Application of in vitro bioassays for water quality monitoring in three drinking water treatment plants using different treatment processes including biological treatment, nanofiltration and ozonation coupled with disinfection†
Received
6th November 2019
, Accepted 12th December 2019
First published on 13th December 2019
Abstract
Surface waters feeding water treatment plants (WTPs) can contain organic micropollutants, which are typically removed during treatment, while disinfection by-products (DBPs) can form after disinfection. The complex mixtures of chemicals in drinking water imply that targeted chemical analysis cannot capture all chemicals present, though in vitro bioassays can be applied alongside chemical analysis to monitor the total chemical burden. The current study applied bioassays indicative of hormone receptor-mediated effects to evaluate micropollutant removal during treatment, while bioassays indicative of adaptive stress responses and mutagenicity were applied to assess DBP formation. Water was extracted with solid-phase extraction from three WTPs using different treatment processes including biological treatment, nanofiltration and ozonation. Of the studied hormone receptors, only estrogenic activity was detected in the source waters feeding the WTPs, with all treatment processes able to remove estrogenic activity in the produced water completely or just above the detection limit. The oxidative stress response and NF-κB response for inflammation were detected in both source and treated water samples, with formed DBPs contributing to the increase in oxidative stress response. None of the samples induced the p53 response for genotoxicity or had a response in the Ames mutagenicity assay. The effects in the produced water were compared to effect-based trigger values (EBT) for activation of estrogenic activity and oxidative stress response, with the observed effect over 10 times lower than the available EBTs. This emphasises the high quality of the produced drinking water and the value of applying in vitro bioassays for water quality monitoring.
Water impact
Source waters feeding water treatment plants (WTPs) contain complex mixtures of micropollutants. The treatment efficacy for removal of toxicity in three WTPs applying different treatment processes was assessed using in vitro bioassays. Despite high effects in source waters, the produced waters had low effects that were below effect-based trigger values, emphasising the utility of applying bioassays for water quality monitoring.
|
1. Introduction
Around 40% of Europe's drinking water is sourced from surface waters,1 but surface water quality can be negatively impacted by human activities related to urbanisation, wastewater effluent discharge and agricultural run-off.2 As a result, micropollutants, such as pesticides, pharmaceuticals and industrial compounds, have been detected in both source water and treated drinking water.3,4 Due to the varying quality of source water, effective treatment processes are required to ensure safe drinking water. Chemical analysis is typically applied to monitor drinking water quality, but there is increasing interest in using in vitro bioassays complementary to chemical analysis.5In vitro bioassays detect the effect of all active known and unknown chemicals in a sample. This is relevant for drinking water where chemicals are often present at low concentrations, potentially below analytical detection limits, but the mixture effects of the many chemicals present at trace levels may still be significant.6
Several studies have applied bioassays indicative of induction of xenobiotic metabolism,7 receptor-mediated effects,8,9 adaptive stress responses10,11 and reactive modes of action12 to assess drinking water quality, though estrogenic activity is the most commonly studied endpoint. Most studies reported decreased estrogenic activity after drinking water treatment, with either no or low estrogenic activity in treated water,8,13 though Rosenmai et al.14 found no change in estrogenic activity in one water treatment plant (WTP). In contrast, mutagenicity and adaptive stress responses, such as the oxidative stress response, often increase after drinking water treatment.10,12,15,16 This is attributed to the formation of disinfection by-products (DBPs) from the reaction of disinfectants, such as chlorine, with natural organic matter and inorganic ions, such as bromide and iodide.17 Unlike chemical analysis, which provides information about the individual chemicals present in a sample, bioassays respond to all active chemicals and cannot distinguish between micropollutants and DBPs. However, Hebert et al.10 compared the effect before and after chlorination to determine what fraction of the oxidative stress response was due to DBP formation, with DBPs explaining up to 58% of the oxidative stress response.
To date, most of the studies focusing on drinking water have considered a single endpoint or several endpoints from the same stage of the cellular toxicity pathway (e.g. hormone receptor-mediated effects). However, bioassay test batteries indicative of different stages of cellular toxicity pathways are recommended for monitoring water quality and assessing treatment efficacy.18 In the current study, we applied eight bioassays indicative of seventeen endpoints to evaluate the chemical burden and treatment efficacy in three WTP in the Paris area, France, over four seasons. The bioassay test battery included assays indicative of hormone receptor-mediated effects, namely activation and inhibition of the estrogen receptor (ER), androgen receptor (AR), glucocorticoid receptor (GR) and progesterone receptor (PR). Three assays indicative of adaptive stress responses were included, specifically the AREc32 assay for Nrf2-mediated oxidative stress response, the NF-κB GeneBLAzer assay for NF-κB response for inflammation and the p53RE GeneBLAzer assay for p53 response for genotoxicity. These assays all use human cell lines, which have greater relevance for human health, though the commonly used bacterial Ames fluctuation test for mutagenicity was also applied to detect reactive modes of action. Water samples were collected throughout the treatment trains of the studied WTPs, as well as from the source waters feeding the WTPs. The results were compared with a previous study that exclusively used mammalian adaptive stress response assays to assess effects in the distribution system of the same three studied WTPs.10 The detected effects were compared with available effect-based trigger values (EBTs) from the literature. The EBTs were derived by reading across from existing chemical drinking water guideline values and can be used to determine whether a response in a bioassay is acceptable or unacceptable.10
2. Materials and methods
2.1. Sample collection
Water samples were collected from three WTPs, Méry-sur-Oise, Choisy-le-Roi and Neuilly-sur-Marne, in the greater Paris area in May, July, October and December 2018 (Fig. 1). At the Méry-sur-Oise WTP, water from the Oise River was treated using nanofiltration (70%) and conventional biological treatment (30%), with the water from the two treatment trains mixed together before chlorination. Water samples were collected from the source water, after nanofiltration, after biological treatment and after chlorination. The Choisy-le-Roi and Neuilly-sur-Marne WTPs apply conventional treatment with pre-ozonation (Choisy-le-Roi WTP only), clarification, sand filtration, ozonation, granular activated carbon, UV and chlorination to treat water from the Seine River and Marne River, respectively. Water samples were collected from the source water, after UV treatment and after chlorination in the Choisy-le-Roi and Neuilly-sur-Marne WTPs. Further information about the treatment processes is available in Hebert et al.10 Water quality parameters for the source water and produced water, including temperature, total organic carbon (TOC), conductivity and residual free chlorine, are provided in Tables S1 and S2 of the ESI.† Two litres of water were collected per sampling site in May and July, while duplicate 2 L samples were collected in October and December. Twenty milligrams per litre of sodium thiosulfate was added to each sample after collection to neutralise the free chlorine.
