DOI:
10.1039/C5AN02208H
(Minireview)
Analyst, 2016,
141, 1185-1195
Electrochemical hydrogen sulfide biosensors
Received
25th October 2015
, Accepted 8th January 2016
First published on 11th January 2016
Abstract
The measurement of sulfide, especially hydrogen sulfide, has held the attention of the analytical community due to its unique physiological and pathophysiological roles in biological systems. Electrochemical detection offers a rapid, highly sensitive, affordable, simple, and real-time technique to measure hydrogen sulfide concentration, which has been a well-documented and reliable method. This review details up-to-date research on the electrochemical detection of hydrogen sulfide (ion selective electrodes, polarographic hydrogen sulfide sensors, etc.) in biological samples for potential therapeutic use.
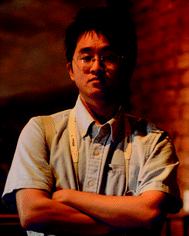 Tailin Xu | Tailin Xu received his BSc degree in Chemistry at the School of Chemistry and Biological Engineering, University of Science & Technology Beijing (USTB, P. R. China). He then joined the Research Center for Bioengineering & Sensing Technology research group at USTB to pursue his PhD degree under the supervision of Prof. Xueji Zhang. From 2013 to 2015, he joined Joseph Wang's group as a joint PHD student at the University of California, San Diego. His research interests include electrochemical sensors, and functional nanomotors. |
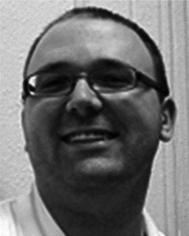 Nikki Scafa | Nikki Scafa is a research assistant at World Precision Instruments Inc. in Sarasota, FL. He graduated from the University of Florida with a bachelor's degree in chemical engineering. He performs quality control testing on sensors in the area of biosensing and tests new and prototype sensors for research applications. In the six years that he has been there, he has performed research testing to facilitate the release of sensors that measure different chemical species, including nitric oxide, hydrogen peroxide, hydrogen sulfide, and oxygen. |
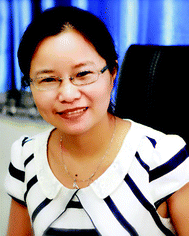 Li-Ping Xu | Li-Ping Xu is an associate professor of chemistry at the Research Center for Bioengineering and Sensing Technology, University of Science & Technology Beijing. She got her PhD degree in physical chemistry from the Institute of Chemistry, Chinese Academy of Science in July 2006. She was a postdoc fellow at the University of California, Santa Cruz from 2006–2007. Her research interests include nanomaterials, functional interfacial materials, electrochemical biosensors, and supramolecular assembly. |
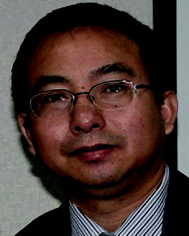 Shufeng Zhou | Shufeng Zhou is presently the Associate Vice President of Global Medical Development & Associate Dean of International Research, College of Pharmacy, University of South Florida, Tampa (USF), Florida. He completed his clinical medical training in China in 1986 and obtained his PhD in 2001 from the School of Medicine, the University of Auckland, New Zealand. Dr Zhou's major research interests entail systems pharmacology, nanomedicine, pharmacokinetics, and pharmacogenomics. He has published more than 350 peer-reviewed journal papers, 18 books and book chapters. His work has been cited more than 10 000 times by other colleagues. |
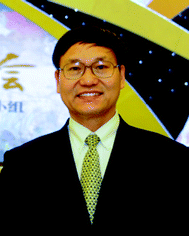 Xueji Zhang | Xueji Zhang is the national chair professor & director of the Research Center for Bioengineering and Sensing Technology, University of Science & Technology, Beijing, China. He obtained his Ph.D. from Wuhan University in 1994. He was a postdoc fellow at the National Institute of Chemistry, Slovenia, Swiss Federal Institute of Technology (ETH), Zurich, and New Mexico State University from 1995–1999. He has worked at World Precision Instruments Inc. USA as a Sr scientist, head of chemistry department, and vice president and Sr vice president of science respectively since 1998. His research has centered on electrochemistry, bioanalysis, micro/nanosensors, and biomedical instrumentation. |
1. Introduction
Hydrogen sulfide (H2S) is well known as a colorless, flammable, and water soluble gas with the distinctive smell of rotten eggs and has received attention as a toxic gas and as an environmental hazard for many decades.1 Recent research has found that H2S is a common constituent of mammalian tissues and cells, notably in the brain, heart, gastrointestinal tract, vascular smooth muscles, and endothelial cells, acting as a messenger much like nitric oxide (NO) and carbon monoxide (CO).2–5 Its important roles in mammalian systems prompt us to explore a fast and sensitive detection method to monitor its concentration in biological samples for fully understanding the effects of its possible physiological and pathophysiological roles, as well as its potential therapeutic use.6–8 Due to the widespread interest in its biological functions, detection methods for sulfide have evolved from simple colorimetric assays to the more recently used techniques, including high pressure liquid chromatography (HPLC) and gas chromatography (GC),9–11 monobromobimane (MBB),12–15 fluorescence probe,8 and electrochemical sensors (ion sensitive electrodes and polarographic H2S sensors, etc.).16,17 Certainly, each technique has its advantages and limitations. Chromatography based methods, which have been used to measure both plasma and tissue H2S sensitivity, however, do not have the capability of real-time measuring in biological samples and require hypoxic or anoxic conditions.10,11,18 In a typical MBB assay, H2S reacts with two molecules of monobromobimane to form the fluorescent sulfide-dibimane, followed by HPLC assay with fluorescence detection. This method requires relatively expensive equipment, and its time consuming process results in unknown reactions in blood samples, thus interfering in the detection of sulfide and making real-time measurements of H2S impossible.12–15 The methylene-blue method is one of the most commonly used spectrometric methods for measuring plasma H2S, which involves the reaction of H2S with N,N-dimethyl-p-phenylenediamine sulfate to produce the light-absorbing methylene blue. Nevertheless, this direct method lacks sensitivity at low concentrations (<1 μM) and requires long incubation periods (>30 min), making it unsuitable for continuous measurement of H2S under real-time, physiological conditions.19,20 Some other spectroscopic methods offer high sensitivity and selectivity for H2S.21–25 For example, it is noteworthy that the most recently developed sulfide-sensitive fluorescent probes enable H2S measurement in cell and blood samples at low concentrations.14,26 In addition, the greatest limitations for fluorescent indicators (i.e. response time and sample damage) leave significant room for improvement in the future.
Chemical and biological methods with several advantages have become one of the most active areas of analytical research in recent years. Electrochemical biosensors, as one of the most common methods, have been widely used to detect biomolecules such as glucose,27 nitric oxide,28,29 ascorbic acid,30 uric acid,31 dopamine,32 and hydrogen peroxide.33 Electrochemical biosensing is also an effective method to measure the H2S concentration in different kinds of samples with distinct advantages of high sensitivity, high selectivity, low-detection limit, stability, long lifetime, wide linear range, fast response time, biocompatibility, reproducibility, and simple operation.18,34–37 These advantages have attracted a number of researchers to develop new electrochemical methods for determining sulfide species in inorganic and organic forms.18,34–37 The present review has made an effort to detail the most recent research on ion sensitive electrodes, polarographic H2S sensors, and enzyme based electrodes for H2S concentration detection in biological samples.
