DOI:
10.1039/D4TA03393K
(Review Article)
J. Mater. Chem. A, 2024,
12, 23147-23178
Enhancing electrochemical performance and corrosion resistance of nickel-based catalysts in seawater electrolysis: focusing on OER and HER
Received
16th May 2024
, Accepted 3rd July 2024
First published on 4th July 2024
Abstract
Hydrogen production by electrochemical hydrogen evolution reaction (HER) using eco-friendly seawater electrolysis can help address the energy shortage. However, insoluble precipitates form from elements of magnesium, calcium, and chlorine ions with the oxygen evolution reaction (OER) will cause electrode degradation. Currently, commercially viable catalysts are mostly comprised of precious metal Pt-based catalysts, which are expensive and limited in availability. The critical factor for hydrogen production from seawater electrolysis is the development of highly efficient and corrosion-resistant catalysts. Nickel-based electrocatalysts, which are cost-effective with conductivity and corrosion resistance, are a prospective substitute for precious metal catalysts. This review summarizes contemporary methods and ideas for improving the performance of nickel-based electrocatalysts and stability for HER and OER in seawater, focusing on strengthening electrochemical performance and corrosion resistance. It is a comprehensive resource for advancing nickel-based electrocatalysts in seawater electrolysis, guiding the development of more efficient electrocatalysts and bolstering long-term reliability and stability in seawater environments.
1. Introduction
In the 21st century, humankind has faced increasingly severe energy crises and environmental problems such as global warming.1,2 These challenges have prompted a search for new energy sources to replace traditional fossil fuels, making carbon neutrality the mainstream direction for international development.3 At the recent 28th Conference of the Parties to the United Nations Framework Convention on Climate Change, UNFCCC (COP28) climate summit in Dubai, 190 countries agreed to move away from fossil fuel dependence, signaling the end of the fossil fuel era and growing demand for renewable energy.4–6 Hydrogen energy, with its high calorific value and clean combustion products, is expected to play a crucial role in future energy structure.7–10 Currently, mainstream hydrogen production processes fall into three main categories: hydrocarbon reforming and pyrolysis, biomass processes, and hydrolysis. Although hydrocarbon reforming and pyrolysis are the most mature hydrogen production processes, they generate carbon by-products and are highly dependent on fossil fuels.11 Water electrolysis is a promising future technology due to its environmental friendliness, lack of carbon emissions, and ease of industrial implementation (Fig. 1a).18 At this stage, the principle research and technical route of direct water electrolysis have become increasingly mature.19 However, since freshwater resources on the planet only account for 3.5% of the total water, using seawater for hydrogen production can lessen the tension of freshwater resources.20 At the same time, renewable seawater resources can support the electrolysis of seawater for hydrogen production to embark on the path of sustainable development. In addition, due to the poor electrical conductivity of freshwater, conventional water electrolysis technology requires the addition of acid, base, or buffer solution to freshwater before electrolysis operation.21 In contrast, seawater is rich in chloride ions (Cl−), sodium ions (Na+), magnesium ions (Mg2+), sulfate ions (SO42−), and calcium ions (Ca2+). The presence of these ions significantly increases the conductivity of seawater, making it a suitable electrolyte for the electrolysis reaction. Therefore, direct electrolysis of seawater for hydrogen production has essential research value22 (Scheme 1).
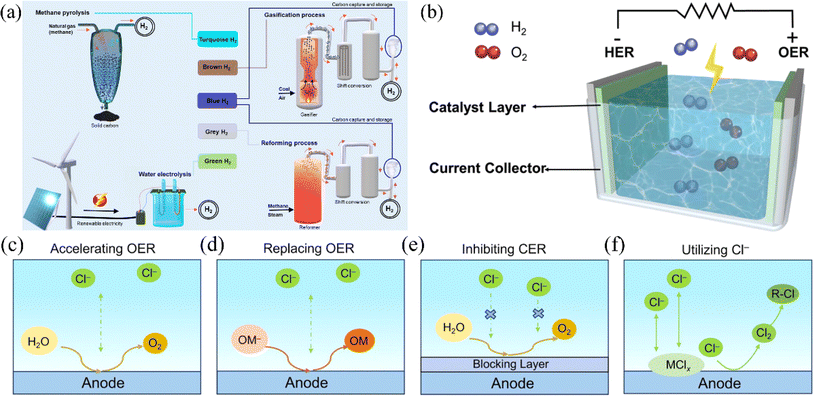 |
| Fig. 1 (a) Various processes for making hydrogen, where electrolysis of water to make hydrogen produces green hydrogen gas. Reproduced with permission.12 Copyright 2021, Springer Nature. (b) Diagram of a water electrolysis unit in which the hydrogen precipitation reaction occurs at the cathode and the oxygen precipitation reaction occurs at the anode. Reproduced with permission.13 Copyright 2021, Wiley-VCH. (c) Corrosion-resistant strategies on the anode. Preparation of high-activity catalysts to increase the selectivity of the OER. (d) Finding other oxides to undergo thermodynamically more favorable oxidation reactions on the anode instead of the OER. (e) Construct a protective layer resistant to chloride ion corrosion to stop the CER. (f) The use of free chloride ions to reconstitute the catalyst or the use of in situ-grown chloride ions to participate in the reaction. Reproduced with permission.14 Copyright 2023, American Chemical Society. | |
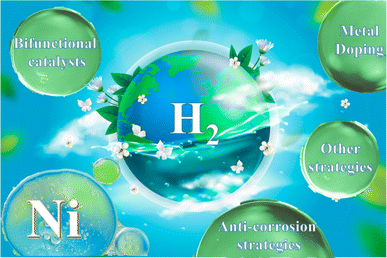 |
| Scheme 1 Research advance of Ni-based catalysts for HER in seawater. | |
However, seawater electrolysis also faces some challenges, as described below. Firstly, seawater electrolysis mainly involves two half-reactions: HER at the cathode and OER at the anode. Nevertheless, both half-reactions are thermodynamically upslope while not being kinetically favorable. Therefore, efficient catalysts are usually needed to lower the thermodynamic energy barrier and increase the reaction rate (Fig. 3a and b).23,27–30 These highly efficient catalysts work by lowering the thermodynamic energy barrier of the reaction, thereby reducing the energy input required for the reaction and increasing the reaction rate. The catalytic activity and stability of the catalysts can be improved by modifying the structure and composition of the catalysts and further modifying the electronic structure of the catalysts. A recent study demonstrated that introducing a Lewis acid layer (e.g., Cr2O3) on transition metal oxide catalysts can enhance the local reaction environment, promoting stability and performance in direct seawater electrolysis.31 Additionally, adjusting the interfacial structure of metal oxide substrates has been identified as a crucial strategy for inducing electronic structure reconstruction in supported catalysts, significantly enhancing their catalytic activity.32 Secondly, two primary challenges are encountered in HER. On the one hand, to speed up the kinetic reaction, it is necessary to enhance the electrical conductivity of the catalyst to promote the charge transfer mechanism and consequently boost the reaction rate. The catalyst's conductivity can be enhanced by strategically structuring its structure and composition, enhancing electron conduction pathways, or using conductive additives. On the other hand, microorganisms and ions in seawater can cause the formation of insoluble compounds on the catalyst's surface, which can block active sites and decrease the catalyst's activity. To ensure durability, it is essential to use electrode materials that strongly resist corrosion from seawater and pollutants and implement corrosion resistance techniques on them.33 Common corrosion resistance strategies on anodes are illustrated in Fig. 1c. Thirdly, for the OER at the anode, seawater is rich in halogen ions. Taking the most abundant chloride ion among them as an example, when the pH of the electrolyte is in the acidic range, Cl− will be oxidized to chlorine gas at the anode (2Cl− → Cl2 + 2e−); when the pH of the electrolyte is in the alkaline range, Cl− will be transformed to ClO− (Cl− + 2OH− → ClO− + H2O + 2e−). The presence of chloride ions reduces the selectivity of the OER.34,35 Although OER is superior to Chlorine Evolution Reaction (CER) in terms of thermodynamic mechanism, CER has kinetic advantages as it involves only two electrons, making it more favorable than the four-electron transfer process in OER.36 Although the kinetically faster CER accelerates the electron reaction and promotes hydrogen production at the cathode, the generated Cl2 and ClO− corrode the electrodes. This corrosion leads to reduced catalyst activity or even deactivation, which is inherently unfavorable for the continuation of the reaction.37
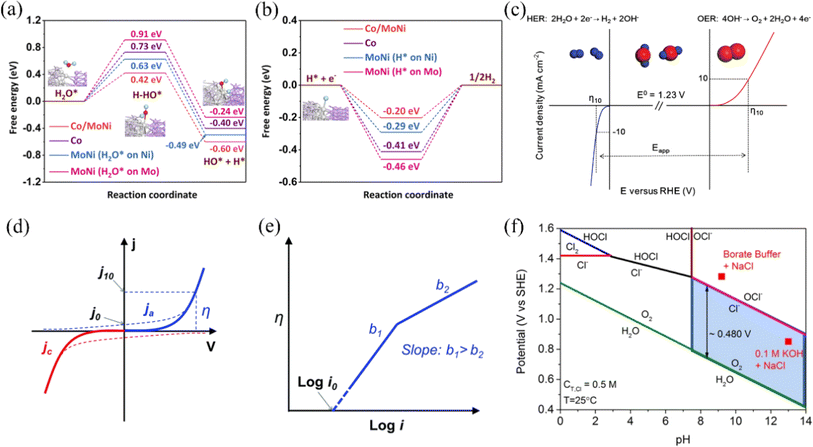 |
| Fig. 3 (a) Water dissociation energy barrier diagrams for Co, MoNi and Co/MoNi. (b) Hydrogen adsorption energy barriers for Co, MoNi and Co/MoNi. Reproduced with permission.23 Copyright 2023, Royal Society of Chemistry. (c) Typical HER and OER polarisation curves are shown as well as overpotentials at a current density of 10 mA cm−2 Reproduced with permission.24 Copyright 2017, Wiley-VCH. (d) Polarisation curves for cathode (left) and anode (right) electrodes, j0 is the exchange current at ja = −jc. (e) Tafel slope. Reproduced with permission.25 Copyright 2017, Royal Society of Chemistry. (f) Pourbaix diagram of the artificial seawater model at 0.5 M NaCl. Reproduced with permission.26 Copyright 2016, Wiley-VCH. | |
