Residential water-soluble organic gases: chemical characterization of a substantial contributor to indoor exposures†
Received
27th February 2019
, Accepted 9th May 2019
First published on 3rd June 2019
Abstract
Characterization of residential indoor air is important to understanding exposures to airborne chemicals. While it is well known that non-polar VOCs are elevated indoors, polar VOCs remain poorly characterized. Recent measurements showed that total polar water-soluble organic gas (WSOG) concentrations are also much higher indoors than directly outdoors (on average 15× greater at 13 homes, on a carbon-mass basis). This work aims to chemically characterize these WSOG mixtures. Acetic, lactic, and formic acids account for 41% on average (30–54% across homes), of the total WSOG-carbon collected inside each home. Remaining WSOGs were characterized via high-resolution positive-mode electrospray ionization mass spectrometry. In total, 98 individual molecular formulas were detected. On average 67% contained the elements CHO, 11% CHN, 11% CHON, and 11% contained sulfur, phosphorus, or chlorine. Some molecular formulas are consistent with compounds having known indoor sources such as diethylene glycol (m/z+ 117.091, C4H10O3), hexamethylenetetramine (m/z+ 141.113, C6H12N4), and methacrylamide (m/z+ 86.060, C4H7NO). Exposure pathways, potential doses, and implications are discussed.
Environmental significance
This paper titled “Residential water-soluble organic gases: chemical characterization of a substantial contributor to indoor exposures” provides essential insight into an environment where we spend much of our time – our homes. Much remains to be known about the chemical composition of indoor air, its chemistry, and its impacts on exposure and health. In this work, we collected water-soluble organic gases (WSOGs) in 13 real homes in the United States and chemically characterized them. About 40% of the collected WSOG by mass can be attributed to organic acids. Of the remainder, 22% by number contained nitrogen. We preliminarily identified many of these compounds, provided dose estimates, and speculated on their potential health implications.
|
1. Introduction
Residential indoor environments are critical locations for exposure to air pollutants since on average in the United States, people spend upwards of 70% of their time in these spaces.1 Despite this, indoor air composition remains poorly characterized. It is though, well known that non-polar volatile organic compound (VOC) concentrations are higher indoors than outdoors.2–5 Several oxygenated and polar VOCs are also known to be much higher indoors than outdoors.6–10 In fact, recent measurements indicate that concentrations of total water-soluble organic gases (WSOGs) were 15 times higher, on average, inside 13 homes in New Jersey and North Carolina than directly outside, on a carbon basis (μg-C m−3).11 While several polar VOCs are commonly targeted for measurement indoors such as aldehydes (e.g., formaldehyde, acetaldehyde),8–10 and measurements of additional compounds are becoming more numerous,7,12–14 polar, oxygenated and water-soluble organics are generally poorly characterized due to analytical challenges. Compound classes that we expect to be in indoor air include: organic acids, nitrates, amines, phenols, peroxides, epoxides and glycols.11 In this work, we used mass-balance and non-targeted approaches to better understand the major components of residential indoor WSOGs and constrain the unknowns.
This work is also motivated by an interest in indoor chemistry in “damp” homes. In the United States, 18–50% of homes are considered damp and that dampness is associated with respiratory health effects such as asthma and wheeze.15,16 WSOGs are likely to participate in aqueous chemistry in liquid water in homes especially in homes that are damp, altering the composition of indoor air in ways that are not yet understood.11 Characterization of water-soluble compounds present indoors is a crucial early step in investigating the hypothesis that indoor chemistry (in addition to microbiology) may play a role in the relationship between dampness and health. To this end, in this work we aim to chemically characterize the WSOGs collected in those 13 New Jersey and North Carolina homes. In addition, we further explore and describe the relatively low-cost WSOG sampling method. Ultimately, this work is designed to inform future measurement campaigns to investigate exposure to residential indoor organic gases and their impact on health.
2. Methods
WSOGs were collected into liquid water from a convenience sample of 13 homes in New Jersey and North Carolina from June through October, 2015 and were analyzed for total organic carbon, organic acids, and other WSOGs. These gases were sampled using Cofer scrubbers, or mist chambers, for 2 hours twice consecutively during the daytime in each home. Technician surveys and participant questionnaires were used to understand each indoor home environment.
2.1 Characterization of the sampling environment
Homes were characterized using participant questionnaires to provide insight into WSOG sources, by technician surveys to gain additional insight into sources and obtain parameters relevant to calculate air exchange rate (AER), and by measuring indoor temperature, relative humidity, and carbon dioxide concentrations to provide auxiliary measurements. Prior to sampling, residents were asked several questions (verbally) aimed at characterizing the sampling environment (see ESI and Table S1† for questionnaire and responses; Rutgers IRB #15-636M and UNC IRB #15-1611). Some questions pertained to potential sources, such as: “Do you have a gas or electric stove?”, “What food have you cooked in the past 24 hours?”, and “Do you have an attached garage?” A technician survey provided additional information relevant to sources (e.g., type of flooring, food cooked during sampling, and number of people present during sampling). Information predictive of air exchange rate (e.g., home age, volume, indoor/outdoor temperature, and CO2 concentration) was obtained and used to provide an air exchange rate estimate as explained in ESI, including eqn (S1) and (S2).† Windows were closed during sampling. Floor area and floor and building height were measured using a laser tape measure (Bosch GLM 15, Robert Bosch Tool Corporation, Mount Prospect, IL). Indoor temperature, relative humidity and CO2 concentrations were recorded hourly with an Extech SD800 CO2/humidity/temperature data logger (Extech, Nashua, NH). Outdoor ozone and temperature data are reported from nearby monitoring sites (Table 1).
Table 1 Characteristics of sampled homes, indoor/outdoor environments, and concentrations of total water-soluble organic gases (WSOG), acetic and formic acids
Home |
Year home built (range) |
Home areaa (m2) |
Building heighta (m) |
Calculated AERa |
Indoor T (mean, °C) |
Indoor WSOG in water sample (μM-C) |
Indoor WSOG (gas phase) (μg-C m−3) |
Indoor acetic acid contribution to WSOG (%) |
Indoor formic acid contribution to WSOG (%) |
Indoor RH (mean, %) |
Indoor CO2 (mean, ppm) |
Occupancy (weighted)b |
Outdoor Tc (mean, °C) |
Outdoor ozoned (ppb) |
Home 6 and 13 shapes were highly irregular, preventing building heights and home areas from being confidently measured and thereby air exchange rates from being calculated.
Occupancy was weighted by multiplying the number of occupants by the time they were present divided by the total sample time.