2. Background perspective of H2S
2.1 H2S in chemistry
Hydrogen sulfide is a weak acid with pKa1 = 6.6, and pKa2 = 13.8 at 25 °C.38 H2S aqueous solution is a mixture of H2S, HS−, S2− and dissociates (H2S ↔ H+ + HS− ↔ 2H+ + S2−). However, there is only a trace of S2− in normal biological samples (pH ∼ 7) because pKa2 is higher than 13. H2S is strongly harmful, toxic, and flammable when released into the atmosphere either as a pollutant from the chemical industry or as a by-product of biological activity. The electric potential between H2S and O2 is really high, making it extremely favorable for oxidation reactions, so H2S can be oxidized to sulfur dioxide (SO2), sulfates, and elemental sulfur. Chemical industry oxidation (H2S + 2O2 → SO42− + 2H+) which is catalyzed by metals or metal oxides, is one of the main sources of acid rain. Moreover, H2S is also harmful in industrial production: fabrication of fuel cells requires the control of H2S at ppb levels in order to extend the lifetime of the proton exchange membrane, while in the food industry, H2S needs to be monitored at a trace level to guarantee the quality of products.
2.2 H2S function in a biological system
Recent studies have established that H2S is an important signalling molecule of comparable importance to NO and CO in mammalian tissues and cells. The production of H2S can occur via two pathways: enzymatic and non-enzymatic. Much emphasis has been placed on the enzymatic production of H2S, which is formed from cysteine by H2S synthases, namely cystathionine β-synthase (CBS), cystathionine γ-lyase (CSE) and 3-mercaptopyruvate sulfurtransferase (MST).39 Non-enzymatic production of H2S occurs through glutathione, inorganic and organic polysulfides, and elemental sulfur.18,39 Apart from the free state, H2S can react with several different biochemical molecules to establish different bio-available pools, such as acid labile sulfides (Fe–S complexes and persulfides) and bound sulfide forms (polythionates, polysulfides, thiosulfate, thiosulfonates, bisorganylpolysulfanes, monoarylthio-sulfonates, and elemental sulfur). However, less is known about the catabolism of H2S in the body. One possible catabolic pathway is the rapid oxidation to thiosulfate (S2O32−) by mitochondria and subsequent conversion to the stable product sulfate (SO42−) in cells.40 H2S is more soluble in lipids than in water, which makes it easier to travel into and out of cell membranes. As one of the messenger molecules throughout the body, H2S can modulate the neuronal transmission, can relax smooth muscles, and is involved in inflammation together with NO and CO.7 Several cardiovascular diseases are also related to its function in cardiovascular physiology and pathophysiology.41 Potential therapeutic uses in ischemia/reperfusion (I/R) injury have been examined extensively in the heart, brain, lungs, and liver.42,43 H2S produces anti-inflammatory effects in neurocytes, which may contribute to atherosclerosis by various mechanisms depending on the disease stage.44 Whereas H2S is toxic to the human body at high levels; it may cause unconsciousness and death in humans at concentrations above 500 ppm.45
2.3 Electrochemical oxidation of H2S
A typical three-electrode configuration, consisting of a working electrode, a reference electrode, and a counter electrode, is employed to detect H2S concentration from different kinds of sources. Hydrogen sulfide can be oxidized at the working electrode with the transfer of two electrons (H2S = S + 2H+ + 2e−E0 = 0.171 V vs. Ag/AgCl) and produce a current. With the transfer of more electrons, it can be further oxidized into sulfite (SO32−) and sulfate (SO42−).46 Electrochemical H2S sensors can be divided into liquid and solid electrolyte sensors based on its electrolytes. Based on their working principles, these sensors can be further divided into amperometric and potentiometric,37 which are two of the most common techniques employed to detect H2S concentration in biological samples. Specifically, an amperometric method can monitor the very low redox current (e.g. pA's) produced by the oxidation of H2S over time at a fixed (poise) voltage potential. This method has a fast response time of only a few seconds, high sensitivity, and provides the quantitative measurement of minute changes in H2S concentration.
3. Electrochemical detection of H2S
Electrochemical sensors have several advantages that make them an attractive method for real-time detection of H2S in biological samples due to their high sensitivity, high selectivity, low detection limit, fast response time, miniaturization capabilities, and the fact that they do not require additional chemical reagents. Solid-electrolyte sensors (such as solid-polymer electrolyte (SPE)-Pt and metal oxide based sensor) are commonly applied for quantitative analysis of hydrogen sulfide gas in the chemical industry.47–49 Ion sensitive electrodes (ISEs) and amperometric (polarographic) H2S sensors (PHSSs) are two well-known devices that have been applied to detect H2S concentration in biological samples.
3.1. Ion sensitive electrodes (ISEs) for H2S detection
Ion sensitive based electrodes are one of the most commonly used methods to measure concentrations of H2S in plasma with a detection range of 1–100 μM. This method is easy to obtain and operate. It is sensitive towards sulfide anions and allows for dynamic detection of sulfide concentration within one minute. Common Ag/Ag2S ISEs are simply prepared by dipping a silver wire into saturated sodium sulfide solution. The silver wire slowly reacts with the S2− ions, forming a thin and dark layer of Ag2S on the surface of the silver wire. The Ag2S layer can serve as a protection membrane preventing the interference of the samples. Carefully checking the electrode surface is necessary to make sure that the thin layer of Ag2S without silver is exposed to the surface. Otherwise, there will be a loss of selectivity due to the silver portion of the electrode being responsive to redox changes in the solution.50 This method was first reported in plasma H2S concentration measurement by Mason et al., but without the details of the procedure.51 Khan et al. made a full detailed procedure of plasma H2S concentration measurement.52 In brief, sulfide antioxidant buffer was added into blood samples at a ratio of 1
:
1. The electrode was rinsed with distilled water and then placed in sample solutions. Next, the value was recorded when a stable reading was displayed. Finally, H2S concentration was calculated by the standard S2− solution.52 Based on this method, commercial sulfide sensitive electrodes (Model 9616, Orion Research, Beverly, MA) were widely used in serum H2S concentration detection.53 Another commercial ISE, known as ArrowH2S™ developed by Lazar Research Laboratories (Los Angeles, CA), is a small ion electrode. This sensor can measure volumes down to 10 microliters with a one-step sample prep-performance directly in the micro container, which obviously prevented H2S loss from the sample and increased accuracy. In addition, unlike other methods, this micro-electrode measurement method has no known interferents and has a detection limit as low as 100 nM.54 A variety of applications of the ISE-based H2S sensor have been listed in Table 1. However, using Ag/Ag2S electrodes detection of H2S has several problems, such as the requirement of an alkaline environment favoring the S2− equilibrium, operating in a low concentration range, and maintaining the connection between the electrolyte bridge and the reference electrode.18,36 Whitfield et al. have demonstrated that S2− levels of trout plasma and BSA placed in alkaline buffer increased by 1 mM over a period of 12 hours, which makes its application to biological samples questionable and requires consideration of strong chemical pretreatment of samples.18,55,56 Another disadvantage of this method is the requirement for the electrode to be reconditioned and calibrated daily due to formation of Ag2S on the electrode surface.