An urgent requirement exists to synthesis catalysts with high catalytic activity and strong corrosion resistance for seawater electrolysis, considering the constraints mentioned above. Currently, platinum (Pt) is found to be the most efficient catalyst for HER, while IrOx and RuOx are considered to be the most efficient catalysts for OER.38–40 However, these catalysts are precious metal catalysts, which are not conducive to large-scale industrialization. In recent years, researchers have found that transition metal electrocatalysts, which have good performance in the electrolysis of seawater and are abundantly available, make ideal raw materials for electrocatalysts. Among these, nickel-based materials, located in the first row of transition metals, are the most widely researched of all non-precious metal transition metal-based catalysts due to their excellent electrical conductivity and corrosion resistance.41 Nickel oxide, nickel hydroxide, nickel phosphide, and nickel sulfide are affordable compounds that show potential as effective catalysts for seawater electrolysis.42 Researchers have modified nickel-based materials to increase their electrochemical activity through doping modification, vacancy engineering, surface modification, and other strategies.43,44 According to recent reports, researchers have found that intercalation in 2D materials has shown promise in enhancing the properties of electrocatalysts, providing a pathway to tailor the electronic structure and improve catalytic performance.45 Numerous explorations have aimed at replacing precious metal catalysts with nickel-based materials, making it possible to use them as HER catalysts, OER catalysts, and even bifunctional catalysts.46 For example, Chen et al.47 found that covering a S-doped Fe(OH)3 layer on NiSe nanowires can provide many active sites for nickel-based materials and improve their corrosion resistance. Liu et al.48 constructed heterostructure CoP/Ni2P@NF on Ni2P materials, and they found that the coupling of CoP and Ni2P optimized the electronic structure of the catalyst's active site and endowed it with excellent HER catalytic performance. Gopalakrishnan et al.49 synthesized Co–Ni–S/NF in situ on nickel foam through a self-templating strategy. The catalyst showed outstanding efficiency in catalyzing both HER and OER due to the combined impact of the bimetal and the effective interfacial layer between the nanomaterials. However, research on nickel-based materials is still in the laboratory stage and needs to be further explored to meet the high volume of industrial production. In addition, enhancing the corrosion resistance and stability of catalysts in seawater environments is also a research priority to promote the early commercialization of nickel-based materials.
Previous reviews in this field have primarily focused on the general mechanisms of seawater electrolysis and the performance of various catalyst materials without delving deeply into nickel-based materials.50,51 For example, unlike a recent review that focused on the impact of seawater composition on electrolysis technologies, this review provides a detailed analysis of material-specific strategies for improving catalyst performance.52 Specifically, it targets the unique properties and enhancements of nickel-based catalysts, making it distinct and highly relevant for researchers focused on non-precious metal electrocatalysts. Additionally, this review comprehensively outlines current progress in improving the catalytic efficiency of nickel-based materials for seawater electrolysis using modification approaches. It also discusses the corrosion resistance methods used for nickel-based materials in seawater conditions. By elucidating the application of diverse strategies and principles, it serves as a valuable resource offering insights and guidance for designing and enhancing more effective nickel-based electrocatalytic materials, thereby fostering the progress of seawater electrolysis technology. Furthermore, the consolidation of corrosion resistance information can contribute to improving the stability and long-term reliability of nickel-based materials within the seawater electrolysis setting.
2. Principle of electrolysis of seawater
To understand seawater electrolysis, it is first necessary to understand the chemical reactions involved and the associated mechanisms. In addition, catalyst performance parameters, such as electrochemical surface area (ECSA), will directly affect the efficiency of seawater electrolysis and the hydrogen production rate. Therefore, understanding the evaluation of catalyst performance parameters is essential to optimize the efficiency of the seawater electrolysis process. In the following, we will elaborate on the principles of seawater electrolysis, the chemical reactions involved, and the importance of catalyst performance parameters.
2.1 Reaction equation and mechanism of electrolysis of seawater
The main principle of seawater electrolysis is based on the electrolysis of water. Electrolysis of water is usually performed in an electrolyzer, which consists of two electrodes (cathode and anode) and an electrolyte solution (Fig. 1b).53 The theoretical potential required to electrolyze water is 1.23 V under standard conditions. However, the potential needed for the actual process will be higher than the theoretical potential, and this extra voltage beyond the theoretical potential is known as the overpotential.54 In the electrolysis process, by applying voltage to the electrolyte to make it undergo electrolytic reaction on the electrode material, H+ in the solution migrates to the cathode and is reduced to H2, and OH− migrates to the anode and is oxidized to O2. The reaction equation is as follows, where the voltage values provided in eqn (2) to (5) are relative to the standard hydrogen electrode (SHE):
Total reaction equation:
| 2H2O(l) → 2H2(g) + O2(g) | (1) |
OER in alkaline environments:
| 4OH− → 2H2O + O2 + 4e− E0anode = 0.4 V | (2) |
OER in acidic environments:
| 2H2O → 4H+ + O2 + 4e− E0anode = 1.23 V | (3) |
HER in an alkaline environment:
| 2H2O + 2e− → 2OH− + H2 E0cathode = −0.83 V | (4) |
HER in an acidic environment:
| 2H+ + 2e− → H2 E0cathode = 0 V | (5) |
The Nernst equation
shows that the different electrode potentials (E) under acidic and basic conditions are mainly due to the different concentrations of H+ and OH−.55 These concentration changes will affect the reaction quotient Q, thereby altering the electrode potential. The HER at the cathode is a two-electron transfer process regardless of the pH conditions. Under an acidic medium, the Volmer reaction occurs first, protons are first adsorbed on the active sites on the catalyst surface to form active H*. This is followed by the Tafel/Heyrovsky reaction to generate hydrogen, where two molecules of active H* are required to generate one molecule of H2 in the Tafel process, while one molecule of H+ is required to combine with one molecule of H* to generate one molecule of H2 in the Heyrovsky process.56 In an alkaline environment, the Volmer reaction also occurs first, with the difference that at this point, water molecules, rather than protons, adsorb on the active sites on the catalyst surface to give active H*. This is followed by the Tafel/Heyrovsky reaction to generate hydrogen, where two molecules of active H* are required to generate one molecule of H2 in the Tafel process. In contrast, one molecule of H2O is required to combine with one molecule of H* to generate one molecule of H2 in the Heyrovsky process.57Fig. 2a illustrates the process, and the reaction equation is shown below:
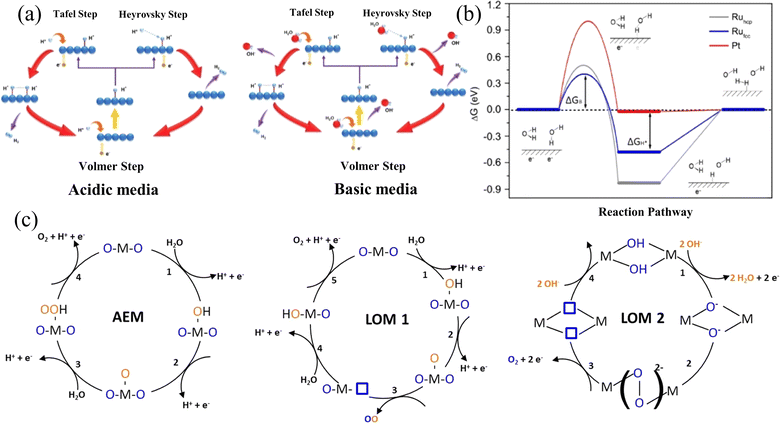 |
| Fig. 2 (a) Reaction pathways of HER in acidic and basic media. Reproduced with permission.15 Copyright 2021, Wiley-VCH. (b) Illustration of ΔGH* on various surfaces, depicting the reactant beginning state, intermediate state, final state, and an additional transition state symbolizing water dissociation. Reproduced with permission.16 Copyright 2021, American Chemical Society. (c) AEM, LOM1 and LOM2 mechanisms of the OER. Reproduced with permission.17 Copyright 2023, Springer Nature. | |
In acidic environments:
Volmer reaction:
Tafel reaction:
Heyrovsky reaction:
In alkaline environments:
Volmer reaction:
| * + H2O + e− → H* + OH− | (9) |
Tafel reaction:
Heyrovsky reaction:
| H* + H2O + e− → * + OH− + H2 | (11) |
The Gibbs free adsorption energy of H* (ΔGH*) is commonly used in HER to react with the electrochemical properties of catalysts.58 The larger the negative deviation of the value of ΔGH* from zero, the stronger the adsorption behavior of H*, while the larger the positive deviation, the weaker the adsorption behavior of H*. When the value of ΔGH* is too small, it favors the Volmer reaction but is unfavorable for the subsequent Tafel/Heyrovsky reaction. Conversely, if the value is too large, the Volmer reaction cannot easily occur, reducing the amount of H* available for the subsequent reaction, which is macroscopically unfavorable to the HER. When ΔGH* is closer to 0 indicates that the HER performance of the catalyst is better (Fig. 2b). The researchers found that acidic conditions are more favorable for HER, however, nickel-based materials are more susceptible to corrosion under acidic conditions than alkaline conditions.59,60 So, the focus in the study of HER catalysts for nickel-based materials is to enhance their corrosion resistance or to prepare catalysts under alkaline conditions to meet industrial needs, which can be done by metal doping to modulate the electronic structure of the active site to bring ΔGH* closer to 0. In addition, in seawater, which is rich in a large number of microorganisms and other ions, the pH near the cathode fluctuates during seawater electrolysis, which can cause cations such as Mg2+ and Ca2+ in seawater to generate insoluble Mg(OH)2 and Ca(OH)2 to cover the electrode surface, blocking the active sites on its surface. To address the aforementioned challenges, it is imperative to optimize the electrolysis conditions or implement alternative measures to avert poisoning and deactivation of the catalyst.