Outdoor temperature was obtained from “Weather Underground” Linden, New Jersey, and Morrisville, North Carolina sites.
Outdoor ozone was obtained from New Jersey Department of Environmental Protection Rider University Campus and North Carolina Department of Environmental Quality Durham Armory ambient air quality sites.
|
1 |
1960–1979 |
>200 |
5–6 |
0.4 |
22 |
680 |
106 |
40.7 |
13.4 |
57 |
1000 |
4 |
23 |
17 |
2 |
1980–1989 |
<100 |
5–6 |
0.5 |
26 |
1150 |
178 |
25.8 |
6.6 |
56 |
620 |
2 |
30 |
43 |
3 |
1980–1989 |
100–200 |
5–6 |
0.4 |
23 |
1050 |
162 |
30.0 |
4.7 |
60 |
850 |
3 |
27 |
28 |
4 |
1990–2015 |
<100 |
5–6 |
0.3 |
23 |
1080 |
167 |
37.1 |
8.3 |
55 |
1010 |
3 |
30 |
58 |
5 |
1930–1950 |
<100 |
3–5 |
0.8 |
24 |
740 |
115 |
28.7 |
5.1 |
61 |
750 |
3 |
25 |
44 |
6 |
1980–1989 |
— |
— |
— |
26 |
710 |
110 |
40.5 |
8.9 |
58 |
750 |
4 |
25 |
49 |
7 |
1990–2015 |
>200 |
6–8 |
0.3 |
25 |
1110 |
172 |
40.3 |
9.6 |
59 |
950 |
2.25 |
32 |
45 |
8 |
1930–1950 |
>200 |
3–5 |
0.5 |
22 |
560 |
87 |
33.3 |
9.1 |
55 |
870 |
4.25 |
20 |
33 |
9 |
1960–1979 |
<100 |
3–5 |
0.5 |
21 |
1240 |
192 |
25.0 |
5.1 |
67 |
1390 |
2.75 |
21 |
No data |
10 |
1980–1989 |
>200 |
5–6 |
0.3 |
23 |
1380 |
215 |
33.0 |
7.6 |
60 |
970 |
2.56 |
27 |
47 |
11 |
1930–1950 |
<100 |
5–6 |
0.8 |
25 |
630 |
98 |
39.1 |
8.5 |
62 |
1130 |
2.13 |
33 |
52 |
12 |
1930–1950 |
100–200 |
6–8 |
0.6 |
20 |
540 |
84 |
31.6 |
9.4 |
58 |
690 |
2.81 |
15 |
8 |
13 |
1960–1979 |
— |
— |
— |
25 |
1280 |
198 |
26.2 |
6.1 |
53 |
1120 |
2.69 |
29 |
59 |
2.2 Sampling
Two mist chambers17 collected WSOGs inside the main living area of the home (e.g., living or dining room) for 2 hours twice sequentially from approximately 10:00 am to 2:00 pm as described previously.11 Briefly, 1 m long 1/2 in diameter Teflon inlets were placed ∼1.5 m from the floor and at least 1.0 m from walls. Each mist chamber sampled particle-filtered air at 25 L min−1 through a refluxing mist created from a reservoir of 25 mL of 17.8 ± 0.5 MΩ cm ultra-pure water. Water lost by evaporation during sampling was replaced as necessary. Particles were removed upstream with a pre-baked quartz filter (QFF; Pall, Port Washington, NY, 47 mm. This type of filter was used because its low-pressure drop does not interfere with mist creation). For each home, aqueous samples (pH = 4.5 ± 0.4) were composited in analysis-sized aliquots. Field water blanks and 2 minute “dynamic” blanks (conducted by placing Pall activated carbon filters in line before the mist chambers) were collected prior to daily sampling.
2.3 Analyses
Aqueous mist chamber samples and blanks were analyzed for total organic carbon (TOC) with a Shimadzu TOC 5000A (reported previously,11 Shimadzu Corporation, Kyoto, Japan), for organic acids by ion chromatography (IC) using a Dionex ICS 3000 (Thermo Fisher Scientific, Waltham, MA), and for other WSOGs by high-resolution quadrupole time-of-flight mass spectrometry with an electrospray ion source (ESI-QTOF-MS, Agilent 6520, Agilent Technologies, Santa Clara, CA). Organic acids were quantified by IC using an IonPac AS11-HC column and guard column with conductivity detection at 35 °C. The eluent was 17.8 ± 0.5 MΩ cm ultra-pure water (flow rate = 0.4 mL min−1) and the buffer was a gradient of potassium hydroxide. TOC and organic acids were quantified with authentic standards (for data quality, see ESI†).
For qualitative characterization of remaining WSOG mass, i.e., to determine molecular formulas of additional collected compounds, aliquots (0.2 mL injection volume) of mist chamber samples were also directly injected into the ESI-QTOF-MS in the positive mode with a 5 ppm mass resolution (which provides elemental formulas) over a mass range of 50–1000 amu. In the positive mode, alcohols, carbonyls, peroxides, reduced nitrogen and some nitro-organic species are ionized by addition of a hydrogen, sodium, or ammonium ion. In the negative mode, ions are generated by H-atom abstraction, which preferentially identifies organic acids. Since the IC analysis was designed to detect organic acids, we operated the ESI-QTOF-MS in positive mode to characterize the remaining mass. For ESI-QTOF-MS analysis, the mobile phase was 50% methanol and 50% 17.8 ± 0.5 MΩ cm ultra-pure water with 0.05% formic acid and flowed at 0.2 mL min−1. The fragmentor voltage was 40 V and the capillary voltage was 3700 V. The nitrogen drying gas (11 L min−1) was held at 350 °C, and the nebulizer gas pressure was 25 psig. Protonated hexakis ((1H,1H,3H-tetrafluoropropoxy)-phosphazine; m/z+ 922.009798) and purine (m/z+ 121.050873) were continuously infused for real-time mass axis correction. Ions (m/z+) were considered to be present in the sample (detected) if their sample abundance was more than the mean + 3σ of the blank. As a note on this analysis technique, electrospray ionization efficiency is compound-class dependent. For example, amines are easily ionized while aromatic alcohols are not. Thus, relative abundance does not indicate relative contribution to total WSOG, and some compounds will be detected at lower concentrations than others (Fig. S1†). Additionally, while we can separate by exact mass and thus know the elemental composition of the ion, several compounds collected may have the same elemental formula.
