The effects of CdSe/ZnS quantum dots on autofluorescence properties and growth of algae Desmodesmus communis: dependence on cultivation medium†
Received
19th December 2023
, Accepted 2nd March 2024
First published on 11th March 2024
Abstract
The discovery and development of quantum dots (QDs), which are tiny luminescent nanoparticles (NPs), was awarded the Nobel Prize in Chemistry in 2023, highlighting the prospects of their technological applications. However, it is still not very well known how such NPs could affect aquatic ecosystems, especially given that natural conditions are varied and unpredictable. Thus, the aim of this study was to determine the influence of natural and artificial aqueous media on the interaction of CdSe/ZnS–COOH QDs with Desmodesmus communis algae using fast non-invasive optical methods. The cumulative short-term effects of QDs on algae growth and population structure were evaluated and changes in the photosynthetic activity of green algae were demonstrated using pulse-amplitude modulated (PAM) fluorometry. The present study demonstrated that natural and artificial cultivation media had different effects on the growth pattern, physiological processes and photoadaptation properties of algae as well as altering their responses to core/shell QDs. Thus, to fully assess the environmental risk of NPs, it is necessary to evaluate the simultaneous interactions of NPs with abiotic and biotic factors using a combination of physico-optical and ecotoxicological tools.
Environmental significance
This short-term study provides new information about how various environmental conditions can alter the effects of nanoparticles on green algae, which play a key role in the food chain in the aquatic environment. There is a possibility that, due to increasing technological needs and applicability, QDs will eventually end up in surface water and groundwater, posing a risk to ecosystems. Our experiments showed that the growth of the tested algae was better in the artificial medium, and the QDs properties changed less compared to natural media. Therefore, it can be assumed that the results obtained using artificial media for algae cultivation, as well as the conclusions and predictions made on their basis, can only be extrapolated to natural aquatic environments with great caution.
|
Introduction
The nanosize range of materials has been the focus of scientific interest and technological applications for some time due to the quantum properties inherent in materials of this size. Although nanoobjects, such as large polymer biomolecules, occur naturally, the smallest artificial semiconductor nanoparticles – quantum dots (QDs) – have large surface areas relative to their volume, resulting in size-dependent photoluminescence (PL) and allowing QDs to interact unpredictably with their environment. Because biocompatible photoluminescent QDs can be easily detected and tracked,1 they are used in optical instruments or monitoring techniques also intended for direct contact with living organisms. The hydrophilic QDs are sensitive to various environmental factors, including temperature,2 medium pH,3 and the presence of ions or proteins.4,5 Therefore, these NPs can be used as sensors for rapid detection of environmental pollutants (for instance, heavy metals, pesticides and antibiotics) in food.6 The interaction of QDs with biological systems7 is closely correlated with their structure, surface properties (including diameter of core, presence of shell, types of surface ligands and coating) and exposure duration.8,9 The metal-containing QDs are widely used in a variety of biomedical and industrial applications,10 which can lead to their appearance in water bodies and then in the food chain,11 thereby creating a potential risk to animal and human health.12
There are a lot of studies that have confirmed the uptake of QDs and demonstrated their translocation in various organisms.7,11,13,14 While aquatic organisms are often used in studies assessing toxicological and ecotoxicological risk due to the sensitivity of their physiological processes (especially photosynthesis) to pollutants and the simplicity of cultivation, there are few works11,15,16 related to the study of the internalization and toxicity of metal-based QDs to algae, which become one of the first targets of QDs in the aquatic environment. Algae are capable of accumulating various contaminants, including heavy metals, and often serve as model organisms for more complex plants in studies to assess the toxic effects of substances on ecosystems and global environmental processes.17 In addition, as primary producers, the algae play a key role in the aquatic food chain. Therefore, according to Grigoriev et al.18 changes in the species composition and structure of algal communities can influence the flow of photosynthetically produced energy in aquatic ecosystems. Generally, the impact of NPs on algae is associated with the occurrence of oxidative stress, which can affect gene expression, metabolism, photosynthesis, nitrogen fixation and growth of algae.19 However, there is insufficient information on the possible mechanisms of QDs phytonanotoxicity.
Transition of core QDs into a less stable state under the influence of various environmental factors (solvent, light, biological materials) is sensitively reflected both in changes in the PL intensity and a spectral shift of the PL band.20 Moreover, the impact of NPs on the photoadaptation of wild type microalgae has been revealed by autofluorescence (AF) measurements. Thus, optical methods work well for non-invasive, fast, real-time and possibly remote assessment of both the stability of QDs and their effects on algae. In a number of studies, pulse-amplitude modulated (PAM) fluorometry has been used to explore the effects of metal on the process of photosynthesis.21,22 The fluorescence (FL) intensity of chlorophyll a (Chl a) serves as an indicator of its content and electron transport inhibition in autotrophs.23 It can also reflect the interactions of metals with photosystem II (PSII), membrane degradation and the efficiency of photosynthetic electron transport. The non-photochemical quenching (NPQ) indicates the relative increase in the sum of the rate constants of the non-photochemical deactivation processes (FL emission, heat dissipation and spillover of an excitation energy from PSII to PSI) relative to the dark-adapted state.23 However, there are very few studies that have used PAM methodology to evaluate the effects of QDs on algae.24 Additionally, the associated changes in the Kausky curves and the quantum yield of FL quenching have not been presented and discussed.
Since there may be a significant mismatch between the results of toxicity studies of NPs conducted only in artificial aquatic media and actual potential contamination scenarios in natural conditions, one artificial algae cultivation medium and two natural aquatic media (deep-well water and lake water) were used in this study, these latter more closely replicating the conditions that QDs interact with algae in nature. The interaction of NPs with biota in natural waters turns out to be interconnected and, when assessing toxicity, it is necessary to take into account the influence of the presence and composition of natural organic matter or exopolymeric substances produced by bacteria, algae and fungi25,26 as well as other abiotic factors.27 Moreover, the different chemical composition of algae cultivation media has influence on QDs stability.28 Even the aggregation of the QDs caused by the higher ionic strength in algae growth media compared to distilled water does not suppress contacts between NPs and algae cells.29,30 Thus, and as yet unstudied, the simultaneous interaction of NPs with abiotic and biotic factors may have an unpredictable impact on organisms in the aquatic ecosystem.
Desmodesmus sp. are among the most popular green freshwater microalgae species used in toxicity studies. However, the toxicity assessment of QDs on algae must be conducted under environmentally relevant conditions that can fully account for heterogeneous causes influenced by various environmental factors. Therefore, after optical measurements of the photostability of negatively charged hydrophilic CdSe QDs capped with ZnS shell in natural and artificial cultivation media and in the presence of Desmodesmus communis, a combination of physico-optical and ecotoxicological techniques was applied in the present study to compare the cumulative short-term effects of QDs on the algal growth, population structure and photosynthesis activity in the selected lake water, deep-well water and artificial algae growth medium.