Compared to the two-electron transfer HER process, the OER process (Fig. 2c) involving four-electron transfer has a slower reaction rate. The figure illustrates three distinct OER mechanisms: the Acidic Environment Mechanism (AEM), Lattice Oxygen Mechanism 1 (LOM 1), and Lattice Oxygen Mechanism 2 (LOM 2). In the AEM, hydroxyl groups (OH−) form on the metal-oxide surface, which subsequently release oxygen (O2), hydrogen ions (H+), and electrons (e−).61 The LOM 1 involves lattice oxygen atoms forming a peroxo species (O–O) that bridges metal-oxide sites and then decomposes to release oxygen (O2). Meanwhile, in LOM 2, two hydroxyl groups (OH−) combine to form a peroxo species (O–O), which also decomposes to release oxygen (O2).62 These mechanisms underscore the complexity and multistep nature of the OER, significantly contributing to its slower reaction rate compared to the HER. This highlights the critical need for developing more efficient and stable catalysts for water splitting applications. In addition, seawater is more than 55% chloride, and there are also other anions such as sulfate ions, bromide, etc. These anions compete with the OER at the anode.63 Below, we describe the CER during the electrolysis of seawater using the reaction equation for chloride ions as an example. The reaction equation is as follows:
In strongly acidic environments (pH < 3)
| 2Cl− → Cl2 + 2e− E0anode = 1.36 V | (12) |
In a weakly acidic environment (3 < pH < 7.5)
| Cl− + H2O → HClO + H+ + 2e− E0anode = (1.494 − 0.0295 pH) V | (13) |
In alkaline environments (pH > 7.5)
| Cl− + 2OH− → ClO− + H2O + 2e− E0anode = (1.795 − 0.0591 pH) V | (14) |
Although the kinetically more favorable CER can macroscopically accelerate the reaction rate and promote hydrogen production, chloride ions are corrosive and can cause severe damage to the electrodes. Based on the Pourbaix diagram (Fig. 3f), Dionigi et al. found that the potential difference between hypochlorite and oxygen under alkaline conditions, when pH > 7.5, is fixed at 0.48 V, and they proposed that the difference between the OER and the standard potential for hypochlorite generation (ΔE0) be used as the condition for the selective operation of the OER.26 In other words, the reaction under alkaline conditions is more favorable for improving the selectivity of the OER and inhibiting the reaction of CER. Developing electrocatalysts with high selectivity and corrosion resistance is crucial due to seawater's corrosive nature of free chlorine ions, which can degrade electrode materials.64 This advancement is essential for reducing corrosion effects and improving electrolysis procedures' effectiveness.
2.2 Evaluation of catalyst performance parameters
In evaluating the catalytic performance of OER/HER electrocatalysts, the catalytic performance can be evaluated by the following important parameters: initial potential and overpotential, current density, Tafel slope, Faraday efficiency (FE), electrochemical surface area, and catalyst stability.
2.2.1 Initial potential and overpotential.
The initial potential (E) is the turning point on the polarization curve (or cyclic voltammetry) from a non-Faraday current to a Faraday current. Overpotential (η) is the fraction of the actual potential greater than the theoretical potential required to achieve a certain current density in an electrochemical reaction (Fig. 3c). For HER and OER, the overpotential is the portion of the potential greater than the standard electrode potential required at a current density of 10 mA cm−2 in order for the corresponding water reduction or oxygen precipitation reaction to take place at the electrode, and its unit is usually expressed in the voltage unit V. In general, a low onset/overpotential means that the catalyst can start promoting reactions at a low potential, which reflects the high activity and efficiency of the catalyst. Conversely, it means that the catalyst is less active. In electrochemical experiments, the starting potential and overpotential of a catalyst can be read from its LSV curve. The overpotential of a catalyst is influenced not only by its intrinsic activity but also by elements like the electrolyte environment. Therefore, relying solely on this parameter to assess the catalyst's performance may be inadequate.
2.2.2 Current density.
Current density (J) is the value of current passing through a unit area, usually in mA cm−2. Current density can directly reflect the activity and efficiency of a catalyst in catalyzing a reaction. A high current density means that the catalyst is able to promote the reaction at a higher rate, while a low current density may mean that the catalyst is inefficient or less active. However, high current densities are often accompanied by more severe electrochemical corrosion and the generation of large numbers of bubbles, which can damage the catalyst. Therefore, researchers need to select suitable catalyst carriers or add adhesives to enhance adhesion properties and use corrosion-resistant materials to enable electrocatalysts to reach higher current densities. Similarly, the LSV curve can reflect the current density of the catalyst at different potentials.
2.2.3 Tafel slope.
According to the Tafel equation (η = a + b
lg(|j|)), where a is the Tafel constant, the Tafel slope (b) reflects the potential difference required for each tenfold increase in current density (Fig. 3e). In electrochemical experiments Tafel slope can be obtained by fitting LSV curves, the smaller the value the smaller the overpotential required for the surface to reach the same current density and the faster the reaction rate. In addition, according to the Butler–Volmer equation, it is known that the magnitude of the Tafel slope can be used to determine the decisive speed step in the reaction process and reveal the corresponding mechanism of the reaction. High-performance electrocatalysts often demonstrate characteristics such as higher current density and lower Tafel slope, as shown in Fig. 3d.
2.2.4 Faraday efficiency.
FE is the efficiency with which energy is transferred from electrical energy to chemical energy and stored in the product oxygen/hydrogen gas. That is, the ratio between the experimentally produced oxygen/hydrogen gas and the theoretically calculated gas volume is a measure of the efficiency of the applied current to drive the expected OER/HER. The actual gas production can be determined by the water–gas displacement method and the gas chromatography (GC) method, and the theoretical gas production can be calculated by Faraday's second law.65,66 Due to the presence of chloride ions in the seawater, a CER occurs at the anode, which can reduce the Faraday efficiency. Faraday efficiency is an important indicator of catalyst activity and efficiency. A high Faraday efficiency is crucial for catalysts as it indicates their ability to drive the HER and OER with high efficiency. This is particularly important for enhancing the efficiency of seawater electrolysis. Achieving a Faraday efficiency close to 100% is ideal for the development of efficient catalysts. At the same time, the Faraday efficiency can also provide important information about the active sites on the catalyst surface and the reaction mechanism.
2.2.5 Electrochemical surface area.
A catalyst's ECSA refers to the surface area that contains effective reactive active sites crucial for electrochemical reactions. Active sites are particular areas on the catalyst surface that can adsorb reactive chemicals and promote the intended reactions. The ECSA is critical for evaluating the catalyst's performance as it directly affects its activity and stability. A larger ECSA implies that more active sites on the catalyst are available for the reaction, leading to an increased reaction rate. The ECSA can be determined from non-Faraday currents, with one method involving the measurement of double electric layer capacitance (Cdl). The bilayer capacitance represents the capacitance between the electrode surface and the electrolyte. When scanning in the bilayer region, the current obtained is proportional to the scanning speed, and the slope can indicate the magnitude of the Cdl. The ratio of the bilayer capacitance to the capacitance of a planar electrode is defined as the roughness factor. The electrochemically active surface area can be calculated by multiplying the roughness factor with the geometric area. Generally, a larger electrochemical surface area corresponds to higher catalyst activity, highlighting the importance of ECSA in enhancing the performance of catalysts in electrochemical reactions.
2.2.6 Stability.
Stability refers to the ability of a catalyst to maintain its performance and structure over long periods of operation or cycling. Catalyst stability is critical in evaluating catalyst performance because prolonged use can lead to gradual deterioration of catalyst performance or structural damage, reducing the efficiency of the electrolysis process. Because seawater contains a variety of salts and impurities, these components may cause corrosion, scaling, or other undesirable reactions to the catalyst, affecting its performance and lifetime. Therefore, the stability of catalysts in electrolyzed seawater needs to be carefully evaluated. The stability of electrocatalysts is usually characterized using several methods, including chrono-potentiostatic (CP) and chronoamperometry (CA). In CP, the stability of catalysts in electrolytic seawater can be assessed by applying a stabilized potential during the electrolysis reaction to simulate the catalyst's prolonged operation. By monitoring the change in current over time, the change in performance of the catalyst during long time operation can be assessed, as well as its stability. In CA, a constant current is applied, and the change in potential is monitored over time. This method helps in understanding the durability of the catalyst under constant operational conditions and provides insights into how the catalyst's performance changes due to potential corrosion or other degradation processes in seawater. In addition, accelerated cyclic voltammetry (CV) and long-term galvanostatic electrolysis are also effective methods to assess electrocatalyst stability. CV involves applying different voltage ranges through multiple cycles in a short period to simulate long-term usage, with changes in CV curves indicating stability and performance in seawater. Long-term galvanostatic electrolysis applies a constant current over an extended period, monitoring resulting voltage changes to evaluate durability under continuous seawater operation.
