessibility-based extraction of crumb rubber samples
Bioaccessibility-based extraction of crumb rubber samples was performed in accordance with the unified bioaccessibility method, utilizing simulated fluids in order to represent the physiochemical properties of the human gastrointestinal tract.14,28–32 200 mg of crumb rubber was weighed into vials in triplicate and spiked with 100 μL of a 1000 ng mL−1 ISTD mixture (see Table S1†). The digestion was performed in two steps: gastric and gastrointestinal. The simulated biological fluids were prepared the day before extraction, and incubated overnight at 37 °C, with rotary shaking. Both stages underwent gastric digestion, through the addition of 3 mL of saliva and 4.6 mL of gastric juice. The samples were then pH adjusted, if necessary, to pH 1.2 ± 0.1 and subjected to incubation and rotary shaking for 1 h at 37 °C. Afterwards, the supernatant was collected from the gastric samples. The gastrointestinal samples underwent further extraction, through the addition of 9.2 mL of duodenal fluid and 3 mL of bile. The samples were pH adjusted to pH 6.3 ± 0.5 and subjected to incubation and rotary shaking for 4 h at 37 °C. The supernatant underwent cleanup via solid-phase extraction (SPE, Oasis HLB 150 mg sorbent, 60 μm particle size, Waters Corporation). After SPE, the samples were eluted with 10 mL of methanol, concentrated to ∼0.75 mL under nitrogen, and gravimetrically brought to 1 mL. Samples were stored at −20 °C overnight, then filtered through 0.2 μm PTFE filters into 2 mL LC vials. This resulted in a final ISTD concentration of 100 ng mL−1. Samples were stored at −20 °C until analysis. Method blanks (n = 3) were included.
2.6 UHPLC-HRMS analysis
Crumb rubber extracts were analyzed using a Thermo Fisher Scientific Vanquish Flex ultra-high performance liquid chromatograph (UHPLC) interfaced to a Thermo Fisher Scientific Exploris 240 quadrupole-orbitrap mass spectrometer equipped with a heated electrospray source. Chromatographic separation was performed using a C18 column (Agilent ZORBAX RRHD Eclipse Plus C18, 2.1 × 100 mm, 1.8 μm). Instrument method details are present in the ESI (see Table S4†). Chemical profiling and suspect screening were performed in both positive and negative ion mode. For targeted quantitation, a parallel reaction monitoring method was utilized (see Tables S5 and S6†). For quality assurance and quality control, the instrument was externally calibrated before each analytical run. Mass accuracy was corrected throughout the analysis using an internal calibrant (EASY-IC). Solvent blanks and internal standard controls were analyzed every 15 samples. Triplicate method blanks and field blanks were analyzed alongside the samples. The retention time (RT) variations of the internal standard mixture components were ±0.1 min, and precursor mass errors were ±3 ppm across all samples.
2.7 Quantitative data analysis
Targeted quantitation was performed in Skyline (ver. 23.1.0.380).33,34 10-point calibration curves were prepared using a standard mixture ranging from 0.1–500 ng mL−1 (0.1, 0.5, 1, 5, 10, 25, 50, 100, 250, 500 ng mL−1). Calibration curve levels were selected based on previously reported concentrations for a subset of compounds in artificial turf crumb rubber, with the upper concentration limit increased to 500 ng mL−1.35 Internal standards were spiked at each concentration level at 100 ng mL−1. Calibration curves were prepared by plotting the peak area ratios of the analyte to its corresponding internal standard against analyte concentration. Intra-day precision (repeatability) was determined by assessing the relative standard deviation of standard samples injected 3 times each (n = 3) at two concentration levels (1 ng mL−1 and 10 ng mL−1 for more sensitive analytes, 10 ng mL−1 and 100 ng mL−1 for others). Inter-day precision (reproducibility) was determined using the same approach, but by injecting the solutions on three non-consecutive days.
Compound identification was performed based on retention time and MS2 spectra matching (at least 2 fragments, RT window ± 0.1 min) using an in-house library (Thermo mzVault ver. 2.3 SP1, Thermo Fisher scientific) then imported into Skyline. Skyline processing settings are shown in the ESI (see Table S7†). Instrument detection and quantitation limits (IDL/IQL) were calculated in accordance with EPA-540-G-90-008 (ref. 36) and method detection limits (MDL) were calculated in accordance with EPA-821-R-16-006 (ref. 37) using the following equations:
|
IDL = t(n−1,1−∝=0.99)S
| (1) |
|
MDLS = t(n−1,1−∝=0.99)SS
| (3) |
where IDL is the instrument detection limit, IQL is the instrument quantitation limit,
S is the standard deviation of replicate analyses at the lowest concentration that is statistically different from a blank. MDL
s is the method detection limit based on spiked samples,
t(n−1, 1−α=0.99) is the Student's
t-value for a single-tailed 99th percentile
t statistic and a standard deviation with
n − 1 degrees of freedom, and
Ss is the sample standard deviation of the replicate spiked sample analysis. Sample concentrations were adjusted to account for background by subtracting the method blank concentration. Intra- and inter-day precision, linear range,
R2 values, IDL, IQL, and MDL values were reported (Tables S8 and S9
†). Method accuracy was assessed using absolute and relative recoveries in spiked samples, prepared by spiking native standards (100 ng mL
−1) into 13 years rubber samples. Recoveries were calculated using a previously described method (Table S10
†).
38
2.8 Suspect screening
Suspect screening data analysis was performed in Compound Discoverer (Thermo Fisher Scientific, ver. 3.3 SP1). Detailed workflow parameters can be found in Table S11†.
2.9 Statistical analysis
The statistical analysis of HRMS data was conducted using Python (3.10.12) with the scikit-learn package (1.2.2).39,40 For principal component analysis, z-score normalization is performed by default to eliminate data skewness and kurtosis:where z is z-score, x is feature peak area in the sample, μ is the average peak area of the feature across all samples, and σ is the standard deviation of the peak areas. The first 2 principal components were plotted for visualization purposes (Fig. 4). For hierarchical clustering analysis (Fig. 5), peak area data was transformed with z-score normalization. Ward linkage algorithm and Euclidean distance metric were used for the analysis (OriginPro, ver. 2021b, OriginLab corporation). Statistical analysis for monotonic trends in the targeted quantitation results were performed using the Mann–Kendall test (α = 0.05)41 in Python with the pyMannKendall package (1.4.3).42
3 Results and discussion
3.1 Targeted quantitation
Targeted quantitation was performed for 19 rubber-derived chemicals from 6 compound classes: plasticizers, benzotriazoles and benzothiazoles (BTHs & BTRs), vulcanizing agents (VAs), p-phenylenediamine antioxidants (PPDs), PPD transformation product 6PPD-quinone (6PPD-Q), and other rubber antioxidants (AOs) (Table S1 and Fig. S1†). Compound concentrations in each tire and turf sample were determined and trends across the different sample preparation methods (leachables, extractables, and bioaccessibility-based extraction) are discussed.
