Selecting a single powdered activated carbon against multiple threats: taste & odour and benzene†
Received
18th January 2022
, Accepted 19th October 2022
First published on 21st October 2022
Abstract
Recurring plans for building a large crude oil pipeline in the immediate neighborhood of the Saint Lawrence River and connected watercourses raise concerns about potential threats to this drinking water source serving over 3 million Canadians. Quebec has the lowest drinking water threshold for benzene in North America (0.5 μg L−1) – a toxic hydrocarbon present in crude oil. With powdered activated carbon (PAC) being an effective benzene adsorbent several municipalities around Montréal consider its application as an emergency barrier. Their current and primary PAC application is taste and odor removal caused by 2-methylisoborneol (MIB) and geosmin. Ideally, a single PAC would be selected as a suitable barrier against all three pollutants. The adsorption of MIB/geosmin was studied on 11 PAC in 3 source waters characterized by different concentrations of low molecular weight natural organic matter – the main competitor for adsorption sites on PAC. Results show that taste and odor removal was low in water with high fractions of small competitive natural organic matter. Benzene removal was studied separately in adsorption tests with 3 selected PAC. The highest (40 to 75%) and the lowest (10 to 15%) benzene removal was achieved by the same PAC types that also performed best and worst respectively for MIB/geosmin.
Water impact
Surface water treatment plants that already dose PAC for effective taste and odour control could use the same PAC as an emergency barrier against residual benzene traces following an oil spill. A PAC carefully selected with respect to pore size distribution and background organic matter for T&O control resulted in effective benzene removal as well.
|
1 Introduction
In the province of Quebec (Canada) 284 drinking water treatment plants (DWTP) are currently using surface waters as their raw water supply. Among these, 35 installations are dosing powdered activated carbon (PAC) for taste and odor (T&O) control. In recent years, discussions about the construction of an additional large crude oil pipeline in the proximity of the Saint Lawrence River and connected watercourses raised concerns about potential threats to drinking water intakes in the region of Montreal. Currently, three pipelines are already crossing four major drinking water sources in the Montreal metropolitan area serving over 3 million Canadians.1 The pipelines are transporting mostly crude oil, petroleum products, and light crude oil. One of the contaminants of concern is benzene, a small aromatic hydrocarbon that is regulated at 0.5 μg L−1 in Quebec's drinking water (5 μg L−1 in the and rest of Canada and the US)2–4 due to its carcinogenic and mutagenic potential. Data obtained during accidental oil spills and case studies show that benzene concentrations in DWTP intakes can range between 1 and 17 μg L−1 even weeks later which shows that not all benzene is removed due to natural weathering processes such as photooxidation or biodegradation.5–8 Besides air-stripping, adsorption on activated carbon is the most effective treatment to remove benzene from water.9 In the case of water sources known to be contaminated with volatiles such as benzene, toluene, ethyl-benzene and xylene (BTEX), water treatment is carried out using granular activated carbon (GAC) fixed-bed adsorbers.9 Full-scale studies have shown that GAC adsorbers are capable of reducing influent benzene concentrations of 10 μg L−1 to below 0.1 μg L−1 in the finished water.9 In emergencies such as oil spills in surface water, however, a quick and flexible response might be needed to deal with trace benzene concentrations. In that case, the dosage of PAC could be an option as it can be more readily applied in an existing DWTP.5 PAC is an effective adsorbent for organic pollutants.10,11 The question arises as whether a DWTP which already doses PAC for T&O control would also at the same time be protected against trace benzene contaminations following a potential oil spill. Few studies exist that evaluate the adsorption of trace benzene concentrations by PAC from natural water.12 A pilot study had found that a combined flocculation/PAC system with 60 mg L−1 of PAC reduced benzene concentrations from 100 to 5 μg L−1 within 2 to 8 minutes.12 A previous study found that PAC doses as high as 80 mg L−1 of PAC were required to reduce residual benzene concentrations in surface water from 14 μg L−1 to 0.5 μg L−1 within 15 to 30 min during ballasted flocculation.5 Adsorption performance for trace organics is highly dependent on the material properties of the adsorbent and the presence of competing NOM.13 A study comparing different PAC and water matrices for the adsorption of both T&O molecules and benzene has not yet been published.
Prior to the here presented study, we carried out a survey among 7 participating DWTP in the Montreal metropolitan area. These DWTP dose 5 to 10 mg L−1 of PAC, either seasonally or all year round, to remove the molecules 2-methylisoborneol (MIB) and geosmin, which are giving a musty/earthy odor to the water and regularly trigger consumer complaints. MIB and geosmin are secondary metabolites mostly produced by cyanobacteria but also other organisms (e.g. actinomycetes) which are present in biofilms or in a planktonic state.14 Typical T&O events occur in summer until late autumn and peaks of 18 to 60 ng L−1 of geosmin and MIB respectively can be detected in the St-Lawrence River around Montreal.15 T&O events with average concentrations of 1 to 6 ng L−1 of geosmin and peak concentrations of 11 to 17 ng L−1 were detected in three water sources near Quebec City.16 Removals of 50 to 90% would be necessary to bring these concentrations below the odor threshold which is usually between 5 and 10 ng L−1 for MIB and geosmin. The research question of this study is: can a PAC chosen for T&O control also remove benzene effectively? We tested the MIB, geosmin, and benzene removal performance of 11 PAC recommended for T&O control in 3 raw waters characterized by different concentrations of natural organic matter. Our study investigated the role of PAC characteristics such as micropore volume and particle size as well as the role of background organic matter on the removal efficiency of MIB, geosmin, and benzene under typical operating conditions of DWTPs.