Experimental
Materials
Two natural and one artificial aqueous media were used in two sets (without and with CdSe/ZnS core/shell quantum dots) to evaluate the growth of green microalgae Desmodesmus communis exposed to QDs. The detailed composition of the natural deep well water (DWW) (pH 8.3) and Lake Balsys water (Balsys) (pH 8.8) is given in Tables S1 and S2.† A description of the modified Wilkins–Chalgren (MWC) algae grow medium (pH 7.6) is available in open sources.31 Analytical or higher-grade reagents (Sigma Aldrich, Germany and Carl Roth, Germany) were used in the preparation of the medium.
The green algae Desmodesmus communis (former Scenedesmus communis (Turpin) Brébisson) isolate 2012/KM/G2 was obtained from the collection of pure cultures of algae and cyanobacteria at the Nature Research Centre, Lithuania.32 Culture isolate was cultivated as unialgal (but non-axenic) culture in MWC with the addition of selenium at +21 °C under continuous illumination (approximately 100 μmol photons per m2 s) using a cool white fluorescent light source.
Commercially available semiconductor CdSe/ZnS core/shell quantum dots coated with a polymer layer (polyethylene glycol (PEG)) having carboxylic acid (–COOH) side groups (a PL peak at 625 ± 5 nm, cat. no. A10200 (Life Technologies, USA)) were used as model hydrophilic nanoparticles possessing a negative surface charge, this allowing facile dispersion of QDs in aqueous solutions with retention of their optical properties. The concentration of QDs suspended in the stock solution prepared using aqueous 50 nM borate buffer (pH 9) was 8 μM. The samples with final concentrations of QDs were prepared for measurements by diluting the stock solution with Lake Balsys water, DWW and MWC medium.
The QDs stability and microalgae autofluorescence
Samples used for photoluminescence and autofluorescence measurements were prepared in a 24-well plate (Buddeberg GmbH, Germany) by pouring 2 mL of the test aqueous medium with or without QDs at a final 4 nM (or 40 nM) concentration and with or without green algae inoculum to get ∼5 × 105 cells per mL (or ∼5 × 106 cells per mL). Samples of each medium (DWW and MWC) were divided into two groups for lower and higher concentrations of QDs and algae, each consisting of six wells. Two wells of each group contained algae with QDs, two wells were filled with the same suspensions of QDs without algae and two wells contained only algae. The well plates were kept in a Shrimp Set Smart aquarium (Aquael, Suwalki, Poland) at about 21 ± 1 °C under continuous illumination with a white light emitting diode lamp (34 μmol photons per m2 s).
Spectral measurements of the samples were carried out in 4 × 10 mm plastic cuvettes (Brand GmbH, Germany) immediately after preparation and continued in the subsequent days of the experiment. The optical density (OD) spectra of QDs were measured using an AvaSpec-3648 fibre optic spectrometer (Avantes, Netherlands). A spectrofluorimeter LS55 (PerkinElmer, USA) was used to register the photoluminescence and autofluorescence spectra. Excitation for PL was set at 405 nm, the excitation slit was 10.0 nm, and the emission slit was 2.5 nm. Excitation for AF was set at 480 nm, 435 nm and 411 nm, the excitation and emission slits were 7 nm. Spectroscopic data from pairs of samples of each type were combined to obtain average spectra. The PAM measurements were performed from the bottom of a well at a randomly chosen spot using a JUNIOR-PAM chlorophyll fluorimeter (Heinz Walz GmbH, Germany) after at least 20 min of adaptation in the dark.
The samples were imaged using a fluorescence microscope Eclipse 80i (Nikon, Japan) through the 40×/0.75 objective Plan Fluor, DIC M/N2 (Nikon, Japan) using three different excitation/emission cubes with following optical properties: UV-2A (an excitation range λex = 330–380 nm, a dichroic mirror (DM) 400 nm, a barrier filter (BA) 420 nm) and G-2A (λex = 510–560 nm, DM 575 nm, BA 590 nm). A Digital Sight DS-SMc colour camera (Nikon, Japan) was used to capture the images.
The assay of microalgae growth inhibition
Isolates of D. communis in an exponential growth phase were used for bioassays following OECD recommendations for growth-inhibition testing of freshwater alga and cyanobacteria.33 Three different media (Balsys, DWW, MWC) without and with QDs (Balsys + QDs, DWW + QDs, MWC + QDs) were used to study the inhibition of D. communis growth and autofluorescence. Bioassays were performed in 100 mL Erlenmeyer flasks (Schott Duran, Mainz, Germany) containing 50 mL of each tested medium (without or with QDs at a final 4 nM concentration) with the green algal inoculum of ∼104 cells per mL. Samples were maintained at +21 °C under continuous illumination of approximately 100 μmol photons per m2 s using a cool white fluorescent light source. All assays were run in quintuplicate for 96 h. Cell numbers were scored through a light microscope Ti (Nikon, Japan) using a Fuchs–Rosenthal chamber at ×400 magnification after 24, 48, 72 and 96 hours of treatment. To evaluate the changes in the structure of the algal population, the numbers of cells in cenobia (one, two, four, and eight-celled) were counted.
Statistical analysis
Bioassay data were first tested for normal distribution by performing Kolmogorov–Smirnov and Shapiro–Wilk tests, and for homogeneity of variances by performing a Levene's test. ANOVA (F-statistic, two-way) followed by post hoc Tukey's HSD test were applied to compare algal responses after different exposure durations (0 h, 24 h, 48 h, 72 h and 96 h) and between corresponding groups of samples with QDs (MWC + QDs, Balsys + QDs and DWW + QDs) and without QDs (MWC, Balsys and DWW) using STATISTICA 10.0 software (TIBCO Software Inc., USA). Bioassay data were expressed as mean ± standard deviations (SD). To express the relationship between the number of cenobia species and the number of individuals, a Shannon–Wiener diversity index was used.34 The OriginPro9 (OriginLab, USA) software was used to analyse spectroscopic data.
Results and discussion
Stability of QDs in media with microalgae
Combined effects of solvent composition and illumination conditions are known to affect the optical properties and structural integrity of QDs. For instance, the degree of reduction in PL intensity induced by light and media depends on both a higher irradiation dose and a lower QDs concentration.35 Proper assessment of toxicity of NPs on algae cells also requires estimating the mutual effects of NPs and other medium components.34,36 Therefore, before determining the effects of CdSe/ZnS–COOH QDs on D. communis, the photostability of the QDs was monitored in the natural algae cultivation media Balsys and DWW and the artificial media MWC. The data of spectral measurements are presented in Fig. 1 and S1–S6.† The optical density spectra of the QDs at the beginning (0 day) and at the end of the experiment (after four days under white light illumination (100 μmol photons per m2 s)) were compared for 4 nM QDs concentration (Fig. 1a). The initial OD spectra of QDs suspended in Balsys and MWC were very similar, but an increased scattering signal was observed in the OD spectra of 4 nM and 40 nM QDs concentrations in DWW (Fig. 1a and S1†). However, excitonic bands at about 475 nm and 615 nm in Balsys slightly decreased and shifted to the red side of the spectrum after 4 days under illumination, while no changes were detected in the OD of the QDs samples in MWC (Fig. 1a). The scattering signal after 4 days decreased markedly along with the OD in the samples of QDs in DWW, and a bathochromic shift of the excitonic absorption band was also observed compared to the QDs samples in MWC.