3.1.1 Comparison of sample preparation methods. Total compound class concentrations were calculated for each sample preparation method. Leachables were found to have the lowest concentration (Σcompounds: <IDL-540 μg g−1, Fig. 1a), followed by extractables (Σcompounds: 14–2300 μg g−1, Fig. 1b), then gastrointestinal samples (Σcompounds: 0.25–2900 μg g−1, Fig. 1d), with the most abundant concentration found in the gastric samples (Σcompounds: 0.26–9900 μg g−1, Fig. 1c). An increase in total concentration from leachables to extractables is expected, as organic solvents more efficiently extract non-polar compounds from the crumb rubber. Unexpectedly, the two bioaccessible fractions have better extraction efficiency than solvent extraction, particularly for PPDs, 6PPD-Q, BTHs and BTRs, and plasticizers.
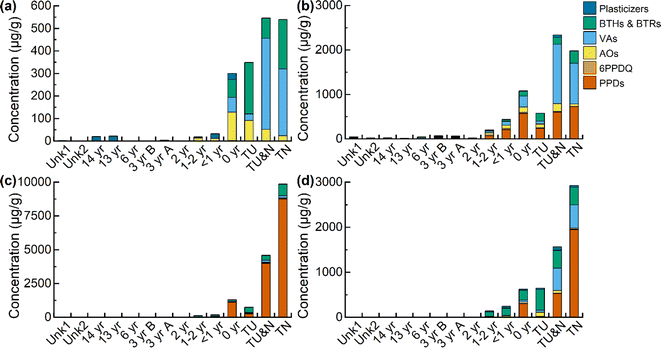 |
| Fig. 1 Concentrations of target rubber-derived chemicals in tire (TU, TU&N, and TN) and turf (0–14 years) samples for (a) leachables (b) extractables (c) gastric fluids and (d) gastrointestinal fluids. | |
Differences in compound classes were observed across the different sample preparation methods. All compound classes were found in the leachable samples, except for PPDs (IPPD and 6PPD) which were below the IQL in all samples. All compound classes were present in the other extraction methods (extractables, gastric, and gastrointestinal), in at least one sample. The top compounds in the leachables were 1,3-diphenylguanidine (DPG), benzothiazole (BTH), dicyclohexylamine, and 2-mercaptobenzothiazole (MBT). These are similar to the top compounds in the extractables, gastric, and gastrointestinal samples, which were 6PPD, DPG, BTH, and MBT.
3.1.2 Quantitation of extractables. Solvent extraction was expected to extract polar and nonpolar organic compounds more exhaustively from crumb rubber compared to the other sample preparation methods. The method succeeded in extracting all target compounds at quantitative levels in at least one rubber sample, except for 1H-benzotriazole (18/19). The most abundant compound classes found in the extractable samples were PPD, AOs, VAs, and BTHs and BTRs (Fig. 1b). The top three compound classes were VAs (ΣVAs, 0.99–1300 μg g−1), PPDs (ΣPPDs, 0.16–720 μg g−1), and BTH&BTRs (ΣBTH&BTRs, 3.8–270 μg g−1). The other compound classes had maximum concentrations ranging from 27–180 μg g−1 (ΣAOs: 1.4–180 μg g−1, 6PPD-Q: 0.45–27 μg g−1, Σplasticizers: 1.8–49 μg g−1). The compounds with the highest individual concentrations were DPG (0.18–1200 μg g−1), 6PPD (0.16–720 μg g−1), MBT (0.47–140 μg g−1), and BTH (0.84–150 μg g−1).
3.1.3 Quantitation of leachables. Only a subset of the target compounds (10/19) was quantitated in the leachable samples: DPG, MBT, BTH, caprolactam, dicyclohexylamine, N-phenyl-1-naphthylamine, 1,3-diphenylurea, hexa(methoxymethyl)melamine (HMMM), 6PPD-Q, and triisopropanolamine (TIPA) (see Table S12†). PPDs were below the limits of detection. 6PPD has been detected in tire crumb rubber leachate previously, however detection is often difficult due to the low desorption tendency of 6PPD.24,25 While 6PPD was not detected, 6PPD-Q was found in the three newest turf samples less than 2 years of age (0 year, <1 year, 1–2 years) in concentrations ranging from 0.61–1.8 μg g−1. The top compounds in the leachables were DPG (<IDL-370 μg g−1), MBT (<IDL-73 μg g−1), BTH (<IDL-220 μg g−1), and dicyclohexylamine (<IDL-91 μg g−1). Polarity may explain the improved leaching of the top compounds, as evident with their lower logP values (BTH: 1.899, DPG: 2.384, MBT: 2.59, see Table S1†).Among these compounds, 6PPD-Q, DPG, MBT, BTH, HMMM, and dicyclohexylamine are of particular concern due to potential health risks.43–47 Additionally, there is concern for all of the detected compounds to be transported into the surrounding environment.48 This point is emphasized by the leaching profile of the new turf collected on the day of installation (0 year). The total concentration of leached compounds in the 0 year turf was 300 μg g−1, with the tire samples ranging from 350–550 μg g−1 (see Table S12†). The fields <1–14 years in age had total leachable compound concentrations ranging from 2.4–32 μg g−1. This suggests a major loss in overall target compounds occurring on artificial turf fields within the first year after installation, implying the leaching or degradation of rubber-derived chemicals from new fields to the environment. Additional studies are needed to assess the mechanisms of such loss.
3.1.4 Quantitation of bioaccessibles. The bioaccessibility of rubber-derived chemicals in digestive fluids was also assessed. Previous research has shown the presence of benzothiazoles, HMMM, DPG, 6PPD, and 6PPD-Q in human and animal simulated digestive fluids.14,28,49–51 Due to a combination of matrix suppression and analyte loss during the sample preparation process of the bioaccessible samples, low compound recoveries were observed (see Table S10†). Compounds with a relative recovery outside of 50–150% are treated as semi-quantitative, and are indicated as such (see Table S12†). Correlation between the age of artificial turf fields with the bioaccessibility of rubber-derived chemicals was assessed for both bioaccessible fractions: gastric (Fig. 1c) and gastrointestinal (Fig. 1d). The most abundant compound class in the gastric samples was PPDs (ΣPPDs: <IDL-8700 μg g−1), followed by BTHs and BTRs (ΣBTHs&BTRs: 0.076–850 μg g−1). A similar trend is observed in the gastrointestinal samples, as PPDs (ΣPPDs: <IDL-1900 μg g−1) and BTHs and BTRs (ΣBTHs&BTRs: 0.059–460 μg g−1) are also the most abundant compound classes. The top compounds in the bioaccessible samples were 6PPD (gastric: <IDL-8700 μg g−1; gastrointestinal: <IDL-1900 μg g−1), DPG (gastric: <IDL-170 μg g−1; gastrointestinal: <IDL-500 μg g−1), BTH (gastric: <IDL-610 μg g−1; gastrointestinal: <IDL-400 μg g−1) and MBT (gastric: <IDL-210 μg g−1; gastrointestinal: <IDL-46 μg g−1, see Table S12†). The order of the top compounds varies between the gastric and gastrointestinal fractions with 6PPD, BTH, MBT, and DPG in gastric, and 6PPD, DPG, BTH, and MBT in gastrointestinal (highest to lowest).Unexpectedly, the bioaccessible fractions were found to have a high concentration of 6PPD, the most nonpolar compound targeted (log
P: 5.127, see Table S1†). While the bioaccessible fractions are water-based, the acidic pH of these two solutions (gastric: pH 1.2, gastrointestinal: pH 6.3) could improve the extraction of some compounds. Estimated values for the pKa of 6PPD and 6PPD-Q have been reported (6PPD: 6.46, 6PPD-Q: −4.02).52 These values suggest that at the pH level of the two bioaccessible fractions, 6PPD is predominantly present in its cationic form and is therefore more soluble. By contrast, 6PPD-Q is mostly in its neutral form at these pH levels and thus is less soluble. This observation could explain the high abundance of 6PPD in the bioaccessible samples, and affirms that solution conditions such as pH play a critical role in the mobility and bioaccessibility of 6PPD and 6PPD-Q.