The catalytic performance of OER/HER electrocatalysts can be evaluated based on the critical parameters mentioned above. The initial potential and overpotential indicate the catalyst's activity level, while current density reflects its efficiency in promoting reactions. The Tafel slope reveals the potential difference required for increased current density and helps determine the reaction mechanism. Faraday efficiency measures the energy transfer efficiency in driving OER/HER. The electrochemical surface area indicates the active sites available for reactions, and catalyst stability is crucial for maintaining performance over time, especially in seawater electrolysis. Stability assessments involve monitoring performance changes during prolonged operation and resistance to corrosion and degradation.
3. Nickel-based electrocatalysts
The intricate four-electron transfer process of OER, coupled with the challenge of excessive overpotential and the competition with CER on the anode, have emerged as significant research bottlenecks impeding the commercial advancement of hydrogen production from electrolytic seawater.61 Additionally, it is necessary to research HER catalysts that have a greater number of active sites. Developing electrocatalysts with high catalytic activity and stability can reduce the energy barrier of the reaction and speed up the process, which is an important area of research.15,17 More rational strategies have been designed from different perspectives to address the above challenges to meet the large-scale commercialization needs of electrolytic seawater. Nickel-based materials show great potential as electrocatalysts due to their plentiful availability, cost efficiency, environmental friendliness, and the ability to control their electrical structure easily. For example, Liang et al. synthesized highly efficient HER catalysts from nickel-doped tungsten oxide nanorods (Ni–WOx) using the property that Ni doping can optimise the activity of hydrogen precipitation reactions (Fig. 4d–g).69 In electrocatalysis, many electrocatalysts based on nickel-based materials have demonstrated striking bifunctional properties compared with traditional electrocatalysts. Specifically, many nickel-based catalysts are not only able to catalyze OER effectively but also able to catalyze HER simultaneously in the same system. Therefore, nickel-based catalysts have attracted much attention in electrocatalysis research and offer new possibilities for achieving efficient energy conversion (Fig. 4a–c).67,70
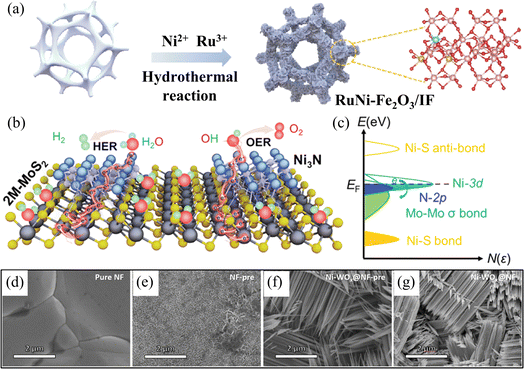 |
| Fig. 4 (a) Synthesis of bifunctional RuNi–Fe2O3/IF by hydrothermal method. Reproduced with permission.67 Copyright 2022, Elsevier. (b) Schematic illustration of water splitting process of the Ni3N@2M-MoS2. (c) A proposal for charge modulation of active electronic states in Ni3N@2M-MoS2 with bifunctionality. Reproduced with permission.68 Copyright 2022, Wiley-VCH. (d) SEM image of NF. (e) SEM image of NF-pre. (f) SEM image of NiWOx@NF-pre. (g) SEM image of Ni–WOx@NF. Reproduced with permission.69 Copyright 2023, Elsevier. | |
In practical applications, nickel-based materials still face some challenges as electrocatalysts, such as the low electronic conductivity of a single nickel-based material and the insufficient stability of synthesized electrode materials.71 Therefore, the current research focuses on modifying nickel-based materials by regulating the morphology of nickel-based materials, doping with metallic elements or non-metallic elements to construct efficient nickel-based catalysts, and controlling the reaction kinetics.72 This section provides an overview of the design and synthesis, structural characterization, and methods to improve the catalytic efficiency of metal-doped, non-metal-doped, and other nickel-based catalysts for OER and HER.
3.1 Nickel-based catalysts for OER
For the OER, nickel-based materials are potential OER catalysts for electrolytic seawater due to their tunable three-dimensional (3D) electronic configurations, diverse crystal structures, and highly reactive end states during the OER process.73 Researchers have constructed efficient nickel-based catalysts and modulated reaction kinetics by tuning the morphology of nickel-based materials, doping metallic elements, or modifying nickel-based materials with non-metallic elements. This section summarizes the domestic and international reports of OER catalysts in recent years.
3.1.1 Nickel-based oxides and layered double hydroxides.
Recently, significant research have been achieved on nickel-based oxides (NiO) in OER, and various strategies have been used to improve their catalytic OER activity. NiO exhibit efficient catalytic activity for the OER by leveraging the synergistic effect at the interface between nickel and nickel oxide (Fig. 5a). This effect lowers the energy barrier for hydrolysis dissociation and promotes the creation of intermediate products (Fig. 5b).74,78 Based on the morphology theory, Khadijeh et al.75 developed nanorod array-based hierarchical NiO microspheres to turn bare nickel foam into hydrophilic NiO. This procedure can transform the nickel foam surface into a complex porous structure like a sea urchin, enhancing surface area, active sites, and corrosion resistance (Fig. 5c). The catalyst exhibited high stability and selectivity throughout a continuous week of electrolysis. Furthermore, the study illustrated the effectiveness of Ni–Fe oxides as anode materials. Theoretical analysis indicated that the incorporation of Fe at the edge of Ni oxides not only enhances the turnover frequency (TOF) but also improves the durability compared to single-metal Ni oxides (Fig. 5d and e).76 Zhang et al. prepared a nickel oxide catalyst, Fe–NiOxHy/CC, doped with a small amount of Fe. The overpotential required for this catalyst was only 206 mV under simulated seawater conditions at a current density of 10 mA cm−2.77 In addition, the catalyst showed remarkable stability in simulated seawater. The researchers analyzed the catalyst by density functional theory (DFT) calculations. They learned that the presence of Ni–Fe bridging sites in the catalyst can regulate the precipitation of Cl*, which is beneficial to increase the OER activity (Fig. 5f). In addition, the construction of defective structures can provide additional reaction centers to electrify the coordination environment of atoms on the catalyst surface, which in turn modulates the electronic structure. The NiOx–FeOx nanocomposites synthesized by Haq et al. require only 380 mV overpotential to achieve a current density of 1000 mA cm−2, which is better than the overpotential required for IrO2 at this current density.79 Additionally, the catalyst is protected by g-C3N4, which helps resist pitting and stress corrosion at the anode, providing high corrosion resistance. The 3D hierarchical porous structure of g-C3N4 provides a short diffusion channel for ion transfer and mass transport, which is very favorable for seawater penetration. At the same time, the amorphous NCs have a unique self-healing and flexible structure to further improve corrosion resistance.
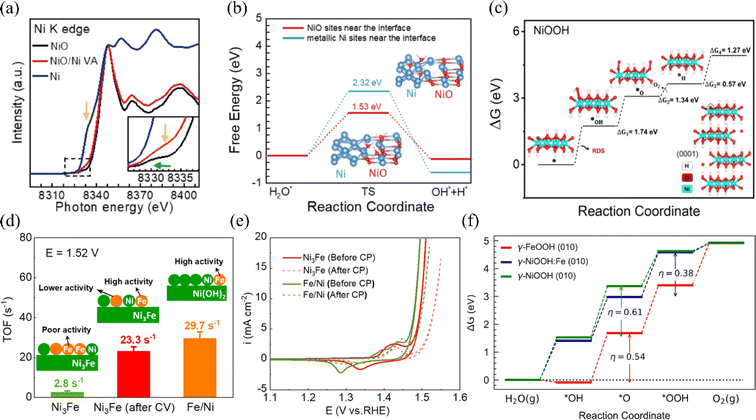 |
| Fig. 5 (a) The nickel K-edge X-ray absorption spectroscopy (XAS) technique can be used to characterize NiO, Ni/NiO VAN, and Ni hybrid systems. (b) Calculations have revealed the energy barriers for water dissociation kinetics at metallic Ni sites and NiO sites located near the interface. Reproduced with permission.74 Copyright 2022, Springer Nature. (c) The OER free-energy diagrams at U = 1.23 V for the O-terminated NiOOH surface have been calculated, using the LOM mechanism. Reproduced with permission.75 Copyright 2023, American Chemical Society. (d) TOF values for uncirculated Ni3Fe, Ni3Fe after 10 cycles between −0.4 and 1.45 V, and Fe/Ni(O) hydroxide catalysts. (e) The OER CV curves of Fe/Ni and Ni–Fe (oxy)hydroxides before and after the CP test. Reproduced with permission.76 Copyright 2022, American Chemical Society. (f) The theoretical OER free energy diagram for NiOOH, FeOOH, and Fe-doped NiOOH has been constructed. Reproduced with permission.77 Copyright 2023, American Chemical Society. | |
Based on the study of electrolyzed water, the researchers found that under alkaline circumstances, nickel–iron layered double hydroxide (NiFe-LDH) exhibits strong catalytic activity and stability for the OER.80–82 LDHs (layered bimetallic hydroxides) are interlayer structures consisting of positively charged bivalent metal ions and negatively charged oxide ions (e.g., hydroxide ions) arranged in a specific ratio (Fig. 6a). This unique structure allows LDHs to have a rich and tunable active surface. Also, it provides channels for the transfer of electrons and ions, which facilitates the rapid release of hydrogen and oxygen produced in the electrolysis reaction of water.85 However, due to the positively charged surface of LDHs, negatively charged chloride ions may be adsorbed on the surface during the electrolysis of seawater, resulting in the corrosion of the catalysts.86,87 Yao et al. introduced Ce into nickel foam NiFe LDH arrays to obtain Ce–NiFe LDH/NF, a strong electrocatalyst for seawater OER. The introduction of Ce serves dual roles during seawater electrolysis: it promotes the formation of the amorphous reactive material NiOOH, while simultaneously generating CeO2, which effectively inhibits carbonate evolution currents (CECs) (Fig. 6b and c).83 Different strategies to improve the OER performance of NF-LDH include the doping of high valence metal ions such as Mo and V. High valence metals can induce the formation of amorphous structures in nickel-based materials, or form defects and lead to the formation of oxygen vacancies, which increase the active sites.72 Qi et al. demonstrated that by intercalating hexavalent molybdenum ions Mo(VI) in NF-LDH, MoSO42− can modulate the electronic structures of Ni and Fe.84 This lowers the OER energy barriers and promotes the generation of NiOOH, thereby increasing the active sites of OER (Fig. 6d). In summary, in recent years, researchers have used various strategies to improve their reactivity, such as interfacial synergism, morphological modulation, and elemental doping, for the application of nickel-based oxides and hydroxides on electrolytic seawater. In addition, LDHs have been shown to have excellent catalytic activity and stability under alkaline conditions. Among them, nickel-based oxide catalysts doped with elements such as aluminum, phosphorus, and iron showed good activity and stability and high electrochemical surface area. Furthermore, the doping of high-valent metals such as molybdenum and vanadium was also found to help increase the number of active sites in nickel–iron bilayer hydroxides.