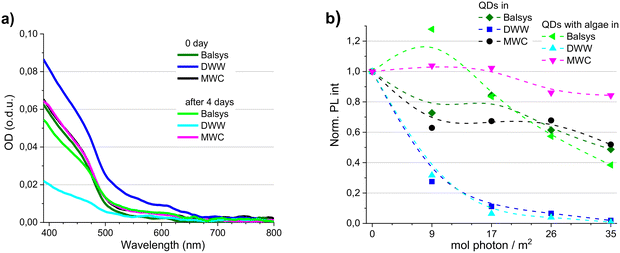 |
| Fig. 1 The optical density (OD) (a) of QDs (4 nM) registered in different media (Balsys, DWW and MWC) at the beginning (0 day) and at the end of the experiment (after 4 days under white light (100 μmol photons per m2 s)). The changes in normalized photoluminescence intensities (Norm. PL int) (b) detected at the peak value of the band (at about 620 nm) of QDs in different media, without and with algae D. communis (initial concentrations ∼104 cells per mL) after 4 days. PL excitation was at 405 nm. The excitation slit was 10.0 nm, and the emission slit was 2.5 nm. The dashed line represents the variation trends, not the experimental results. o.d.u. means optical density units. | |
The initial PL band of the QDs was red-shifted in Balsys and in DWW with respect to that of the QDs in MWC (Fig. S2–S4†). An additional bathochromic shift of the PL band was observed in all three media during the experiment, with the smallest shift being in MWC. The PL intensity also decreased in all QDs samples within 4 days, but the degree of decrease depended on the composition of the medium and chosen illumination conditions (Fig. 1b, S5c and S6†). After 4 days, the PL intensity of QDs was the lowest in DWW, in which the strongest QDs aggregation was observed during the experiment. On the other hand, the PL intensity of QDs in MWC retained about 50% of its initial values. The relative decrease in PL intensity of QDs in Balsys within 4 days was comparable to that in the MWC. Imaging of QDs solutions in DWW and MWC media revealed the presence of the red photoluminescence from the QDs in both media, but the influence of the composition of the surrounding medium on the QDs stability was clearly manifested in the DWW medium, in which the QDs were the least photostable, and the sedimentation of nanoparticles was observed (Fig. S5a and b†). The optical properties of QDs (the intensities of OD and PL, spectral characteristics of PL) in Balsys water demonstrated an intermediate stability compared to other media. Thus, the stability and photostability of the QDs were affected less in the artificial algae cultivation media (MWC) than in the natural media (Lake Balsys and DWW), while the QDs were the least photostable in DWW.
It has been implied that natural exudates in aquatic systems comprising extracellular polymeric substances and protein-rich metabolites can eventually adsorb onto the QDs surface, which can effectively mitigate the inherent toxic effects of the QDs.24 The presence of D. communis algae did not have a noticeable effect on the spectral properties of the QDs immediately after preparation of the samples (Fig. S2–S6†). Although the PL intensity of illuminated QDs also decreased in all three media during the experiment (with one exception in Balsys after one day), the PL of QDs with algae in the case of MWC was more stable than in corresponding samples without algae (Fig. 1b). Also, a smaller bathochromic shift of the PL band was observed in the presence of algae in all media (Fig. S3 and S4†). No improvement in stability of PL intensity of QDs was detected in samples with algae in DWW. These results on algae exposed to QDs complement our previous findings20 that D. communis algae can improve the stability of the optical properties of CdSe/ZnS–COOH QDs in aqueous media under illumination and demonstrate that the protective effect depends not only on the light intensity and exposure time, but also on the composition of the medium. However, when the damaging effects of the medium or light on the stability of the QDs reach a certain critical level, even the presence of algae in the medium cannot help QDs to retain their spectroscopic properties.
The effect of QDs on autofluorescence spectra of microalgae
Physiological processes (especially photosynthesis) in algae as primary producers and indicators of environmental changes are sensitive to sublethal concentrations of contaminants.37 Thus, monitoring of algae autofluorescence by means of optical methods is suitable for evaluating the pressure of surroundings, for example, under exposure to NPs.38 The autofluorescence of D. communis occurs mainly due to the fluorescence of chlorophyll a, thus living algae cells fluoresce in red and dying cells – in blue (Fig. 2). The QDs in media with D. communis localize on cells in both states and may cause the disintegration and death of some cells, − even using a barrier filter at 590 nm, which cut of the photoluminescence signal of QDs, we still saw the bright red fluorescent spots leaked from the cells in the fluorescence images (Fig. 2). However, the cause-and-effect relationship between the localization of QDs and algae status is unclear.
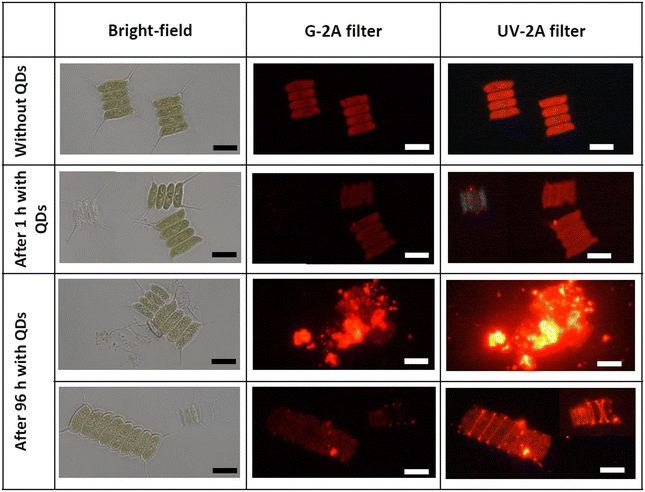 |
| Fig. 2 The bright field and fluorescence images of D. communis without QDs, after 1 hour and after 96 hour incubation with QDs (4 nM) in DWW and MWC media. UV-2A filter (an excitation range λex = 330–380 nm, a dichroic mirror (DM) 400 nm, a barrier filter (BA) 420 nm) and G-2A filter (λex = 510–560 nm, DM 575 nm, BA 590 nm). The scale bar is 10 μm. | |
The AF spectra in the MWC and DWW media had the main peak at 683 nm and the shoulder at about 740 nm (Fig. 3). The higher AF intensity at 683 nm was excited with 480 nm light and was relatively higher in DWW than in MWC (Fig. S7†). The fluorescence spectrum of samples with algae and QDs consisted of both the AF of algae and the PL of QDs (Fig. S8a and b†). The PL spectra of QDs in the corresponding media without algae were normalized at 650 nm (Fig. S8c and d†) and subtracted from normalized spectra of algae with QDs (Fig. 3c and d). An increase in the AF intensity of samples was recorded in both media over 96 hours, regardless of the presence of QDs. However, the relative intensity of the AF spectral bands changed over time. Thus, in the MWC medium without QDs, the peak at 683 nm apparently did not increase between 48 and 72 hours, but an increase was at about 720 nm (Fig. 3b). The opposite tendency was observed in the MWC medium with QDs between 72 and 96 hours (Fig. 3d). The spectral properties of the AF of algae with QDs (4 nM, Fig. 3) in both media (DWW and MWC) did not differ markedly from the corresponding samples without QDs on each day of measurements. However, the changes in the ratio of AF intensities of algae samples measured at 683 nm and 720 nm showed bifurcation of behaviour in two different media from the second day, regardless of the presence of QDs (Fig. 4). It is noteworthy that the relative increase in AF intensity in the spectral region of 720 nm was more pronounced in MWC compared to that in DWW. After four days the ratio of intensities 683/720 nm became larger than on the initial day for the samples in the DWW media (from 7 to about 9) and decreased for the samples in MWC (from 7 to about 5). Apparently, the changes in ratio were caused by cessation of AF intensity growth at 720 nm in DWW after 48 hours (Fig. S9†).