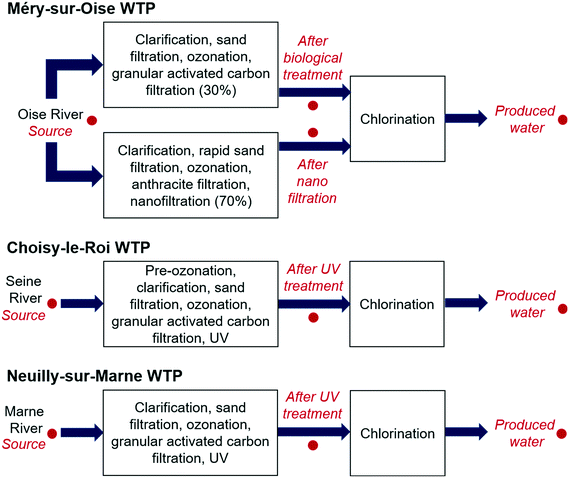 |
| Fig. 1 Treatment processes at the three studied water treatment plants (WTP), with the sampling locations indicated in red. | |
2.2. Sample extraction for bioanalysis
The water samples were extracted using solid-phase extraction (SPE), with 2 L of water enriched using 500 mg Oasis HLB SPE cartridges. The cartridges were eluted using 20 mL of methanol and 10 mL of methyl tertbutyl ether (MTBE). The solvent extracts were blown to dryness and then resolubilised in 1 mL of methanol, giving an enrichment factor (EF) of 2000. Glass bottled Evian water with and without sodium thiosulfate was also enriched by SPE and served as controls in the bioassays. It is important to note that SPE will only enrich non-volatile chemicals, so the effect of any volatile chemicals will not be captured in the bioassays. More information about sample extraction can be found in Hebert et al.10
2.3. Bioassays
Details about the applied bioassays are provided in Table 1. All cell-based bioassays have been used previously for water quality monitoring, with the methods fully described in König et al.19 and Neale et al.18 All samples were run in ERα GeneBLAzer and the adaptive stress response assays, but due to the limited sample volume, the non-responsive endpoints were split and the May and June samples were run in AR GeneBLAzer, GR GeneBLAzer and PR GeneBLAzer, while the Ames assay was performed with the samples from October and December. This approach allowed higher sample volumes to be dosed to assure that the negative responses in these assays were not false negatives due to insufficient enrichment. To prevent any solvent effects, all methanolic water extracts were blown to dryness and redissolved in assay media before bioanalysis. Cell viability was measured in parallel using the IncuCyte S3 live cell imaging system (Essen BioScience, Ann Arbor, Michigan, USA) as described in Nivala et al.20 Cell viability was measured based on confluency, so this approach was not suitable for the NF-κB GeneBLAzer assay, which uses a suspension cell line. Cytotoxicity generally differs very little between cell lines21 and therefore it is justified to use the cytotoxicity from an adherent cell line as a proxy for a suspension cell line. Therefore, cell viability data from the AREc32 assay was used to exclude likely cytotoxic concentrations in the NF-κB GeneBLAzer assay. The bacterial Ames fluctuation test using Salmonella typhimurium test strains TA98, TA100 and YG7108 was run based on the method outlined in Reifferscheid et al.22 with some modifications. Firstly, cytotoxicity of the water extracts was assessed independently for TA98 by measuring the growth rate via optical density at 600 nm after 0, 30, 60, 90 and 120 min in a 96 well plate. The growth rate μ for exponential growth was determined by plotting the OD600nm,t/OD600nm,t=0 as a function of time and deriving μ from the slope of the linear regression using eqn (1), with cytotoxicity calculated using eqn (2). | 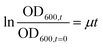 | (1) |
| 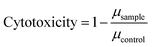 | (2) |
Only non-cytotoxic concentrations were evaluated in the Ames fluctuation test for mutagenic potential. Briefly, samples were serially diluted and each concentration was exposed in four replicates with or without S9 at 0.15 mgprotein mL−1 to S. typhimurium TA98, TA100 and YG7108 for 100 min at 37 °C in a 384 well plate. The incubated samples were then transferred with a 384-tip pipette head (Hamilton Star, Bonaduz, Switzerland) to twelve 384-well plates containing reversion indicator medium (leading to 48 replicates per tested concentration) and incubated for a further 48 h at 37 °C for TA98 and TA100 and 72 h for YG7108. The number of revertants per concentration for each sample was determined by measuring optical density at 414 nm, with a maximum of 48 revertants per concentration, and converted to % revertants. The source and produced water extracts from October were run in the TA98 and TA100 strains, while all samples from October and December were run in YG7108.
2.4. Data evaluation
Cytotoxicity was calculated from cell viability in the mammalian cell lines based on the approach outlined in Escher et al.21 The concentration causing 10% inhibition (IC10) was calculated using linear concentration-effect curves and any concentrations causing greater than 10% cytotoxicity were excluded from further data evaluation. A stricter cytotoxicity cut-off of 1% (IC01) was set for assays indicative of inhibition of hormone receptors as antagonism cannot be differentiated from cytotoxicity.20
Table 1 Overview of bioassays applied in the current study
Endpoint |
Assay |
Method reference |
Positive reference compound |
EC value |
Positive reference compound EC value (M) |
Positive reference compound EC value (ng L−1) |
Nivala et al.;20 SE: standard error; CI: confidence interval.