2.4 Sampler characterization
Historically, mist chambers have been used to measure individual compounds.18,19 While they have also been used to measure total WSOGs in outdoor air,20,21 characterization of their performance is limited although improving.22 In a 14th home, described in detail previously6 where home area, estimated air exchange rate, and total WSOG were within range of those in the 13 homes reported in this study,6 we characterize mist chamber collection of total WSOG and formic and acetic acids as a function of sampling time. Here, we sampled the same air using different sample collection times, 7.5 min, 15 min, 30 min, 1 h, 2 h, 4 h, and 8 h (see Fig. S2 and ESI† for further sampling information). Fig. 1 shows that the total WSOG concentrations in the mist chamber samples increased with increasing collection time. With increasing collection time, the rate of increase slowed, suggesting that the water in the mist chamber reservoir was approaching Henry's law equilibrium, but had not yet reached it, at 8 h. Concentrations of collected acetic and formic acids increased similarly (Fig. 1b). Also, the contributions of acetic and formic acids relative to the total mixture are smaller with shorter collection times and larger with longer collection times (Fig. 1c). This may be because more water-soluble compounds are collected preferentially (such as glyoxal (H = 4
000
000 M atm−1)) compared to less water-soluble compounds.18 Longer sample times provide higher concentration samples and mixtures weighted by water solubility. Shorter sample times provide higher collection efficiencies and more composited sample volume for replicate analyses. A 2 hour sampling time, as used here, balances these competing considerations.
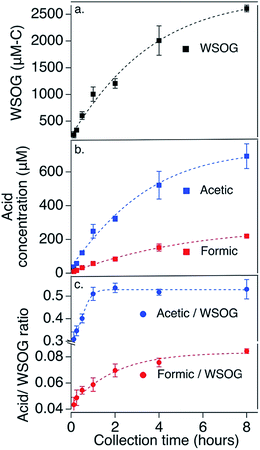 |
| Fig. 1 (a) Total water-soluble organic gas concentration (WSOG in μM-carbon) in mist chamber water versus collection time. (b) Acetic and formic acid concentrations (μM-compound) in mist chamber water versus collection time. (c) Acetic and formic acid (μM-carbon) normalized to WSOG (μM-carbon) versus collection time. | |
In addition, in this same home, collection efficiencies for total WSOG, formic acid, and acetic acid were determined for 2 hour sampling times by placing two sets of paired mist chambers in series as detailed in ESI.† These collection efficiency tests were conducted 10 times with the order of the mist chambers switched after each test (Fig. S3†). Concentrations reported below are corrected for collection efficiencies; uncertainties account for variations in collection efficiency between samplers.
3. Results
WSOG concentrations and home characteristics are provided in Table 1. In a step-wise multiple linear regression (p-value < 0.05), “year home built” was the only predictor of the residential indoor WSOG concentration (a positive correlation with an R2 = 0.61, see Fig. S4†). The other variables regressed against indoor WSOG concentration were outdoor WSOG concentration, indoor temperature, outdoor temperature, indoor–outdoor temperature difference, indoor RH, outdoor ozone, indoor carbon dioxide, number of occupants, and home area. The association between home age and WSOG may exist because newer homes often have lower air exchange rates (AERs), leading to higher concentrations of indoor-generated WSOGs due to lower exfiltration rates. It is also possible that newer buildings have elevated levels of WSOG emissions and/or WSOG formation. Calculated AER was negatively correlated with the indoor WSOG concentration, as expected, but was not a strong predictor (R2 = 0.34). As a note, calculated AERs included building height, floor area, and year built (see ESI†) but did not take into consideration home-to-home variations in indoor–outdoor temperature difference or wind speed and likely underestimate home-to-home variability in air exchange rate.
Organic acids account for 41% (range = 30–54%) of gas phase WSOGs, on average, on a carbon basis, across all homes (Fig. 2). Acetic + lactic acid (Hacetic = 4000 M atm−1, Hlactic = 12
000 M atm−1) contributed 33% on average (range = 25–41%); formic acid (Hformic = 9000 M atm−1) contributed 8% on average (range = 5–13%). Note that lactic acid eludes too closely to acetic acid to quantify separately. Acetic acid is the dominant peak. However, lactic acid was clearly present as a shoulder on the acetic acid peak in the ion chromatogram (Fig. S5†) and detected by negative mode ESI-QTOF-MS. Lactic acid has been previously measured indoors in association with human activity.6,7 In this work, acetic + lactic acid was quantified as acetic acid and will be referred to as acetic acid henceforth. Average concentrations of gas phase acetic and formic acid (Table S2†) are within previously measured ranges.7,23,24
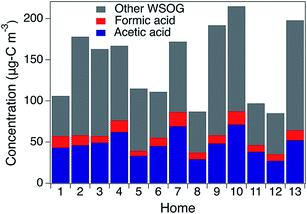 |
| Fig. 2 Contribution of acetic and formic acids (on a carbon basis) to total water-soluble organic gases (μg-C m−3). | |
Positive mode ESI-QTOF-MS provides insights into the remaining (∼60%) of the WSOG carbon mass (Fig. 3 and Table 2). Fig. 3a shows a number balance of compounds (elemental formulas) detected in each home. A total of 13–40 elemental formulas were detected in any given home, with a median of 28. On average, the majority were CHO compounds (67%), while 22% were nitrogen containing (11% CHN and 11% CHNO). Since these were detected in the positive mode, these nitrogenated compounds are presumably reduced nitrogen or nitro-containing compounds. Ions containing chlorine, phosphorus, and/or sulfur were also detected (11%, labeled “other” in Fig. 3a). Average nitrogen-to-carbon (N
:
C) and oxygen-to-carbon (O
:
C) ratios were 0.25 and 0.31, respectively. This N
:
C ratio is higher and the O
:
C ratio is lower than found for WSOGs collected similarly outdoors in the summer in Alabama, United States.25
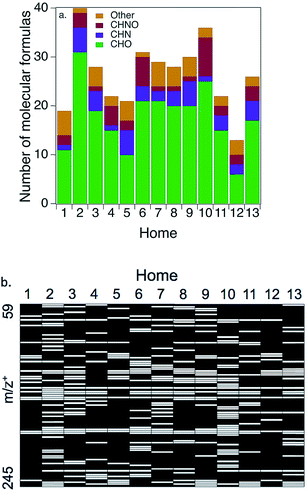 |
| Fig. 3 (a) Number of ions detected by home and by compound class: CHO, CHN, CHNO and “other”. Other includes chlorine, phosphorous and sulfur-containing ions. (b) Individual ions (m/z+) detected by home are shown as white bars, from m/z+ 59.049 to m/z+ 245.079. | |
Table 2 Elemental formulas identified with a mass accuracy 5 ppm or better, and probable compounds
Subgroup |
Formula |
m/z+a |
Ionization ion |
N
|
DBEc |
Probable compound |
Some compounds appeared multiple times due to detection of C13 and C14 isotopes and their ionization with hydrogen and sodium ions.