2 Materials and methods
2.1 Adsorbents
Local PAC suppliers on Quebec's market were contacted to send up to 3 PAC samples recommended for T&O control. In total, 11 PAC characterized by different base-materials were selected for MIB/geosmin adsorption tests (Table 1). All carbons were characterized for their pore size distribution and specific surface area applying the non-linear density functional theory for slit/cylindrical pore shapes (NLDFT) to recorded nitrogen adsorption isotherms (Quantachrome Autosorb 1 MP, relative pressure P/P0 varying between 10−6 and 100 at 77 K). Particle size distributions have been recorded using a Mastersizer 3000. The carbons were dried at 105 °C overnight and cooled down in a desiccator to remove humidity. PACs were pre-wetted for >12 h by preparing a PAC slurry in 50 mL of water that was shaken on a horizontal shaker overnight until use the next day. For benzene adsorption experiments, three PAC were selected according to their performance (medium and high) during the MIB/geosmin experiments.
Table 1 PAC characteristics
PAC type |
Brand |
Base material |
Micro pore volume |
Mesopore volume |
Specific surface area |
Iodine # |
D
50
|
D
10
|
D
90
|
D
60/D10 |
mL g−1 |
mL g−1 |
m2 g−1 |
mg g−1 |
μm |
μm |
μm |
WPC |
Calgon |
Coconut |
0.25 |
0.27 |
869 |
800 |
15 |
2 |
47 |
9.9 |
WPH W900 |
Wood |
0.34 |
1.88 |
1358 |
900 |
27 |
7 |
74 |
4.8 |
WPH |
Bituminous |
0.31 |
1.12 |
1067 |
800 |
18 |
4 |
49 |
5.9 |
COL-PL60-600 |
Canada Colors & Chemicals |
Bituminous |
0.19 |
0.58 |
766 |
650 |
23 |
5 |
68 |
6.5 |
COL-PL-60-800 |
Bituminous |
0.29 |
0.83 |
1071 |
800 |
16 |
4 |
40 |
5.5 |
PAC-500-NSF |
Chemco/Carbon Canada |
Lignite |
0.21 |
0.82 |
852 |
500 |
18 |
3 |
18 |
6.7 |
PAC-600-NSF |
Lignite |
0.25 |
0.58 |
839 |
600 |
26 |
5 |
26 |
7.1 |
Hydrodarco-B |
Norit |
Lignite |
0.30 |
0.14 |
1154 |
550 |
16 |
3 |
45 |
5.8 |
Hydrodarco-M |
Lignite |
0.16 |
0.81 |
686 |
550 |
15 |
4 |
45 |
5.4 |
Hydrodarco-W |
Lignite |
0.15 |
0.98 |
605 |
500 |
15 |
5 |
59 |
4.0 |
AquaSorb CB-MW |
Jacobi |
Blend (coal and coconut) |
0.38 |
0.16 |
1256 |
950 |
13 |
4 |
37 |
5.1 |
2.2 Water matrices and contaminants
Adsorption experiments were carried out using raw water from the Mille Îles River, the L'Assomption River, and the Saint Lawrence River, which are differing concerning their NOM content (Table 2). Waters were filtered through 0.45 μm filters (PALL Supor450 PES, previously rinsed with 1 L ultra-pure water) and stored at 4 °C to remove solids and to reduce microbial activity until use. The raw waters were characterized on the sampling day and the day of the adsorption experiments concerning their dissolved organic carbon (DOC) (Standard Methods APHA 2012, TOC meter Sievers 5310-C), UVA254 (Standard Methods APHA 2012, 5910B), pH, turbidity (Standard Methods APHA 2012, 2320B, Hach turbidimeter) and alkalinity (Standard Methods APHA 2012, 2130B). For adsorption experiments in ultra-pure water (18.2 MΩ cm−1 at 25 °C), water was filtered using a Milli-Q water purification system (Millipore Sigma, Bedford, MA) with subsequent adjustment with NaCl and NaOH to obtain an ionic strength and pH similar to the river water. MIB and geosmin were bought in methanol (drinking water odor standards, Sigma Aldrich, Canada), and a small volume of stock solution was prepared in a 20 mL glass vial with ultra-pure water the same day of the experiments. Benzene stock solutions were prepared from pure benzene (HPLC grade, 99.9% purchased at Sigma Aldrich, St-Louis, MO). To avoid the volatilization of benzene during the preparation of the stock solution, pure benzene was injected with an air-tight glass syringe through the Teflon septum (crimp caps) into a headspace-free glass bottle. The solution was stirred with a magnetic stirrer for at least 30 min before use. The aliquots of the stock solution were taken through the septum using an airtight glass syringe.
Table 2 Characteristics of the tested water matrices for MIB/geosmin and benzene tests
Tested contaminant |
Water type |
DOC |
UVA254 |
pH |
Turbiditya |
Alkalinitya |
(mg L−1) |
(1 cm−1) |
(−) |
(NTU) |
(mg CaCO3 per L) |
Before filtration through 0.45 μm.