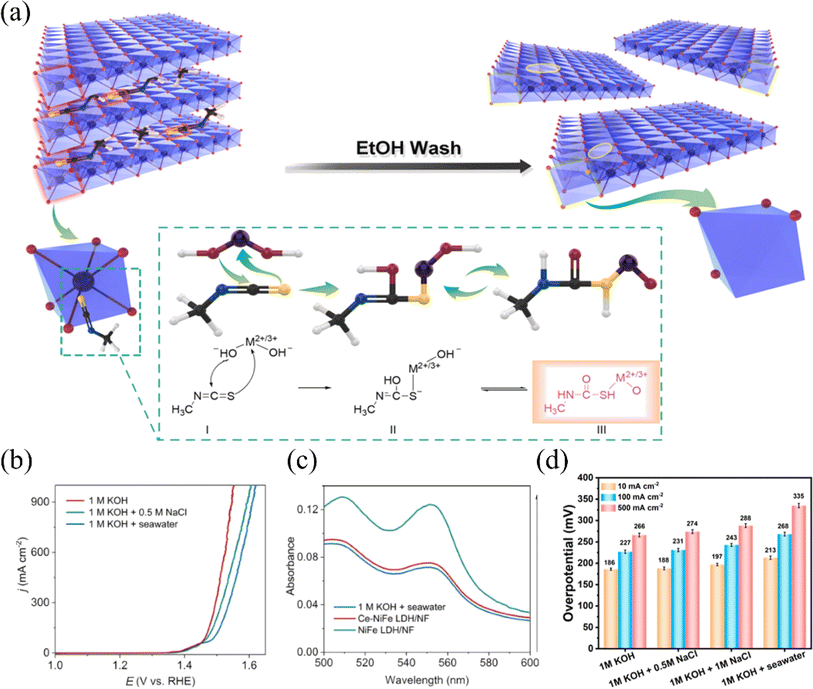 |
| Fig. 6 (a) Designing LDHs with metal and oxygen multivacancies requires targeting specific atoms to create these vacancies. Reproduced with permission.81 Copyright 2020, Elsevier. (b) Ce–NiFe LDH/NF was used as the catalyst, and LSV curves were measured in different electrolytes to evaluate its performance. (c) After the stability test, UV-vis absorption spectra of Ce–NiFe LDH/NF and NiFe LDH/NF electrolytes were obtained. Reproduced with permission.83 Copyright 2024, Elsevier. (d) Mo0.25–NiFe LDH at different current densities in different electrolytes, corresponding overpotentials were measured. Reproduced with permission.84 Copyright 2023, American Chemical Society. | |
3.1.2 Nickel-based phosphides.
Phosphorus, sulfur, nitrogen, and other anions in nickel-based catalysts can enhance the electrical configuration and catalytic sites on the catalyst's surface, increase the conductivity, and provide suitable adsorption strength for the intermediates.88 This section will focus on the recently reported nickel-based phosphides. Researchers have employed surface modification, nanostructure optimization, and electronic structure regulation to modify and tune nickel-based phosphides, aiming to enhance their intrinsic catalytic activity for the OER (Fig. 7h).91–93 Liu et al. synthesized acicular Fe–Ni2Pv through Fe doping and phosphorization on nickel foam, guided by DFT predictions (Fig. 7a).89 This material demonstrated bifunctional catalysis for OER and HER in an alkaline simulated seawater electrolyte. Fe doping combined with P vacancies promotes the regeneration of active sites on the OER surface and boosts the hydrogen adsorption free energy (ΔGH*) for the HER (Fig. 7b). In this case, Ni sites become the dominant OER active centers (Fig. 7c), while Fe atoms become the active centers of HER. In addition, the introduction of P vacancies also improves the electrical conductivity of this catalyst, enabling the Fe–Ni2Pv to achieve current densities of 1.0 and 3.0 A cm−2 to meet the industrial requirements at a low voltage of 1.68 V and 1.73 V, respectively, in a 6.0 M KOH environment at 60 °C (Fig. 7d). Using a typical in situ self-assembly strategy, Yu et al. synthesized Ni-based heterostructured catalysts (NiO/Ni3S2@Ni5P4) with phosphorylated layers to enhance the OER in seawater electrolysis. They experimentally demonstrated that the overpotentials of this catalyst for the OER in freshwater and seawater at a current density of 10 mA cm−2 were 280 mV and 310 mV, respectively, significantly lower than those of commercial RuO2 catalysts (Fig. 7e).90 This catalyst has outstanding OER performance is primarily due to the electronic synergy between the NiO/Ni3S2 heterostructure and the phosphide layer. This synergy activates the active sites of Ni, while the outer phosphide layer enhances corrosion resistance, structural compactness, and electron transfer rate on the catalyst's surface. Therefore, the catalyst can maintain stability for 100 h at a current density of 100 mA cm−2 (Fig. 7f and g).
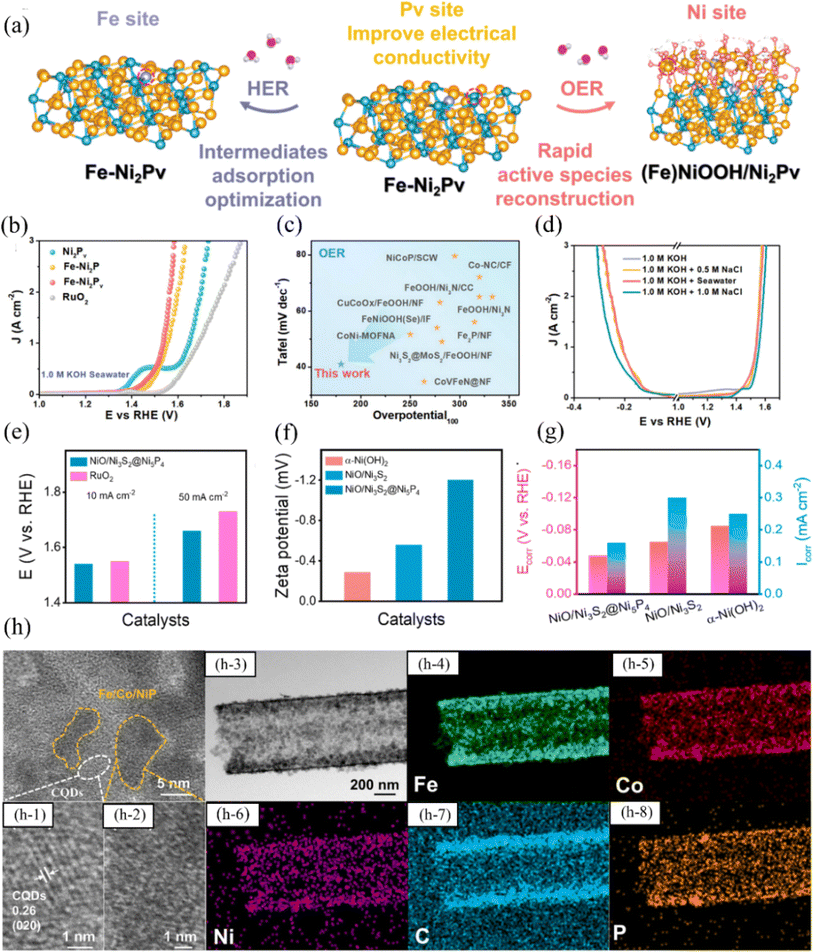 |
| Fig. 7 (a) Fe–Ni2Pv in 1.0 M KOH seawater electrolyte during HER and OER was represented schematically, showing the genuine phases and active sites. (b) Fe–Ni2Pv and comparison catalysts were subjected to iR-compensated LSV polarization testing for OER, and the resulting curves were analyzed and compared. (c) The Tafel slope and overpotential at 100 mA cm−2 of the Fe–Ni2Pv catalyst were compared with those of the recently reported OER catalysts. (d) Different electrolyte solutions were used to obtain polarization curves of the Fe–Ni2Pv catalyst with iR-correction. Reproduced with permission.89 Copyright 2023, Wiley-VCH. (e) The overpotentials for OER in seawater electrolytes were compared between NiO/Ni3S2@Ni5P4 and RuO2 catalysts. (f) Zeta potentials of NiO/Ni3S2@Ni5P4, NiO/Ni3S2, and α-Ni(OH)2 in seawater electrolyte. (g) The corrosion potentials and corrosion current densities in seawater electrolyte were compared among NiO/Ni3S2@Ni5P4, NiO/Ni3S2, and α-Ni(OH)2. Reproduced with permission.90 Copyright 2023, Elsevier. (h) Structural characterisation of FCNP@CQDs. The HRTEM images of a FCNP@CQDs: (h-1) show clear lattice spacing corresponding to the (020) plane, (h-2) show a low crystalline or amorphous phase of Fe/Co/Ni phosphide; and (h-3) TEM image of a FCNP@CQDs nanotube and the corresponding mapping for (h-4) Fe, (h-5) Co, (h-6) Ni, (h-7) C and (h-8) P. Reproduced with permission.91 Copyright 2023, Elsevier. | |
Fu et al. used electroplating to generate a series of Ni–P nano microspheres on an asbestos substrate that is both flexible and corrosion-resistant, and synthesized NiPx@HA with both catalytic HER and OER functions.94 Due to the strong chemisorption between the nickel-rich material and the two-dimensional layered substrate, and the hexahedral crystal structure of Ni5P4 enhancing electron transfer, the catalyst exhibits overpotentials of 208 mV for HER and 392 mV for OER under a current density of 200 mA cm−2. Furthermore, the catalyst maintains stability for 960 h at a current density of 500 mA cm−2. Moreover, large-area NiPx-based electrodes of 22 cm × 22 cm can be synthesized using elemental doping engineering, enabling large-scale hydrogen production in alkaline seawater electrolytes. Although some progress has been made in the study of nickel-based phosphide electrocatalysts in seawater electrolysis, some challenges still need to be addressed. Firstly, consideration needs to be given to how to scale up the preparation of nickel-based phosphide electrocatalysts, which requires the development of novel and simple methods. Currently, most of the research progress has been limited to the preparation of such catalysts on a small scale, and further research on how to realize the scale-up of the structures is still needed, which is essential for practical applications. In addition, nickel-based phosphides as OER catalysts also need to improve the reaction selectivity.95 Many studies primarily concentrate on achieving high current density in catalyst materials, often overlooking the critical issue of addressing catalyst oxidation.96 This oversight results in the durability of the catalyst not meeting the stringent standards required for industrial applications.