N depicts the number of homes detected.
DBE is number of double bond equivalents.
|
CHO |
C3H6O |
59.049 |
H+ |
3 |
1 |
Acetone |
C3H6O3 |
113.021 |
Na+ |
1 |
1 |
Lactic acid |
C3H8O2 |
99.042 |
Na+ |
11 |
0 |
Propylene glycol |
C4H6O2 |
87.044 |
H+ |
1 |
2 |
|
C4H6O4 |
141.016 |
Na+ |
3 |
2 |
|
C4H8O |
73.065 |
H+ |
4 |
1 |
2-Butanone |
C4H8O2 |
89.060 |
H+ |
2 |
1 |
Ethyl acetate |
C4H8O3 |
127.037 |
Na+ |
2 |
1 |
Methyl lactate |
C4H10O2 |
113.058 |
Na+ |
5 |
0 |
1,4-Butanediol |
C4H10O3 |
107.070 |
H+ |
5 |
0 |
Diethylene glycol |
129.053 |
Na+ |
10 |
C5H8O2 |
101.060 |
H+ |
3 |
2 |
4-Oxopentanal |
C5H8O3 |
139.037 |
Na+ |
1 |
2 |
|
C5H10O3 |
119.070 |
H+ |
3 |
1 |
Ethyl lactate |
141.053 |
Na+ |
C5H12O3 |
143.068 |
Na+ |
5 |
1 |
Trimethylolethane |
C6H12O |
101.097 |
H+ |
1 |
1 |
2-Hexanone |
C6H12O2 |
117.091 |
H+ |
1 |
1 |
Ethyl butyrate |
C6H12O3 |
155.068 |
Na+ |
2 |
1 |
|
C6H12O4 |
171.063 |
Na+ |
3 |
1 |
|
C6H14O2 |
141.089 |
Na+ |
4 |
0 |
2-Butoxyethanol |
C6H14O3 |
135.102 |
H+ |
13 |
0 |
Dipropylene glycol |
157.084 |
Na+ |
158.087 |
Na+ |
C6H14O4 |
173.078 |
Na+ |
2 |
0 |
Triethylene glycol |
C7H14O2 |
131.107 |
H+ |
2 |
1 |
Amyl acetate |
C7H12O4 |
161.081 |
H+ |
3 |
1 |
Diethyl malonate |
183.063 |
Na+ |
C7H16O3 |
149.117 |
H+ |
10 |
0 |
Di(propylene glycol) methyl ether |
171.099 |
Na+ |
172.103 |
Na+ |
C8H14O4 |
175.096 |
H+ |
3 |
2 |
Diethyl succinate |
197.078 |
Na+ |
C8H16O |
129.127 |
H+ |
1 |
1 |
Octanal |
C8H16O2 |
167.104 |
Na+ |
3 |
1 |
Hexyl acetate |
C8H16O3 |
183.099 |
Na+ |
8 |
1 |
|
C8H18O3 |
163.133 |
H+ |
13 |
0 |
Diethylene glycol butyl ether |
164.136 |
H+ |
185.115 |
Na+ |
186.118 |
Na+ |
187.120 |
Na+ |
C9H18O4 |
191.128 |
H+ |
2 |
1 |
|
213.110 |
Na+ |
214.114 |
Na+ |
C9H20O3 |
199.131 |
Na+ |
1 |
0 |
|
C10H8O3 |
177.054 |
H+ |
2 |
7 |
|
C10H18O2 |
171.139 |
H+ |
2 |
2 |
γ-Decanolactone |
C10H22O3 |
191.164 |
H+ |
12 |
0 |
Diethylene glycol hexyl ether |
213.146 |
Na+ |
214.149 |
Na+ |
215.151 |
Na+ |
C10H22O4 |
207.159 |
H+ |
3 |
0 |
|
C11H22O3 |
203.164 |
H+ |
1 |
1 |
|
C12H22O2 |
199.168 |
H+ |
3 |
2 |
|
200.172 |
H+ |
CHN |
C3H8N6 |
129.088 |
H+ |
1 |
3 |
Cyclic amine |
C4H6N2 |
83.060 |
H+ |
3 |
3 |
Methylimidazole |
C5H8N2 |
97.076 |
H+ |
1 |
3 |
Dimethylimidazole |
C5H12N6 |
157.12 |
H+ |
1 |
2 |
|
C6H12N4 |
141.113 |
H+ |
9 |
4 |
Hexamethylenetetramine |
142.116 |
H+ |
C6H12N6 |
169.120 |
H+ |
5 |
4 |
|
C7H8N4 |
171.064 |
Na+ |
1 |
6 |
|
C8H10N6 |
191.104 |
H+ |
1 |
6 |
|
C9H18N4 |
205.143 |
Na+ |
2 |
3 |
|
C11H12N4 |
223.096 |
Na+ |
7 |
8 |
|
CHNO |
C3H7NO |
96.042 |
Na+ |
2 |
1 |
Dimethylformamide |
C3H8N2O |
111.053 |
Na+ |
1 |
1 |
Dimethylurea |
C4H4N6O2 |
169.047 |
H+ |
1 |
6 |
|
C4H7NO |
86.060 |
H+ |
1 |
2 |
Methacrylamide |
C5H9NO |
123.061 |
Na+ |
1 |
2 |
n-Methyl-2-pyrrolidone |
C5H10N6O2 |
187.094 |
H+ |
1 |
4 |
|
C6H6N6O2 |
195.063 |
H+ |
1 |
7 |
|
C6H8N6O |
181.084 |
H+ |
1 |
6 |
|
C6H11NO |
114.092 |
H+ |
7 |
2 |
Caprolactam |
136.074 |
Na+ |
C6H13NO2 |
132.102 |
H+ |
1 |
1 |
Leucine |
C6H15NO2 |
134.117 |
H+ |
2 |
0 |
Diisopropanolamine |
C7H10N6O |
195.100 |
H+ |
1 |
6 |
|
C7H10N6O2 |
211.094 |
H+ |
1 |
5 |
|
C7H12N6O |
197.115 |
H+ |
4 |
5 |
|
C7H14N6O2 |
215.126 |
H+ |
4 |
4 |
|
C8H12N6O |
209.114 |
H+ |
2 |
6 |
|
C9H19NO2 |
174.149 |
H+ |
1 |
1 |
|
C12H17NO |
192.138 |
H+ |
2 |
5 |
Diethyltoluamide (DEET) |
Other |
C2H3Cl3O2 |
164.927 |
Na+ |
1 |
0 |
Chloral hydrate |
C3H5O2PS |
158.964 |
Na+ |
9 |
|
Organophosphate |
C4H3ClN2O |
131.001 |
H+ |
1 |
4 |
|
C5H5OP |
134.997 |
H+ |
7 |
4 |
Formylphosphole |
136.000 |
H+ |
C6H5OP |
146.997 |
Na+ |
4 |
5 |
Oxo(phenyl)phosphine |
C6H7OP |
149.012 |
Na+ |
8 |
4 |
Phosphinophenol |
150.016 |
Na+ |
C7H11N2O4P |
219.054 |
H+ |
5 |
5 |
|
Fig. 3b illustrates the considerable variability in composition across homes. A total of 98 distinct ions were detected across all homes from m/z+ 59.049–245.079. (Sometimes up to four different ions represented the same molecular formula, ionized by both H+ and Na+ and/or detected as isotopes; see Table 2.) Only 3 ions were detected in every home: m/z+ 157.084 (C6H14O3 + Na+) which is consistent with dipropylene glycol (H = 1.8 × 105 M atm−1), and 163.133 (C8H18O3 + H+) and 185.115 (C8H18O3 + Na+) which are both consistent with diethylene glycol monobutyl ether (H = 15 M atm−1).