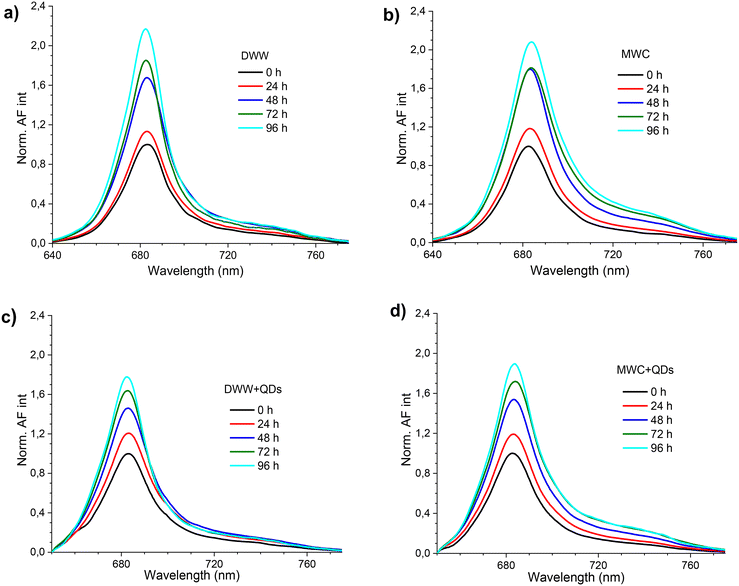 |
| Fig. 3 The representative normalized autofluorescence intensity (Norm. AF int) spectra of D. communis (initial concentration ∼5 × 105 cells per mL) in DWW (a), in MWC (b), in DWW with 4 nM QDs (c) and in MWC with 4 nM QDs (d) over 96 hours under white light (34 μmol photons per m2 s). Excitation was at 480 nm. The excitation and emission slits were 7 nm. | |
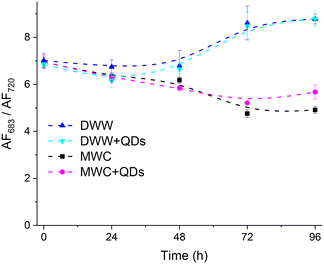 |
| Fig. 4 The changes of autofluorescence (AF) intensities ratio at 683 nm and 720 nm band of D. communis (initial concentration ∼5 × 105 cells per mL) in DWW and MWC media without and with QDs (4 nM) over 96 h under white light (34 μmol photons per m2 s). Excitation at 480 nm. The excitation and emission slits were 7 nm. N = 3, mean ± SD. The dashed line represents the variation trends, not the experimental results. | |
Thus, the AF properties of D. communis were influenced more by the type of growth medium (natural DWW and artificial MWC) than by the presence of QDs in it over 96 hours. Apparently, the composition of the environment, in which algae grow, affects the adaptive abilities of algal cells, which may be due to differences in the development of PSI and PSII and their contribution to the photosynthetic process. It has been shown39 that core/shell copper oxide NPs have a deleterious effect on chlorophyll a by inducing the photoinhibition of PSII in Chlamydomonas reinhardtii algae samples, resulting in inhibition of photosynthetic electron transport and a strong energy dissipation process via non-photochemical pathways. The observed lower AF683/AF720 ratio implies a higher PSI/PSII chlorophyll emission ratio for algae cells in MWC.
The effect of QDs on photosynthesis parameters of microalgae
Analysis of chlorophyll fluorescence induction curves measured with a PAM fluorometer (Fig. 5) allows the evaluation of the physiological state of PSII and components of a photosynthetic electron transport chain. Once in the dark or after adaptation to light conditions all PS II reaction centres are opened, a maximal photochemical quenching is reached, and the FL intensity is reduced.
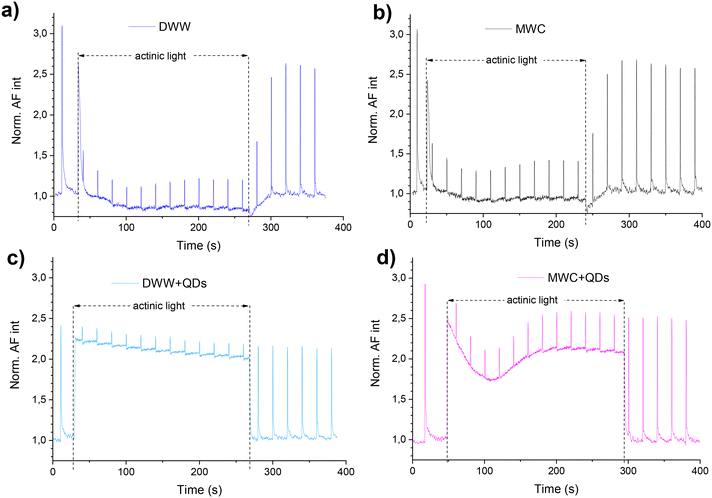 |
| Fig. 5 The representative D. communis (initial concentration ∼5 × 106 cells per mL) normalized autofluorescence intensity (Norm. AF int) induction and recovery curves in DWW (a and c) and MWC (b and d) media after 24 hour incubation without (a and b) and with QDs (40 nM) (c and d) under white light (34 μmol photons per m2 s). Dash lines show the actinic light period. | |
Although, under actinic light, the AF of D. communis in both media (DWW and MWC) decreased during the first 20 s (Fig. 5a and b), the decrease of AF of algae after incubation with QDs for 24 hours was reduced in both media (Fig. 5c and d). During the same time in actinic light, the decrease in AF was less than 15% (from 2.3 a.u. to about 2 a.u., Fig. 5c) in DWW. In the MWC + QDs medium, the AF intensity at first decreased faster than in the DWW + QDs when actinic light was turned on, but the AF intensity began to increase after 100 seconds, reaching a relative value of 2 a.u. (Fig. 5d). However, this increase of signal intensity registered in both algae samples (in DWW + QDs and MWC + QDs) was not induced by photoenhancement of the QDs PL. Due to the presence of aggregates in DWW, the PL intensity values measured from the bottom of a plate were higher than in MWC, where QDs did not precipitate during the 96 hours (Fig. S10†). The PL intensity of QDs decreased a little immediately after saturating pulses in DWW and slightly recovered in the dark (Fig. S10b†), but it did not react to actinic light. By measuring PAM, changes in photosynthetic processes can be determined, which helps explain the mechanisms by which QDs affect algae.