3.1.3 Nickel-based sulfides.
Similar to the phosphatization of nickel-based materials, many studies have been reported in recent years for sulfides of nickel-based materials. Since transition metal sulfides can be used to reconstruct the catalyst surface, based on this property, sulfide-based electrocatalysts have been successfully synthesized in direct electrolysis of water studies (Fig. 8a).97,101 The application of bimetallic sulfides in seawater electrolysis was explored for the first time by Wang et al. through NiCoS NSAs prepared by a simple one-step hydrothermal method. The surface-incorporated sulfides enhanced the selectivity and corrosion resistance of the catalysts. Furthermore, the NiCoS NSAs, with their large surface area backbone structure, enabled the catalysts to achieve a current density of 800 mA cm−2 at a voltage of 2.08 V in natural seawater, maintaining continuous operation for 100 h (Fig. 8b and c).98 Incorporating phosphorus and sulfur (P/S) into a single doped sulfide material exhibits a synergistic effect and robust coupling between these non-metallic elements. This unique property can be leveraged to create a nickel sulfide/phosphide interface, enabling the development of electrocatalysts for seawater electrolysis that offer both high activity and bifunctionality. Wang et al. prepared NiPS/NF microsphere electrocatalysts via a simple electrodeposition method. Phosphorization and sulfation modifications induced electron rearrangement at the heterointerface, facilitating easier adsorption of intermediates (Fig. 8d).70 Meanwhile, NiPS/NF is a bifunctional catalyst for OER and HER, and can reach a high current density of 100 mA cm−2 in alkaline seawater with only 344 mV and 188 mV overpotentials, respectively, without degradation. This performance is attributed to the synergistic effect of Ni2P and NiS2, which imparts excellent corrosion resistance to the catalyst. Luo et al. synthesized Ni3S2/Fe–NiPx with a spherical tesseract structure composed of nanocubes by directly etching Ni3S2 with potassium ferrocyanide. This unique structure exposed more active sites, and the synergistic action of P and S during the OER process created a defect-rich active intermediate layer of Ni(OH)2/Ni(Fe)OOH, thereby regulating the adsorption free energies of the intermediates.100 Ni3S2/Fe–NiPx in alkaline seawater requires only 351 mV overpotential at a current density of 1000 mA cm−2, with a stability of more than 225 h, and exhibits lower overpotentials (Fig. 8g). Combining the advantages of NiFe LDHs in catalyzing OER with the corrosion resistance of sulfide layers in electrolytic seawater. Sun et al.99 prepared NiFe-LDH-S by altering the surface morphology of NF-LDH via plasma treatment technique. The transition metal sulfide layer formed on the catalyst can effectively inhibit the adsorption of chloride ions due to electrostatic repulsion. NiFe-LDH-S exhibits a minimum overpotential of 296 mV at a current density of 100 mA cm−2 in simulated seawater (Fig. 8e and f). It shows negligible potential drop in a 12 h continuous CP test, demonstrating corrosion resistance and stability that meet industrial requirements.
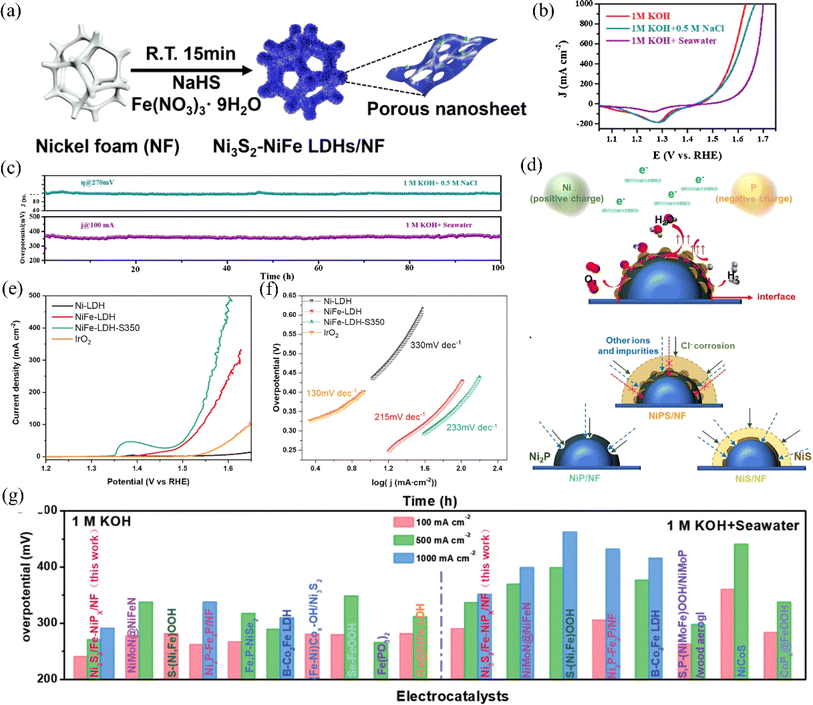 |
| Fig. 8 (a) An illustration depicting the synthesis process of Ni3S2–NiFe LDHs/NF. Reproduced with permission.97 Copyright 2022, Elsevier. (b) CV backward scan curves for the NiCoS electrode tested in different electrolytes. (c) The long-term stability of the NiCoS electrode in various electrolytes was evaluated through chronoamperometry and chronopotentiometry measurements. Reproduced with permission.98 Copyright 2021, Elsevier. (d) A schematic illustration explains the enhanced electrocatalytic activity and stability of NiPS/NF for water electrolysis in anodic seawater splitting. Reproduced with permission.70 Copyright 2022, Elsevier. (e) The electrochemical behavior of Ni-LDH, NiFe-LDH, and NiFe-LDH-S350 electrodes was evaluated using linear sweep voltammetry (LSV) measurements in an electrolyte comprising 1.0 M KOH and 0.5 M NaCl. (f) The Tafel slopes of the samples were determined by calculating the slope of the linear portion of the LSV curves. Reproduced with permission.99 Copyright 2021, Elsevier. (g) Ni3S2/Fe–NiPx/NF and other catalysts with excellent performance, were compared in terms of the overpotentials needed to reach current densities of 100, 500, and 1000 mA cm−2 for the OER in 1 M KOH and alkaline natural seawater. Reproduced with permission.100 Copyright 2022, Wiley-VCH. | |
3.1.4 Nickel-based nitrides.
Compared to nickel-based phosphides and nickel-based sulfides, nickel-based nitrides have slow kinetics of catalyst reactions due to the inherent chemical inertness of elemental N.102 However, nickel-based nitrides have also demonstrated good electrochemical properties by applying vacancy engineering, metal doping, etc. Wang et al. reported a 3D multilayered heterostructured nanoarray electrocatalyst (Ni3FeN@C/NF) (Fig. 9a) on which the N bonds contracted the d-band of the metal catalyst, obtaining a higher Fermi energy and exhibiting better electrochemical activity (Fig. 9c).103,106 Meanwhile, the synergistic coupling between the carbon coating covered on this catalyst and the Ni3FeN encapsulated within it ensures the electron transport flux. The final assembled electrolytic seawater tank can realize a current density of 500 mA cm−2 at a low cell voltage of 1.91 V (Fig. 9b). In addition to single N-doped compounds, Zhao et al. enhanced electrocatalytic activity by creating an interface between nitride and sulfide materials. They reported a NiNS electrode synthesized via a simple one-step calcination process, using nickel foam and thiourea under vacuum conditions.104 The electrode is bifunctional with excellent catalytic properties, and the interfacial structure between Ni3N and Ni3S2 served as an electrochemically active site and contributed to the dissociation and adsorption of water molecules. The overpotentials of this catalyst for HER/OER were 197 mV and 404 mV, respectively, at 100 mA cm−2 current, which was significantly better than those of most non-metal-based electrocatalysts, such as Ni3N–Co and Co3Se4/Co (Fig. 9d). Sun et al. synthesized sandwich-like NiCoHPi@Ni3N/NF by electrodepositing bimetallic nickel cobalt hydrogen phosphate (NiCoHPi) onto the surface of nickel nitride (Ni3N) supported on nickel foam substrate.105 The synergistic effect of the NiCoHPi deposition layer and Ni3N substrate enhances charge redistribution and electron transfer on the catalyst surface. Additionally, vertically grown nanosheet arrays on nickel foam increase the electrocatalytic surface area, providing more active sites. At the same time, this hierarchical three-dimensional porous structure also promotes mass transfer. Therefore, NiCoHPi@Ni3N/NF exhibits excellent bifunctional catalytic performance in simulated seawater electrolyte, with the overpotentials of 174 mV and 365 mV for HER and OER, respectively, at a current density of 100 mA cm−2, and exhibits a stability of more than 120 h at 200 mA cm−2 (Fig. 9e–g).