|
Hormone receptor-mediated effects
|
|
Activation of ER |
ERα GeneBLAzer |
König et al.19 |
17β-Estradiol |
EC10 ± SE |
(1.60 ± 0.06) × 10−11 |
(4.36 ± 0.15) × 100 |
Inhibition of ER |
ERα GeneBLAzer |
König et al.19 |
Tamoxifen |
ECSR0.2 ± SE |
(5.86 ± 3.67) × 10−6 |
(2.18 ± 1.36) × 106 |
Activation of AR |
AR GeneBLAzer |
König et al.19 |
R1881 (metribolone) |
EC10 ± SE |
(4.10 ± 0.43) × 10−11a |
(1.17 ± 0.12) × 101a |
Inhibition of AR |
AR GeneBLAzer |
König et al.19 |
Cyproterone acetate |
ECSR0.2 ± SE |
(1.40 ± 0.15) × 10−8 |
(5.85 ± 0.61) × 103 |
Activation of GR |
GR GeneBLAzer |
König et al.19 |
Dexamethasone |
EC10 ± SE |
(3.48 ± 0.44) × 10−10 |
(1.37 ± 0.17) × 102 |
Inhibition of GR |
GR GeneBLAzer |
König et al.19 |
RU486 (mifepristone) |
ECSR0.2 ± SE |
(1.15 ± 0.12) × 10−10 |
(4.93 ± 0.49) × 101 |
Activation of PR |
PR GeneBLAzer |
König et al.19 |
Promegestone |
EC10 ± SE |
(7.61 ± 0.28) × 10−11 |
(2.48 ± 0.09) × 101 |
Inhibition of PR |
PR GeneBLAzer |
König et al.19 |
RU486 |
ECSR0.2 ± SE |
(9.41 ± 1.50) × 10−12 |
(4.04 ± 0.64) × 100 |
Adaptive stress responses
|
|
Oxidative stress response |
AREc32 |
Wang et al.45 |
tert-Butyl hydroquinone (tBHQ) |
ECIR1.5 ± SE |
(3.09 ± 0.06) × 10−6 |
(5.13 ± 0.10) × 105 |
Escher et al.46 |
p53 response |
p53RE GeneBLAzer |
König et al.19 |
Mitomycin |
ECIR1.5 ± SE |
(1.54 ± 0.10) × 10−7 |
(5.15 ± 0.33) × 104 |
NF-κB response |
NF-κB GeneBLAzer |
König et al.19 |
Tumor necrosis factor alpha (TNFα) |
ECIR1.5 ± SE |
— |
(1.29 ± 0.05) × 101 |
Reactive mode of action
|
|
Mutagenicity (TA98 −S9) |
Ames fluctuation test |
Reifferscheid et al.22 |
4-Nitro-O-phenylenediamine |
EC50 (95% CI) |
6.02(5.21–7.00) × 10−5 |
0.92(0.80–1.07) × 101 mg L−1 |
Mutagenicity (TA98 +S9) |
2-Aminoanthracene |
EC50 (95% CI) |
1.03(0.90–1.16) × 10−6 |
1.99(1.75–2.25) × 10−1 mg L−1 |
Mutagenicity (TA100 −S9) |
Nitrofurantoin |
EC50 (95% CI) |
5.49(3.99–8.22) × 10−7 |
1.31(0.95–1.96) × 10−1 mg L−1 |
Mutagenicity (TA100 +S9) |
2-Aminoanthracene |
EC50 (95% CI) |
2.51(2.22–2.83) × 10−6 |
4.84(4.29–5.46) × 10−1 mg L−1 |
Mutagenicity (YG7108 −S9) |
N-Nitrosodimethylamine (NDMA) |
EC50 (95% CI) |
1.07(0.93–1.26) × 10−2 |
7.95(6.88–9.31) × 102 mg L−1 |
Mutagenicity (YG7108 +S9) |
NDMA |
EC50 (95% CI) |
1.57(1.38–1.79) × 10−4 |
1.16(1.02–1.33) × 101 mg L−1 |
Linear concentration-effect curves up to 30% effect were used to determine the effect concentration causing 10% effect (EC10) for assays indicative of activation of hormone receptors, while the effect concentration causing a suppression ratio of 0.2 (ECSR0.2) was calculated for assays indicative of inhibition of hormone receptors. The adaptive stress response assays do not reach a maximum effect, so the response was expressed as an induction ratio (IR) relative to the control. Linear concentration-effect curves up to an IR of 4 were used to determine the effect concentration causing an induction ratio of 1.5 (ECIR1.5). For the Ames assay, the validity of the test was assessed according to ISO 1135023 with 10 out of 48 wells (20%) with revertant growth considered negative. In the present test set-up, the resolution was much higher, so that typically as low as 5 to 10% effect could be differentiated from the controls. Therefore, log-sigmodal concentration-effect curves were used to determine the concentration causing 50% effect (EC50) for the Ames assay. Further information about the applied data evaluation approach can be found in Neale et al.18 and Escher et al.24 The duplicate samples from October and December were evaluated together, giving a single EC value for each sample because the differences were minimal.
The EC values were expressed in units of relative enrichment factor (REF), which considers the sample EF and the dilution factor in the bioassays. For example, an EC value of REF 10 indicates that a sample needs to be enriched 10 times before an effect is observed. The EC value was translated into a bioanalytical equivalent concentration (BEQbio), which converts the response in a sample to the concentration of a reference compound (ref) that would have the same effect (eqn (3)).
| 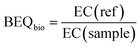 | (3) |
For the estrogenicity assay the reference compound is 17β-estradiol and hence the BEQ
bio is termed estradiol equivalent concentration, EEQ
bio. The BEQ
bio for the oxidative stress response assay was expressed as a
tert-butyl hydroquinone (
tBHQ) equivalent concentration (
tBHQ-EQ
bio), while tumor necrosis factor alpha (TNFα) equivalent concentration (TNFα-EQ
bio) was used for the NF-κB response assay.
3. Results and discussion
3.1. Hormone receptor-mediated effects
Estrogenic activity was detected in all source water samples using the ERα GeneBLAzer assay, with effects detected after 1.1 to 26 times enrichment (Table S3† and Fig. 2). Example concentration-effect curves from Choisy-le-Roi in May 2018 are shown in Fig. S1.† Source water from the Marne River had the greatest effect in all four sampling campaigns, followed by the Seine River, while the water feeding the Méry-sur-Oise WTP had the lowest effect. The source water for the Méry-sur-Oise WTP is a natural reservoir fed by the Oise River, with no recreational activities, such as boating or swimming, permitted. Consequently, the detected effects were rather low. For Neuilly-sur-Marne and Choisy-le-Roi, the effect in the source water was highest in May. The flow rates of the Marne and Seine rivers were over twice as high in May than the other months (Table S1†). The May sampling campaign followed a long flooding period, explaining the higher flow rates.