Table 2 lists all elemental formulas detected via positive mode ESI-QTOF-MS. It is quite possible that multiple water-soluble compounds with the same elemental formula (isomers) could exist in the indoor environment. It is also likely that some compounds (e.g., aldehydes) are present in their hydrated form in these aqueous samples but are dehydrated in indoor air. Since methanol was added to the mobile phase during analysis, compounds could be hydrated either with H2O or CH3OH. In addition, some molecular formulas may be adducts even though the ESI-QTOF-MS fragmentor voltage was optimized to avoid this. For example, C12H22O2 is not likely to exist in the gas phase or to be water-soluble, so is probably an adduct of two smaller ions. In many cases, a detected elemental formula corresponds with a compound that is likely to be found indoors from reasonable sources;11 in those cases, the probable compound was also provided in Table 2.
4. Discussion
This work provides insights into the concentrations and composition of WSOGs inside several US residences. People spend the majority of their time indoors,1 and recent evidence suggests that residential WSOG concentrations are substantially elevated indoors compared to outdoors.11 This suggests that residential indoor environments are important contributors to total WSOG exposures. However, little is known about the composition, fate, exposure, and toxicity, of indoor WSOGs. For example, the lifetime and fate of WSOGs may differ from non-polar VOCs due to reactive uptake onto damp surfaces or into air conditioning systems.6,11 Their relative contributions to dermal and inhalation exposures may also be different.
To our knowledge, this research is the first effort to conduct a species mass balance on WSOG-carbon in homes. Organic acids (acetic, lactic, and formic acids) accounted for, on average, 41% of the total WSOG collected in each home, on a carbon basis. These acids are quite water-soluble and present in all 13 homes. Acetic and formic acids are released from household products, building materials, and candle burning and formed from VOC – ozone reactions.24,26,27 These acids have been measured extensively indoors using analytical techniques such as high performance liquid chromatography and recently with a high-resolution time-of-flight chemical ionization mass spectrometer equipped with iodide reagent ion chemistry (I-HR-ToF-CIMS), with reported ranges from 8.8–216 μg m−3 and 3.0–62 μg m−3, for acetic and formic acid respectively.6,7,24,26,27 Measurements herein fall within that range (Table 1). In addition, lactic acid, released from human perspiration and detected here but not quantified, was recently measured in real-time in a university classroom at concentrations of 0.9–27 μg m−3 (ref. 7) and in a home at concentrations up to 360 μg m−3 during cooking events.6
The remaining mass was highly complex and variable (Table 2). Measurements are consistent with the presence of common solvents such as acetone (C3H6O), ethyl acetate (C4H8O2), dipropylene glycol (C4H10O3), and diethylene glycol butyl ether (C8H18O3).28 The molecular formula C6H11NO was also detected, which is consistent with caprolactam, a known plastic degradation product.29 Additional detected compounds suggest the presence of known microbial VOCs (MVOCs) such as 2-butanone (C4H8O), 2-hexanone (C6H12O), and octanal (C8H16O).30 4-Oxopentanal (C5H8O2) is a major skin lipid decomposition product.31 Other elemental formulas are consistent with the presence of food flavorings and additives, such as ethyl butyrate (C6H12O2) and amyl acetate (C7H14O2)32,33 and formed from Maillard chemistry in cooking, such as methylimidazole (C4H6N2).34 Also, molecular formulas consistent with pesticides and insect repellents such as DEET (C12H17NO) were detected in some homes. Additionally, C2H3Cl3O2 likely indicates the presence of the disinfection byproduct, chloral hydrate.35
Thirty individual molecular formulas contained nitrogen. Since they were detected in the positive mode of ESI-QTOF-MS, we expect that they are reduced nitrogen species, such as amines or imidazoles. In one study, C1–C6 amines were measured indoors in a research trailer at the pptv level, much higher than concentrations measured directly outdoors.36 Other studies show that the relationship between amine concentrations indoors and outdoors depended on conditions such as the presence of smoking or poor ventilation.37,38 Indoors, ammonia is released from human sweat and breath, house pets and cleaning products;39 amines are emitted from sources such as tobacco smoke, kitchen and human waste, cleaning products and cooking40 or secondarily formed from ammonia reactions.41 Higher concentrations of reduced nitrogen species indoors (compared to outdoors), when present, may lead to differences in gas-phase and interfacial chemistry.