Several parameters related to a physiological state of photosynthesis were calculated from the induction and recovery curves of the algae AF measured in the DWW and MWC media after 24 hour incubation of algae samples without and with QDs (40 nM). During illumination intervals, the rate of photosynthetic electron transport (ETR) increased in all samples (Fig. 6a), but higher values were registered in the MWC medium, regardless of the presence of QDs. The exposure to QDs reduced the ETR values in algae by two times in MWC and even four times in DWW. The photochemical quantum yield of the photosystem II (Y(II)) estimates the photochemical use of excitation energy in the light, the value of which in the case of algae was 0.31 in MWC medium and about 0.26 in DWW (Fig. 6b). However, the QDs reduced the Y(II) values in both algae media to 0.17 and 0.07, respectively. The low value of Y(II) in DWW + QDs medium in weak light shows a strongly reduced activity of the Calvin–Benson cycle. The non-photochemical fluorescence quenching Y(NPQ) quantifies the fraction of excitation energy, which is dissipated as heat via photo-protective mechanisms. Due to an increased emission of excitation energy in light-acclimated algae samples, the quantum yield of the Y(NPQ) was decreased in algae samples with QDs compared to the samples in the same media without QDs (Fig. 6c). Being well-acclimated to dark conditions algae samples have all reaction centres in the open state, and non-photochemical dissipation of excitation energy is minimal. The yield of all other non-photochemical losses in dark-acclimated algae is given by the parameter Y(NO), which was about 0.3 in the MWC and DWW media (Fig. 6d). In the samples with QDs this parameter was higher, by almost three times – in DWW and by a little less – in the MWC media.
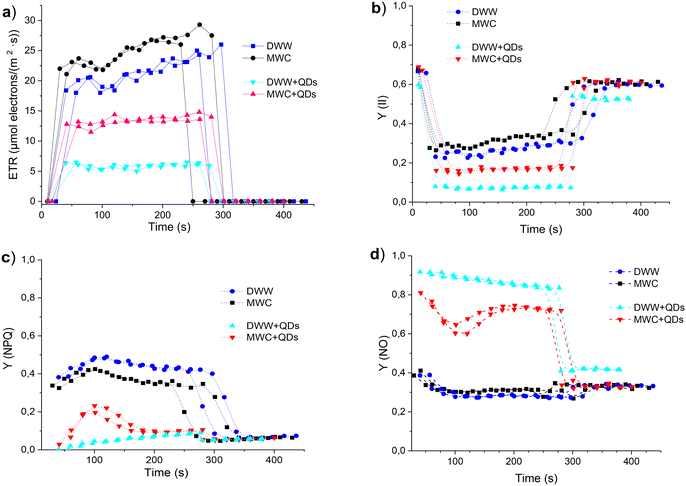 |
| Fig. 6 The photosynthetic electron transport rate (ETR) (a), photochemical quantum yield (Y) of photosystem II (b), and the kinetics of quantum yield of non-photochemical fluorescence quenching (NPQ) due to an increased emission of excitation energy in light (c) and quantum yield of non-regulated heat dissipation and fluorescence emission (NO) (d) of D. communis (initial concentration ∼5 × 106 cells per mL) in DWW and MWC after 24 hour incubation without and with QDs (40 nM) under white light (34 μmol photons per m2 s). The dashed line represents the variation trends, not the experimental results. | |
The studies on the autofluorescence of algae in the presence of less stable core QDs have revealed their ability to retard the photoadaptation of wild type microalgae.20 However, the presence of more stable core/shell QDs apparently affects photoadaptation of algae too, which was reflected in 3-fold higher value of Y(NO) in comparison with algae cells in the corresponding control media. Thus, while D. communis in the DWW medium had lower values of ETR, Y(II) and Y(NO) and higher values of Y(NPQ) than algae in the MWC medium, the changes in those values induced by the presence of QDs were stronger in the case of DWW. In turn, the growth medium not only differently affects the growth pattern, physiological processes and photoadaptation properties of D. communis algae, but also modifies their response to quantum dots.
Microalgae growth inhibition
The obtained results showed that the growth of the algae and the changes in their population structure significantly depended on the composition of the growth media (DWW, MWC and Balsys) (Fig. 7 and 8). The presence of QDs in the growth media affected the proliferation of D. communis to varying degrees (Fig. 7). Analysis of the cumulative effect of treatment and exposure duration in groups with QDs and without QDs revealed statistically significant differences in algal growth in both Balsys and MWC media (F(3.32) = 4.29, p < 0.001 and F(3.32) = 457.82, p < 0.001, respectively, according to two-way ANOVA). However, no significant differences were found in DWW (F(3.32) = 1.93, p = 0.144, two-way ANOVA). The inhibition of algal growth over 96 hours of exposure was markedly dependent on the medium type. The proliferation of algae in the MWC + QDs medium was significantly higher compared to the Balsys + QDs and DWW + QDs media (Fig. 7). Significant effects after 24 h were observed for D. communis when exposed to QDs in Lake Balsys water (p = 0.046) compared to this lake water without QDs. However, no further differences in proliferation of algae were identified with extended duration of exposure to QDs in Lake Balsys water (p > 0.05). Cell numbers in DWW medium did not change significantly during exposure to QDs compared to DWW without QDs (p > 0.05). The proliferation of D. communis was markedly decreased by QDs in MWC compared to the control, especially between 48 and 96 hours (in all cases p < 0.001). In most observed cases, there was an increase in algal growth with QDs exposure duration. For instance, the algae growth observed in MWC + QDs medium after 24 hours was notably different from that seen after 96 hours (p < 0.01).