|
MIB/geosmin |
Mille Îles River, raw |
6.20 |
0.185 |
7.18 |
18.0 |
50 |
L'Assomption River, raw |
4.67 |
0.126 |
7.15 |
5.16 |
52 |
Saint Lawrence River, raw |
2.71 |
0.041 |
7.98 |
1.33 |
70 |
Benzene |
Mille Îles River, raw |
6.67 |
0.223 |
6.96 |
17.0 |
44 |
Mille Îles River, treated |
2.33 |
0.118 |
6.33 |
0.35 |
13 |
Saint Lawrence River, raw |
2.70 |
0.136 |
7.88 |
0.71 |
60 |
2.3 Analytical methods
NOM was characterized by quantifying five size fractions including biopolymers, humic acids, building blocks, low molecular weight (LMW) acids and LMW neutrals using LC-OCD. The LC-OCD system employed a weak cation exchange column (polymethacrylate based, TSK HW 50S, TOSOH, Japan) equipped with different detectors, among them the organic carbon detector (OCD), and the UV (254 nm) detector (UVD).20 The organic carbon properties of the NOM components were characterized and quantified using a software program provided by the manufacturer (ChromCALC, DOC-LABOR, Karlsruhe, Germany). Also, DOC was determined using the bypass DOC of the LC-OCD. Prior to LC-OCD analysis, all water samples were pre-filtered through a 0.45 μm PES filter (Supor®, Pall Corporation), and shipped within 24 h on ice to the laboratory.
All analytical method parameters and validation data for benzene, MIB and geosmin analysis are summarized in Table S1 in the ESI.† Two different methods were used to analyze the target compounds, one for benzene, and the other for MIB and geosmin (see ESI†). Certified standards and relative internal standards of benzene, MIB and geosmin were purchased from Sigma-Aldrich (St. Louis, MO). Target compounds were analyzed via stir bar sorptive extraction coupled to a gas chromatography-mass spectrometry system (SBSE-GC-MS). A PAL auto sampler (Zwingen, Switzerland) and a Gerstel Twister microextraction system (Baltimore, MD) coupled to a GC 3800-MS 4000 from Varian (Pablo Alto, CA) was used to perform the analyses. Separation of target compounds was done with a SLB-5 ms column (30 m × 0.25 mm × 0.25 μm film thickness) from Sigma Aldrich (Saint-Louis, MO). Helium was used as carrier gas flow at 1.5 mL min−1. Stir bars were thermally desorbed in glass thermal desorption tubes and cryo-focused at −100 °C using liquid nitrogen in a thermal desorption unit-cooled injection system (TDU-CIS) provided by Gerstel (Baltimore, MD). Thermal desorption gradients for each method are described in Table S1.† Injection was done in splitless mode for benzene, while it was done in solvent venting mode for MIB and geosmin. Ionization of target compounds was done by electron impact (EI). The mass spectrometer was used in single ion monitoring mode (SIM) to increase sensitivity in quantitative analysis. Target compounds were monitored by measuring the most abundant ions and specific ions were also used for confirmation (Table S1†).
Sample preparation included filtration through 0.3 μm glass fiber filters (Sterlitech GF-75) and microextraction. Since target compounds were volatile, internal standards (i.e. benzene-d6 and cis-decahydro-1-naphtol) were used to correct the signal. Analytical controls (10 and 50 ng L−1) were also added throughout the analysis sequence to control signal drifting. Target compounds were extracted from 50 mL water samples in a vial of about 60 mL with polydimethylsiloxane (PDMS) coated stir bars (20 mm × 0.5 mm − 48 μL of PDMS) provided by Gerstel (Baltimore, MD). About 10 g of NaCl salt was added to the water samples for MIB and geosmin to help extraction, while none was added for benzene samples. The addition of NaCl modifies the ionic strength of the sample solution, reduces the water solubility, and improves the extraction efficiency of polar compounds such as MIB and geosmin.21–23 PDMS coated stir bars were put into the water samples for MIB and geosmin extraction. While, for benzene extraction, the stir bars were held in place within the vial cap with the help of a magnet in order to adsorb this highly volatile compound in the vial headspace. After extraction, the PDMS coated stir bars were rinsed with Milli-Q water and dried on tissue paper before desorption.
2.4 Adsorption tests for MIB and geosmin
For MIB/geosmin adsorption experiments, a batch of 7 L raw water was spiked with a MIB/geosmin stock solution to obtain a concentration of 100 ng L−1 of each contaminant which simulates a worst-case T&O event in the water intake.15 The spiked solution was stirred for 30 min with little headspace using a magnetic stirrer. Adsorption experiments were carried out in 500 mL amber glass bottles closed with a Teflon cap. The spiked raw water was treated with a typical PAC dose of 5 mg PAC per L (dosed as a slurry) and a contact time of 15 min. These conditions were chosen to represent typical PAC treatment conditions in the participating municipal drinking water treatment plants in the greater Montreal metropolitan area. In Quebec (Canada), conventional treatment of drinking water is commonly realized with the Actiflo® process combining ballasted flocculation, which involves the addition of microsand with a lamellar flocculator for rapid settling (up to 85 m h−1). Typical contact times of PAC in such a system are 10 to 15 minutes.24
The reaction was stopped by filtering the suspension immediately through a 0.45 μm PES filter (Supor®, Pall Corporation). Controls containing the spiked water matrix without PAC were added to each experimental series. The controls showed that losses of MIB and geosmin due to volatilization or adsorption on the equipment were negligible.