Table 1 Measurement of the concentration of H2S by using an ISE in biological samples
Standard |
Plasma or blood H2S concentration |
Samples |
Applications |
Ref. |
Na2S |
10 |
Rat blood |
Sulfide oxidation |
51
|
Na2S |
2 |
Ewes and steers blood |
|
52
|
NaHS |
|
Rat blood |
KATP channel |
53
|
NaHS |
38 |
Trout plasma |
Smooth muscle |
57
|
H2S |
34 |
Human plasma |
Chronic obstructive pulmonary disease |
58
|
S2− |
51.7 |
Human plasma |
Coronary heart disease |
59
|
NaHS |
47 |
Rat plasma |
KATP channels |
60
|
S2− |
65.7 |
Children plasma |
Hypertension |
61
|
H2S |
39.7 |
Rat plasma |
Balloon injury |
62
|
S2− |
46 |
Rat plasma |
L-Arginine/NO pathway |
63
|
H2S |
21 |
Rat plasma |
Lung injury |
64
|
Na2S |
23.8 |
Rat plasma |
Metabolic function |
65
|
NaHS |
40 |
Rat plasma |
Hypertension |
54
|
NaHS |
90 |
Rainbow trout plasma |
Adrenergic stress response |
66
|
NaHS |
57.7 |
Rat plasma |
Atherosclerotic lesions |
44
|
H2S |
|
Rat plasma |
Pancreatic insulin metabolism |
67
|
NaHS or H2S |
|
Human plasma |
Respiratory infections |
68
|
NaHS or H2S |
30.8 |
Rat plasma |
Airway inflammation |
69
|
NaHS |
|
Rat plasma |
S-sulfhydration |
70
|
NaHS |
1.75 nM mg−1 |
Rat cellular H2S |
Methylglyoxal function |
71
|
NaHS |
|
Leaf supernatant |
Gasotransmitter |
72
|
NaHS |
|
Leaf supernatant |
Autophagy, and transcriptional modulator |
73
|
|
|
Rat liver |
Dietary restriction |
74
|
3.2. Polarographic H2S sensors (PHSSs)
Although it is clear that H2S plays an important physiological role in mammalian systems, the effects of H2S under physiological conditions remain largely undefined without the benefit of real-time H2S measurements. An amperometric H2S sensor offers a reliable real-time method and has shown promise in detecting the concentration of H2S in whole blood and mammalian tissues, without altering the sample as summarized in Table 2.36 This was first developed by Jeroschewski et al., based on a standard Clark-type oxygen electrode, and used for H2S measurement in aquatic environments with a detection limit as low as 2 μM.75 Kraus and Doeller modified the polarographic oxygen sensor, enabling it for the real-time measurement of H2S gas from biological samples with a lower detection limit in the nM range under anoxic conditions.76 In short, macro PHSSs (L = 25 mm, D = 12 mm) were designed originally from a polarographic oxygen sensor (POS, model 2120, Orbisphere, Geneva, Switzerland) with platinum wires (1 and 0.5 mm diameter) as the anode and cathode, respectively. The PHSS housing was machined from polyether ether ketone (PEEK). The electrolyte was held in the sensor tip reservoir with a two-layer membrane made of an H2S-permeable membrane (MEM-213, 25 μm thick, WPI, Inc.), cemented (Silicone Adhesive RTV 167, GE, Waterford, NY) to a 25 μm thick H2S-impermeable membrane. The H2S-permeable membrane allows the permeation of H2S through a hole (D = 0.5 mm) into an alkaline K3Fe(CN)6 internal electrolyte solution. H2S was dissociated into HS− after crossing the permeable membrane and then chemically oxidized to S0 while simultaneously reducing Fe(CN)63− to Fe(CN)64−. The Fe(CN)64− is then electrochemically oxidized back to Fe(CN)63− (0.1–0.2 V vs. SCE) on the surface of the platinum electrode, producing a current proportional to H2S concentration in biological samples. Kraus's group developed another miniature PHSS based on the original design of the macro one. This miniature PHSS utilizes a cylindrical platinum anode that was electrically insulated along the side from a coiled platinum cathode. The core was housed in a 2 mm outside diameter stainless steel cylindrical sleeve and a 25 μm thick H2S-permeable membrane to cover the tip. The reservoir between the sleeve and core was filled with the ferricyanide electrolyte solution. The end of the sensor, contained within the sleeve (L = 50 mm), was fitted into a port of a PEEK stopper (contained two sensor ports and an injection port). Whitfield et al. made further modifications of PHSS. In brief, this design of the PHSS consisted of a heat-sealed platinum wire and a platinum wire cathode coiled around the capillary tube. The whole assembly was then inserted into an outer glass case containing a solution of 50 mM potassium ferricyanide in pH 10, 0.5 M sodium carbonate buffer. An O-ring was seated in a groove in the outer glass case to hold in place a 25 μm thick silicone polycarbonate membrane (SSP-M213; Specialty Silicone Products, Inc., Ballston Spa NY). The 2 mm diameter PHSS (response time <5 s, detection limit <5 nM, sensitivity = 2 pA nM−1) described above is also available from World Precision Instruments (model ISO-H2S-2, Sarasota, FL, USA), which was connected to a multichannel Free Radical Analyzer (TBR4100,WPI Inc., Sarasota, FL, USA).34 Moreover, this company developed an ultra-micro PHSS (ISO-H2S-100-CXX, WPI, Inc., Sarasota, FL, USA) with a diameter of only 100μm, which can obviously reduce the damage of the samples and offers several advantages, including requiring no sleeves or filling solutions, durability for long-term use, rapid response time, and a bigger linear range. Compared to an ISE based H2S sensor, this type of sensor detects H2S without an external electrical potential, has a simple structure, good reproducibility, a short response time, and less containment of noble metals. However, a greater disadvantage of the liquid electrolyte sensors is that they leak easily, are prone to dry up, and have large residual current due to impurities in the solution.
Table 2 Measurement of the concentration of H2S by using a PHSS in biological samples
Mode |
Standard |
Sample |
Applications |
Related disease |
Ref. |
|
Na2S |
Mussel (gill tissue) |
Metabolic pathways |
|
76
|
ISO-H2S-2 |
Na2S |
Rat organ homogenate |
Sulfide production and consumption |
|
34
|
|
Na2S |
Human blood |
Consumption of garlic |
|
77
|
ISO-H2S-2 |
H2S |
Rat tissues |
Vasoactivity and O2 dependent |
|
78
|
ISO-H2S-2 |
H2S |
Mice |
Preservation of mitochondrial function |
Ischemia-reperfusion injury |
42
|
|
Na2S |
Cow, sea lions lung |
O2 dependent H2S metabolism |
Hypoxic pulmonary vasodilation |
79
|
ISO-H2S-2 |
|
RAW264.7 macrophages |
H2S donor (GYY4137) function |
Inflammatory |
80
|
NS-ISO-H2S PF100H |
|
Spinacia oleracea seedlings |
Modulating photosynthesis |
|
81
|
ISO-H2S-2 |
NaHS |
Diabetic rat plasma |
Reduce ROS formation |
Diabetic |
82
|
ISO-H2S-100 |
|
Peripheral blood lymphocytes |
AKT/GSK3β signal pathway |
Systemic lupus erythematosus |
83
|
ISO-H2S-2 |
|
Rat plasma |
S-Propargyl-Cysteine Formulation |
Heart failure |
84
|
ISO-H2S-100 |
NaHS |
Mitochondria (rat liver), mouse hepatoma cells |
Intramitochondrial H2S-producing pathway |
|
85
|
|
Na2S |
Rats tissue, Bovine lung and heart, sea lamprey |
Oxygen sensing |
|
86
|
ISO-H2S-2 |
|
Micelle solutions |
H2S delivery |
|
87
|
ISO-H2S-2 |
Na2S |
Extracellular H2S |
Up-regulate nicotinamide phosphoribosyltransferase |
Cancer cells recover |
88
|
ISO-H2S-100 |
Na2S |
Rat plasma |
KATP channel expression |
Hypertension |
89
|
ISO-H2S-2 |
Na2S |
Rats (serum, renal slice culture, renal tissue) |
CBS/H2S pathway |
Hypertension |
90
|
3.3 Enzyme based electrochemical H2S biosensors
Enzyme-based electrochemical biosensors have become more practical than other electrochemical detection methods due to their high sensitivity and selectivity in recent years. An enzyme-inhibition biosensor depends on both the concentration of the target analyte in the sample and the type of inhibited enzyme, which are commonly used for measurement of the concentration of inhibitory compounds.91 The common design of this type of H2S sensor involves an electrochemical transducer and an enzyme as the recognition element. Early in the 1990s, cytochrome oxidase-based inhibited enzyme electrodes were studied by researchers with a detection limit concentration of H2S down to 1 ppm in the gas phase. However, this electrode responds to other target analytes (HCN, and azide ion), making its application to biological samples questionable and developing a need for chemical pretreatment of samples.92 Horseradish peroxidase is stable and has a number of potential inhibitors in homogeneous solutions. Therefore, it has been widely used as an inhibited enzyme electrode by researchers for H2S detection. Colloidal gold-immobilized horseradish peroxidase for H2S detection has been reported by Zhao et al., but it seems more sensitive to cyanide and thiourea than sulfide. In addition, its detection limit is only 8 μM which is not enough for analyzing environmental samples.93 Yang et al. developed an amperometric horseradish peroxidase inhibition H2S biosensor based on a thiolate self-assembled monolayer (SAM), which has a much lower detection limit (0.3 μM). Nevertheless, the selectivity of SAM-based biosensors is poor for many common cations which greatly limits their application for analysis of environmental water.94 Based on the method Yang et al. developed, Liu et al. reported a multilayer film-modified biosensor via layer-by-layer (LBL) biospecific binding of concanavalin A and horseradish peroxidase on the surface of the gold electrode, which shows no interference of the common anions and cations in environmental water.95 Coprinus cinereus peroxidase immobilized on a screen printed electrode was reported by Savizi et al. This was used as an enzyme inhibition H2S biosensor which has a detection limit of 0.3 μm (linear response in the range of 1.09–16.3 μM) and a response time of 43 s.47 Another enzyme inhibition H2S biosensor, ascorbate oxidase, was immobilized on a Clark electrode and protected with a nylon membrane. This electrode can detect H2S at a low detection limit and prevent interference of main cations and anions.96 The enzyme based H2S biosensor mentioned above shows great advantages of selectivity and sensitivity. However, one of the most regrettable characteristics of the enzyme inhibition-based biosensors is the different inhibition degrees caused by different inhibitors. Moreover, this kind of sensor also highly depends on the pH concentration due to its inhibition on the activity of enzyme, making it difficult to apply to in vivo H2S detection.