3.1.5 Comparison of artificial turf crumb rubber by age. Across the sample preparation methods, we observe a decrease in total compound concentration with increasing sample age (Fig. 1). Similarity between the new artificial turf crumb rubber (0 year) and the tire samples was assessed by comparing total concentration for each sample preparation method. In the leachables, the 0 year turf (300 μg g−1) is close to the level in the tire samples (350–550 μg g−1). In the other extraction methods (extractable, gastric, gastrointestinal), the concentration of the 0 year turf crumb rubber falls between that of the used tire (TU) and the mixture of new and used tires (TU&N) (see Table S13†). When comparing among turf samples, the newest turf sample (0 year) has total compound concentrations that are 2.5–17× higher than the next two newest samples (<1 year and 1–2 years) across all sample preparation methods. This suggests that the total concentration of targeted compounds in the new turf sample is similar to that of tire samples, but decreases within the first two years of environmental aging. This observation is further supported by time-trend analysis of the tire and artificial turf samples based on age. Time-trend analysis was performed for each compound class, as well as the total concentration of all target analytes (Table S14†). For all sample preparation methods, a decreasing trend in total compound concentration was found to be statistically significant (p-value: <0.05; leachable: 0.0091 gastric: 0.00044, gastrointestinal: 0.00028, extractable: 0.00078). Additionally, statistically significant trends in compound class concentrations were found for PPDs AOs, VAs, BTHs & BTRs, and plasticizers in the gastric, gastrointestinal and extractable samples. A decreasing monotonic trend was also observed for 6PPD-Q in gastric and extractable samples, as well as for plasticizers in the extractable samples.When assessing individual compound trends, a non-monotonic trend was observed for only a subset of analytes; 6PPD-Q, 2-amino-benzothiazole (2-amino-BTH), and 4-phenylazodiphenylamine, the highest concentration of these compounds can be found in the turf samples that are less than 2 years old (see Fig. S2†). For these three compounds, there is an increase in concentration with increased tire age, with an apex reached in either the newest turf sample (0 year) or the next newest turf sample (<1 year), followed by a decrease in concentration with increased turf age (2–14 years) in the extractables. The trend in 6PPD-Q concentration suggests that it may peak in turf fields one to two years post-installation. However, quantitation of all 6PPD TPs was not performed, therefore we cannot assess time-based trends in total TP concentration. For 2-amino-BTH and 4-(phenylazo)diphenylamine, more investigation is needed to explain this trend, as these are not known TPs. When comparing total sample concentration based on turf age in the extractables (Fig. 1b), the age unknown samples Unk1 (Σcompounds, 45 μg g−1) and Unk2 (Σcompounds, 21 μg g−1) have total concentrations that fall between samples 2–14 years old (Σcompounds, 14–69 μg g−1). Based on the previous estimations, the two unknown fields are most likely 2–14 years in age, with field Unk1 possibly being newer than Unk2 due to a higher concentration of extractable compounds.
3.1.6 Relative concentration of parent and TPs by age. The relative concentration of the transformation product 6PPD-Q to its parent compound 6PPD was assessed by normalizing to total concentration in each sample (Table S15†). The resulting % concentration of 6PPD and 6PPD-Q can be seen in Fig. 2a. The tire and newest turf samples (0 year) had a % 6PPD-Q concentration ranging from 0.7–6.3%. The % 6PPD-Q concentration begins to increase in the turf samples from less than two years in age (11–24%), with the turf samples aged three years and older ranging from 58–92%. This agrees with prior observations that indicate the first 2 years of aging may be critical for artificial turf crumb rubber.
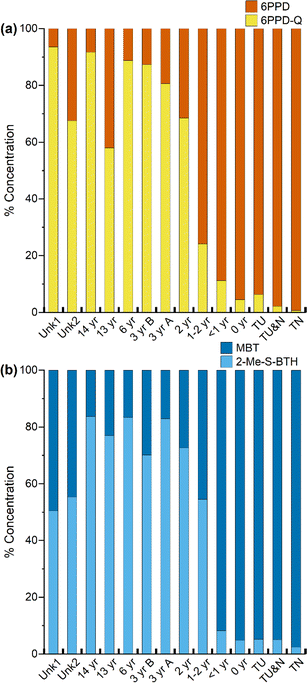 |
| Fig. 2 Relative concentrations of compounds normalized to total concentration in rubber extractables for (a) 6PPD and 6PPD-Q and (b) MBT and 2-Me-S-BTH. | |
Another compound class of concern is BTH and its derivatives, which have been found in tire wear, road dust, roadside soil, roadway runoff, rubber-based consumer products and in artificial turf fields.53 Of interest is MBT, which is observed to degrade when subjected to thermal aging, potentially forming 2-(methylthio)benzothiazole (2-Me-S-BTH).24,25,35,54 The relative concentration of the parent compound MBT to its proposed transformation product 2-Me-S-BTH was assessed (Fig. 2b). Similar with 6PPD/6PPD-Q, there is initially a low relative concentration of MBT in the tire and new turf sample (2.4–5.1%), followed by an increase with the samples less than two years of age (8.2–54%), and then a higher relative concentration of the TP in the sample aged more than three years (70–84%). Plots were generated for all other compound classes selected for quantitation (Fig. S3†). However, because all other compound classes only contain parent compounds, no trends were observed.
3.2 Chemical profiling and suspect screening
3.2.1 Chemical profiling. Trends of rubber-derived chemicals in samples can be further investigated via analysis of all HRMS features (unique combinations of RT and m/z) without structure identification. By investigating all features, the diversity, abundance, polarity, and molecular mass of the compounds in all samples across the sample preparation methods can be compared. To accomplish this total peak area, median retention time, median m/z, and total number of features were determined (Table S16†).The relationship between total number of features and total peak area for each sample preparation method across samples of different ages was assessed (Fig. S4 and S5†). In comparing positive and negative ion modes, negative ion mode exhibited fewer features and a lower total peak area. Time-based trends in total peak area and number of features observed in the extractable and gastric preparation methods demonstrate a decrease in sample diversity and abundance with increasing age. Consistent with the quantitative results, the leachable samples had the lowest number of features and total peak area of all the sample preparation methods. While it is possible to detect thousands of features in the gastrointestinal samples, there is also no time-based trend. However, this information only provides insight into the bulk chemical properties and does not assess if the features are changing with time (i.e., due to the formation of TPs). More controlled aging experiments are needed to elucidate this.