2.5 Adsorption tests for benzene
Benzene adsorption isotherm and kinetic experiments were carried out as well as adsorption experiments with a single contact time (15 min) and PAC dose (5 mg L−1) in order to compare the results with the MIB/geosmin results. In all experiments the bottle-point technique was used. Three selected PACs (AquaSorb CB1-MW, WPH, and Hydrodarco-W) were chosen for the benzene experiments, based on the results of the previous MIB/geosmin experiments and their different base materials (coal-coconut, bituminous and lignite). To avoid losses of benzene due to volatilization, an airtight setup was designed for the adsorption experiments (Fig. S1†). Each bottle was prepared by adding a magnetic stirrer and filling it up to the brim with the respective water matrix. The water was spiked with the benzene stock solution, immediately closed with the cap without leaving a headspace, and stirred for 10 s at high speed. At the beginning of an adsorption experiment, PAC was added quickly as a slurry. The cap of each bottle was equipped with Teflon tubing and a valve. At the end of each test, each bottle was connected to a nitrogen reservoir and to a stainless-steel filter support. The adsorption test was stopped by pressurizing the test-bottle with nitrogen and filtering the solution through a 0.2 μm glass fiber filter (the first 40 mL of sample were discarded to rinse the setup). Samples were collected in 40 mL vials without headspace and closed immediately with a Teflon cap until analysis. Two control bottles containing the spiked solution without PAC were added to each experiment in order to obtain an initial benzene concentration of the experiment that accounts for losses due to volatilization and adsorption on the materials.
3 Results and discussion
3.1 Variability of MIB & geosmin sorption performance using 11 PACs
With typical contact times of 15 min and a PAC dose of 5 mg L−1, removal performances varied widely between PAC types (0 to 45% for both MIB and geosmin, initial concentration of 80 ng L−1 MIB and geosmin) in the three tested water matrices (Fig. 1a and S4†). The low or insufficient performance (<10%) of some of these carbons occurred despite the fact that all of the carbons were initially recommended for T&O control. For typical T&O peaks (10 to 30 ng L−1 MIB and geosmin), the currently applied PAC doses of 5 to 10 mg L−1 in the participating DWTP might not be sufficient to lower concentrations below the odor threshold of 5 to 10 ng L−1 of MIB and geosmin. When expressed as relative performance (with best PAC = 100%), the worst performing PAC is up to a factor 10 less efficient than the best PAC tested (Fig. 1b and S5†). Among the 11 PACs, two products were the best in all three water matrices: AquaSorb CB1-MW (a mixture of coconut and coal-based PAC) and WPH W900 (wood-based PAC). The third best PAC was Hydrodarco-B (lignite-based PAC). Previous studies reported a better removal of geosmin by PAC compared to MIB25,26 which is related to the lower molecular weight of geosmin, its slightly lower solubility and, flatter molecular structure letting it access also smaller pores. The removal performances for MIB and geosmin in our study, however, were very similar in all tests (paired t-test for dependent variables with α = 0.05, p-value 0.67). In 90% of the tests, the best PAC for MIB removal was also the best for geosmin removal.
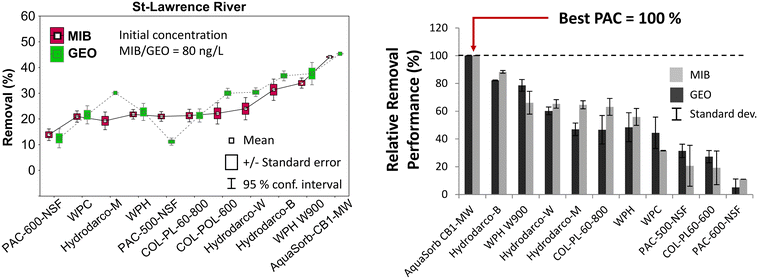 |
| Fig. 1 Left: MIB and geosmin removal from pre-filtered St-Lawrence River water and right: relative removal performance normalized to the best performing PAC in water from the L'Assomption River (performances in other water matrices can be found Fig. S4 and S5†). | |
3.2 The influence of PAC properties and natural organic matter on performance
Selecting PAC for T&O removal is a challenge because the adsorption kinetics and capacity depend (i) on the PAC characteristics but (ii) also on the water matrix.13 Ideally, each water utility would test different PACs and select according to its performance/price ratio. However, testing PAC performance for specific contaminants such as MIB/geosmin is often too expensive and time consuming for municipalities. Instead, PAC is bought according to a few criteria such as (i) approved by the NSF, (ii) iodine number higher than 500 (and other criteria such as ashes content according to the AWWA Standard), and among those they select (iii) the cheapest bidder. The base materials of the 11 carbons tested included lignite, bitumen, coconut, wood, and coal (Table 1). The three best performing carbons at a short contact time of 15 min were coconut-coal-based (AquaSorb CB1-MW), wood-based (WPH900) and lignite-based (Hydrodarco B). Previously, chemically-activated wood-based carbons were found to have inferior adsorption capacity at equilibrium due to the more hydrophilic surface.27 Later studies have shown that the pore size distribution is the most important carbon characteristic for micropollutant adsorption.28 High micropore volume has been associated with high equilibrium adsorption capacities for small micropollutants29 while mesopores were identified as crucial for adsorption kinetics, favoring rapid diffusion to adsorption sites located in micropores.27 Typical PAC contact times during water treatment are 5 min to 2 h where adsorption kinetics are more important than equilibrium considerations (often reached after >24 h). A chemically-activated wood-based carbon that showed inferior equilibrium adsorption capacity compared to highly microporous coconut PAC was showing superior performance at contact times <2 h because this wood based PAC had a higher volume of larger transport pores.26,27
The micropore volume of all PAC used in our study (15 min contact time and 5 mg PAC per L) varied between 0.15 to 0.38 mL g−1, the mesopore volumes varied between 0.16 to 1.88 mL g−1 (Table 1). The best performing PAC (AquaSorb CB1-MW) had a narrow pore size distribution (Fig. S8†) with the highest micropore volume (0.38 mL g−1) and the lowest mesopore volume (0.16 mL g−1) of all carbons tested. The second-best PAC was also characterized by a high micropore volume (0.34 mL g−1) but also a very high mesopore volume (1.88 mL g−1). While micropore volume seems to be an important factor for MIB and geosmin removal during our experience, it was not linearly correlated to removal performance (see Fig. 2, R2 = 0.18). While this non-linear correlation seems to contrast with existing data,29 our study was carried out at 15 minutes contact time (not at equilibrium) where kinetics are still dominating the adsorption process and both micro- and mesopores are relevant.