4. Calibration of the H2S electrode
Calibration of an H2S sensor is important in order to ensure the exact experimental results. In general, the PHSS was calibrated with stepwise additions of H2S stock solution at the pH and temperature of the subsequent experiment. Detection of the H2S content in the sample was calculated from the calibration curve of current (pA) vs. concentration of H2S. H2S stock solution was freshly prepared by directly bubbling distilled water with pure H2S gas (Matheson, Newark, CA) to make the saturated H2S solution (0.09 M at 30 °C). The H2S stock solution was diluted to different concentrations into cell culture medium and the pH of the medium was adjusted to 7.4. Stock solutions of H2S can also be conveniently made with the salt, Na2S·9H2O (Sigma-Aldrich). This method avoids the need for a special gas-handling apparatus required for pure H2S gas, and the additional micromolar sodium, as Na2S, dissociates to form Na+ and the three sulfide species H2S, HS− and S2− in pH dependent ratios.76 The calibration of the ISE based H2S sensor should be carried out daily. Four 10 ml aliquots of 10 ppm S2− standard solution were pipetted into 50 ml beakers. Electrodes were immersed in the solution and then measurements were recorded once the reading became stable (approx. 1 min). Then, 1 ml 100 ppm S2− standard solution was immediately added and the second reading was recorded after l min. Finally, the resulting difference in the potential was used to calculate the exact concentration of the standardizing solution by applying the Nernst equation.52
5. Characterization of H2S electrodes
An excellent H2S electrochemical sensor can be characterized by high sensitivity, high selectivity, low-detection limit, stability, long lifetime, wide linear range, fast response time, biocompatibility, and reproducibility.17 The sensitivity of the electrochemical H2S sensor depends on the reactive surface, electrode material, the assisted membrane material, and pH of the solution. The measurement of the S2− form of sulfide species using an ISE-based H2S sensor requires an alkaline environment. A PHSS is also highly dependent on solution pH, and has a sensitivity of 5.5 nA μM−1 at pH = 6 where H2S is approximately 90% of the total sulfide.17 However, high sensitivity does not imply a low detection limit; the low detection limit of the sensor can make it more practical for a wide variety of samples. The detection limit of a PHSS is dependent on the pH, in anoxic 10 mM PBS at pH 7.0 and 37 °C it was near 10 nM, while the detection limit of an ISE sensor could be 100 nM; both thesensors are sufficient for biological applications.34,55 Selectivity is usually controlled by the applied voltage and the selective layer provided by each kind of H2S sensor. The acceptable voltage can reduce the noise signal level of other biological matrices oxidized on the electrode. An Ag/Ag2S ISE H2S electrode with a permissible Ag2S membrane specifically allows S2− ions to migrate across the thin layer which can effectively reduce interference from other biological matrices. The membranes of the PHSS are cemented together with silicone cement, which can prevent the interference from O2−, NO, and millimolar concentrations of hydrogen peroxide, sulfite, and sulfate. An enzyme-based H2S sensor with a special inhibited enzyme as the recognition element can make it just respond to H2S.17 Linear range and response time are important before performing any measurement for accurate results, as a wide linear range means that the sensor can be applied in additional sample detection. A typical commercially available H2S sensor from World Precision Instruments (PHSS, Sarasota, FL, USA) has a concentration range from 0.3 to 200 μM, which is much wider than that of ISE and enzyme based H2S sensors. However, the amperometric signal loses linearity at high H2S concentrations. The PHSS typically takes less than 10 s to reach 90% of the new signal in response to H2S changes by addition or removal. Sensor stability, long lifetime, and reproducibility are important parameters in order to make sure that there is no sensitivity loss. PHSS has a loss of H2S sensitivity and an elevated background current after several calibrations compared to earlier ones, these sensors require membrane or electrolyte replacement to ensure their sensitivity. To determine the precision of the PHSS response, 5 to 10 successive 2 μL injections of a 10 mM anoxic stock were made into a 2 mL anoxic chamber at pH 7.3 and 37 °C, yielding 10 μM H2S at each step, these stepwise injections produced a stepwise increase in current that differed by less than ±2% for each injection. Response accuracy was determined by periodically sampling the chamber solution and determining the H2S concentration with 2-PDS. Calibration of the PHSS was within ±1% of the 2-PDS assay. Biocompatibility is also extremely important when measuring H2S in vivo; the reactivity between the tissue and the electrochemical components must be minimal in order to verify there is no contribution to tissue inflammation. Since the PHSS electrochemical components are separated from sample solution by a selective membrane, there is no indication of altered biological processes.
6. Biological application of H2S electrochemical sensors
Detection of sulfide in biosamples has gained significant attention from analytical and biological applications; currently, several techniques have been used to measure H2S concentration in biological samples and biological sources like blood, serum, tissue, and breath.18 The continuous refinement of these techniques has improved detection specificity and lowered threshold limitations. An electrochemical method is still an effective and fast way to measure H2S and its derivatives in biological samples.