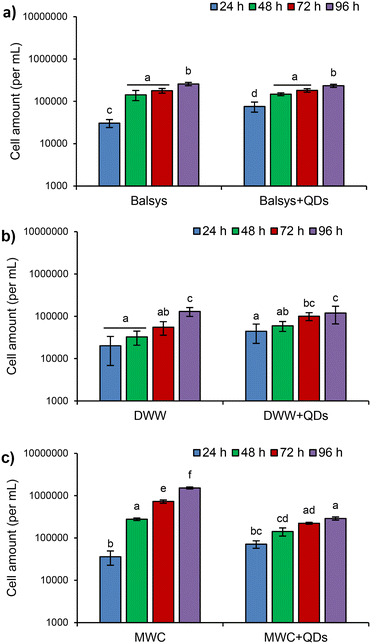 |
| Fig. 7 Cell amount (per 1 mL) of algae D. communis in different growth media (Balsys (a), DWW (b), MWC (c)) without and with QDs (4 nM) under white light (100 μmol photons per m2 s). The initial concentration of algae was ∼104 cells per mL. Different letters for data in each medium indicate significant differences between groups with QDs and without QDs after different exposure durations; identical letters in doublets indicate the absence of significant differences for the corresponding pair of groups (two-way ANOVA, p < 0.05, mean ± SD). | |
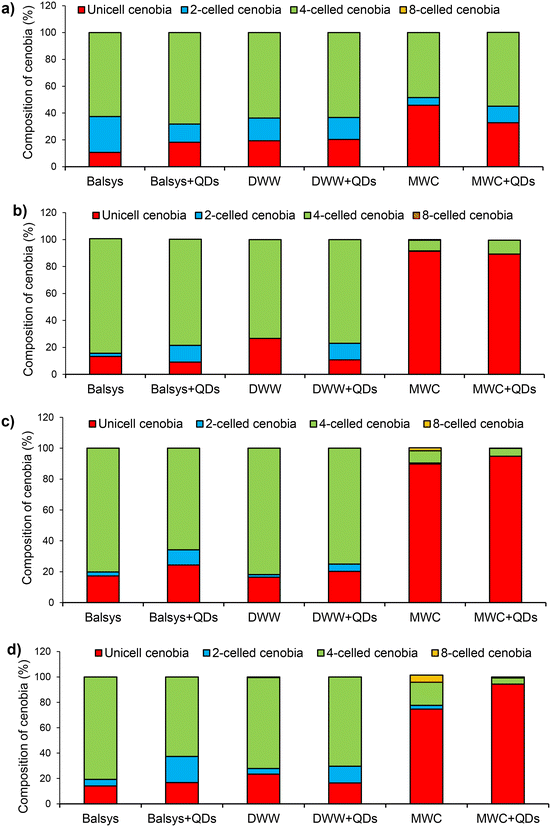 |
| Fig. 8 Structural composition of colonies after 24 h (a), 48 h (b), 72 h (c) and 96 h (d) of algae D. communis in different growth media (Balsys, DWW, MWC) without and with QDs (4 nM) under white light (100 μmol photons per m2 s). | |
The presence of QDs caused changes in the structure of D. communis algae colonies (cenobia) growing in different incubation media (Fig. 8). Rapid formation and predominance of unicellular algal colonies (after 48 h) were observed in MWC and MWC + QDs media. The number of unicellular colonies in these media reached 91% and 89%, respectively (Fig. 8b). The predominance of single-cell colonies was maintained throughout the entire incubation period. However, after 96 h of incubation, a significant increase in the number of four-cell and eight-cell colonies in the MWC medium was observed as compared to the MWC + QDs medium (Fig. 8d). It should be noted that the composition of the colonies in the algae population did not differ significantly in the water of Lake Balsys and DWW after 96 h of incubation. However, when QDs were added, differences in composition of the colonies were observed in both media (Fig. 8). The present study also investigated the influence of treatment with QDs and exposure duration on the Shannon–Wiener diversity index of algae growth in the Balsys, DWW, and MWC media (Fig. S11†). The results showed that different treatment groups and exposure duration had a significant effect on the Shannon–Wiener diversity index in the Balsys and MWC media (F(4.40) = 7.494, p < 0.001 and F(4.40) = 32.499, p < 0.002, respectively; two-way ANOVA), but not in DWW (F(4.40) = 2.232, p = 0.083, two-way ANOVA) (Fig. S11†).
According to Cornwallis et al.,40 multicellularity has allowed new levels of morphological complexity to develop, but it is still unclear what ecological advantages or benefits multicellularity provides. It has been suggested that possible benefits of forming multicellular groups as an adaptation are to protect unicellular organisms from various environmental stressors such as predation and toxins.40,41 Earlier studies examining the costs and benefits of group formation in algae have produced mixed results.41,42 According to Lürling et al.,42 multicelled colonies of algae have a higher sinking rate and lower ability to absorb nutrients compared to unicellular algae, but according to Cornwallis et al.,40 they can protect themselves from various stressors. Thus, the formation of four-celled colonies initiated by QDs in the population of D. communis in natural media may be associated with a protecting reaction of the algae to the effects of toxins. In our opinion, the predominance of single cells in the MWC and MWC + QDs media as well as the inability of the population in the MWC + QDs media to restore their pre-exposure diversity within 96 h showed that algae cannot adequately respond to stress factors when cultured in artificial media. Therefore, the results obtained using such media, as well as the conclusions and predictions made from these data, cannot be widely extrapolated to the aquatic environment. This study suggests that if the physical and chemical characteristics of freshwater media and NPs (e.g. core/shell, negative surface charge) are similar to those tested, then only in this case the fate of NPs and their effects on green algae will be comparable.
Most experimental findings in aquatic nanotoxicology are based on standardized laboratory studies. The extrapolation of these findings to multiple stress environments is complex and poses numerous questions.43,44 Our data suggest that nanotoxicity studies conducted under optimal, low-stress conditions in standardised laboratory tests can only serve as a reference, since they cannot provide sufficient information on the susceptibility of organisms to NPs in their natural habitat, where NPs, other pollutants, organisms and environmental factors act simultaneously and the interactions between them can be very complex. Translating toxicity data from the laboratory to the field appears to be a major challenge in nanoecotoxicology.
Conclusions
The joint application of optical and ecotoxicological methods to monitor changes induced in Desmodesmus communis, green freshwater microalgae, after exposure to CdSe/ZnS–COOH core/shell QDs revealed the complex effects on their growth, population structure, and photosynthetic activity. The PAM technique was the most sensitive in detecting differences between photosynthetic activity parameters in unaffected and QDs-affected algae after 24 h of incubation. After 48 hours, spectral changes in autofluorescence were recorded, indicating the effect of QDs on algae photosystems. However, the changes induced in the population structure and growth of algae appeared only after 72 h. The diverse impact of QDs on the photoadaptation ability of D. communis in different media was demonstrated by variation in their autofluorescence and photosynthetic parameters, this being closely related to PSII, with its extent depending on the composition of growth medium. The higher growth rate and photosynthetic efficiency, as well as the stronger effect of QDs on algae, that were all observed in the artificial medium could be related with higher prevalence of unicellular cenobia compared to the natural media, implying that young, rapidly growing and dividing algae were less resistant to a negative QDs impact. However, it should be taken into account that the stability of the optical properties of QDs depends, among other things, on the composition of the algae growth medium and the presence of algae. The stronger aggregation occurring in DWW, as opposed to MWC, may also be a contributing factor to the differences in the observed effects on the algae. Therefore, when assessing the effect of QDs on algae or other organisms, it is important to have experimental conditions as close as possible to natural ones. As this study showed, the actual response of microalgae depends on the complex nature of the interaction of QDs with living systems, which appears to be interrelated with the properties of quantum dots, as well as their stability, and with the type of algae, their growth conditions and the treatment used.