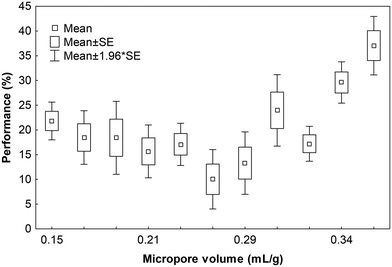 |
| Fig. 2 MIB & geosmin removal performance of 11 PAC as a function of micropore volume. | |
While measuring micropore volume is often not an option for DWTPs, the iodine number is mostly referred to as an easy predictor for PAC performance and is a parameter that is generally reported in the technical data sheets of the products. Due to the small size of iodine, the iodine number (mg iodine per g PAC) is thought to be correlated to the internal surface area and thus to the adsorption capacity of small organic pollutants.30,31 For the 11 tested carbons in this study, the iodine number was only weakly linearly related to the specific surface area (R2 = 0.56) and slightly better correlated to the micropore volume (pore diameter <2 nm) (R2 = 0.66) (Fig. S2†). In general, the iodine number was not a good predictor for the MIB/geosmin removal performance (Fig. S3†) as has already previously been reported.29
Although no satisfying linear correlation between micropore volume (or iodine number) and MIB/geosmin removal performance exists, it should be noted, that the highest removals observed under the chosen conditions were indeed achieved by the carbons with the highest micropore volume of 0.34 and 0.38 mL L−1 and the highest iodine numbers i.e. >900 mg g−1 (Fig. S3†).
The 11 PAC particle size distributions were characterized by D10 values between 2 μm and 7 μm, D50 values between 13 μm and 27 μm and uniformity coefficients Uc (D60/D10) varying between 4 and 10 (Table 1). Carbons with higher amounts of smaller particles (smaller D50 or smaller Uc) are expected to have higher loading of pollutants due to faster kinetics in our 15 min experiments. However, linear correlations between MIB/geosmin removal performance in different matrices and distribution descriptors such as D10, D50 or Uc were generally poor (R2 < 0.1).
The background NOM of the water matrix is known to influence the adsorption of T&O molecules and other small organic pollutants due to the competition for adsorption sites.13 In natural water, NOM is present at much higher concentration than the target T&O pollutant and in general, the adsorption of T&O compounds is lower in water with high DOC. In our study, the removal of MIB and geosmin was affected by the water matrix – although the effect of the carbon type was more important (Fig. 3). Differences in removal performance of 30 to 40% were observed between water types (Fig. 3). For both pollutants, MIB and geosmin, their removal was best in the St. Lawrence River water: paired t-tests with α = 0.05 showed that removals in St. Lawrence river where significantly different from both other matrices (with p < 0.01 when compared to the L'Assomption River and p < 0.01 when compared to the Mille Îles River). The St. Lawrence River water is characterized by the lowest DOC of 2.7 mg L−1 among the waters tested (Fig. 4 and S7†). Removals for both molecules in the two other water matrices were not statistically different, (comparing removals in L'Assomption River and Mille Îles River water using paired t-tests with p = 0.301), despite the different DOC concentrations (L'Assomption River and the Mille Îles River were 4.7 mg L−1 and 6.2 mg L−1 respectively) in these raw waters.
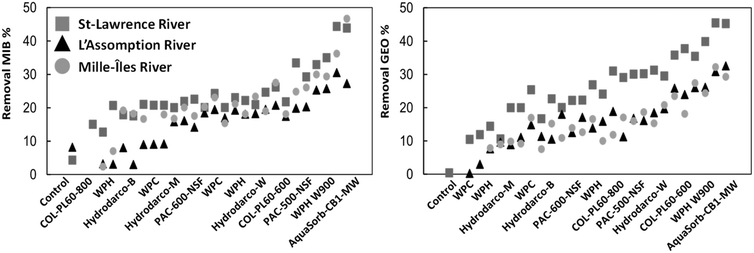 |
| Fig. 3 Adsorption results of all PAC types in different water matrices. Duplicates are shown as individual data points, not as average with error bars. | |
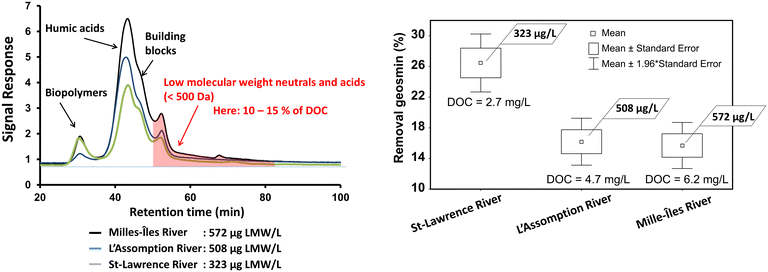 |
| Fig. 4 Left: Size distribution of natural organic matter NOM and right: the influence of LMW NOM (ug C per L) on the removal of geosmin (the results for MIB can be found in the ESI† Fig. S7). | |
While the DOC is a measure to quantify NOM, it does not allow quantifying its competitiveness for sorption sites. Only the NOM fraction with a low molecular weight (defined as the fraction <500 Da) competes with small organic target pollutants for the same adsorption sites.32 LC-OCD analysis revealed that LMW NOM, which consists of LMW acids and LMW neutrals, represented only 10 to 15% of the total NOM (Fig. 4a). This is typical for surface water NOM which is usually dominated by a high percentage of humic substances and much lower percentage of biopolymers, building blocks, LMW acids and LMW neutrals.33,34 The concentration of LMW NOM in the St. Lawrence River was with a concentration of 323 μg C per L 44% lower than in the Mille Îles River (572 μg C per L), while the LMW NOM in the L'Assomption River was with 508 μg C per L only 11% lower than in the Mille Îles River. In the present study, the differences in the removal performance of PAC in the 3 water matrices were related to the LMW NOM fraction, with higher removals observed in water with low LMW NOM and low removals in water with high LMW NOM (Fig. 4b).