6.1. Electrochemical detection of H2S in plasma and blood
Detection of H2S in plasma (or blood) has significantly enhanced the understanding of pathophysiological conditions and predicted clinical outcomes.36 Sulfide species exist with an approximate ratio of 20% H2S, 80% HS−, and 0% S2− under physiological conditions at 37 °C and pH 7.4. Similar to NO and CO, H2S is lipophilic and permeates plasma membranes freely.19 Almost all studies reported that sulfide concentration in plasma (or blood) was between 10 and 300 μM by using the MBB methods, fluorescence probes, or ISE based methods.37 However, MBB and fluorescence methods require practically irreversible derivative reactions and take free sulfide out of the sample, which induces liberating sulfide from some of the reversibly bound sulfide complexes. An ISE H2S sensor is the most common method employed in plasma H2S detection, which was first reported in the detection of rat plasma H2S concentration to study the role of sulphide and sulphide oxidation in the copper molybdenum antagonism in 1987.51 Khan et al. made a full detailed procedure to rapidly estimate H2S concentration in blood using a sulfide ion electrode (Model 94-16A).52 With the properties of great availability and easy operation, ISE H2S electrochemical sensors have been widely used to detect plasma (or blood) H2S concentration in rat or trout blood to study its biological function as shown in Table 1. However, one of the major problems that existed in detection of sulfide in plasma (or blood) is to overcome the interference of other thiol species (GSH, protein cys derivatives etc.). Therefore, relatively high blood sulfide concentrations were detected using ISE based H2S sensors because the required alkaline condition of these sensors released some sulfide from a proposed sulfide–biomolecule.97 Free blood sulfide levels are much lower than the total species of sulfide. In order to overcome interference of biomolecules and other problems that ISE H2S sensors face, the PHSS was developed with a sulfide-selective membrane to protect the electrode material from sample solution (section 3.2). Olson's research reported that the ISE may not accurately measure free sulfide in the plasma due to its rapid consumption by blood.56 Furthermore, H2S consumption was also O2 dependent which was governed by the balance between constitutive production and oxidation.56 Wintner et al. also detected low free sulfide levels in animal blood using the MBB method; they measured a similar rate of added sulfide loss in human plasma samples with the MBB method and a polarographic sensor.13
6.2 Electrochemical detection of H2S in tissues and mitochondria
In order to better understand the biological roles of sulfide, it is necessary to build robust technologies that allow dynamic detection of endogenous sulfide in organs, tissues, or in vivo. However, dynamic measurements of sulfide in tissue samples are more problematic than that in the plasma (or blood) due to continuous enzymatic sulfide production and consumption processes. PHSSs, fluorescent probes, and MBB methods are shown to be able to detect sulfide levels in organs and tissues. Among these three methods, PHSS is well documented and is a reliable method to detect H2S levels in tissues or organs as reported by Kraus's groups and the Olson laboratory.34,76,98 These sensors can be employed for real-time free H2S detection with a low detection limit of 10 nM, and with great advantages of good reproducibility, short response, and a long linear range during detection without significant damage to the samples (section 3.2). With this approach, the production of H2S was found in almost every organ system, such as trout gill and heart, bovine heart and lung, and rat and seal lung.17,18,34,76,78 In short, the detection of H2S production using a PHSS in animal organ homogenate (or cells) was demonstrated by adding the substrate L-cysteine (L-cys) and the cofactor pyridoxal phosphate (PLP) for the enzymes cystathionineβ·synthase (CBS) and cystathionine γ lyase (CGL). These were used to dilute homogenate supernatant in a respirometer chamber using 5 μM O2, and the rise in the PHSS signal was recorded on a periodic basis. H2S production rate was determined at the initial steepest slope of each trace. Recent studies on H2S function suggested that the metabolism of H2S could serve as an oxygen sensor in many cell types in various species. PHSS provided the first direct measurements showing that there is an inverse relationship between O2 and H2S, thus making it a potential oxygen sensor for both the pulmonary and systemic systems.18,56,98–100 The detection of H2S consumption was exhibited by mitochondria isolated from the animal tissues (an inhabitant of sulfide-rich intertidal sediment) in an O2− dependent manner. Under air-equilibrated conditions, it was observed that mitochondrial H2S consumption led to simultaneous increase in O2 consumption, causing some investigators to use H2S-stimulated O2 consumption as an indirect and largely qualitative measure of H2S consumption. Using the PHSS to measure H2S consumption as well as O2 consumption provided a dynamic metabolic stoichiometry of H2S to O2 consumption; this demonstrated that H2S can be consumed in the absence of O2. This detailed assessment of mitochondrial H2S metabolism provided evidence of specific enzymatic steps and insight into mammalian H2S metabolism.
6.3. H2S in modulation of plant physiological processes
H2S is not just emerging as a third messenger molecule after nitric oxide (NO) and carbon monoxide (CO) involvement in modulation of physiological processes in animals systems,2–5 the role of H2S metabolism in a plant system has also attracted attention in recent years. The positive effect of H2S is also being identified as it is an important endogenous signalling molecule or bioregulator involved in many vital aspects of plant growth and responses against abiotic stresses.101,102 However, the metabolic pathways of H2S at different developmental stages of plants under various environmental perturbations is unclear. The electrochemical method with several advantages has become an important detection method to explore the H2S physiological processes in plants in recent years. Chen et al. used an electrochemical method (WPI, Inc., Sarasota, FL, USA) to detect the H2S in spinacia oleracea seedlings, which provides an effective way to understand its further roles in physiological processes of photosynthesis such as promoting chloroplast biogenesis, photosynthetic enzyme expression, and thiol redox modification.81 Garcia-Mata et al. measured endogenous H2S in leaf supernatant using a micro sulfide ion electrode (LIS-146AGSCM, Lazar Research Laboratories, Los Angeles, CA, USA) to study its functions of stomatal closure and participation in an ATP-binding cassette dependent signaling in guard cells.72 This method was also reported by Alvarez et al. to understand its specific role of sulfide as a general repressor of autophagy and a transcriptional modulator in Arabidopsis.73
6.4 Electrochemical detection of H2S for diagnoses and clinical therapeutic uses
The ultimate purpose of our study of H2S metabolism in bacteria, plants, invertebrates, and fungi is to evaluate our understanding of the important role of H2S in regulating human body function. Directly measuring H2S concentration in human plasma (or tissue) provides an effective way to explore its biological role and its potential therapeutic uses. Once a statistically significant difference between patients and normal control subjects is observed, we can conclude that H2S can be used as a biomarker for treatment decisions.36,39 An electrochemical H2S sensor is a fast and effective method to detect H2S in human plasma in order to explore the relationship between H2S concentration and several diseases such as chronic obstructive pulmonary disease, coronary heart disease, hypertension, etc. Significantly higher H2S concentration in serum was observed in patients with stable chronic obstructive pulmonary disease (COPD) than patients with acute exacerbation of COPD using an ISE H2S sensor (Model 9616, Orion Research, Beverly, MA).58,103 In addition, the study of age-matched control objects suggested that H2S can be used as a surrogate marker with the need for antibiotics.58,68,103 A lower plasma H2S concentration was observed in coronary heart disease (CHD) patients compared with angiographically normal control subjects by measuring H2S concentration in 40 patients with CHD and 17 angiographically normal patients using a sulfide-sensitive electrode.59 H2S concentration was also reported related to essential hypertension; metabolic imbalance of homocysteine (Hcy) was markedly increased and the final product of this pathway (plasma H2S) was significantly decreased in hypertensive children compared with normal subjects using an ionic meter (PXS-270, Shanghai Leici, China).61,104 Zhang et al. explored the predictive value of plasma H2S in differentiating between vasovagal syncope (VVS) and postural orthostatic tachycardia syndrome (POTS) in children (PXS-270, Shanghai Leici, China). Plasma H2S levels were significantly higher in children with VVS and POTS; the increased erythrocyte H2S production may be involved in the pathogenesis of VVS in children.105,106 Han et al. found that the abnormal activation of the AKT/GSK3β signal pathway in lymphocytes from systemic lupus erythematosus (SLE) patients plays an important role in the pathogenesis of the disease (ISO-H2S-100, WPI). Benavides et al. explored human RBCs converting garlic-derived organic polysulfides into H2S (measured in real time using a novel polarographic H2S sensor), suggesting that the major beneficial effects (cardiovascular diseases etc.) of garlic rich diets are mediated by the biological production of H2S from garlic-derived organic polysulfides.77 All the evidence has indicated that electrochemical detection is becoming a leading method to detect H2S for diagnoses and clinical therapeutic uses.