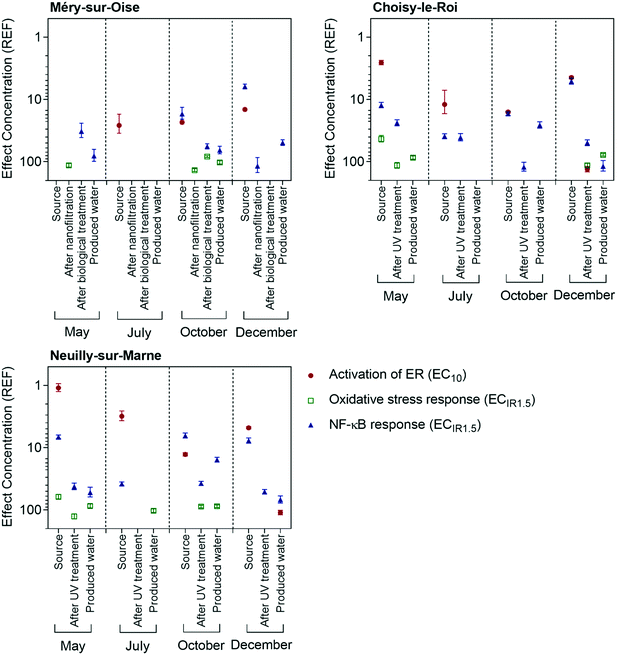 |
| Fig. 2 Comparison of effect concentrations EC for activation of ER (EC10, closed red circles), oxidative stress response (ECIR1.5, open green squares) and NF-κB response (ECIR1.5, open blue triangles) in units of relative enrichment factor (REF) in Méry-sur-Oise, Choisy-sur-Roi and Neuilly-sur-Marne. Note the scale is logarithmic and inverse, because a low EC indicates a high effect. | |
When expressed in units of EEQbio, the effect in the source water ranged from 0.17 to 3.98 ngE2 L−1 (Table 2). This is within a similar range as previously measured in source water feeding Paris WTPs (0.7 to 1.8 ngE2 L−1).8 The estrogenic activity in the source water in the current study is higher than previously measured in the US (0.044 to 0.47 ngE2 L−1),13 though much lower than detected in source water in China (8.00 to 129 ngE2 L−1).7 The detected effect was also similar to effects measured in Australian surface waters from urban and agricultural areas (0.1 to 1.18 ng L−1) using the ERα GeneBLAzer assay.25
Table 2 BEQbio values for the studied bioassays
WTP |
Méry-sur-Oise |
Choisy-le-Roi |
Neuilly-sur-Marne |
Sample type |
Source |
After nano filtration |
After biological treatment |
Produced water |
Source |
After UV treatment |
Produced water |
Source |
After UV treatment |
Produced water |
|
Activation of ER (EEQbio (ngE2 L−1))
|
May |
N/A |
<4.00 × 10−2 |
Cytotoxic |
<4.00 × 10−2 |
(1.70 ± 0.15) × 100 |
<4.00 × 10−2 |
<4.00 × 10−2 |
(3.98 ± 0.61) × 100 |
<4.00 × 10−2 |
<4.00 × 10−2 |
July |
(1.68 ± 0.57) × 10−1 |
<4.00 × 10−2 |
Cytotoxic |
<4.00 × 10−2 |
(3.64 ± 1.49) × 10−1 |
<4.00 × 10−2 |
<4.00 × 10−2 |
(1.40 ± 0.25) × 100 |
<4.00 × 10−2 |
<4.00 × 10−2 |
October |
(1.88 ± 0.14) × 10−1 |
<3.00 × 10−2 |
<3.00 × 10−2 |
<3.00 × 10−2 |
(2.47 ± 0.14) × 10−1 |
<3.00 × 10−2 |
<3.00 × 10−2 |
(3.41 ± 0.25) × 10−1 |
<3.00 × 10−2 |
<3.00 × 10−2 |
December |
(3.04 ± 0.18) × 10−1 |
<3.00 × 10−2 |
N/A |
<3.00 × 10−2 |
(9.80 ± 0.57) × 10−1 |
(3.31 ± 0.35) × 10−2 |
<3.00 × 10−2 |
(9.12 ± 0.51) × 10−1 |
<3.00 × 10−2 |
(3.96 ± 0.35) × 10−2 |
|
Oxidative stress response (tBHQ-EQbio (ngtBHQ L−1))
|
May |
N/A |
(4.50 ± 0.31) × 103 |
<5.14 × 103 |
<5.14 × 103 |
(1.19 ± 0.14) × 104 |
(4.48 ± 0.48) × 103 |
(5.98 ± 0.40) × 103 |
(8.35 ± 0.69) × 103 |
(4.04 ± 0.38) × 103 |
(5.96 ± 0.45) × 103 |
July |
Cytotoxic |
<5.14 × 103 |
<5.14 × 103 |
<5.14 × 103 |
Cytotoxic |
<5.14 × 103 |
<5.14 × 103 |
<5.14 × 103 |
<5.14 × 103 |
(5.00 ± 0.36) × 103 |
October |
Cytotoxic |
(3.77 ± 0.22) × 103 |
(6.24 ± 0.26) × 103 |
(5.01 ± 0.31) × 103 |
Cytotoxic |
<3.42 × 103 |
<3.42 × 103 |
Cytotoxic |
(5.83 ± 0.31) × 103 |
(5.88 ± 0.31) × 103 |
December |
Cytotoxic |
<3.42 × 103 |
N/A |
<3.42 × 103 |
Cytotoxic |
(4.49 ± 0.34) × 103 |
(6.62 ± 0.33) × 103 |
Cytotoxic |
<3.42 × 103 |
<3.42 × 103 |
|
NF-κB response (TNFα-EQbio (ngTNFα L−1))
|
May |
N/A |
<1.29 × 10−1 |
(3.97 ± 1.03) × 10−1 |
(1.60 ± 0.35) × 10−1 |
(1.04 ± 0.11) × 100 |
(5.40 ± 0.65) × 10−1 |
<1.29 × 10−1 |
(1.92 ± 0.15) × 100 |
(3.06 ± 0.39) × 10−1 |
(2.45 ± 0.45) × 10−1 |
July |
<1.29 × 10−1 |
<1.29 × 10−1 |
<1.29 × 10−1 |
<1.29 × 10−1 |
(3.29 ± 0.32) × 10−1 |
(3.15 ± 0.43) × 10−1 |
<1.29 × 10−1 |
(3.38 ± 0.27) × 10−1 |
<1.29 × 10−1 |
<1.29 × 10−1 |
October |
(7.58 ± 1.70) × 10−1 |
<8.57 × 10−2 |
(2.26 ± 0.23) × 10−1 |
(1.97 ± 0.28) × 10−1 |
(7.59 ± 0.56) × 10−1 |
(1.06 ± 0.18) × 10−1 |
(4.98 ± 0.63) × 10−1 |
(2.02 ± 0.20) × 100 |
(3.43 ± 0.29) × 10−1 |
(8.24 ± 0.79) × 10−1 |
December |
(2.06 ± 0.20) × 100 |
(1.10 ± 0.30) × 10−1 |
N/A |
(2.60 ± 0.29) × 10−1 |
(2.51 ± 0.30) × 100 |
(2.59 ± 0.29) × 10−1 |
(1.09 ± 0.21) × 10−1 |
(1.67 ± 0.17) × 100 |
(2.52 ± 0.23) × 10−1 |
(1.88 ± 0.26) × 10−1 |
Despite the detected estrogenic effects in the source waters, the treatment processes in all three WTPs reduced the estrogenic activity to below the limit of detection in all samples, except for the final water from Neuilly-sur-Marne in December, which had an EC10 of 110 REF. This indicates that the sample needed to be enriched 110 times to cause 10% activation of ER, which is a higher enrichment than is typically applied in most studies. The treatment efficacy of Neuilly-sur-Marne in December was 95.7% and the EEQbio value of the final water was 0.04 ngE2 L−1. The excellent treatment efficacy in the current study fits well with previous studies, with complete removal of estrogenic activity during drinking water treatment processes often observed.8,12,13
It should be noted that one control sample, bottled water with sodium thiosulfate from May, had a strong response in ERα GeneBLAzer, with an EC10 value of REF 2.34 (Table S3†). The bottled water control in July did not have an effect up to REF 100, while the same samples from October and December did not have an effect up to REF 150. Consequently, the high effect in May is a singular outlier expected to be due to sample contamination during sample enrichment or elution steps.