To evaluate the respective influence of the PAC characteristics and the water matrix, their strength as a predictor in a general linear model for T&O removal performance was tested via stepwise regression with backward elimination (Statistica 13 academic software, Tibco, USA). We included the categorical predictors “water matrix” and “pollutant”, the continuous predictors “micropore volume” and “uniformity coefficient” or “diameter D50” as well as their interactions (matrix × pollutant, matrix × micropore volume, pollutant × micropore volume, etc.). Micropore volume, uniformity coefficient and water matrix were statistically significant predictors (p = 0.005, 0.002 and p < 0.001) in all models (Table S3†), while D50 as a single value of the particles size distribution was a weak predictor. Lower uniformity coefficients characterize a steeper particle size distribution and thus PACs with bigger fractions of small particles. Among these predictors, the micropore volume had the most important effect highlighting the importance of the PAC characteristics and confirming its relatively higher importance with respect to the water matrix. Replacing the categorical predictor “water matrix” with the continuous predictors “low molecular weight NOM” or “DOC” reduced the model's predictive power for overall pollutant removal performance (lower coefficient of determination R2) (Table S3†).
3.3 Benzene adsorption
Benzene removal by PAC was tested under the same conditions as described above for the T&O molecules MIB and geosmin. Volatilization was not considered as a removal mechanism for benzene. After 15 min contact time, 15% to 60% of the initial benzene (C0 = 16 to 24 μg L−1) was removed in water containing the highest concentration of DOC and LMW NOM (Mille Îles River water). The best PAC (AquaSorb CB1-MW) removed 60% of the initial benzene – a higher removal compared to the MIB & geosmin removals observed previously (45%). AquaSorb CB1-MW was also the best performing one for MIB and geosmin removal indicating that its high micropore volume and specific surface plays an important role in the removal of these contaminants (Fig. 5). A greater variation was recorded for benzene and is related to its vapor pressure which is much lower than that of MIB/geosmin, suggesting that this compound is much more volatile (Table 3). The better benzene removal might be related to the smaller size of the benzene molecule compared to MIB & geosmin (Table 3). Benzene removal kinetics for the lowest-performing PAC tested (WPH) showed that >50% of the initial benzene was removed after 30 min contact time (78% of PAC's adsorption capacity) (Fig. 5b).
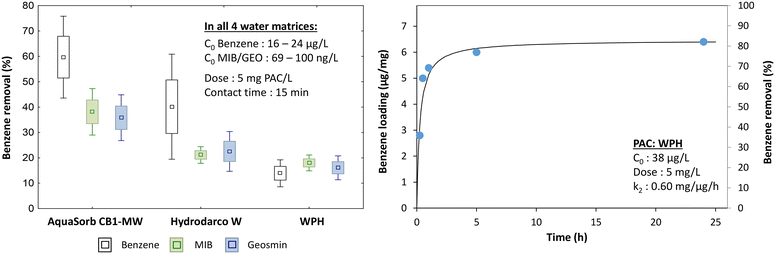 |
| Fig. 5 Left: Benzene removal compared to MIB and geosmin removal and right: benzene removal kinetics. | |
Table 3 Contaminant characteristics
Pollutant |
Moleculea |
Molecular weighta (g mol−1) |
pKab at 25 °C |
Log Kowc |
Vapor pressured (atm) at 20 °C |
Typical smell |
Odour thresholde (ng L−1) |
Detection limitf GC/MS (ng L−1) |
PubChem online database.
Obtained from the ChemAxon online calculator, all three contaminants have negligible acid–base properties.
Log Kow values for MIB and geosmin,17 value for benzene from the PubChem database.
MIB and geosmin obtained from,18 benzene from PubChem.
Odour threshold for MIB & geosmin.19
Average detection limits during the tests of this study.
|
Geosmin |
|
182.3 |
−0.0042 |
3.70 |
5.49 × 10−5 |
Earthy, camphor |
1–10 |
0.7 |
2-Methyl-isoborneol (MIB) |
|
168.28 |
−0.42 |
3.13 |
7.26 × 10−5 |
Soil, musty |
5–10 |
1.4 |
Benzene |
|
78.11 |
43 |
2.13 |
9.8 × 10−3 |
Sweet aromatic, gasoline-like |
700–1000 |
60 |
Benzene removals of 15 to 75% under typical operating conditions (15 min contact time and 5 mg PAC per L) would not be sufficient to remove benzene trace contaminations below the drinking water threshold of Québec (0.5 μg L−1). Few studies report trace benzene removal by PAC: in a study with initial benzene concentrations in the range of 5 to 40 mg L−1 and PAC concentrations of 20 to 160 mg L−1 (exact conditions of the kinetic tests are not reported) benzene removal reached equilibrium after only 15 min.35 Other authors found that 60 mg PAC per L were necessary to remove 95% of benzene (initial concentration of 100 μg L−1) in a jet-flocculator with 2–8 min PAC residence time in the reactor.12 This indicates that with typically short residence times in the participating DWTP, PAC used for MIB and geosmin removal is suited to deal with benzene contamination but the dose needs to be drastically increased in order to remove benzene below the drinking water threshold.