7. Conclusions
The enormous activity in the field of H2S biosensors is a reflection of the major clinical importance of this topic. An electrochemical method provides an effective, direct, and responsive real-time detection of the concentration of H2S without significant damage to living tissue. This approach is suitable for real-time detection of the concentration of H2S in different kinds of biological samples, and has significantly enhanced the understanding of H2S physiology functions. As researchers continue to unravel the complex biological roles of H2S and develop therapies based on H2S, the need for highly sensitive, highly selective, reproducible, biocompatible, and accurate H2S measurement devices will increase in the future. Finally, the increasing clarification regarding H2S in cells and tissues will lead to a better understanding of its role in the biological system. Due to the unique properties of electrochemical H2S, the future of H2S pathophysiology and chemical biology will be much clearer, and more research should be devoted to the detection of H2S in blood and tissues to meet the increasing requirement of in vivo studies and clinical diagnoses.
Acknowledgements
The work is supported by the National Natural Science Foundation of China (NSFC Grant No. 21475009, 21475008, 21175140, 21073203, 2127007, 21275017), the Fundamental Research Funds for the Central Universities (FRF-TP-15-014A3) and Beijing Higher Education Young Elite Teacher Project (YETP0424). The authors would like to express their sincere appreciation to the Deanship of Scientific Research at King Saud University for its funding of this research through the Research Group Project No. PRG-1436-011.
Notes and references
- V. Lantto and J. Mizsei, Sens. Actuators, B, 1991, 5, 21–25 CrossRef CAS
.
- H. Kimura, Mol. Neurobiol., 2002, 26, 13–19 CrossRef CAS PubMed
.
- D. Boehning and S. H. Snyder, Annu. Rev. Neurosci., 2003, 26, 105–131 CrossRef CAS PubMed
.
- K. Abe and H. Kimura, J. Neurosci., 1996, 16, 1066–1071 CAS
.
- R. Hosoki, N. Matsuki and H. Kimura, Biochem. Biophys. Res. Commun., 1997, 237, 527–531 CrossRef CAS PubMed
.
- C. Szabo, Nat. Rev. Drug Discovery, 2007, 6, 917–935 CrossRef CAS PubMed
.
- H. Kimura, Amino Acids, 2011, 41, 113–121 CrossRef CAS PubMed
.
- Y. Zhao, T. D. Biggs and M. Xian, Chem. Commun., 2014, 50, 11788–11805 RSC
.
- T. Ubuka, J. Chromatogr. Biomed. Appl., 2002, 781, 227–249 CrossRef CAS
.
- J. Furne, A. Saeed and M. D. Levitt, Am. J. Physiol.: Regul., Integr. Comp. Physiol., 2008, 295, R1479–R1485 CrossRef CAS PubMed
.
- M. D. Levitt, M. S. Abdel-Rehim and J. Furne, Antioxid. Redox Signaling, 2011, 15, 373–378 CrossRef CAS PubMed
.
- X. Shen, C. B. Pattillo, S. Pardue, S. C. Bir, R. Wang and C. G. Kevil, Free Radicals Biol. Med., 2011, 50, 1021–1031 CrossRef CAS PubMed
.
- E. A. Wintner, T. L. Deckwerth, W. Langston, A. Bengtsson, D. Leviten, P. Hill, M. A. Insko, R. Dumpit, E. VandenEkart, C. F. Toombs and C. Szabo, Br. J. Pharmacol., 2010, 160, 941–957 CrossRef CAS PubMed
.
- M. Nishida, T. Sawa, N. Kitajima, K. Ono, H. Inoue, H. Ihara, H. Motohashi, M. Yamamoto, M. Suematsu, H. Kurose, A. van der Vliet, B. A. Freeman, T. Shibata, K. Uchida, Y. Kumagai and T. Akaike, Nat. Chem. Biol., 2012, 8, 714–724 CrossRef CAS PubMed
.
-
X. Shen, G. K. Kolluru, S. Yuan and C. G. Kevil, in Methods Enzymol, ed. C. Enrique and P. Lester, Academic Press, 2015, vol. 554, pp. 31–45 Search PubMed
.
- X. Hu and B. Mutus, Rev. Anal. Chem., 2013, 32, 247–256 CAS
.
-
D. W. Kraus, J. E. Doeller and X. Zhang, in Electrochemical Sensors, Biosensors and their Biomedical Applications, ed. J. Wang, X. Zhang and J. Huangxian, Academic Press, San Diego, 2008, pp. 213–235 Search PubMed
.
- G. K. Kolluru, X. Shen, S. C. Bir and C. G. Kevil, Nitric Oxide, 2013, 35, 5–20 CrossRef CAS PubMed
.
- K. R. Olson, Biochim. Biophys. Acta, 2009, 1787, 856–863 CrossRef CAS PubMed
.
- K. R. Olson, Antioxid. Redox Signaling, 2012, 17, 32–44 CrossRef CAS PubMed
.
- A. R. Lippert, E. J. New and C. J. Chang, J. Am. Chem. Soc., 2011, 133, 10078–10080 CrossRef CAS PubMed
.
- C. Liu, J. Pan, S. Li, Y. Zhao, L. Y. Wu, C. E. Berkman, A. R. Whorton and M. Xian, Angew. Chem., Int. Ed., 2011, 50, 10327–10329 CrossRef CAS PubMed
.
- H. Peng, Y. Cheng, C. Dai, A. L. King, B. L. Predmore, D. J. Lefer and B. Wang, Angew. Chem., Int. Ed., 2011, 50, 9672–9675 CrossRef CAS PubMed
.
- Y. Qian, J. Karpus, O. Kabil, S. Y. Zhang, H. L. Zhu, R. Banerjee, J. Zhao and C. He, Nat. Commun., 2011, 2, 495 CrossRef PubMed
.
- K. Sasakura, K. Hanaoka, N. Shibuya, Y. Mikami, Y. Kimura, T. Komatsu, T. Ueno, T. Terai, H. Kimura and T. Nagano, J. Am. Chem. Soc., 2011, 133, 18003–18005 CrossRef CAS PubMed
.
- V. S. Lin, W. Chen, M. Xian and C. J. Chang, Chem. Soc. Rev., 2015, 44, 4596–4618 RSC
.
- J. Wang, Chem. Rev., 2008, 108, 814–825 CrossRef CAS PubMed
.
- T. Xu, N. Scafa, L.-P. Xu, L. Su, C. Li, S. Zhou, Y. Liu and X. Zhang, Electroanalysis, 2014, 26, 449–468 CrossRef CAS
.
- F. Bedioui and S. Griveau, Electroanalysis, 2013, 25, 587–600 CrossRef CAS
.
- A. Malinauskas, R. Garjonyt, R. Mazeikien and I. Jureviciut, Talanta, 2004, 64, 121–129 CrossRef CAS PubMed
.
- D. Lakshmi, M. J. Whitcombe, F. Davis, P. S. Sharma and B. B. Prasad, Electroanalysis, 2011, 23, 305–320 CrossRef CAS
.
- M. K. Zachek, A. Hermans, R. M. Wightman and G. S. McCarty, J. Electroanal. Chem., 2008, 614, 113–120 CrossRef CAS PubMed
.
- W. Chen, S. Cai, Q. Q. Ren, W. Wen and Y. D. Zhao, Analyst, 2012, 137, 49–58 RSC
.
- J. E. Doeller, T. S. Isbell, G. Benavides, J. Koenitzer, H. Patel, R. P. Patel, J. R. Lancaster Jr., V. M. Darley-Usmar and D. W. Kraus, Anal. Biochem., 2005, 341, 40–51 CrossRef CAS PubMed
.
- C. E. Paulsen and K. S. Carroll, Chem. Rev., 2013, 113, 4633–4679 CrossRef CAS PubMed
.
- K. R. Olson, E. R. DeLeon and F. Liu, Nitric Oxide, 2014, 41, 11–26 CrossRef CAS PubMed
.
- S. K. Pandey, K.-H. Kim and K.-T. Tang, TrAC, Trends Anal. Chem., 2012, 32, 87–99 CrossRef CAS
.