No other hormonal activity in AR, PR and GR was observed in any of the samples from May and July neither in agonist nor in antagonist mode (Tables S4 to S10, Fig. S2 to S8†). However, some of the samples caused cytotoxicity, particularly in antagonist mode. The lack of activity fits with the findings of previous studies on drinking water from countries including Australia, Sweden and Spain, with activation or inhibition of AR, PR and GR not commonly detected.9,14,26,27 Consequently, assays indicative of indicative of AR, PR and GR were not applied in the October and December sampling campaigns.
3.2. Adaptive stress responses
Three assays indicative of adaptive stress responses, oxidative stress response, NF-κB response for inflammation and p53 response for genotoxicity, were applied in the current study. Example concentration-effect curves are shown in Fig. S9 to S11.† Adaptive stress responses are viewed as sensitive indicators of chemical stressors as these pathways are activated in cells after damage and can either help return the cell to homeostasis or initiate apoptosis.28
The oxidative stress response in most source water samples was mostly masked by cytotoxicity, with only two of the source water samples from May active after 43 to 61 times enrichment (Fig. 2 and Table S11†). The treated and produced water samples induced a response in the oxidative stress response assay after 78 to 136 times enrichment, though several samples had no effect up to the highest tested concentrations. The produced water from Neuilly-sur-Marne and Choisy-le-Roi tended to have a greater effect in the AREc32 assay than the final water from Méry-sur-Oise. The TOC concentrations in the source waters for all three WTPs were within a similar range (Table S1†), but the treatment processes at Méry-sur-Oise removed 79 to 90% of the TOC, compared to 55 to 64% at Neuilly-sur-Marne and Choisy-le-Roi. This resulted in lower TOC concentrations in the produced water from Méry-sur-Oise (Table S2†). Organic matter is a DBP precursor and DBPs can induce the oxidative stress response,29 explaining why the effect was lower in the produced water from Méry-sur-Oise. Lundqvist et al.11 also found that treatment processes that reduced the organic carbon concentration in a pilot water treatment plant resulted in decreased oxidative stress response.
To assess the contribution of DBPs and micropollutants to the oxidative stress response, BEQbio,DBP was calculated by comparing BEQbio before chlorination (after UV treatment) and after chlorination (eqn (4)) based on the approach outlined in Hebert et al.10 All BEQbio values are provided in Table 2.
| BEQbio,DBP = BEQbio,after chlorination − BEQbio,before chlorination | (4) |
The formed DBPs explained 32 ± 8.2% and 25 ± 9.4% of the oxidative stress response in produced water from Neuilly-sur-Marne and Choisy-le-Roi, respectively, in May, while 32 ± 6.1% of the oxidative stress response in Choisy-le-Roi in December was due to DBP formation. This is within a similar range as previously observed by Hebert
et al.10 in the distribution networks of the WTPs of the current study. In contrast, less than 1% (0.84 ± 7.4%) of the oxidative stress response was attributed to DBP formation in the produced water from Neuilly-sur-Marne in October. The reason why DBP formation did not contribute to the oxidative stress response in October is not clear, with similar TOC concentrations and chlorine residuals in May, where 32% of the response was due formed DBPs. However, seasonal differences can alter the organic carbon composition,
30 potentially explaining the difference in DBP formation between May and October. Overall, effect levels are very low, hence changes are small and subject to uncertainty. Determining the contribution of DBPs to the oxidative stress response in the produced water from Méry-sur-Oise was not as straightforward as the other WTPs as the water from the nanofiltration and biological treatment trains were combined before chlorination. Assuming the mixed water contained 70% nanofiltration treated water and 30% conventional treated water, the formed DBPs contributed to 10 ± 11% of the oxidative stress response in the final water from Méry-sur-Oise in October.
The oxidative stress response in the current study was 1.5 to 2.3 times lower than the effect in samples from the same WTPs immediately after chlorination (0 h) in 2015/2016, which had an effect after 24 to 73 times enrichment.10 Despite the same treatment processes being applied, the average TOC concentration was 12–29% lower in the produced water in the current study, with the reduced TOC concentration explaining the decreased effect. Source water samples were not analysed in the previous study, but the prolonged flood period prior to the current study may have contributed to the lower TOC concentrations. While most of the source water samples were cytotoxic, thereby masking any oxidative stress response, the effect in the source water in May was similar to the oxidative stress response in surface waters from Germany31 and Switzerland.32
The NF-κB GeneBLAzer assay was more responsive than the AREc32 assay, with effects detected after 5.1 to 118 times enrichment (Fig. 2 and Table S12†). This is within a similar range as previously measured in treated drinking water in France10 and surface water from the Danube River.33 In most cases, the NF-κB response was highest in the source water and decreased with treatment, though effects were still detected in most produced water extracts. The causative compounds were well removed by nanofiltration in Méry-sur-Oise but were not removed by biological treatment in May and October, resulting in the mixed water after chlorination still having a response in the NF-κB GeneBLAzer assay. Few micropollutants activate NF-κB,34 with many inhibiting the NF-κB response.35,36 Further, commonly detected DBPs are inactive in the NF-κB GeneBLAzer assay.29 Endotoxins, which are natural complex bacterial lipopolysaccharides, are active in the NF-κB GeneBLAzer assay and can be co-extracted by SPE, with co-extracted endotoxins explaining most of the effect in surface water extracts from Australia.37 While treatment processes such as sand filtration and ozonation are expected to reduce the endotoxin concentration, biological treatment can increase the endotoxin levels in water.38 Therefore, the observed NF-κB response may be due to co-extracted endotoxins, though further testing is needed to confirm this hypothesis.
While some of the source water samples were cytotoxic in the p53RE GeneBLAzer assay for genotoxicity (Table S13†), none of the treated samples induced a response up to a REF of 100 (May, July) and 150 (October, December). This emphasizes the high quality of the treated water and fits with previous observations for drinking water from these WTPs.10
3.3. Mutagenicity
None of the source or produce water samples from October inhibited growth in TA98 (Fig. S12†) and therefore the Ames assay was performed at REF up to 200. All positive controls gave valid responses (Fig. S13†). Source and produced water from October were run in TA98 and TA100, while all samples from October and December were run in YG7108. However, none of the samples showed any mutagenic response in any of the three investigated Ames strains, S. typhimurium TA98, TA100 and YG7108, up to REF 200 with and without metabolic activation (Fig. S14†). The lack of mutagenicity observed in the current study fits with a study by Guzzella et al.,39 who did not detect any response using the S. typhimurium TA98 and TA100 strains before and after disinfection of surface water from Italy. In contrast, Heringa et al.40 observed an increase in mutagenicity in drinking water collected from the Netherlands and the US after UV/hydrogen peroxide treatment using the TA98 strain, but the effect was removed after granular activated carbon post-treatment. Further, drinking water from Australia had an ECIR1.5 value ranging from REF 3.2 to 5 in S. typhimurium TA98 and TA100 strains in Escher et al.,27 though many of the other water samples, including surface water, also had a response. The lack of response in the Ames assay in the current study further highlights the high quality of the produced water. However, it should be noted that the applied SPE method enriches ionized DBPs, such as haloacetic acids, with a low yield only,41 potentially contributing to the lack of mutagenicity observed.