4 Conclusions and recommendations
Our study has shown that PAC applied at typical concentrations of 5 mg L−1 and low contact time of 15 min can remove a maximum of 30 to 45% of MIB and geosmin and up to 75% of benzene with the best PAC selected. These low removals are mostly insufficient to remove MIB and geosmin below the odor threshold during T&O events surpassing 20 ng L−1 of either molecule. The same was observed for residual benzene that could be present in the raw water following an oil spill with reported concentrations of 1 to 17 μg L−1.5 Even the maximum removals recorded during this study cannot sufficiently remove benzene traces below the Quebec drinking water threshold (0.5 μg L−1). We observed performance variations by factors 3 to 4 between PAC types and up to a factor of 2 between water matrices. The best performing PACs were best in all water matrices and for all tested contaminants. The micropore volume was the only significant predictor for T&O removal performance with the best PAC having micropore volumes exceeding 0.3 mL g−1.
Our research has shown that DWTP dosing 5 mg L−1 PAC for T&O control in the Montreal area have no sufficient barrier in place to protect themselves against toxic benzene residuals following an oil spill. Selecting a better performing PAC for T&O will also improve the removal of benzene. However, planning for the injection of higher PAC doses will most likely be necessary to comply with benzene drinking water threshold in Canada and the US.
Abbreviations
BTEX | Benzene, toluene, ethyl-benzene and xylene |
DOC | Dissolved organic carbon |
DWTP | Drinking water treatment plant |
GAC | Granular activated carbon |
LMW | Low molecular weight |
MIB | 2-Methylisoborneol |
NOM | Natural organic matter |
PAC | Powdered activated carbon |
PDMS | Polydimethylsiloxane |
T&O control | Taste and odor control |
Conflicts of interest
There are no conflicts to declare.
Acknowledgements
Thanks to Lin Shen who did the LC-OCD analysis at NSERC IRC at the University of Waterloo. Thanks to the participating municipalities (Rosemère, L'Assomption) and the industrial partners of the NSERC Chair at Polytechnique Montreal (Montreal, Veolia Canada, Laval, Longueuil, Repentigny) for funding this research. The research was conducted at the CREDEAU, a Canada Foundation for Innovation (CFI) funded infrastructure.
References
-
S. Barrette, Vulnérabilité des usines de traitement d'eau potable de la région métropolitaine de Montréal aux déversements accidentels d'oléoducs, M.Sc.A, Polytechnique Montréal, 2018 Search PubMed.
-
Ministère du Développement Durable de l'Environnement et des Parcs du Québec (MDDEP), Guide d'interprétation du règlement sur la qualité de l'eau potable, 2012 Search PubMed.
-
INSPQ, Fiches synthèses sur l'eau potable et la santé humaine - benzène, https://www.inspq.qc.ca/eau-potable, (accessed 25-10-2022, 2022) Search PubMed.
-
United States Environmental Protection Agency (USEPA), 2011 Edition of the drinking water standards and health advisories, Office of Water, Washington, DC, USA, 2011 Search PubMed.
- O. Okoro, K. Lompe, I. Papineau, M. Solliec, L. Fradette and B. Barbeau, Simultaneous powdered activated carbon and coagulant injection during ballasted flocculation for trace benzene removal from diesel and gasoline-contaminated surface waters, J. Water Process. Eng., 2021, 40, 101846 CrossRef.
- R. Galvez-Cloutier, G. Guesdon and A. Fonchain, Lac-Mégantic: analyse de l'urgence environnementale, bilan et évaluation des impacts, Can. J. Civ. Eng., 2014, 41, 531–539 CrossRef.
-
K. C. Lee, M. Boufadel, B. Chen, J. Foght, P. Hodson, S. Swanson and A. Venosa, Expert Panel Report on the Behaviour and Environmental Impacts of Crude Oil Released into Aqueous Environments, Royal Society of Canada, Ottawa, ON, 2015 Search PubMed.
-
M. Fingas and M. Fingas, Oil Spill Science and Technology, Elsevier Science & Technology, Saint Louis, UNITED STATES, 2010 Search PubMed.
-
Health Canada, Guidelines for Canadian Drinking Water Quality: Guideline Technical Document—Benzene. Water, Air and Climate Change Bureau, Healthy Environments and Consumer Safety Branch, Health Canada, Ottawa, Ontario, 2009 (Catalogue No. H128-1/09-589E) Search PubMed.
- A. A. M. Daifullah and B. S. Girgis, Impact of surface characteristics of activated carbon on adsorption of BTEX, Colloids Surf., A, 2003, 214, 181–193 CrossRef CAS.
- I. N. Najm, V. L. Snoeyink, B. W. Lykins Jr. and J. Q. Adams, Using powdered activated carbon: A critical review, J. - Am. Water Works Assoc., 1991, 83, 65–76 CrossRef CAS.
- A. H. Sobrinho Jose, T. Thiem Leon and A. Alkhatib Eid, Benzene Removal by PAC in Jet Flocculation System, J. Environ. Eng., 1997, 123, 1011–1018 CrossRef.
-
J. C. Crittenden, J. H. Borchardt and W. H. Montgomery, MWH's water treatment: Principles and design, John Wiley & Sons, Hoboken, N.J., 2012 Search PubMed.