Feature polarity and size were investigated by determining the median retention time and median m/z, respectively (Fig. 3 and S6†). As reverse-phase chromatography was performed we anticipate that a shorter median RT will indicate more polar features. Median m/z of singly charged ions was utilized as a proxy for feature size, with smaller m/z indicating features with a smaller monoisotopic mass. The tire samples appear to consist of smaller, more polar features than the turf samples in the leachables (Fig. 3a). This trend is also observed for the extractable samples, but is not as prominent (Fig. 3b). An inverse trend in polarity is observed in the bioaccessible sample preparation methods, as the tire samples appear to be more nonpolar compared to the turf samples (Fig. 3c and d). The trend in median m/z shows an increase in size for the turf compared to the tire samples across all sample preparation methods, possibly indicating the formation of TPs.
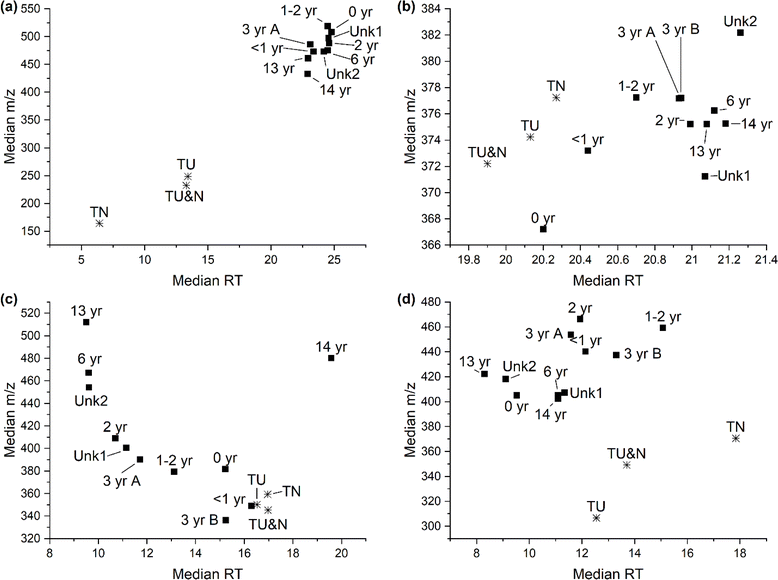 |
| Fig. 3 Relationship between median retention time (RT) and median m/z with crumb rubber age in positive ion mode for (a) leachables, (b) extractables, (c) gastric and (d) gastrointestinal sample preparation methods. Asterisks represent tire rubber samples, and squares represent crumb rubber samples. | |
Principal component analysis (PCA) of all features was performed (Fig. 4, S7 and S8†). The resulting PCA showed that samples clustered into three broad groups, tire samples (TN, TU&N, TU), new turf samples (<2 years), and older turf samples (2–14 years). Principal component 1 (PC1) appears to distinguish samples based on age. Across PC1, the newer turf samples cluster between the tire and older turf, indicating that the new turf samples are similar to tire, but still have properties that make them unique. This apparent trend is consistent with the quantitative results in which we observe an overall decrease in additive concentrations as the turf samples age. The PC1 clustering also provides further support that the turf samples of unknown installation date, Unk1 and Unk2, are more similar to the samples aged more than two years.
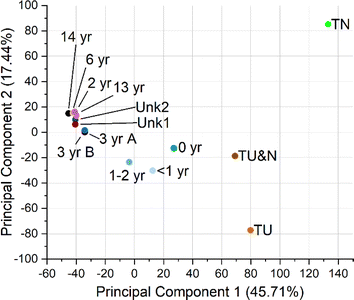 |
| Fig. 4 Principal component analysis based on all features detected in the extractables of tire and turf samples. | |
3.2.2 Suspect screening of samples. Suspect screening was performed for all samples. Compounds were identified as level 1–4 based on guidelines established by Schymanski et al. (Fig. S9–12 and Table S17†).55 81 different chemicals from 13 broad chemical classes were identified in the rubber extractables in positive ion mode (Table S17†). Of these, 16 compounds were identified at level 1, 53 with level 2, 7 at level 3, and 5 at level 4. These identifications encompass 11 BTHs, 5 ethanolamides, 5 plasticizers, 6 PPDs, 20 PPD TPs, 2 rosin acids, 8 rubber antioxidants (other than PPDs), 1 surfactant, 9 vulcanization compounds, 5 benzanilide-related compounds, 1 UV absorber, 1 secondary amino compound, and 7 cyclic amines (Table S18†). A subset of PPD TPs (8/20) were previously detected as products of 6PPD ozonation, but still lack full structure elucidation.52,56 Suspect screening of the rubber leachables in positive ion mode resulted in 9 compounds (BTHs, cyclic amines, PPD TPs, and rubber antioxidants), all of which were also identified in the rubber extractables (Table S19†). No additional compounds were identified in negative ion mode for both sample preparation methods. Suspect screening results of the rubber gastric and gastrointestinal samples, in both polarities, did not result in the identification of any additional compounds that were not already selected for targeted quantitation. As a result, these samples are not discussed further.The results of the suspect screening were visualized using hierarchical cluster analysis (HCA) of the peak areas (Fig. 5 and S13†). For the extractable samples, samples branch into two main groups: tire samples and the newest turf samples (TN, TU&N, TU, <1 year turf), and turf samples from 1–14 years in age and the unknown turf samples. This clustering, based on the 81 identified compounds, is consistent with the quantitative analysis and chemical profiling. This is also supported by the results of the leachables, which split into two main groups: newer tire and turf (TN, TU&N, 0 year) and aged tire and turf (TU, <1–14 years turf, unknown turf samples) (Fig. S13†). These results support the previous observation that the first two years of aging play a critical role in the chemical composition of artificial turf crumb rubber.