Author contributions
A. Kalnaitytė-Vengelienė, D. Montvydienė and S. Bagdonas: conceptualization, methodology, validation, investigation, formal analysis, writing – original draft, visualization, data curation. E. Januškaitė: validation, investigation, formal analysis. Ž. Jurgelėnė, M. Kazlauskas and N. Kazlauskienė: methodology, validation, investigation, formal analysis, visualization, writing – original draft.
Conflicts of interest
There are no conflicts to declare.
Acknowledgements
This work was funded by the Research Council of Lithuania, Project No. S-MIP-20-22. The authors are also grateful to Dr. Jūratė Karosienė and Dr. Judita Koreivienė from the Laboratory of Algology and Microbial Ecology at Nature Research Centre for help and advice during the experiments.
References
- J. Zhou, Y. Yang and C.-Y. Zhang, Toward Biocompatible Semiconductor Quantum Dots: From Biosynthesis and Bioconjugation to Biomedical Application, Chem. Rev., 2015, 115, 11669–11717 CrossRef CAS PubMed
.
- G. W. Walker, V. C. Sundar, C. M. Rudzinski, A. W. Wun, M. G. Bawendi and D. G. Nocera, Quantum-dot optical temperature probes, Appl. Phys. Lett., 2003, 83, 3555–3557 CrossRef CAS
.
- A. Mandal and N. Tamai, Influence of acid on luminescence properties of Thioglycolic acid-capped CdTe quantum dots, J. Phys. Chem. C, 2008, 112, 8244–8250 CrossRef CAS
.
- Y. F. Chen and Z. Rosenzweig, Luminescent CdS quantum dots as selective ion probes, Anal. Chem., 2002, 74, 5132–5133 CrossRef CAS PubMed
.
- W. Zhong, J. Liang and J. Yu, Systematic study of the interaction of cobalt ions with different-sized CdTe quantum dots, Spectrochim. Acta, Part A, 2009, 74, 603–606 CrossRef PubMed
.
- C. Niu, Z. Yao and S. Jiang, Synthesis and application of quantum dots in detection of environmental contaminants in food: A comprehensive review, Sci. Total Environ., 2023, 882, 163565, DOI:10.1016/j.scitotenv.2023.163565
.
- E. Oh, R. Liu, A. Nel, K. B. Gemill, M. Bilal, Y. Cohen and I. L. Medintz, Meta-analysis of cellular toxicity for cadmium-containing quantum dots, Nat. Nanotechnol., 2016, 11, 479–486 CrossRef CAS PubMed
.
- A. Hoshino, K. Fujioka, T. Oku, M. Suga, Y. F. Sasaki, T. Ohta, M. Yasuhara, K. Suzuki and K. Yamamoto, Physicochemical properties and cellular toxicity of nanocrystal quantum dots depend on their surface modification, Nano Lett., 2004, 4, 2163–2169 CrossRef CAS
.
- M. Giroux, Z. Zahra, O. A. Salawu, R. M. Burgess, K. T. Ho and A. S. Adeleye, Assessing the Environmental Effects Related to Quantum Dot Structure, Function, Synthesis and Exposure, Environ. Sci.: Nano, 2022, 9, 867–910 RSC
.
- A. H. Abdellatif, M. A. Younis, M. Alsharidah, O. Al Rugaie and H. M. Tawfeek, Biomedical Applications of Quantum Dots: Overview, Challenges, and Clinical Potential, Int. J. Nanomed., 2022, 17, 1951–1970 CrossRef PubMed
.
- H. E. Elzorkany, M. A. Farghali, M. A. Hassan, K. El-Sayed, M. Canonico, G. Konert, K. Farroh, H. A. Elshoky and R. Kaňa, Ecotoxicology impact of silica-coated CdSe/ZnS quantum dots internalized in Chlamydomonas reinhardtii algal cells, Sci. Total Environ., 2019, 666, 480–489 CrossRef CAS PubMed
.
- J. Liu, C. Yang, J. Liu, R. Hu, Y. Hu, H. Chen, W. C. Law, M. T. Swihart, L. Ye, K. Wang and K. T. Yong, Effects of Cd-based Quantum Dot Exposure on the Reproduction and Offspring of Kunming Mice over Multiple Generations, Nanotheranostics, 2017, 1, 23–37 CrossRef PubMed
.
- C. Zhu, Z. Chen, S. Gao, B. L. Goh, I. B. Samsudin, K. W. Lwe, Y. Wu, C. Wu and X. Su, Recent advances in non-toxic quantum dots and their biomedical applications, Prog. Nat. Sci.: Mater. Int., 2019, 29, 628–640 CrossRef CAS
.
- L. Ren, L. Wang, M. Rehberg, T. Stoeger, J. Zhang and S. Chen, Applications and Immunological Effects of Quantum Dots on Respiratory System, Front. Immunol., 2021, 12, 795232, DOI:10.3389/fimmu.2021.795232
.
- B. P. Jackson, D. Bugge, J. F. Ranville and C. Y. Chen, Bioavailability, toxicity, and bioaccumulation of quantum dot nanoparticles to the amphipod Leptocheirus plumulosus, Environ. Sci. Technol., 2012, 46, 5550–5556 CrossRef CAS
.
- A. Xiao, C. Wang, J. Chen, R. Guo, Z. Yan and J. Chen, Carbon and metal quantum dots toxicity on the microalgae Chlorella pyrenoidosa, Ecotoxicol. Environ. Saf., 2016, 133, 211–217, DOI:10.1016/j.ecoenv.2016.07.026
.
- H. Hu, Y. Deng, Y. Fan, P. Zhang, H. Sun, Z. Gan, H. Zhu and Y. Yao, Effects of artificial sweeteners on metal bioconcentration and toxicity on a green algae Scenedesmus obliquus, Chemosphere, 2016, 150, 285–293 CrossRef CAS PubMed
.
- I. V. Grigoriev, R. D. Hayes, S. Calhoun, B. Kamel, A. Wang, S. Ahrendt, S. Dusheyko, R. Nikitin, S. J. Mondo, I. Salamov and A. Kuo, PhycoCosm, a comparative algal genomics resource, Nucleic Acids Res., 2021, 49, D1004–D1011 CrossRef CAS PubMed
.
- F. R. Chen, Z. G. Xiao, L. Yue, J. Wang, Y. Feng, X. S. Zhu, Z. Y. Wang and B. S. Xing, Algae response to engineered nanoparticles: Current understanding mechanisms and implications, Environ. Sci.: Nano, 2019, 6, 1026–1042 RSC
.
- A. Kalnaitytė and S. Bagdonas, Light-mediated effects of CdTe-MSA quantum dots on the autofluorescence of freshwater green microalgae: Spectroscopic studies, J. Photochem. Photobiol., B, 2019, 199, 111629, DOI:10.1016/j.jphotobiol.2019.111629
.