- F. Juttner and S. B. Watson, Biochemical and ecological control of geosmin and 2-methylisoborneol in source waters, Appl. Environ. Microbiol., 2007, 73, 4395–4406 CrossRef CAS PubMed.
- J. J. Ridal, B. Brownlee and D. R. S. Lean, Is Lake Ontario the Source of Taste and Odor Compounds to the Upper St. Lawrence River?, J. Great Lakes Res., 2000, 26, 315–322 CrossRef CAS.
- J. Parinet, M. J. Rodriguez and J. Sérodes, Influence of water quality on the presence of off-flavour compounds (geosmin and 2-methylisoborneol), Water Res., 2010, 44, 5847–5856 CrossRef CAS.
- M. Pirbazari, H. S. Borow, S. Craig, V. Ravindran and M. J. McGuire, Physical Chemical Characterization of Five Earthy-Musty-Smelling Compounds, Water Sci. Technol., 1992, 25, 81–88 CrossRef CAS.
- P. Ömür-Özbek and A. M. Dietrich, Determination of Temperature-Dependent Henry's Law Constants of Odorous Contaminants and Their Application to Human Perception, Environ. Sci. Technol., 2005, 39, 3957–3963 CrossRef.
- D. Benanou, F. Acobas and M.-R. de Roubin, Optimization of stir bar sorptive extraction applied to the determination of odorous compounds in drinking water, Water Sci. Technol., 2004, 49, 161–170 CrossRef CAS.
- S. A. Huber, A. Balz, M. Abert and W. Pronk, Characterisation of aquatic humic and non-humic matter with size-exclusion chromatography – organic carbon detection – organic nitrogen detection (LC-OCD-OND), Water Res., 2011, 45, 879–885 CrossRef CAS PubMed.
- S. Nakamura, N. Nakamura and S. Ito, Determination of 2-methylisoborneol and geosmin in water by gas chromatography-mass spectrometry using stir bar sorptive extraction, J. Sep. Sci., 2001, 24, 674–677 CrossRef CAS.
- A. Prieto, O. Basauri, R. Rodil, A. Usobiaga, L. A. Fernández, N. Etxebarria and O. Zuloaga, Stir-bar sorptive extraction: A view on method optimisation, novel applications, limitations and potential solutions, J. Chromatogr. A, 2010, 1217, 2642–2666 CrossRef CAS PubMed.
- Y.-H. Sung, T.-Y. Li and S.-D. Huang, Analysis of earthy and musty odors in water samples by solid-phase microextraction coupled with gas chromatography/ion trap mass spectrometry, Talanta, 2005, 65, 518–524 CrossRef CAS.
- E. Guibelin, F. Delsalle and P. Binot, The ACTIFLO® Process: A Highly Compact And Efficient Process To Prevent Water Pollution By Stormwater Flows, Water Sci. Technol., 1994, 30, 87–96 CrossRef.
- G. Chen, B. W. Dussert and I. H. Suffet, Evaluation of granular activated carbons for removal of methylisoborneol to below odor threshold concentration in drinking water, Water Res., 1997, 31, 1155–1163 CrossRef.
- D. Cook, G. Newcombe and P. Sztajnbok, The application of powdered activated carbon for MIB and geosmin removal: predicting PAC doses in four raw waters, Water Res., 2001, 35, 1325–1333 CrossRef.
- G. Newcombe, J. Morrison and C. Hepplewhite, Simultaneous adsorption of MIB and NOM onto activated carbon. I. Characterisation of the system and NOM adsorption, Carbon, 2002, 40, 2135–2146 CrossRef.
- G. Newcombe and D. Cook, Influences on the removal of tastes and odours by PAC, J. Water Supply: Res. Technol.--AQUA, 2002, 51, 463–474 CrossRef CAS.
- J. Yu, M. Yang, T.-F. Lin, Z. Guo, Y. Zhang, J. Gu and S. Zhang, Effects of surface characteristics of activated carbon on the adsorption of 2-methylisobornel (MIB) and geosmin from natural water, Sep. Purif. Technol., 2007, 56, 363–370 CrossRef CAS.
- F. Zietzschmann, J. Altmann, A. S. Ruhl, U. Dunnbier, I. Dommisch, A. Sperlich, F. Meinel and M. Jekel, Estimating organic micro-pollutant removal potential of activated carbons using UV absorption and carbon characteristics, Water Res., 2014, 56, 48–55 CrossRef CAS PubMed.
-
V. L. Snoeyink and R. S. Summers, in Water quality and treatment: A handbook of community water supplies, American Water Work Association, 5th edn, 1999, pp. 13.11–13.83 Search PubMed.
- G. Newcombe, M. Drikas and R. Hayes, Influence of characterised natural organic material on activated carbon adsorption: II. Effect on pore volume distribution and adsorption of 2-methlylisobornoel, Water Res., 1997, 31, 1065–1073 CrossRef CAS.
-
J. Croft, Characterization of Natural Organic Mater removal through full scale drinking water treatment; implications for membrane fouling control, MSc, University of Waterloo, 2012 Search PubMed.
- S. A. Baghoth, S. K. Sharma, M. Guitard, V. Heim, J. P. Croué and G. L. Amy, Removal of NOM-constituents as characterized by LC-OCD and F-EEM during drinking water treatment, J. Water Supply: Res. Technol.--AQUA, 2011, 60, 412–424 CrossRef CAS.
- M. A. El-Dib, A. S. Moursy and M. I. Badawy, Role of adsorbents in the removal of soluble aromatic hydrocarbons from drinking waters, Water Res., 1978, 12, 1131–1137 CrossRef.
|
This journal is © The Royal Society of Chemistry 2022 |