- E. Bitziou, M. B. Joseph, T. L. Read, N. Palmer, T. Mollart, M. E. Newton and J. V. Macpherson, Anal. Chem., 2014, 86, 10834–10840 CrossRef CAS PubMed
.
- B. D. Paul and S. H. Snyder, Nat. Rev. Mol. Cell Biol., 2012, 13, 499–507 CrossRef CAS PubMed
.
- M. Goubern, M. Andriamihaja, T. Nubel, F. Blachier and F. Bouillaud, FASEB J., 2007, 21, 1699–1706 CrossRef CAS PubMed
.
- D. J. Polhemus and D. J. Lefer, Circ. Res., 2014, 114, 730–737 CrossRef CAS PubMed
.
- J. W. Elrod, J. W. Calvert, J. Morrison, J. E. Doeller, D. W. Kraus, L. Tao, X. Jiao, R. Scalia, L. Kiss, C. Szabo, H. Kimura, C.-W. Chow and D. J. Lefer, Proc. Natl. Acad. Sci. U. S. A., 2007, 104, 15560–15565 CrossRef CAS PubMed
.
- C. K. Nicholson and J. W. Calvert, Pharmacol. Res., 2010, 62, 289–297 CrossRef CAS PubMed
.
- Y. Wang, X. Zhao, H. Jin, H. Wei, W. Li, D. Bu, X. Tang, Y. Ren, C. Tang and J. Du, Arterioscler., Thromb., Vasc. Biol., 2009, 29, 173–179 CrossRef CAS PubMed
.
- R. J. Reiffenstein, W. C. Hulbert and S. H. Roth, Annu. Rev. Pharmacol. Toxicol., 1992, 32, 109–134 CrossRef CAS PubMed
.
- A. V. Kroll, V. Smorchkov and A. Y. Nazarenko, Sens. Actuators, B, 1994, 21, 97–100 CrossRef CAS
.
- I. S. P. Savizi, H.-R. Kariminia, M. Ghadiri and R. Roosta-Azad, Biosens. Bioelectron., 2012, 35, 297–301 CrossRef CAS PubMed
.
- J.-L. Chang, G.-T. Wei, T.-Y. Chen and J.-M. Zen, Electroanalysis, 2013, 25, 845–849 CrossRef CAS
.
- Y. Wang, H. Yan and E. F. Wang, Sens. Actuators, B, 2002, 87, 115–121 CrossRef CAS
.
- G. Li, B. J. Polk, L. A. Meazell and D. W. Hatchett, J. Chem. Educ., 2000, 77, 1049 CrossRef CAS
.
- J. Mason, C. J. Cardin and A. Dennehy, Res. Vet. Sci., 1978, 24, 104–108 CAS
.
- S. U. Khan, G. F. Morris and M. Hidiroglou, Microchem. J., 1980, 25, 388–395 CrossRef CAS
.
- W. Zhao, J. Zhang, Y. Lu and R. Wang, EMBO J., 2001, 20, 6008–6016 CrossRef CAS PubMed
.
- G. Yang, L. Wu, B. Jiang, W. Yang, J. Qi, K. Cao, Q. Meng, A. K. Mustafa, W. Mu, S. Zhang, S. H. Snyder and R. Wang, Science, 2008, 322, 587–590 CrossRef CAS PubMed
.
- N. L. Whitfield, E. L. Kreimier, F. C. Verdial, N. Skovgaard and K. R. Olson, Am. J. Physiol.: Regul., Integr. Comp. Physiol., 2008, 294, R1930–R1937 CrossRef CAS PubMed
.
- K. R. Olson, M. J. Healy, Z. Qin, N. Skovgaard, B. Vulesevic, D. W. Duff, N. L. Whitfield, G. Yang, R. Wang and S. F. Perry, Am. J. Physiol.: Regul., Integr. Comp. Physiol., 2008, 295, R669–R680 CrossRef CAS PubMed
.
- R. A. Dombkowski, M. J. Russell and K. R. Olson, Am. J. Physiol.: Regul., Integr. Comp. Physiol., 2004, 286, R678–R685 CrossRef CAS PubMed
.
- Y. H. Chen, W. Z. Yao, B. Geng, Y. L. Ding, M. Lu, M. W. Zhao and C. S. Tang, Chest, 2005, 128, 3205–3211 CrossRef CAS PubMed
.
- H. L. Jiang, H. C. Wu, Z. L. Li, B. Geng and C. S. Tang, Di Yi Jun Yi Da Xue Xue Bao, 2005, 25, 951–954 CAS
.
- E. Distrutti, L. Sediari, A. Mencarelli, B. Renga, S. Orlandi, E. Antonelli, F. Roviezzo, A. Morelli, G. Cirino, J. L. Wallace and S. Fiorucci, J. Pharmacol. Exp. Ther., 2006, 316, 325–335 CrossRef CAS PubMed
.
- L. Chen, S. Ingrid, Y. G. Ding, Y. Liu, J. G. Qi, C. S. Tang and J. B. Du, Chin. Med. J., 2007, 120, 389–393 CAS
.
- Q. H. Meng, G. Yang, W. Yang, B. Jiang, L. Wu and R. Wang, Am. J. Pathol., 2007, 170, 1406–1414 CrossRef CAS PubMed
.
- B. Geng, Y. Cui, J. Zhao, F. Yu, Y. Zhu, G. Xu, Z. Zhang, C. Tang and J. Du, Am. J. Physiol.: Regul., Integr. Comp. Physiol., 2007, 293, R1608–R1618 CrossRef CAS PubMed
.
- T. Li, B. Zhao, C. Wang, H. Wang, Z. Liu, W. Li, H. Jin, C. Tang and J. Du, Exp. Biol. Med., 2008, 233, 1081–1087 CrossRef CAS PubMed
.
- G. P. Volpato, R. Searles, B. Yu, M. Scherrer-Crosbie, K. D. Bloch, F. Ichinose and W. M. Zapol, Anesthesiology, 2008, 108, 659–668 CrossRef CAS PubMed
.
- S. F. Perry, B. McNeill, E. Elia, A. Nagpal and B. Vulesevic, Am. J. Physiol.: Regul., Integr. Comp. Physiol., 2009, 296, R133–R140 CrossRef CAS PubMed
.
- L. Wu, W. Yang, X. Jia, G. Yang, D. Duridanova, K. Cao and R. Wang, Lab. Invest., 2009, 89, 59–67 CrossRef CAS PubMed
.
- Y.-H. Chen, W.-Z. Yao, J.-Z. Gao, B. Geng, P.-P. Wang and C.-S. Tang, Respirology, 2009, 14, 746–752 CrossRef PubMed
.
- Y.-H. Chen, R. Wu, B. Geng, Y.-F. Qi, P.-P. Wang, W.-Z. Yao and C.-S. Tang, Cytokine, 2009, 45, 117–123 CrossRef CAS PubMed
.
- A. K. Mustafa, M. M. Gadalla, N. Sen, S. Kim, W. Mu, S. K. Gazi, R. K. Barrow, G. Yang, R. Wang and S. H. Snyder, Sci. Signaling, 2009, 2, ra72 Search PubMed
.
- T. Chang, A. Untereiner, J. Liu and L. Wu, Antioxid. Redox Signaling, 2010, 12, 1093–1100 CrossRef CAS PubMed
.
- C. Garcia-Mata and L. Lamattina, New Phytol., 2010, 188, 977–984 CrossRef CAS PubMed
.
- C. Alvarez, I. Garcia, I. Moreno, M. E. Perez-Perez, J. L. Crespo, L. C. Romero and C. Gotor, Plant Cell, 2012, 24, 4621–4634 CrossRef CAS PubMed
.