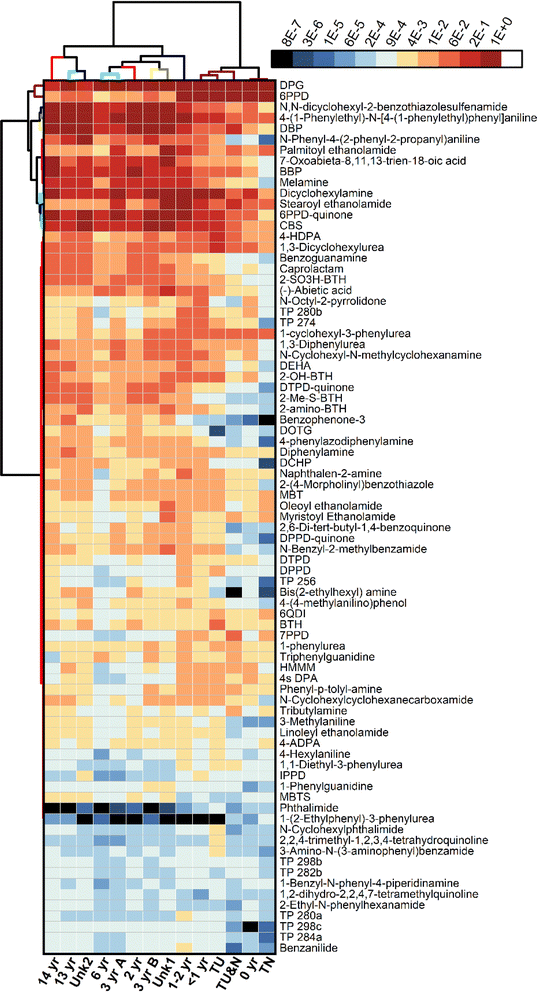 |
| Fig. 5 Hierarchical clustering of tire and turf crumb rubber extractables in positive ion mode. Peak area data was transformed to the log10 scale. Ward linkage algorithm and Euclidean distance metric were used for the analysis. | |
Suspect screening of the crumb rubber extractables revealed the presence of potentially harmful compounds. These include the PPD TPs 4-nitrosodiphenylamine, 4-aminodiphenylamine, and diphenylamine, which are potentially carcinogenic and genotoxic.57–59 Phthalates are another compound class of concern due to their status as endocrine disruptors.60 Three phthalates were identified: dibutyl phthalate, benzyl butyl phthalate and dicyclohexyl phthalate. Another plasticizer, bis(2-ethylhexyl)adipate, was also detected and is a potential human carcinogen.61 Genotoxic rubber antioxidants were identified including: 2-naphthylamine and dicyclohexylamine.62,63
Another major class of compounds identified in the suspect screening were benzothiazoles and their TPs, which have been previously detected in tire crumb rubber.64–67 Additional benzothiazoles not selected for targeted quantitation were identified during suspect screening. These include 2-hydroxybenzothiazole (2-OH-BTH) which exhibits genotoxicity and cytotoxicity.53 Also detected was N-cyclohexyl-2-benzothiazol-amine (NCBA), a component formed in the vulcanization of N-cyclohexyl-2-benzothiazole sulfenamide (CBS), and 2,2-dithiobis(benzothiazole) (MBTS), which is generated from oxidation of 2-mercaptobenzothiazole (MBT).68–70 We also detected N,N′-dicyclohexyl-2-benzothiazole sulfenamide (DCBS), a potential TP formed when dicyclohexylamine undergoes hydrolysis18,71
4 Conclusions
This research aimed to assess the chemical profile and transport pathways of artificial turf and tire crumb rubber of varied ages by developing a multi-faceted workflow using high-resolution mass spectrometry. To assess transport pathways, samples underwent three complementary preparations: extraction with organic solvent, water leaching, and a bioaccessibility-based extraction to mimic ingestion. Both quantitative and qualitative information was gained by utilizing two complementary instrumentation techniques. First, our results demonstrate that different sample preparation methods provide different fractions of rubber-derived chemicals. Using 6PPD and 6PPD-quinone as an example, we found that in addition to polarity, pH also controls the transport of compounds. Through targeted quantitation, chemical profiling, and suspect screening, we found that the aging of artificial turf crumb rubber affects the transformation and transport of rubber-derived chemicals. The rate of formation of transformation products and clustering analysis suggests that the first two years of aging can be critical for the environmental impact of artificial turf fields. However, more studies with controlled aging experiments over longer periods of time are needed to assess this further. Our analysis provided insight into the high chemical complexity of crumb rubber samples of different ages, and emphasizes the current limitations within the field. While we can utilize chemical profiles to understand complex samples by assessing diversity, abundance, polarity, and molecular mass, we still recognize that the limited compound identification is a major bottleneck in our analysis. More extensive non-target screening of tire and artificial turf crumb rubber samples of varied ages is needed to prioritize compounds of interest and give insight into the environmental impact of artificial turf fields.
Data availability
The data supporting this article have been included as part of the ESI.†
Author contributions
Madison H. McMinn: formal analysis, methodology, investigation, validation, visualization, writing – original draft, writing – review & editing. Ximin Hu: formal analysis, software, writing – review & editing. Katherine Poisson: methodology, writing – review & editing. Phillip Berger: writing – review & editing. Paola Pimentel: investigation. Xinwen Zhang: investigation. Pranali Ashara: investigation. Ella L. Greenfield: investigation. Jessica Eig: investigation. Zhenyu Tian: conceptualization, supervision, writing – original draft, writing – review & editing.
Conflicts of interest
There are no conflicts to declare.
Acknowledgements
This work was supported by the Northeastern University TIER 1 Seed Grant and NSF grant # 2404911. We thank the National Science Foundation Graduate Research Fellowship Program for financial support to M. H. M. We thank the McIntyre group at Washington State University and the Kolodziej group at the University of Washington for providing tire wear particle samples. We thank the US tire Manufacturers Association for providing cryo-milled tire tread samples.
References
- A. C. Cummins, Global ELT Management – A Global State of Knowledge on Regulation, Management Systems, Impacts of Recovery and Technologies, 2019 Search PubMed.
- P. Grammelis, N. Margaritis, P. Dallas, D. Rakopoulos and G. Mavrias, A Review on Management of End of Life Tires (ELTs) and Alternative Uses of Textile Fibers, Energies, 2021, 14, 571, DOI:10.3390/en14030571.
- F. Valentini and A. Pegoretti, End-of-life options of tyres. A review, Adv. Ind. Eng. Polym. Res., 2022, 5, 203–213, DOI:10.1016/j.aiepr.2022.08.006.
- A. N. Perkins, S. H. Inayat-Hussain, N. C. Deziel, C. H. Johnson, S. S. Ferguson, R. Garcia-Milian, D. C. Thompson and V. Vasiliou, Evaluation of potential carcinogenicity of organic chemicals in synthetic turf crumb rubber, Environ. Res., 2019, 169, 163–172, DOI:10.1016/j.envres.2018.10.018.
- U. S. EPA, Synthetic Turf Field Recycled Tire Crumb Rubber Research Under the Federal Research Action Plan Final Report Part 1–Tire Crumb Rubber Characterization Volume 1, 2019 Search PubMed.
- Z. Tian, H. Zhao, K. T. Peter, M. Gonzalez, J. Wetzel, C. Wu, X. Hu, J. Prat, E. Mudrock, R. Hettinger, A. E. Cortina, R. G. Biswas, F. V. C. Kock, R. Soong, A. Jenne, B. Du, F. Hou, H. He, R. Lundeen, A. Gilbreath, R. Sutton, N. L. Scholz, J. W. Davis, M. C. Dodd, A. Simpson, J. K. McIntyre and E. P. Kolodziej, A ubiquitous tire rubber-derived chemical induces acute mortality in coho salmon, Science, 2021, 371, 185–189, DOI:10.1126/science.abd6951.
- D. Armada, M. Llompart, M. Celeiro, P. Garcia-Castro, N. Ratola, T. Dagnac and J. de Boer, Global evaluation of the chemical hazard of recycled tire crumb rubber employed on worldwide synthetic turf football pitches, Sci. Total Environ., 2022, 812, 152542, DOI:10.1016/j.scitotenv.2021.152542.
- P. Zuccaro, D. C. Thompson, J. De Boer, A. Watterson, Q. Wang, S. Tang, X. Shi, M. Llompart, N. Ratola and V. Vasiliou, Artificial turf and crumb rubber infill: An international policy review concerning the current state of regulations, Environ. Challenges, 2022, 100620 CrossRef PubMed.
- M. Wang, L. Zhang, A. Li, M. Irfan, Y. Du and W. Di, Comparative pyrolysis behaviors of tire tread and side wall from waste tire and characterization of the resulting chars, J. Environ. Manage., 2019, 232, 364–371, DOI:10.1016/j.jenvman.2018.10.091.