3.4. Comparison with available effect-based trigger values
The EEQbio values for source water in the ERα GeneBLAzer assay were compared with a proposed assay-specific EBT for surface water derived using environmental quality standards (EQS) from the European Water Framework Directive (WFD).42 All source water samples from the Neuilly-sur-Marne and Choisy-le-Roi WTPs exceeded the proposed EBT for estrogenicity of 0.34 ngE2 L−1, with the exception of the Choisy-le-Roi source water sample in October. The water feeding the Méry-sur-Oise WTP was already below the EBT in all sampling campaigns. All treatment processes effectively reduced EEQbio, with only the produced water from Neuilly-sur-Marne in December active. The EEQbio value, 0.04 ngE2 L−1, was 45 times lower than the proposed drinking water EBT for ERα GeneBLAzer of 1.8 ngE2 L−1.43 This EBT was derived from the Australian Drinking Water Guidelines (ADWG) and the Australian Guidelines for Water Recycling (AGWR) for augmentation of drinking water supplies, so is not specific to Europe.
The oxidative stress response in the produced water from the three plants was compared with the proposed AREc32 EBT for drinking water, ECIR1.5 of REF 6,44 which was also derived from Australian drinking water guidelines. A low EC value indicates a greater effect than a high EC value, which can cause confusion, so the EBT was converted to tBHQ-EQbio using the ECIR1.5 value of tBHQ from the current study. The EBT of ECIR1.5 of REF 6 gave a tBHQ-EQbio of 85.5 μg L−1 (85
526 ng L−1), which was between 13 to 17 times higher than the tBHQ-EQbio values of the produced water samples (Table 2). While the proposed EBTs are still considered preliminary at this stage, the large difference between the effect detected in the produced water and the EBTs emphasises the high quality of the final water.
4. Conclusions
The presence of a complex cocktail of micropollutants in source water as well as the formation of DBPs during disinfection means that targeted chemical analysis alone is unable to effectively monitor the chemical burden in drinking water. In the current study, a bioassay test battery indicative of different modes of action was applied to evaluate treatment efficacy and DBP formation in three WTPs over four seasons. Despite the poor quality of the source water, drinking water treatment processes were able to remove estrogenic activity, with the effect in all but one of the produced waters below the detection limit. The effect in the one active produced water sample was close to the detection limit. While the formation of DBPs contributed to the oxidative stress response in May and October, the oxidative stress response in the produced waters was low due to the low TOC concentrations. The high quality of the produced water was emphasised by lack of mutagenic effects quantified with the Ames assay and by comparison with available EBTs, with the effects in the produced waters over an order of magnitude lower than the proposed drinking water EBTs. Consequently, the current study highlights the value of applying in vitro bioassays for monitoring drinking water quality.
Conflicts of interest
Cedric Feliers and Caroline Lecarpentier declare that they are employees of Veolia Eau d'Ile de France.
Acknowledgements
This study was funded by Veolia Eau d'Ile de France. We thank Jenny John (UFZ) for experimental assistance. Delphine Brillant, Jonathan Coulmin and Valérie Ingrand (all Veolia R&I Environment & Health Department, Chemical Analysis and Mechanisms Unit) are thanked for water sample extraction.
References
-
European Environmental Agency, Quality of Europe's water for people's use has improved, but challenges remain to keep it clean and healthy, https://www.eea.europa.eu/highlights/quality-of-europes-water-for, (accessed 17th April 2019) Search PubMed.
- A. Pal, K. Y. H. Gin, A. Y. C. Lin and M. Reinhard, Impacts of emerging organic contaminants on freshwater resources: Review of recent occurrences, sources, fate and effects, Sci. Total Environ., 2010, 408, 6062–6069 CrossRef CAS PubMed.
- S. T. Glassmeyer, E. T. Furlong, D. W. Kolpin, A. L. Batt, R. Benson, J. S. Boone, O. Conerly, M. J. Donohue, D. N. King, M. S. Kostich, H. E. Mash, S. L. Pfaller, K. M. Schenck, J. E. Simmons, E. A. Varughese, S. J. Vesper, E. N. Villegas and V. S. Wilson, Nationwide reconnaissance of contaminants of emerging concern in source and treated drinking waters of the United States, Sci. Total Environ., 2017, 581, 909–922 CrossRef PubMed.
- R. Tröger, P. Klöckner, L. Ahrens and K. Wiberg, Micropollutants in drinking water from source to tap - Method development and application of a multiresidue screening method, Sci. Total Environ., 2018, 627, 1404–1432 CrossRef PubMed.
- P. A. Neale and B. I. Escher,
In vitro bioassays to assess drinking water quality, Curr. Opin. Environ. Sci. Health, 2019, 7, 1–7 CrossRef.
- E. Silva, N. Rajapakse and A. Kortenkamp, Something from "nothing" - Eight weak estrogenic chemicals combined at concentrations below NOECs produce significant mixture effects, Environ. Sci. Technol., 2002, 36, 1751–1756 CrossRef CAS PubMed.
- P. Shi, S. C. Zhou, H. X. Xiao, J. F. Qiu, A. M. Li, Q. Zhou, Y. Pan and H. Hollert, Toxicological and chemical insights into representative source and drinking water in eastern China, Environ. Pollut., 2018, 233, 35–44 CrossRef CAS PubMed.
- M. L. Jugan, L. Oziol, M. Bimbot, V. Huteau, S. Tamisier-Karolak, J. P. Blondeau and Y. Levi,
In vitro assessment of thyroid and estrogenic endocrine disruptors in wastewater treatment plants, rivers and drinking water supplies in the greater Paris area (France), Sci. Total Environ., 2009, 407, 3579–3587 CrossRef CAS PubMed.
- Y. Valcárcel, A. Valdehíta, E. Becerra, M. López de Alda, A. Gil, M. Gorga, M. Petrovic, D. Barceló and J. M. Navas, Determining the presence of chemicals with suspected endocrine activity in drinking water from the Madrid region (Spain) and assessment of their estrogenic, androgenic and thyroidal activities, Chemosphere, 2018, 201, 388–398 CrossRef PubMed.
- A. Hebert, C. Feliers, C. Lecarpentier, P. A. Neale, R. Schlichting, S. Thibert and B. I. Escher, Bioanalytical assessment of adaptive stress responses in drinking water: A predictive tool to differentiate between micropollutants and disinfection by-products, Water Res., 2018, 132, 340–349 CrossRef CAS PubMed.