4.1 Exposure implications
Fig. 4 demonstrates the potential for both dermal and inhalation routes to contribute to doses of WSOG. For this simple illustration it was assumed that residents spent 24 h per day, 365 days per year exposed to a constant WSOG concentration of 170 μg m−3. Inhalation rates, body weights, skin surface areas, and life-time exposure durations representative of residents 70, 10, and 0.5 years-old were used to calculate average daily dose via inhalation and dermal routes as documented in ESI.† For this illustration, the WSOG permeability coefficients were varied by setting them equal to the permeability coefficients for 3 WSOGs preliminarily detected herein: diethyl phthalate (DEP) Kp = 3.4 m h−1, 4-oxopentanal (4-OPA) Kp = 0.56 m h−1, and butanol, Kp = 0.053 m h−1.42
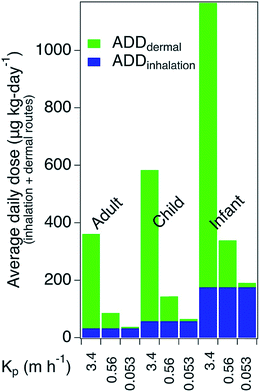 |
| Fig. 4 Average daily doses of WSOG via the inhalation and dermal routes for three age groups, assuming a WSOG concentration of 170 μg m−3, and a permeability coefficient of 3.4 m h−1 (like diethyl phthalate), 0.56 m h−1 (like 4-oxopentanal), and 0.053 m h−1 (like butanol). | |
Variation in inhalation dose was driven by age, with the average daily dose being highest for the youngest occupant (i.e., six-month-old infants). In contrast, for the dermal pathway, the average daily dose was largely driven by the permeability coefficient. This result suggests that dermal uptake and inhalation will both contribute to WSOG exposure, with dermal exposure being the predominant route for some WSOGs, and inhalation for others.
While the health effects of the total WSOG mixture is unknown, the health effects of a few components of WSOG mixtures have been studied. Dicarbonyls and aldehydes, such as diacetyl, glyoxal, methylglyoxal, 4-oxopentanoic acid, and glutaraldehyde are reported to increase cytokine levels in pulmonary epithelial cells and increase respiratory and dermal sensitivity in models.43,44 Due to their high water-solubilities, these compounds can dissolve in fluid in the respiratory tract and onto damp skin and eyes. A study of microbial VOCs (many of which are water-soluble) reported prevalence of mucus symptoms of occupants in homes with elevated levels of 1-octen-3-ol.45 Despite this important work, much remains unknown about the health effects of WSOG components and mixtures.
4.2 Limitations
A quartz fiber filter was used to remove particles upstream of the mist chamber, during WSOG collection. It is well recognized that organic gases can adsorb to quartz fiber filters, reducing the concentration of WSOG downstream until gas phase – adsorbed phase equilibrium is achieved in the vicinity of the filter. For this reason, the concentrations reported should be considered a lower bound.
Uncertainties in mist chamber collection efficiencies are the major determinants of uncertainties in reported concentrations. These uncertainties are larger than they would be if we designed the sampling protocol for measurement of a single water-soluble compound rather than a complex mixture of WSOGs because the collection efficiency of total WSOG will vary with composition and because of a need to balance collection efficiency/breakthrough with detection of lower abundance species. For all 13 homes, as well as the 14th home in which the collection efficiency experiments were conducted, acetic and formic acids comprised between 30 and 54% of total WSOG-carbon.
In addition, it is important to note that compounds with low water solubility will not be effectively collected. For example, using eqn (S1)† from Spaulding et al., the theoretical maximum collection efficiency for glyoxal (H = 4
000
000 M atm−1) is 100%, while it is 0.22% for 2-hexanone (H = 11 M atm−1). Also, while compounds must be water soluble to be collected in the mist chambers, some oxidation of collected WSOGs could take place during sampling. Specifically, while the hydroxyl radical is too reactive to make it into the mist chamber,46 some ozone could be collected. In this case, ozone could react with unsaturated WSOGs at the carbon–carbon double bond47 during sampling, altering sample composition. Such reactions could potentially also form hydroxyl radicals to further aqueous oxidation of collected compounds.48,49 While the magnitude of this sampling artifact is unknown, we speculate that it is minimal given the measured outdoor ozone concentrations (Table 1) and the fact that only a fraction (20 to 70%) of outdoor ozone will penetrate and persist indoors.50
5. Conclusions
This work improves the understanding of the indoor composition of WSOG and supports work to understand multiphase indoor chemistry.11 Some detected compounds have been measured indoors previously (e.g., acetic acid and formic acid), others are known to be emitted from indoor sources (octanol, acetone, ethyl acetate, 2-butoxyethanol), and yet others are known to be formed indoors through secondary gas phase chemistry (butanone).11 While this work represents a step forward in characterizing indoor WSOG, considerable effort remains to positively identify and quantify WSOG components and to understand their indoor chemistry, fate, and health effects. Ultimately, this work will improve our understanding of inhalation and dermal exposures in residential indoor environments.
Conflicts of interest
There are no conflicts to declare.
Acknowledgements
First and foremost, the authors gratefully acknowledge the participation of the study volunteers who allowed us into their homes for interviews and sample collection; without their involvement, this work would not be possible. The authors would also like to thank Ronald Lauck for his assistance with TOC analysis and Sophie Tomaz, Jason Surratt, and Glenn Morrison for their scientific insights. Finally, the authors gratefully acknowledge the support of the Alfred P. Sloan Foundation (grants G-2015-13886 and G-2017-9794), the National Institute of Environmental Health Sciences Exposure Training Grant (grant #T32ES019854), and UNC Biomarker Mass Spectrometry Facility funded by the National Institute of Environmental Health Sciences (grant #P30ES010126).
References
- N. E. Klepeis, W. C. Nelson, W. R. Ott, J. P. Robinson, A. M. Tsang, P. Switzer, J. V. Behar, S. C. Hern and W. H. Engelmann, The National Human Activity Pattern Survey (NHAPS): A Resource for Assessing Exposure to Environmental Pollutants, J. Exposure Anal. Environ. Epidemiol., 2001, 11(3), 231–252 CrossRef CAS PubMed.
- E. D. Pellizzari, T. D. Hartwell, R. L. Perritt, C. M. Sparacino, L. S. Sheldon, H. S. Zelon, R. W. Whitmore, J. J. Breen and L. Wallace, Comparison of Indoor and Outdoor Residential Levels of Volatile Organic Chemicals in Five U.S. Geographical Areas, Environ. Int., 1986, 12(6), 619–623 CrossRef CAS.
- I. Paciência, J. Madureira, J. Rufo, A. Moreira and E. de Oliveira Fernandes, A Systematic Review of Evidence and Implications of Spatial and Seasonal Variations of Volatile Organic Compounds (VOC) in Indoor Human Environments, J. Toxicol. Environ. Health, Part B, 2016, 19(2), 47–64 Search PubMed.
- F.-C. Su, B. Mukherjee and S. Batterman, Determinants of Personal, Indoor and Outdoor VOC Concentrations: An Analysis of the RIOPA Data, Environ. Res., 2013, 126, 192–203 CrossRef CAS PubMed.
- J. L. Adgate, L. E. Eberly, C. Stroebel, E. D. Pellizzari and K. Sexton, Personal, Indoor, and Outdoor VOC Exposures in a Probability Sample of Children, J. Exposure Anal. Environ. Epidemiol., 2004, 14(suppl. 1), S4–S13 CrossRef CAS PubMed.