- J. Rausch, D. Jaramillo-Vogel, S. Perseguers, N. Schnidrig, B. Grobety and P. Yajan, Automated identification and quantification of tire wear particles (TWP) in airborne dust: SEM/EDX single particle analysis coupled to a machine learning classifier, Sci. Total Environ., 2022, 803, 149832, DOI:10.1016/j.scitotenv.2021.149832.
- M. L. Kreider, J. M. Panko, B. L. McAtee, L. I. Sweet and B. L. Finley, Physical and chemical characterization of tire-related particles: comparison of particles generated using different methodologies, Sci. Total Environ., 2010, 408, 652–659, DOI:10.1016/j.scitotenv.2009.10.016.
- M. E. Stack, K. Hollman, N. Mladenov, B. Harper, F. Pinongcos, K. E. Sant, C. M. Rochman, W. Richardot, N. G. Dodder and E. Hoh, Micron-size tire tread particles leach organic compounds at higher rates than centimeter-size particles: Compound identification and profile comparison, Environ. Pollut., 2023, 334, 122116, DOI:10.1016/j.envpol.2023.122116.
- S. Kim, J. Y. Yang, H. H. Kim, I. Y. Yeo, D. C. Shin and Y. W. Lim, Health risk assessment of lead ingestion exposure by particle sizes in crumb rubber on artificial turf considering
bioavailability, Environmental Health and Toxicology, 2012, 27, e2012005, DOI:10.5620/eht.2012.27.e2012005.
- D. Armada, A. Martinez-Fernandez, M. Celeiro, T. Dagnac and M. Llompart, Assessment of the bioaccessibility of PAHs and other hazardous compounds present in recycled tire rubber employed in synthetic football fields, Sci. Total Environ., 2023, 857, 159485, DOI:10.1016/j.scitotenv.2022.159485.
- J. Gomes, H. Mota, J. Bordado, M. Cadete, G. Sarmento, A. Ribeiro, M. Baiao, J. Fernandes, V. Pampulim, M. Custodio and I. Veloso, Toxicological assessment of coated versus uncoated rubber granulates obtained from used tires for use in sport facilities, J. Air Waste Manage. Assoc., 2010, 60, 741–746, DOI:10.3155/1047-3289.60.6.741.
- P. Klöckner, T. Reemtsma, P. Eisentraut, U. Braun, A. S. Ruhl and S. Wagner, Tire and road wear particles in road environment–Quantification and assessment of particle dynamics by Zn determination after density separation, Chemosphere, 2019, 222, 714–721, DOI:10.1016/j.chemosphere.2019.01.176.
- K. T. Peter, Z. Tian, C. Wu, P. Lin, S. White, B. Du, J. K. McIntyre, N. L. Scholz and E. P. Kolodziej, Using High-Resolution Mass Spectrometry to Identify Organic Contaminants Linked to Urban Stormwater Mortality Syndrome in Coho Salmon, Environ. Sci. Technol., 2018, 52, 10317–10327, DOI:10.1021/acs.est.8b03287.
- B. Seiwert, P. Klöckner, S. Wagner and T. Reemtsma, Source-related smart suspect screening in the aqueous environment: search for tire-derived persistent and mobile trace organic contaminants in surface waters, Anal. Bioanal. Chem., 2020, 412, 4909–4919 CrossRef CAS PubMed.
- M. Kovochich, J. A. Parker, S. C. Oh, J. P. Lee, S. Wagner, T. Reemtsma and K. M. Unice, Characterization of Individual Tire and Road Wear Particles in Environmental Road Dust, Tunnel Dust, and Sediment, Environ. Sci. Technol. Lett., 2021, 8, 1057–1064, DOI:10.1021/acs.estlett.1c00811.
- M. Brinkmann, D. Montgomery, S. Selinger, J. G. P. Miller, E. Stock, A. J. Alcaraz, J. K. Challis, L. Weber, D. Janz, M. Hecker and S. Wiseman, Acute Toxicity of the Tire Rubber-Derived Chemical 6PPD-quinone to Four Fishes of Commercial, Cultural, and Ecological Importance, Environ. Sci. Technol. Lett., 2022, 9, 333–338, DOI:10.1021/acs.estlett.2c00050.
- K. Hiki and H. Yamamoto, The Tire-Derived Chemical 6PPD-quinone Is Lethally Toxic to the White-Spotted Char Salvelinus leucomaenis pluvius but Not to Two Other Salmonid Species, Environ. Sci. Technol. Lett., 2022, 9, 1050–1055, DOI:10.1021/acs.estlett.2c00683.
- D. E. Latch, J. L. Packer, W. A. Arnold and K. McNeill, Photochemical conversion of triclosan to 2,8-dichlorodibenzo-p-dioxin in aqueous solution, J. Photochem. Photobiol., A, 2003, 158, 63–66, DOI:10.1016/S1010-6030(03)00103-5.
- R. Liu and S. A. Mabury, Organophosphite Antioxidants in Indoor Dust Represent an Indirect Source of Organophosphate Esters, Environ. Sci. Technol., 2019, 53, 1805–1811, DOI:10.1021/acs.est.8b05545.
- L. Fohet, J. M. Andanson, T. Charbouillot, L. Malosse, M. Leremboure, F. Delor-Jestin and V. Verney, Time-concentration profiles of tire particle additives and transformation products under natural and artificial aging, Sci. Total Environ., 2023, 859, 160150, DOI:10.1016/j.scitotenv.2022.160150.
- S. Weyrauch, B. Seiwert, M. Voll, S. Wagner and T. Reemtsma, Accelerated aging of tire and road wear particles by elevated temperature, artificial sunlight and mechanical stress - A laboratory study on particle properties, extractables and leachables, Sci. Total Environ., 2023, 904, 166679, DOI:10.1016/j.scitotenv.2023.166679.
- F. Zhao, J. Z. Yao, X. Y. Liu, M. Deng, X. J. Chen, C. Z. Shi, L. Yao, X. F. Wang and M. L. Fang, Occurrence
and Oxidation Kinetics of Antioxidant p-Phenylenediamines and Their Quinones in Recycled Rubber Particles from Artificial Turf, Environ. Sci. Technol. Lett., 2024, 11, 335–341, DOI:10.1021/acs.estlett.3c00948.
- U. S. T. M. Association, Cryo-Milled Tire Tread (CMTT) Overview of Production, Characterization and Composition Search PubMed.
- T. Masset, B. J. D. Ferrari, W. Dudefoi, K. Schirmer, A. Bergmann, E. Vermeirssen, D. Grandjean, L. C. Harris and F. Breider, Bioaccessibility of Organic Compounds Associated with Tire Particles Using a Fish In Vitro Digestive Model: Solubilization Kinetics and Effects of Food Coingestion, Environ. Sci. Technol., 2022, 56, 15607–15616, DOI:10.1021/acs.est.2c04291.
- B. T. Pavilonis, C. P. Weisel, B. Buckley and P. J. Lioy, Bioaccessibility and Risk of Exposure to Metals and SVOCs in Artificial Turf Field Fill Materials and Fibers, Risk Anal., 2014, 34, 44–55, DOI:10.1111/risa.12081.