- H. L. Ou-Yang, X. Z. Kong, M. Lavoie, W. He, N. Qin, Q. S. He, B. Yang, R. Wang and F. L. Xu, Photosynthetic and cellular toxicity of cadmium in Chlorella Vulgaris, Environ. Toxicol. Chem., 2013, 32, 2762–2770 CrossRef CAS PubMed
.
- R. C. Gebara, L. O. G. Alho, A. D. S. Mansano, G. S. Rocha and M. D. G. G. Melão, Single and combined effects of Zn and Al on photosystem II of the green microalgae Raphidocelis subcapitata assessed by pulse-amplitude modulated (PAM) fluorometry, Aquat. Toxicol., 2023, 254, 106369, DOI:10.1016/j.aquatox.2022.106369
.
- K. S. Kumar, H. U. Dahms, J. S. Lee, H. C. Kim, W. C. Lee and K. H. Shin, Algal Photosynthetic responses to toxic metals and herbicides assessed by chlorophyll a fluorescence, Ecotoxicol. Environ. Saf., 2014, 104, 51–71 CrossRef
.
- D. Chakraborty, K. R. Ethiraj, N. Chandrasekaran and A. Mukherjee, Mitigating the toxic effects of CdSe quantum dots towards freshwater alga Scenedesmus obliquus: Role of eco-corona, Environ. Pollut., 2021, 270, 116049, DOI:10.1016/j.envpol.2020.116049
.
- S. J. Zhang, Y. Jiang, C. S. Chen, J. Spurgin, K. A. Schwehr, A. Quigg, W. C. Chin and P. H. Santschi, Aggregation and dissolution of quantum dots in marine environments: Importance of extracellular polymeric substances, Environ. Sci. Technol., 2012, 46, 8764–8772 CrossRef CAS PubMed
.
- A. Quigg, W. C. Chin, C. S. Chen, S. Zhang, Y. Jiang, A. J. Miao, K. A. Schwehr, X. Chen and P. H. Santschi, Direct and Indirect Toxic Effects of Engineered Nanoparticles on Algae: Role of Natural Organic Matter, ACS Sustainable Chem. Eng., 2013, 1, 686–702 CrossRef CAS
.
- R. Hardman, A toxicologic review of quantum dots: toxicity depends on physicochemical and environmental factors, Environ. Health Perspect., 2006, 114, 165–172 CrossRef PubMed
.
- E. Morelli, P. Cionia, M. Posarelli and E. Gabellieri, Chemical stability of CdSe quantum dots in seawater and their effects on a marine microalga, Aquat. Toxicol., 2012, 122–123, 153–162 CrossRef CAS PubMed
.
- S. Lin, P. Bhattacharya, N. C. Rajapakse, D. E. Brune and P. Chun Ke, Effects of quantum dots adsorption on algal photosynthesis, J. Phys. Chem. C, 2009, 113, 10962–10966 CrossRef CAS
.
- E. Morelli, E. Salvadori, R. Bizzarri, P. Cioni and E. Gabellieri, Interaction of CdSe/ZnS quantum dots with the marine diatom Phaeodactylum tricornutum and the green alga Dunaliella tertiolecta: a biophysical approach, Biophys. Chem., 2013, 182, 4–10 CrossRef CAS PubMed
.
- Description of MWC algae grow medium, https://norcca.scrol.net/node/3959 (accessed October 2022).
- J. Koreivienė, J. Kasperovičienė, K. Savadova, J. Karosienė and I. Vitonytė, Collection of pure cultures of algae and cyanobacteria for research, teaching and biotechnological applications (Nature Research Centre, Lithuania), Bot. Lith., 2016, 22, 87–92 Search PubMed
.
-
OECD, Freshwater alga and cyanobacteria, growth inhibition test No. 201, Guidelines for the Testing Chemicals, 2011, vol. 25, DOI:10.1787/9789264069923-en.
- X. S. Liu and J. M. Wang, Algae (Raphidocelis subcapitata) mitigate combined toxicity of microplastic and lead on Ceriodaphnia dubia, Front. Environ. Sci. Eng., 2020, 14, 97, DOI:10.1007/s11783-020-1276-3
.
- A. Kalnaitytė, S. Bagdonas and R. Rotomskis, The dose-dependent photobleaching of CdTe quantum dots in aqueous media, J. Lumin., 2018, 201, 434–441 CrossRef
.
- X. S. Liu, J. M. Wang and Y. W. Huang, Understanding the role of nano-TiO2 on the toxicity of Pb on C. dubia through modeling—Is it additive or synergistic?, Front. Environ. Sci. Eng., 2022, 16, 59, DOI:10.1007/s11783-021-1493-4
.
- M. Mayer-Pinto, J. Ledet, T. P. Crowe and E. L. Johnston, Sublethal effects of contaminants on marine habitat-forming species: a review and meta-analysis, Biol. Rev., 2020, 95, 1554–1573 CrossRef PubMed
.
- J. Farkas and A. M. Booth, Are fluorescence-based chlorophyll quantification methods suitable for algae toxicity assessment of carbon nanomaterials?, Nanotoxicology, 2017, 11, 569–577 CrossRef CAS PubMed
.
- C. Saison, F. Perreault, J. C. Daigle, C. Fortin, J. Claverie, M. Morin and R. Popovic, Effect of core-shell copper oxide nanoparticles on cell culture morphology and photosynthesis (photosystem II energy distribution) in the green alga, Chlamydomonas reinhardtii, Aquat. Toxicol., 2010, 96, 109–114 CrossRef CAS PubMed
.
- C. K. Cornwallis, M. Svensson-Coelho, M. Lindh, Q. Li, F. Stabile, L. A. Hansson and K. Rengefors, Single-cell adaptations shape evolutionary transitions to multicellularity in green algae, Nat. Ecol. Evol., 2023, 7, 889–902 CrossRef PubMed
.
- S. E. Kapsetaki and S. A. West, The costs and benefits of multicellular group formation in algae, Evolution, 2019, 73, 1320–1321 CrossRef PubMed
.
- M. Lürling and E. Van Donk, Grazer-induced colony formation in Scenedesmus: are there costs to being colonial?, Oikos, 2000, 88, 111–118 CrossRef
.
- R. Hjorth, L. M. Skjolding, S. N. Sørensen and A. Baun, Regulatory adequacy of aquatic ecotoxicity testing of nanomaterials, NanoImpact, 2017, 8, 28–37 CrossRef
.
- Ž. Jurgelėnė, D. Montvydienė, S. Semčuk, M. Stankevičiūtė, G. Sauliutė, J. Pažusienė, A. Morkvėnas, R. Butrimienė, K. Jokšas, V. Pakštas, N. Kazlauskienė and V. Karabanovas, The impact of co-treatment with graphene oxide and metal mixture on Salmo trutta at early development stages: The sorption capacity and potential toxicity, Sci. Total Environ., 2022, 838, 156525, DOI:10.1016/j.scitotenv.2022.156525
.
|
This journal is © The Royal Society of Chemistry 2024 |
Click here to see how this site uses Cookies. View our privacy policy here.