- J. Lundqvist, A. Andersson, A. Johannisson, E. Lavonen, G. Mandava, H. Kylin, D. Bastviken and A. Oskarsson, Innovative drinking water treatment techniques reduce the disinfection-induced oxidative stress and genotoxic activity, Water Res., 2019, 155, 182–192 CrossRef CAS PubMed.
- S. H. Xiao, X. M. Lv, Y. F. Zeng, T. Jin, L. Luo, B. B. Zhang, G. Zhang, Y. H. Wang, L. Feng, Y. Zhu and F. Tang, Mutagenicity and estrogenicity of raw water and drinking water in an industrialized city in the Yangtze River Delta, Chemosphere, 2017, 185, 647–655 CrossRef CAS PubMed.
- J. M. Conley, N. Evans, H. Mash, L. Rosenblum, K. Schenck, S. Glassmeyer, E. T. Furlong, D. W. Kolpin and V. S. Wilson, Comparison of in vitro estrogenic activity and estrogen concentrations in source and treated waters from 25 US drinking water treatment plants, Sci. Total Environ., 2017, 579, 1610–1617 CrossRef CAS PubMed.
- A. K. Rosenmai, J. Lundqvist, T. le Godec, A. Ohisson, R. Troger, B. Hellman and A. Oskarsson,
In vitro bioanalysis of drinking water from source to tap, Water Res., 2018, 139, 272–280 CrossRef CAS PubMed.
- P. A. Neale, A. Antony, M. E. Bartkow, M. J. Farre, A. Heitz, I. Kristiana, J. Y. M. Tang and B. I. Escher, Bioanalytical assessment of the formation of disinfection byproducts in a drinking water treatment plant, Environ. Sci. Technol., 2012, 46, 10317–10325 CAS.
- X. M. Lv, Y. Lu, X. M. Yang, X. R. Dong, K. P. Ma, S. H. Xiao, Y. Z. Wang and F. Tang, Mutagenicity of drinking water sampled from the Yangtze River and Hanshui River (Wuhan section) and correlations with water quality parameters, Sci. Rep., 2015, 5, 9572 CrossRef CAS PubMed.
-
S. D. Richardson and C. Postigo, in Recent Advances in Disinfection by-Products, ed. T. Karanfil, B. Mitch, P. Westerhoff and Y. Xie, American Chemical Society, 2015, vol. 1190, pp. 189–214 Search PubMed.
- P. A. Neale, R. Altenburger, S. Ait-Aissa, F. Brion, W. Busch, G. D. Umbuzeiro, M. S. Denison, D. Du Pasquier, K. Hilscherova, H. Hollert, D. A. Morales, J. Novak, R. Schlichting, T. B. Seiler, H. Serra, Y. Shao, A. J. Tindall, K. E. Tollefsen, T. D. Williams and B. I. Escher, Development of a bioanalytical test battery for water quality monitoring: Fingerprinting identified micropollutants and their contribution to effects in surface water, Water Res., 2017, 123, 734–750 CrossRef CAS PubMed.
- M. König, B. I. Escher, P. A. Neale, M. Krauss, K. Hilscherova, J. Novak, I. Teodorovic, T. Schulze, S. Seidensticker, M. A. K. Hashmi, J. Ahlheim and W. Brack, Impact of untreated wastewater on a major European river evaluated with a combination of in vitro bioassays and chemical analysis, Environ. Pollut., 2017, 220, 1220–1230 CrossRef PubMed.
- J. Nivala, P. A. Neale, T. Haasis, S. Kahl, M. Konig, R. A. Muller, T. Reemtsma, R. Schlichtinge and B. I. Escher, Application of cell-based bioassays to evaluate treatment efficacy of conventional and intensified treatment wetlands, Environ. Sci.: Water Res. Technol., 2018, 4, 206–217 RSC.
- B. I. Escher, L. Glauch, M. Konig, P. Mayer and R. Schlichting, Baseline toxicity and volatility cutoff in reporter gene assays used for high-throughput screening, Chem. Res. Toxicol., 2019, 32, 1646–1655 Search PubMed.
- G. Reifferscheid, H. M. Maes, B. Allner, J. Badurova, S. Belkin, K. Bluhm, F. Brauer, J. Bressling, S. Domeneghetti, T. Elad, S. Fluckiger-Isler, H. J. Grummt, R. Guertler, A. Hecht, M. B. Heringa, H. Hollert, S. Huber, M. Kramer, A. Magdeburg, H. T. Ratte, R. Sauerborn-Klobucar, A. Sokolowski, P. Soldan, T. Smital, D. Stalter, P. Venier, C. Ziemann, J. Zipperle and S. Buchinger, International round-robin study on the Ames fluctuation test, Environ. Mol. Mutagen., 2012, 53, 185–197 CrossRef CAS.
-
ISO 11350, Water Quality - Determination of the genotoxicity of water and waste water - Salmonella/microsome fluctuation test (Ames fluctuation test), International Organization for Standardization (ISO), Geneva, Switzerland, 2012 Search PubMed.
- B. I. Escher, P. A. Neale and D. L. Villeneuve, The advantages of linear concentration-response curves
for in vitro bioassays with environmental samples, Environ. Toxicol. Chem., 2018, 37, 2273–2280 CrossRef CAS.
- P. D. Scott, H. M. Coleman, S. Khan, R. Limc, J. A. McDonald, J. Mondon, P. A. Neale, E. Prochazka, L. A. Tremblay, M. S. Warne and F. D. L. Leusch, Histopathology, vitellogenin and chemical body burden in mosquitofish (Gambusia holbrooki) sampled from six river sites receiving a gradient of stressors, Sci. Total Environ., 2018, 616, 1638–1648 CrossRef.
- F. D. L. Leusch, P. A. Neale, C. Arnal, N. H. Aneck-Hahn, P. Balaguer, A. Bruchet, B. I. Escher, M. Esperanza, M. Grimaldi, G. Leroy, M. Scheurer, R. Schlichting, M. Schriks and A. Hebert, Analysis of endocrine activity in drinking water, surface water and treated wastewater from six countries, Water Res., 2018, 139, 10–18 CrossRef CAS PubMed.
- B. I. Escher, M. Allinson, R. Altenburger, P. A. Bain, P. Balaguer, W. Busch, J. Crago, N. D. Denslow, E. Dopp, K. Hilscherova, A. R. Humpage, A. Kumar, M. Grimaldi, B. S. Jayasinghe, B. Jarosova, A. Jia, S. Makarov, K. A. Maruya, A. Medvedev, A. C. Mehinto, J. E. Mendez, A. Poulsen, E. Prochazka, J. Richard, A. Schifferli, D. Schlenk, S. Scholz, F. Shiraish, S. Snyder, G. Y. Su, J. Y. M. Tang, B. van der Burg, S. C. van der Linden, I. Werner, S. D. Westerheide, C. K. C. Wong, M. Yang, B. H. Y. Yeung, X. W. Zhang and F. D. L. Leusch, Benchmarking organic micropollutants in wastewater, recycled water and drinking water with in vitro bioassays, Environ. Sci. Technol., 2014, 48, 1940–1956 CrossRef CAS PubMed.