- J. J. Zhang, I. K. Han, L. Zhang and W. Crain, Hazardous chemicals in synthetic turf materials and their bioaccessibility in digestive fluids, J. Exposure Sci. Environ. Epidemiol., 2008, 18, 600–607, DOI:10.1038/jes.2008.55.
- D. Mohamed, D. Y. Kim, J. An, M. Kim, S. H. Chun and J. H. Kwon, Simplified Unified BARGE Method to Assess Migration of Phthalate Esters in Ingested PVC Consumer Products, Int. J. Environ. Res. Public Health, 2023, 20, 1907, DOI:10.3390/ijerph20031907.
- S. Denys, J. Caboche, K. Tack, G. Rychen, J. Wragg, M. Cave, C. Jondreville and C. Feidt, In vivo validation of the unified BARGE method to assess the bioaccessibility of arsenic, antimony, cadmium, and lead in soils, Environ. Sci. Technol., 2012, 46, 6252–6260, DOI:10.1021/es3006942.
- L. K. Pino, B. C. Searle, J. G. Bollinger, B. Nunn, B. MacLean and M. J. MacCoss, The Skyline ecosystem: Informatics for quantitative mass spectrometry proteomics, Mass Spectrom. Rev., 2020, 39, 229–244, DOI:10.1002/mas.21540.
- K. J. Adams, B. Pratt, N. Bose, L. G. Dubois, L. St John-Williams, K. M. Perrott, K. Ky, P. Kapahi, V. Sharma, M. J. MacCoss, M. A. Moseley, C. A. Colton, B. X. MacLean, B. Schilling, J. W. Thompson and C. Alzheimer's Disease Metabolomics, Skyline for Small Molecules: A Unifying Software Package for Quantitative Metabolomics, J. Proteome Res., 2020, 19, 1447–1458, DOI:10.1021/acs.jproteome.9b00640.
- H. N. Zhao, X. Hu, M. Gonzalez, C. A. Rideout, G. C. Hobby, M. F. Fisher, C. J. McCormick, M. C. Dodd, K. E. Kim, Z. Tian and E. P. Kolodziej, Screening p-Phenylenediamine Antioxidants, Their Transformation Products, and Industrial Chemical Additives in Crumb Rubber and Elastomeric Consumer Products, Environ. Sci. Technol., 2023, 57, 2779–2791, DOI:10.1021/acs.est.2c07014.
- U. S. EPA, Guidance for Data Useability in Risk Assessment, Report EPA/540/G-90/008, 1990 Search PubMed.
- U. S. EPA, Definition and Procedure for the Determination of the Method Detection Limit, Revision 2, 2016 Search PubMed.
- F. Hou, Z. Tian, K. T. Peter, C. Wu, A. D. Gipe, H. Zhao, E. A. Alegria, F. Liu and E. P. Kolodziej, Quantification of organic contaminants in urban stormwater by isotope dilution and liquid chromatography-tandem mass spectrometry, Anal. Bioanal. Chem., 2019, 411, 7791–7806, DOI:10.1007/s00216-019-02177-3.
- P. Virtanen, R. Gommers, T. E. Oliphant, M. Haberland, T. Reddy, D. Cournapeau, E. Burovski, P. Peterson, W. Weckesser, J. Bright, S. J. van der Walt, M. Brett, J. Wilson, K. J. Millman, N. Mayorov, A. R. J. Nelson, E. Jones, R. Kern, E. Larson, C. J. Carey, I. Polat, Y. Feng, E. W. Moore, J. VanderPlas, D. Laxalde, J. Perktold, R. Cimrman, I. Henriksen, E. A. Quintero, C. R. Harris, A. M. Archibald, A. H. Ribeiro, F. Pedregosa, P. van Mulbregt and C. SciPy, SciPy 1.0: fundamental algorithms for scientific computing in Python, Nat. Methods, 2020, 17, 261–272, DOI:10.1038/s41592-019-0686-2.
- V. Pedregosa FGael, A. Gramfort, V. Michel, B. Thirion and O. Grisel, et al, Scikit-learn: Machine learning in Python, J. Mach. Learn. Res., 2011, 12, 2825–2830, DOI:10.5555/1953048.2078195.
- J. S. D. W. Meals, S. A. Dressing, and J. B. Harcum, Statistical Analysis for Monotonic Trends, 2011 Search PubMed.
- D. R. Helsel and L. M. Frans, Regional Kendall test for trend, Environ. Sci. Technol., 2006, 40, 4066–4073, DOI:10.1021/es051650b.
- S. Tisler, P. Savvidou, M. B. Jorgensen, M. Castro and J. H. Christensen, Supercritical Fluid Chromatography Coupled to High-Resolution Mass Spectrometry Reveals Persistent Mobile Organic Compounds with Unknown Toxicity in Wastewater Effluents, Environ. Sci. Technol., 2023, 57, 9287–9297, DOI:10.1021/acs.est.3c00120.
- C. Liao, U. J. Kim and K. Kannan, A Review of Environmental Occurrence, Fate, Exposure, and Toxicity of Benzothiazoles, Environ. Sci. Technol., 2018, 52, 5007–5026, DOI:10.1021/acs.est.7b05493.
- L. Chibwe, J. L. Parrott, K. Shires, H. Khan, S. Clarence, C. Lavalle, C. Sullivan, A. M. O'Brien, A. O. De Silva, D. C. G. Muir and C. M. Rochman, A Deep Dive into the Complex Chemical Mixture and Toxicity of Tire Wear Particle Leachate in Fathead Minnow, Environ. Toxicol. Chem., 2022, 41, 1144–1153, DOI:10.1002/etc.5140.
- M. Marques Dos Santos, C. Cheriaux, S. Jia, M. Thomas, H. Gallard, J. P. Croue, P. Carato and S. A. Snyder, Genotoxic effects of chlorinated disinfection by-products of 1,3-diphenylguanidine (DPG): Cell-based in-vitro testing and formation potential during water disinfection, J. Hazard. Mater., 2022, 436, 129114, DOI:10.1016/j.jhazmat.2022.129114.
- C. J. De Hoogh, A. J. Wagenvoort, F. Jonker, J. A. Van Leerdam and A. C. Hogenboom, HPLC-DAD and Q-TOF MS techniques identify cause of Daphnia biomonitor alarms in the River Meuse, Environ. Sci. Technol., 2006, 40, 2678–2685, DOI:10.1021/es052035a.
- H. Y. Zhang, Z. Huang, Y. H. Liu, L. X. Hu, L. Y. He, Y. S. Liu, J. L. Zhao and G. G. Ying, Occurrence and risks of 23 tire additives and their transformation products in an urban water system, Environ. Int., 2023, 171, 107715, DOI:10.1016/j.envint.2022.107715.
- X. Peng, T. Yang, S. Guo, J. Zhou, G. Chen, Z. Zhu and J. Tan, Revealing chemical release from plastic debris in animals' digestive systems using nontarget and suspect screening and simulating digestive fluids, Environ. Pollut., 2024, 348, 123793, DOI:10.1016/j.envpol.2024.123793.