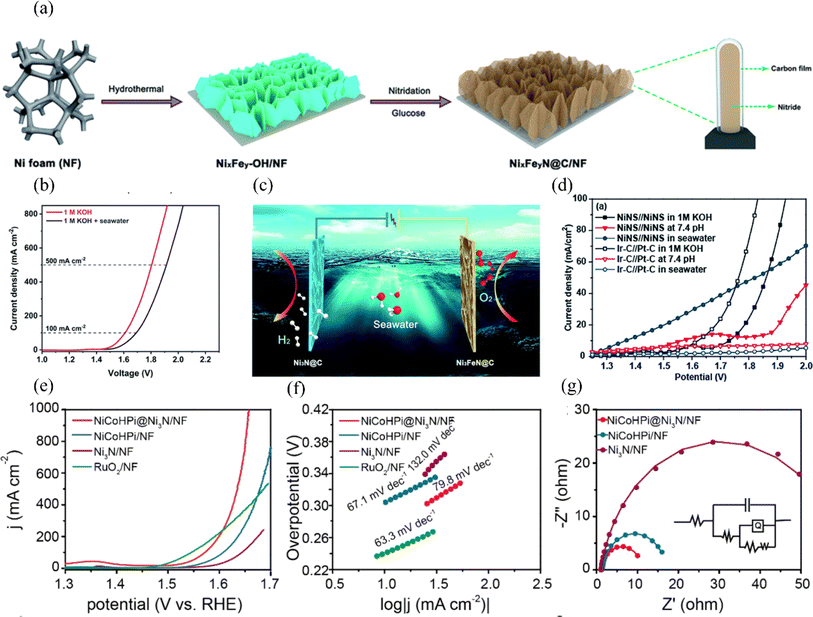 |
| Fig. 9 (a) Synthesis process of NixFeyN@C/NF. (b) The polarization curves of the electrolyzer were obtained by testing it in electrolytes consisting of 1 M KOH and a mixture of seawater and 1 M KOH. (c) A visual representation of the electrolyzer is depicted, where Ni3FeN@C/NF is used as the anode and Ni3N@C/NF serves as the cathode. Reproduced with permission.103 Copyright 2021, Royal Society of Chemistry. (d) In 1.0 M KOH electrolyte, polarization curves were obtained for the NiNS//NiNS and Ir–C//Pt–C overall water splitting systems. Additionally, polarization curves were also measured in buffer solution with a pH of 7.4 and seawater. Reproduced with permission.104 Copyright 2019, Royal Society of Chemistry. (e) LSV curves of NiCoHPi@Ni3N/NF, NiCoHPi/NF, Ni3N/NF, and Pt/C/NF samples. (f) Tafel plots of NiCoHPi@Ni3N/NF, NiCoHPi/NF, Ni3N/NF, and Pt/C/NF samples. (g) Nyquist plots were obtained at a potential of 1.49 V vs. RHE for the NiCoHPi@Ni3N/NF, NiCoHPi/NF, Ni3N/NF, and Pt/C/NF samples. The polarization curves were also obtained with an 85% iR correction. Reproduced with permission.105 Copyright 2022, Elsevier. | |
Nickel-based phosphides/sulfides/nitrides can enhance the OER performance by adjusting the proportions and configurations of various elements. Enhancements in catalyst structure optimization, electrocatalytically active site density, electron transfer rate, and surface catalytic activity can greatly improve its OER performance.107,108 Furthermore, it is essential to thoroughly evaluate and enhance the stability and activity of the catalysts in various settings and circumstances to improve the efficiency of the OER reaction process.
3.1.5 Other nickel-based catalysts.
In addition to the catalysts reported above, in recent years researchers have also utilized other methods to modify nickel-based catalysts. Metal-doped nickel-based catalysts have essential application prospects in OER.109 By doping different metal elements, the electronic structure and surface active sites of the catalysts can be modulated to enhance their catalytic activity and stability. It was discovered that doping extremely small quantities of noble metals can improve the catalyst's intrinsic electronic structure and increase the catalyst's exposure to more active sites.110 Cui et al. synthesized lily-like RuNi–Fe2O3/IF catalysts with ultra-low Ru doping using a one-step hydrothermal method, and they found that the appropriate Ru/Ni molar ratio has a significant effect on the formation of lily-like morphology.67 Meanwhile, the doping of trace noble metal Ru could lead to the exposure of highly active sites on the catalyst surface. Ru doping could also modulate the electronic structure of Ni, which led to the charge redistribution and significantly enhanced the charge number near the Fermi energy. In addition, the applied density of states (DOS) indicates that the DOS of RuNi–Fe2O3/IF at the Fermi energy is significantly increased compared to Fe2O3, which suggests that more electrons are involved in the catalytic reaction, the conductivity of the catalyst is improved, and the catalytic activity increases. Ultimately, RuNi–Fe2O3 achieved an overpotential of only 329.0 mV with a current density of 100 mA cm−2. In addition to doping trace amounts of noble metals, the strategy of bimetallic co-doping can also significantly improve the performance of the electrocatalysts due to the coordinated interactions between the constituent metals.111 Huang et al. designed CoFe–Ni2P catalysts with layered nanosheet morphology via secondary hydrothermal doping of Fe and Co onto nickel phosphide.112 Fe heteroatoms enhance electrical conductivity, while Co heteroatoms accelerate electrode surface self-reconstruction. It is worth noting that the co-doping of Co and Fe during the synthesis process caused the intermediate product CoFe–Ni(OH)2 to be “melted” after the phosphatization process, which became a solidifying agent and bonded with the neighboring microchips to form a robust microsphere structure. The layer-by-layer lamellar structure is substantial, which ensures that the CoFe–Ni2P electrode maintains good solidity and efficient electron transfer during high-current-density electrolysis in seawater environments (Fig. 10a and b). Ultimately, CoFe–Ni2P requires only a low overpotential of 304 mV to achieve a current density of 500 mA cm−2 in a seawater electrolyte of 6 M KOH while operating stably for 600 h.
 |
| Fig. 10 (a) A reaction energy diagram was constructed to illustrate the OER at the Ni sites on the surface of Ni2P, Fe–Ni2P, Co–Ni2P, and CoFe–Ni2P materials. (b) The adsorption energies of *Cl and *OH species at the Ni sites on the surfaces of NiOOH, Fe–NiOOH, Co–NiOOH, and CoFe–NiOOH were assessed. Reproduced with permission.112 Copyright 2023, Elsevier. (c) The charge density difference of γ-(NiFe)OOH after the reformation with MoO42− was visualized, where yellow regions indicate electron accumulation and blue regions indicate electron depletion. (d) Visualization of the projected density of states (pDOS) plots for Mo-4d, Ni-3d, and O-2p in the NiMoFe/NM and NiFe/NM systems. (e) The calculated values of the interfacial charge transfer (ICOHP) and a schematic illustration depicting the reduced strength of oxygen-intermediate adsorption in the NiMoFe/NM system. Reproduced with permission.113 Copyright 2023, Wiley-VCH. (f) The efficient water electrocatalysis activity mechanism diagram, highlighting the roles of CoFeLDH@Ni2P in the process. Reproduced with permission.114 Copyright 2024, Elsevier. | |
Ni-based alloy catalysts are highly suitable for OER applications due to their intrinsic polarity arising from the incorporation of different metals. They also boast high mechanical strength, excellent conductivity, and a variety of valence state transitions. Li et al. synthesized a high-performance oxygen evolution electrode, NiMoFe/NM, via thermal shock, exhibiting excellent OER activity and stability for over 1500 h in simulated alkaline seawater and 550 h in natural seawater.113 The oxidized NiFeMo electrode forms quickly, and its amorphous surface provides more defect sites, enhancing OER activity (Fig. 10c and d). At the same time, the NiMoFe/NM system reduces the energy barrier of adsorption of translation intermediates (Fig. 10e). In a recent report, Xu et al.114 prepared CoFeLDH@Ni2P electrocatalysts using cobalt-iron layered double hydroxides and nickel phosphide by hydrothermal and electrodeposition industries. The strong coupling effect between the amorphous cobalt–iron layered double hydroxide interface and the crystalline nickel phosphide interface alters the electronic configuration of the catalyst's active sites. This design approach enhances the electrocatalyst's performance by featuring amorphous–crystalline interfaces. Stability analysis shows the catalyst undergoes unique reconstructive behavior during the OER, forming bimetallic co-precipitated NiOOH (CoFe–NiOOH) and Ni(OH)2, which enhance OER activity and corrosion resistance (Fig. 10f). The catalyst requires a lower overpotential of 398 mV to achieve a current density of 100 mA cm−2 in alkaline seawater electrolyte, surpassing most reported nickel-based materials (Table 1).