- S. M. Duncan, S. Tomaz, G. Morrison, M. Webb, J. Atkin, J. D. Surratt and B. J. Turpin, Dynamics of Residential Water-Soluble Organic Gases: Insights into Sources and Sinks, Environ. Sci. Technol., 2019, 53(4), 1812–1821 CrossRef CAS PubMed.
- S. Liu, S. L. Thompson, H. Stark, P. J. Ziemann and J. L. Jimenez, Gas-Phase Carboxylic Acids in a University Classroom: Abundance, Variability, and Sources, Environ. Sci. Technol., 2017, 51(10), 5454–5463 CrossRef CAS PubMed.
- W. Liu, J. Zhang, L. Zhang, B. Turpin, C. Weisel, M. Morandi, T. Stock, S. Colome and L. Korn, Estimating Contributions of Indoor and Outdoor Sources to Indoor Carbonyl Concentrations in Three Urban Areas of the United States, Atmos. Environ., 2006, 40(12), 2202–2214 CrossRef CAS.
- T. Salthammer, S. Mentese and R. Marutzky, Formaldehyde in the Indoor Environment, Chem. Rev., 2010, 110(4), 2536–2572 CrossRef CAS PubMed.
- M. Cheng, I. E. Galbally, S. B. Molloy, P. W. Selleck, M. D. Keywood, S. J. Lawson, J. C. Powell, R. W. Gillett and E. Dunne, Factors Controlling Volatile Organic Compounds in Dwellings in Melbourne, Australia, Indoor Air, 2016, 26(2), 219–230 CrossRef CAS PubMed.
- S. M. Duncan, K. G. Sexton and B. J. Turpin, Oxygenated VOCs, Aqueous Chemistry, and Potential Impacts on Residential Indoor Air Composition, Indoor Air, 2018, 28, 198–212 CrossRef CAS PubMed.
- S. Liu, R. Li, R. J. Wild, C. Warneke, J. A. de Gouw, S. S. Brown, S. L. Miller, J. C. Luongo, J. L. Jimenez and P. J. Ziemann, Contribution of Human-Related Sources to Indoor Volatile Organic Compounds in a University Classroom, Indoor Air, 2016, 26(6), 925–938 CrossRef CAS PubMed.
- X. Tang, P. K. Misztal, W. W. Nazaroff and A. H. Goldstein, Volatile Organic Compound Emissions from Humans Indoors, Environ. Sci. Technol., 2016, 50(23), 12686–12694 CrossRef CAS PubMed.
- Y. Liu, P. K. Misztal, J. Xiong, Y. Tian, C. Arata, R. J. Weber, W. W. Nazaroff and A. H. Goldstein, Characterizing Sources and Emissions of Volatile Organic Compounds in a Northern California Residence Using Space- and Time-resolved Measurements, Indoor Air, 2019 DOI:10.1111/ina.12562.
- M. I. Gunnbjörnsdóttir, K. A. Franklin, D. Norbäck, E. Björnsson, D. Gislason, E. Lindberg, C. Svanes, E. Omenaas, E. Norrman and R. Jõgi,
et al., Prevalence and Incidence of Respiratory Symptoms in Relation to Indoor Dampness: The RHINE Study, Thorax, 2006, 61(3), 221–225 CrossRef PubMed.
- M. J. Mendell, A. G. Mirer, K. Cheung, M. Tong and J. Douwes, Respiratory and Allergic Health Effects of Dampness, Mold, and Dampness-Related Agents: A Review of the Epidemiologic Evidence, Environ. Health Perspect., 2011, 119(6), 748–756 CrossRef CAS PubMed.
- W. R. Cofer, V. G. Collins and R. W. Talbot, Improved Aqueous Scrubber for Collection of Soluble Atmospheric Trace Gases, Environ. Sci. Technol., 1985, 19(6), 557–560 CrossRef CAS PubMed.
- R. S. Spaulding, R. W. Talbot and M. J. Charles, Optimization of a Mist Chamber (Cofer Scrubber) for Sampling Water-Soluble Organics in Air, Environ. Sci. Technol., 2002, 36(8), 1798–1808 CrossRef CAS PubMed.
- J. Stutz, H.-J. Oh, S. I. Whitlow, C. Anderson, J. E. Dibb, J. H. Flynn, B. Rappenglück and B. Lefer, Simultaneous DOAS and Mist-Chamber IC Measurements of HONO in Houston, TX, Atmos. Environ., 2010, 44(33), 4090–4098 CrossRef CAS.
- R. S. Spaulding, Characterization of Secondary Atmospheric Photooxidation Products: Evidence for Biogenic and Anthropogenic Sources, J. Geophys. Res., 2003, 108(D8) DOI:10.1029/2002jd002478.
- C. J. Hennigan, M. H. Bergin, A. G. Russell, A. Nenes and R. J. Weber, Gas/Particle Partitioning of Water-Soluble Organic Aerosol in Atlanta, Atmos. Chem. Phys., 2009, 9(11), 3613–3628 CrossRef CAS.
- C. J. Hennigan, M. M. H. El-Sayed and A. Hodzic, Detailed Characterization of a Mist Chamber for the Collection of Water-Soluble Organic Gases, Atmos. Environ., 2018, 188, 12–17 CrossRef CAS.
- J. Zhang, Q. He and P. J. Lioy, Characteristics of Aldehydes: Concentrations, Sources, and Exposures for Indoor and Outdoor Residential Microenvironments, Environ. Sci. Technol., 1994, 28(1), 146–152 CrossRef CAS PubMed.
- R. Reiss, P. B. Ryan, S. J. Tibbetts and P. Koutrakis, Measurement of Organic Acids, Aldehydes, and Ketones in Residential Environments and Their Relation to Ozone, J. Air Waste Manage. Assoc., 1995, 45(10), 811–822 CrossRef CAS.
- N. Sareen, A. G. Carlton, J. D. Surratt, A. Gold, B. Lee, F. D. Lopez-Hilfiker, C. Mohr, J. A. Thornton, Z. Zhang and Y. B. Lim,
et al., Identifying Precursors and Aqueous Organic Aerosol Formation Pathways during the SOAS Campaign, Atmos. Chem. Phys., 2016, 16(22), 14409–14420 CrossRef CAS.
- J. Zhang, W. E. Wilson and P. J. Lioy, Sources of Organic Acids in Indoor Air: A Field Study, J. Exposure Anal. Environ. Epidemiol., 1994, 4(1), 25–47 CAS.