- C. Hine, E. Harputlugil, Y. Zhang, C. Ruckenstuhl, B. C. Lee, L. Brace, A. Longchamp, J. H. Treviño-Villarreal, P. Mejia, C. K. Ozaki, R. Wang, V. N. Gladyshev, F. Madeo, W. B. Mair and J. R. Mitchell, Cell, 2015, 160, 132–144 CrossRef CAS PubMed
.
- P. Jeroschewski, C. Steuckart and M. Kühl, Anal. Chem., 1996, 68, 4351–4357 CrossRef CAS
.
- D. W. Kraus and J. E. Doeller, J. Exp. Biol., 2004, 207, 3667–3679 CrossRef CAS PubMed
.
- G. A. Benavides, G. L. Squadrito, R. W. Mills, H. D. Patel, T. S. Isbell, R. P. Patel, V. M. Darley-Usmar, J. E. Doeller and D. W. Kraus, Proc. Natl. Acad. Sci. U. S. A., 2007, 104, 17977–17982 CrossRef CAS PubMed
.
- J. R. Koenitzer, T. S. Isbell, H. D. Patel, G. A. Benavides, D. A. Dickinson, R. P. Patel, V. M. Darley-Usmar, J. R. Lancaster, Jr., J. E. Doeller and D. W. Kraus, Am. J. Physiol.: Heart Circ. Physiol., 2007, 292, H1953–H1960 CrossRef CAS PubMed
.
- K. R. Olson, N. L. Whitfield, S. E. Bearden, J. St Leger, E. Nilson, Y. Gao and J. A. Madden, Am. J. Physiol.: Regul., Integr. Comp. Physiol., 2010, 298, R51–R60 CrossRef CAS PubMed
.
- M. Whiteman, L. Li, P. Rose, C. H. Tan, D. B. Parkinson and P. K. Moore, Antioxid. Redox Signaling, 2010, 12, 1147–1154 CrossRef CAS PubMed
.
- J. Chen, F. H. Wu, W. H. Wang, C. J. Zheng, G. H. Lin, X. J. Dong, J. X. He, Z. M. Pei and H. L. Zheng, J. Exp. Bot., 2011, 62, 4481–4493 CrossRef CAS PubMed
.
- K. Suzuki, G. Olah, K. Modis, C. Coletta, G. Kulp, D. Gero, P. Szoleczky, T. Chang, Z. Zhou, L. Wu, R. Wang, A. Papapetropoulos and C. Szabo, Proc. Natl. Acad. Sci. U. S. A., 2011, 108, 13829–13834 CrossRef CAS PubMed
.
- Y. Han, F. Zeng, G. Tan, C. Yang, H. Tang, Y. Luo, J. Feng, H. Xiong and Q. Guo, Cell. Physiol. Biochem., 2013, 31, 795–804 CrossRef CAS PubMed
.
- C. Huang, J. Kan, X. Liu, F. Ma, B. H. Tran, Y. Zou, S. Wang and Y. Z. Zhu, PLoS One, 2013, 8, e69205 CAS
.
- K. Modis, C. Coletta, K. Erdelyi, A. Papapetropoulos and C. Szabo, FASEB J., 2013, 27, 601–611 CrossRef CAS PubMed
.
- K. R. Olson, E. R. Deleon, Y. Gao, K. Hurley, V. Sadauskas, C. Batz and G. F. Stoy, Am. J. Physiol.: Regul., Integr. Comp. Physiol., 2013, 305, R592–R603 CrossRef CAS PubMed
.
- U. Hasegawa and A. J. van der Vlies, MedChemComm, 2015, 6, 273–276 RSC
.
- E. A. Ostrakhovitch, S. Akakura, R. Sanokawa-Akakura, S. Goodwin and S. Tabibzadeh, Exp. Cell Res., 2015, 330, 135–150 CrossRef CAS PubMed
.
- Y. Sun, Y. Huang, R. Zhang, Q. Chen, J. Chen, Y. Zong, J. Liu, S. Feng, A. D. Liu, L. Holmberg, D. Liu, C. Tang, J. Du and H. Jin, J. Mol. Med., 2015, 93, 439–455 CrossRef CAS PubMed
.
- P. Huang, S. Chen, Y. Wang, J. Liu, Q. Yao, Y. Huang, H. Li, M. Zhu, S. Wang, L. Li, C. Tang, Y. Tao, G. Yang, J. Du and H. Jin, Nitric Oxide, 2015, 46, 192–203 CrossRef CAS PubMed
.
- M. D. Luque de Castro and M. C. Herrera, Biosens. Bioelectron., 2003, 18, 279–294 CrossRef CAS PubMed
.
- W. John Albery, A. E. G. Cass, B. P. Mangold and Z. X. Shu, Biosens. Bioelectron., 1990, 5, 397–413 CrossRef
.
- J. Zhao, R. W. Henkens and A. L. Crumbliss, Biotechnol. Prog., 1996, 12, 703–708 CrossRef CAS PubMed
.
- Y. Yang, M. Yang, H. Wang, J. Jiang, G. Shen and R. Yu, Sens. Actuators, B, 2004, 102, 162–168 CrossRef CAS
.
- L. Liu, Z. Chen, S. Yang, X. Jin and X. Lin, Sens. Actuators, B, 2008, 129, 218–224 CrossRef CAS
.
- N. Z. M. Poor, L. Baniasadi, M. Omidi, G. Amoabediny, F. Yazdian, H. Attar, A. Heydarzadeh, A. S. H. Zarami and M. H. Sheikhha, Enzyme Microb. Technol., 2014, 63, 7–12 CrossRef CAS PubMed
.
- P. Nagy, Z. Pálinkás, A. Nagy, B. Budai, I. Tóth and A. Vasas, Biochim. Biophys. Acta, 2014, 1840, 876–891 CrossRef CAS PubMed
.
- K. R. Olson, J. Exp. Biol., 2008, 211, 2727–2734 CrossRef CAS PubMed
.
- K. R. Olson, R. A. Dombkowski, M. J. Russell, M. M. Doellman, S. K. Head, N. L. Whitfield and J. A. Madden, J. Exp. Biol., 2006, 209, 4011–4023 CrossRef CAS PubMed
.
- K. R. Olson and N. L. Whitfield, Antioxid. Redox Signaling, 2010, 12, 1219–1234 CrossRef CAS PubMed
.
- Z.-G. Li, M. Gong, H. Xie, L. Yang and J. Li, Plant Sci., 2012, 185–186, 185–189 CrossRef CAS PubMed
.
- L.-Y. Hu, S.-L. Hu, J. Wu, Y.-H. Li, J.-L. Zheng, Z.-J. Wei, J. Liu, H.-L. Wang, Y.-S. Liu and H. Zhang, J. Agric. Food Chem., 2012, 60, 8684–8693 CrossRef CAS PubMed
.
- Y.-H. Chen, W.-Z. Yao, Y.-L. Ding, B. Geng, M. Lu and C.-S. Tang, Pulm. Pharmacol. Ther., 2008, 21, 40–46 CrossRef CAS PubMed
.
- M. Zheng, Q. Zeng, X. Q. Shi, J. Zhao, C. S. Tang, N. L. Sun and B. Geng, Chin. Med. J., 2011, 124, 3693–3701 CAS
.
- F. Zhang, X. Li, C. Stella, L. Chen, Y. Liao, C. Tang, H. Jin and J. Du, J. Pediatr., 2012, 160, 227–231 CrossRef CAS PubMed
.
- J. Yang, H. Li, T. Ochs, J. Zhao, Q. Zhang, S. Du, Y. Chen, P. Liu, Y. Wang, X. Feng, C. Zhang, C. Tang, J. Du and H. Jin, J. Pediatr., 2015, 166, 965–969 CrossRef CAS PubMed
.
|
This journal is © The Royal Society of Chemistry 2016 |
Click here to see how this site uses Cookies. View our privacy policy here.