- S. O. Simmons, C. Y. Fan and R. Ramabhadran, Cellular stress response pathway system as a sentinel ensemble in toxicological screening, Toxicol. Sci., 2009, 111, 202–225 CrossRef CAS.
- D. Stalter, E. O'Malley, U. von Gunten and B. I. Escher, Fingerprinting the reactive toxicity pathways of 50 drinking water disinfection by-products, Water Res., 2016, 91, 19–30 CrossRef CAS PubMed.
- E. L. Sharp, S. A. Parsons and B. Jefferson, Seasonal variations in natural organic matter and its impact on coagulation in water treatment, Sci. Total Environ., 2006, 363, 183–194 CrossRef CAS PubMed.
- M. E. Müller, B. I. Escher, M. Schwientek, M. Werneburg, C. Zarfl and C. Zwiener, Combining in vitro reporter gene bioassays with chemical analysis to assess changes in the water quality along the Ammer River, Southwestern Germany, Environ. Sci. Eur., 2018, 30, 20 CrossRef PubMed.
- P. A. Neale, N. A. Munz, S. Aït-Aïssa, R. Altenburger, F. Brion, W. Busch, B. I. Escher, K. Hilscherová, C. Kienle, J. Novák, T.-B. Seiler, Y. Shao, C. Stamm and J. Hollender, Integrating chemical analysis and bioanalysis to evaluate the contribution of wastewater effluent on the micropollutant burden in small streams, Sci. Total Environ., 2017, 576, 785–795 CrossRef CAS PubMed.
- P. A. Neale, S. Ait-Aissa, W. Brack, N. Creusot, M. S. Denison, B. Deutschmann, K. Hilscherova, H. Hollert, M. Krauss, J. Novak, T. Schulze, T. B. Seiler, H. Serra, Y. Shao and B. I. Escher, Linking in vitro effects and detected organic micropollutants in surface water using mixture-toxicity modeling, Environ. Sci. Technol., 2015, 49, 14614–14624 CrossRef CAS.
-
US EPA, Interactive Chemical Safety for Sustainability (iCSS) Dashboard v2, http://actor.epa.gov/dashboard/, (accessed Accessed 3rd April 2019) Search PubMed.
- H. Khalaf, L. Salste, P. Karlsson, P. Ivarsson, J. Jass and P. E. Olsson,
In vitro analysis of inflammatory responses following environmental exposure to pharmaceuticals and inland waters, Sci. Total Environ., 2009, 407, 1452–1460 CrossRef CAS.
- S. C. Miller, R. L. Huang, S. Sakamuru, S. J. Shukla, M. S. Attene-Ramos, P. Shinn, D. Van Leer, W. Leister, C. P. Austin and M. H. Xia, Identification of known drugs that act as inhibitors of NF-κB signaling and their mechanism of action, Biochem. Pharmacol., 2010, 79, 1272–1280 CrossRef CAS.
- P. A. Neale, F. D. L. Leusch and B. I. Escher, What is driving the NF-κB response in environmental water extracts?, Chemosphere, 2018, 210, 645–652 CrossRef CAS PubMed.
- D. Simazaki, M. Hirose, H. Hashimoto, S. Yamanaka, M. Takamura, J. Watanabe and M. Akiba, Occurrence and fate of endotoxin activity at drinking water purification plants and healthcare facilities in Japan, Water Res., 2018, 145, 1–11 CrossRef CAS PubMed.
- L. Guzzella, S. Monarca, C. Zani, D. Feretti, I. Zerbini, A. Buschini, P. Poli, C. Rossi and S. D. Richardson,
In vitro potential genotoxic effects of surface drinking water treated with chlorine and alternative disinfectants, Mutat. Res., Genet. Toxicol. Environ. Mutagen., 2004, 564, 179–193 CrossRef CAS.
- M. B. Heringa, D. J. H. Harmsen, E. F. Beerendonk, A. A. Reus, C. A. M. Krul, D. H. Metz and G. F. Ijpelaar, Formation and removal of genotoxic activity during UV/H2O2-GAC treatment of drinking water, Water Res., 2011, 45, 366–374 CrossRef CAS PubMed.
- D. Stalter, L. I. Peters, E. O'Malley, J. Y. M. Tang, M. Revalor, M. J. Farre, K. Watson, U. von Gunten and B. I. Escher, Sample enrichment for bioanalytical assessment of disinfected drinking water: Concentrating the polar, the volatiles, and the unknowns, Environ. Sci. Technol., 2016, 50, 6495–6505 CrossRef CAS PubMed.
- B. I. Escher, S. Ait-Aissa, P. A. Behnisch, W. Brack, F. Brion, A. Brouwer, S. Buchinger, S. E. Crawford, D. Du Pasquier, T. Hamers, K. Hettwer, K. Hilscherova, H. Hollert, R. Kase, C. Kienle, A. J. Tindall, J. Tuerk, R. van der Oost, E. Vermeirssen and P. A. Neale, Effect-based trigger values for in vitro and in vivo bioassays performed on surface water extracts supporting the environmental quality standards (EQS) of the European Water Framework Directive, Sci. Total Environ., 2018, 628–629, 748–765 CrossRef CAS PubMed.
- B. I. Escher, P. A. Neale and F. D. L. Leusch, Effect-based trigger values for in vitro bioassays: Reading across from existing water quality guideline values, Water Res., 2015, 81, 137–148 CrossRef CAS PubMed.
- B. I. Escher, C. van Daele, M. Dutt, J. Y. M. Tang and R. Altenburger, Most oxidative stress response in water samples comes from unknown chemicals: The need for effect-based water quality trigger values, Environ. Sci. Technol., 2013, 47, 7002–7011 CrossRef CAS PubMed.
- X. J. Wang, J. D. Hayes and C. R. Wolf, Generation of a stable antioxidant response element-driven reporter gene cell line and its use to show redox-dependent activation of Nrf2 by cancer chemotherapeutic agents, Cancer Res., 2006, 66, 10983–10994 CrossRef CAS PubMed.
- B. I. Escher, M. Dutt, E. Maylin, J. Y. M. Tang, S. Toze, C. R. Wolf and M. Lang, Water quality assessment using the AREc32 reporter gene assay indicative of the oxidative stress response pathway, J. Environ. Monit., 2012, 14, 2877–2885 RSC.
Footnote |
† Electronic supplementary information (ESI) available. See DOI: 10.1039/c9ew00987f |
|
This journal is © The Royal Society of Chemistry 2020 |