- H. Destaillats, M. M. Lunden, B. C. Singer, B. K. Coleman, A. T. Hodgson, C. J. Weschler and W. W. Nazaroff, Indoor Secondary Pollutants from Household Product Emissions in the Presence of Ozone: A Bench-Scale Chamber Study, Environ. Sci. Technol., 2006, 40(14), 4421–4428 CrossRef CAS PubMed.
- California Air Resources Board, Consumer Product Solvent Database, https://www.arb.ca.gov/db/solvents/all_cmpds.htm, accessed Dec 14, 2017.
- Y. Tokiwa, B. P. Calabia, C. U. Ugwu and S. Aiba, Biodegradability of Plastics, Int. J. Mol. Sci., 2009, 10(9), 3722–3742 CrossRef CAS PubMed.
- A. Korpi, J. Järnberg and A.-L. Pasanen, Microbial Volatile Organic Compounds, Crit. Rev. Toxicol., 2009, 39(2), 139–193 CrossRef CAS PubMed.
- A. Wisthaler and C. J. Weschler, Reactions of Ozone with Human Skin Lipids: Sources of Carbonyls, Dicarbonyls, and Hydroxycarbonyls in Indoor Air, Proc. Natl. Acad. Sci. U. S. A., 2010, 107(15), 6568–6575 CrossRef CAS PubMed.
- P. M. Jenner, E. C. Hagan, J. M. Taylor, E. L. Cook and O. G. Fitzhugh, Food Flavourings and Compounds of Related Structure I. Acute Oral Toxicity, Food Cosmet. Toxicol., 1964, 2, 327–343 CrossRef CAS.
-
United States Food and Drug Administration, Questions and Answers on Caramel Coloring and 4-MEI, Center for Food Safety and Applied Nutrition, 2014 Search PubMed.
- J.-K. Moon and T. Shibamoto, Formation of Carcinogenic 4(5)-Methylimidazole in Maillard Reaction Systems, J. Agric. Food Chem., 2011, 59(2), 615–618 CrossRef CAS PubMed.
- S. W. Krasner, H. S. Weinberg, S. D. Richardson, S. J. Pastor, R. Chinn, M. J. Sclimenti, G. D. Onstad and A. D. Thruston, Occurrence of a New Generation of Disinfection Byproducts, Environ. Sci. Technol., 2006, 40(23), 7175–7185 CrossRef CAS PubMed.
- Y. You, V. P. Kanawade, J. A. De Gouw, A. B. Guenther, S. Madronich, M. R. Sierra-Hernández, M. Lawler, J. N. Smith, S. Takahama and G. Ruggeri,
et al., Atmospheric Amines and Ammonia Measured with a Chemical Ionization Mass Spectrometer (CIMS), Atmos. Chem. Phys., 2014, 14, 12181–12194 CrossRef.
- G. Palmiotto, G. Pieraccini, G. Moneti and P. Dolara, Determination of the Levels of Aromatic Amines in Indoor and Outdoor Air in Italy, Chemosphere, 2001, 43(3), 355–361 CrossRef CAS PubMed.
- J. Zhu and B. Aikawa, Determination of Aniline and Related Mono-Aromatic Amines in Indoor Air in Selected Canadian Residences by a Modified Thermal Desorption GC/MS Method, Environ. Int., 2004, 30(2), 135–143 CrossRef CAS PubMed.
- M. A. Sutton, U. Dragosits, Y. S. Tang and D. Fowler, Ammonia Emissions from Non-Agricultural Sources in the UK, Atmos. Environ., 2000, 34(6), 855–869 CrossRef CAS.
- X. Ge, A. S. Wexler and S. L. Clegg, Atmospheric Amines – Part I. A Review, Atmos. Environ., 2011, 45(3), 524–546 CrossRef CAS.
-
J. H. Seinfeld and S. N. Pandis, Atmospheric Chemistry and Physics: From Air Pollution to Climate Change, J. Wiley, 2006 Search PubMed.
- C. J. Weschler and W. W. Nazaroff, Dermal Uptake of Organic Vapors Commonly Found in Indoor Air, Environ. Sci. Technol., 2014, 48(2), 1230–1237 CrossRef CAS PubMed.
- S. E. Anderson, J. Wells, A. Fedorowicz, L. F. Butterworth, B. Meade and A. E. Munson, Evaluation of the Contact and Respiratory Sensitization Potential of Volatile Organic Compounds Generated by Simulated Indoor Air Chemistry, Toxicol. Sci., 2007, 97(2), 355–363 CrossRef CAS PubMed.
- S. E. Anderson, L. G. Jackson, J. Franko and J. R. Wells, Evaluation of Dicarbonyls Generated in a Simulated Indoor Air Environment Using an In Vitro Exposure System, Toxicol. Sci., 2010, 115(2), 453–461 CrossRef CAS PubMed.
- A. Araki, T. Kawai, Y. Eitaki, A. Kanazawa, K. Morimoto, K. Nakayama, E. Shibata, M. Tanaka, T. Takigawa and T. Yoshimura,
et al., Relationship between Selected Indoor Volatile Organic Compounds, so-called Microbial VOC, and the Prevalence of Mucous Membrane Symptoms in Single Family Homes, Sci. Total Environ., 2010, 408(10), 2208–2215 CrossRef CAS PubMed.
- T. Ingham, A. Goddard, L. K. Whalley, K. L. Furneaux, P. M. Edwards, C. P. Seal, D. E. Self, G. P. Johnson, K. A. Read and J. D. Lee,
et al., A Flow-Tube Based Laser-Induced Fluorescence Instrument to Measure OH Reactivity in the Troposphere, Atmos. Meas. Tech., 2009, 2(2), 465–477 CrossRef CAS.
- C. C. D. Yao and W. R. Haag, Rate Constants for Direct Reactions of Ozone with Several Drinking Water Contaminants, Water Res., 1991, 25(7), 761–773 CrossRef CAS.
- J. Hoigné and H. Bader, Rate Constants of Reactions of Ozone with Organic and Inorganic Compounds in Water—I: Non-Dissociating Organic Compounds, Water Res., 1983, 17(2), 173–183 CrossRef.
- J. Hoigné and H. Bader, The Role of Hydroxyl Radical Reactions in Ozonation Processes in Aqueous Solutions, Water Res., 1976, 10(5), 377–386 CrossRef.
- C. J. Weschler, Ozone in Indoor Environments: Concentration and Chemistry, Indoor Air, 2000, 10(4), 269–288 CrossRef CAS PubMed.
Footnote |
† Electronic supplementary information (ESI) available. See DOI: 10.1039/c9em00105k |
|
This journal is © The Royal Society of Chemistry 2019 |