- K. Schneider, M. de Hoogd, P. Haxaire, A. Philipps, A. Bierwisch and E. Kaiser, ERASSTRI - European Risk Assessment Study on Synthetic Turf Rubber Infill - Part 2: Migration and monitoring studies, Sci. Total Environ., 2020, 718, 137173, DOI:10.1016/j.scitotenv.2020.137173.
- T. Masset, B. J. D. Ferrari, D. Oldham, W. Dudefoi, M. Minghetti, K. Schirmer, A. Bergmann, E. Vermeirssen and F. Breider, In Vitro Digestion of Tire Particles in a Fish Model (Oncorhynchus mykiss): Solubilization Kinetics of Heavy Metals and Effects of Food Coingestion, Environ. Sci. Technol., 2021, 55, 15788–15796, DOI:10.1021/acs.est.1c04385.
- X. M. Hu, H. Q. N. N. Zhao, Z. Y. Tian, K. T. Peter, M. C. Dodd and E. P. Kolodziej, Transformation Product Formation upon Heterogeneous Ozonation of the Tire Rubber Antioxidant 6PPD (N-(1,3-dimethylbutyl)-N′-phenyl-p-phenylenediamine), Environ. Sci. Technol. Lett., 2022, 9, 413–419, DOI:10.1021/acs.estlett.2c00187.
- Y. Ye, J. Weiwei, L. Na, M. Mei, R. Kaifeng and W. Zijian, Application of the SOS/umu test and high-content in vitro micronucleus test to determine genotoxicity and cytotoxicity of nine benzothiazoles, J. Appl. Toxicol., 2014, 34, 1400–1408, DOI:10.1002/jat.2972.
- Y. J. Zhou, L. Yixi, Q. Q. Kong, J. L. Peng, Y. H. Pan, J. L. Qiu and X. Yang, Sunlight-Induced Transformation of Tire Rubber Antioxidant N-(1,3-Dimethylbutyl)-N′-phenyl-p-phenylenediamine (6PPD) to 6PPD-Quinone in Water, Environ. Sci. Technol. Lett., 2023, 10, 798–803, DOI:10.1021/acs.estlett.3c00499.
- E. L. Schymanski, J. Jeon, R. Gulde, K. Fenner, M. Ruff, H. P. Singer and J. Hollender, Identifying small molecules via high resolution mass spectrometry: communicating confidence, Environ. Sci. Technol., 2014, 48, 2097–2098, DOI:10.1021/es5002105.
- H. N. Zhao, X. Hu, Z. Tian, M. Gonzalez, C. A. Rideout, K. T. Peter, M. C. Dodd and E. P. Kolodziej, Transformation Products of Tire Rubber Antioxidant 6PPD in Heterogeneous Gas-Phase Ozonation: Identification and Environmental Occurrence, Environ. Sci. Technol., 2023, 57, 5621–5632, DOI:10.1021/acs.est.2c08690.
- 4-Nitrosodiphenylamine, U. S. EPA, https://comptox.epa.gov/dashboard/chemical/details/DTXSID1021031 (accessed May 14, 2024) Search PubMed.
- Diphenylamine, U. S. EPA, https://comptox.epa.gov/dashboard/chemical/details/DTXSID4021975 (accessed May 14, 2024) Search PubMed.
- N-Phenyl-1,4-benzenediamine, U. S. EPA, https://comptox.epa.gov/dashboard/chemical/details/DTXSID7025895 (accessed May 14, 2024) Search PubMed.
- K. Baralic, A. Buha Djordjevic, K. Zivancevic, E. Antonijevic, M. Andelkovic, D. Javorac, M. Curcic, Z. Bulat, B. Antonijevic and D. Dukic-Cosic, Toxic Effects of the Mixture of Phthalates and Bisphenol A-Subacute Oral Toxicity Study in Wistar Rats, Int. J. Environ. Res. Public Health, 2020, 17, 746, DOI:10.3390/ijerph17030746.
- Bis(2-ethylhexyl)hexanedioate, U. S. EPA, https://comptox.epa.gov/dashboard/chemical/details/DTXSID0020606 (accessed May 14, 2024) Search PubMed.
- 2-Naphthylamine, U. S. EPA, https://comptox.epa.gov/dashboard/chemical/details/DTXSID2020921 (accessed May 14, 2024) Search PubMed.
- Dicyclohexylamine, U. S. EPA, https://comptox.epa.gov/dashboard/chemical/details/DTXSID6025018 (accessed May 14, 2024) Search PubMed.
- M. Feltracco, G. Mazzi, E. Barbaro, B. Rosso, F. Sambo, S. Biondi, C. Barbante and A. Gambaro, Occurrence and phase distribution of benzothiazoles in untreated highway stormwater runoff and road dust, Environ. Sci. Pollut. Res., 2023, 30, 107878–107886, DOI:10.1007/s11356-023-30019-4.
- C. Bertoldi, A. de Cassia Campos Pena, A. Dallegrave, A. N. Fernandes and M. Gutterres, Photodegradation of Emerging Contaminant 2-(tiocyanomethylthio) Benzothiazole (TCMTB) in Aqueous Solution: Kinetics and Transformation Products, Bull. Environ. Contam. Toxicol., 2020, 105, 433–439, DOI:10.1007/s00128-020-02954-2.
- E. Borowska, E. Felis and J. Kalka, Oxidation of benzotriazole and benzothiazole in photochemical processes: Kinetics and formation of transformation products, Chem. Eng. J., 2016, 304, 852–863, DOI:10.1016/j.cej.2016.06.123.
- K. Kowalska, E. Felis, A. Sochacki and S. Bajkacz, Removal and transformation pathways of benzothiazole and benzotriazole in membrane bioreactors treating synthetic municipal wastewater, Chemosphere, 2019, 227, 162–171, DOI:10.1016/j.chemosphere.2019.04.037.
- H. Kumata, J. Yamada, K. Masuda, H. Takada, Y. Sato, T. Sakural and K. Fujiwara, Benzothiazolamines as tire-derived molecular markers: sorptive behavior in street runoff and application to source apportioning, Environ. Sci. Technol., 2002, 36, 702–708, DOI:10.1021/es0155229.
- H. R. Li, F. Y. Han, L. J. Jiang, T. Q. Yang, L. Du and J. Q. Zhu, Continuous Synthesis of N-Cyclohexyl-2-benzothiazole Sulfenamide with Microfluidics and Its Kinetic Study, Ind. Eng. Chem. Res., 2021, 60, 14134–14142, DOI:10.1021/acs.iecr.1c02881.
- S. H. Pan, Y. L. Sun, G. Zhang, J. Li, Q. L. Xie and P. Chaktaborty, Assessment of 2-(4-morpholinyl) benzothiazole (24MoBT) and N-cyclohexyl-2-benzothiazolamine (NCBA) as traffic tracers in metropolitan cities of China and India, Atmos. Environ., 2012, 56, 246–249, DOI:10.1016/j.atmosenv.2012.03.029.
- C. Johannessen, J. Liggio, X. M. Zhang, A. Saini and T. Harner, Composition and transformation chemistry of tire-wear derived organic chemicals and implications for air pollution, Atmos. Pollut. Res., 2022, 13 DOI:10.1016/j.apr.2022.101533.
|
This journal is © The Royal Society of Chemistry 2024 |