Table 1 Performance of recently reported nickel-based OER electrocatalysts
Catalysts |
Electrolyte |
Overpotential for specific current density |
Stability |
Ref. |
NRAHM-NiO/NF |
1.0 M KOH + seawater |
340 mV@100 mA cm−2 |
100 h@500 mA cm−2 |
75
|
Fe–NiOxHy/CC |
1.0 M KOH +0.6 M NaCl |
206 mV@10 mA cm−2 |
12 h@10 mA cm−2 |
77
|
NiOx–FeOx@g-C3N4/NF |
1.0 M KOH + seawater |
380 mV@1000 mA cm−2 |
100 h@1000 mA cm−2 |
79
|
Ce–NiFe LDH/NF |
1.0 M KOH + seawater |
390 mV@1000 mA cm−2 |
500 h@1000 mA cm−2 |
83
|
Mo0.25–NiFe LDH/NF |
1.0 M KOH + seawater |
335 mV@500 mA cm−2 |
500 h@500 mA cm−2 |
84
|
NiMoFe/NM |
1.0 M KOH + seawater |
241 mV@10 mA cm−2 |
1500 h@100 mA cm−2 |
113
|
Fe–Ni2Pv/NF |
1.0 M KOH + seawater |
306 mV@1000 mA cm−2 |
100 h@100 mA cm−2 |
89
|
NiO/Ni3S2@Ni5P4/NF |
1.0 M KOH + seawater |
310 mV@10 mA cm−2 |
100 h@100 mA cm−2 |
90
|
NiPx/HA |
1.0 M KOH +0.5 M NaCl |
392 mV@200 mA cm−2 |
960 h@500 mA cm−2 |
94
|
NiCoS NSAs/NF |
1.0 M KOH + seawater |
440 mV@500 mA cm−2 |
100 h@100 mA cm−2 |
98
|
NiPS/NF |
1.0 M KOH + seawater |
392 mV@500 mA cm−2 |
60 h@200 mA cm−2 |
70
|
Ni3S2/Fe–NiPx |
1.0 M KOH + seawater |
351 mV@1000 mA cm−2 |
225 h@1000 mA cm−2 |
100
|
NiFe-LDH-S |
1.0 M KOH +0.5 M NaCl |
296 mV@100 mA cm−2 |
12 h@100 mA cm−2 |
99
|
Ni3FeN@C/NF |
1.0 M KOH +0.5 M NaCl |
351 mV@500 mA cm−2 |
100 h@100 mA cm−2 |
103
|
NiNS/NF |
1.0 M KOH + seawater |
404 mV@100 mA cm−2 |
12 h@100 mA cm−2 |
104
|
NiCoHPi@Ni3N/NF |
1.0 M KOH + seawater |
396 mV@100 mA cm−2 |
120 h@200 mA cm−2 |
105
|
RuNi–Fe2O3/IF |
1.0 M KOH + seawater |
497 mV@1000 mA cm−2 |
100 h@100 mA cm−2 |
67
|
CoFe–Ni2P/NF |
6.0 M KOH + seawater |
304 mV@500 mA cm−2 |
600 h@500 mA cm−2 |
112
|
CoFeLDH@Ni2P/NF |
1.0 M KOH +0.5 M NaCl |
363 mV@100 mA cm−2 |
55 h@100 mA cm−2 |
114
|
3.2 Nickel-based catalysts for HER
For the HER, Ni has the smallest |ΔGH*| among all transition metals, the ability to reach the maximum current density at the same overpotential, and the ability to tune the electronic configuration of the surrounding elements, which contributes to the enhancement of the HER activity.69,115 Researchers have modified nickel-based materials by defect engineering and elemental doping to construct efficient nickel-based HER catalysts. This section summarizes the HER catalysts reported in recent years at home and abroad.
3.2.1 Nickel-based catalysts with noble metal traces.
It was found that the doping of small amounts of noble metals can improve the performance of catalysts in several ways compared to non-precious metal catalysts.116 Firstly, small quantities of precious metal doping improved the surface structure of the catalysts and increased the number of active sites on their surfaces. This enables the catalyst to provide more effective reaction sites, thus enhancing the reaction activity.117 Secondly, doping with a small amount of noble metals enhances the electron arrangement and transport properties on the catalyst surface. This modulation of the electronic structure increases the adsorption and electron transfer rate of reactants, thereby promoting the catalytic reaction.110,118 Therefore, doping small amounts of precious metals play an essential role in enhancing catalytic activity and promoting HER.119 Through the combined effect of these mechanisms, precious metal doping can exert its unique catalytic properties, providing new strategies and solutions for efficient energy conversion and storage. Using this strategy, Zhai et al. doped a small amount of Ir onto a nickel–iron-based MOF substrate with a high specific surface area using a one-step hydrothermal method, resulting in MIL-(IrNiFe)@NF (Fig. 11b).120 The tri-metal synergy significantly increased the active sites on the catalyst surface, enhancing its overall catalytic performance. The addition of Ir induces the formation of a unique microsphere structure on the catalyst surface, while the MOF structure enhances ion diffusion paths. Additionally, the nickel foam substrate increases the surface's electrical conductivity, further improving the catalyst's performance. Combined with the above reasons, the MOFs (MIL-(IrNiFe)@NF) have excellent HER catalytic activity as well as corrosion resistance in alkaline seawater electrolytes. It only requires 235 mV to catalyze the HER to reach a current density of 1000 mA cm−2 in alkaline natural seawater (Fig. 11a). Hu et al. prepared Pt–Ni@NiMoN by ammonolysis after embedding and loading a very low amount of Pt (0.07 wt%) onto transition metal nitride (NiMoN) carriers (Fig. 11c).121 This resulted in Pt–Ni@NiMoN exhibiting superior HER catalytic properties compared to commercially available 20 wt% Pt/C electrocatalysts. Due to the strong inter-electronic interactions between Pt and other atoms and enhanced water polarization, the catalyst has an overpotential of only 90 mV in seawater up to a current density of 500 mA cm−2 and remains stable for more than 200 h in a highly concentrated (2 M) seawater sodium chloride solution, which has the potential to be used in large-scale industrial applications (Fig. 11d and e). Based on a similar strategy, Su et al. combined ultra-low-content Pt with substable-phase hexagonal close-packed Ni to prepare Pt-hcp Ni NBs (Fig. 11f), whose unique crystal structure optimizes the adsorption process of the reaction intermediates, with an overpotential of 137 mV at 10 mA cm−2 in real seawater electrolysis.122 Although the doping of trace precious metals will improve the activity of nickel-based catalysts, due to the scarcity and high cost of precious metals, gradually finding methods to replace the doping of trace precious metals will be a future research direction.
 |
| Fig. 11 (a) Comparison of the OER polarization curves for the electrolyzer containing MIL-(IrNiFe)@NF catalyst in two different electrolyte conditions: 1.0 M KOH + seawater and 1.0 M KOH + seawater + 0.5 M N2H4. (b) Illustrative diagram depicting the step-by-step formation process of the MIL-(IrNiFe)@NF catalyst. Reproduced with permission.120 Copyright 2021, Royal Society of Chemistry. (c) Calculated DOS for Ni@NiMoN and Pt–Ni@NiMoN. Bare NF, NiMoO4/NF, Pt–NiMoO4/NF, Ni@NiMoN/NF, Pt–Ni@NiMoN/NF and Pt–C/NF in 1 M KOH + real seawater. (d) The LSV curves. (e) The Tafel plots. Reproduced with permission.121 Copyright 2023, Royal Society of Chemistry. (f) Enlarged view of Ni sites and the Pt site mechanism in the reaction. Reproduced with permission.122 Copyright 2023, American Chemical Society. | |
3.2.2 Nickel-based catalysts with non-precious metal doping.
The doping strategy of non-precious metal elements shows apparent advantages in catalyzing the HER in seawater electrolysis. Firstly, compared with the doping of trace precious metals, the direct doping of inexpensive metals can further reduce the cost of catalyst preparation, which is conducive to the realization of large-scale industrial applications.123 Secondly, introducing transition metals, for example, can introduce additional active sites for nickel-based catalysts and modulate the surface and electronic structures of catalysts.124 Finally, the introduction of some metal elements can reduce the ΔGH* and also accelerate the desorption of OH*, which results in the rapid release of active sites, thus accelerating the kinetics of the HER.125 Lu et al. synthesized Mn–NiO–Ni/Ni–F by pyrolyzing manganese-based metal–organic frameworks (Mn-MOF) on nickel foam.126 The Mn-doped Mn–NiO–Ni/Ni–F demonstrated superior catalytic performance compared to the pristine NiO–Ni composite, as the presence of Mn decreased the ΔGH*, enhancing catalytic activity for HER (Fig. 12a). Additionally, the presence of Mn was found to enhance the catalytic stability of NiO–Ni composites. This improvement is attributed to the formation of NiMnOx on the catalyst surface, which protects the outer layer from oxidation. As a result, the catalytic activity remains stable for hundreds of hours during the HER. In the electrolysis of natural seawater test, the catalyst showed superior HER catalytic performance to Pt/C with lower onset overpotential. To investigate strategies for enhancing the HER activity and stability of nickel-based materials through elemental doping, Ysea et al. compared NiMo alloy catalysts, Ni(WO3), and Ni(Nb2O5) composite catalysts with pure nickel-based catalysts (Fig. 12e–h).128 They found that all doped catalysts, unlike the pure nickel-based ones, exhibited irregular surface areas, which increased the number of active sites and surface roughness, thereby improving catalytic activity. It was found that NiMo alloys have higher solubility under corrosive conditions, while nickel matrix composites exhibit better corrosion resistance. This is due to the higher proportion of (111) nickel planes compared to (200) planes, resulting in lower surface energy. The higher I(111)/I(200) ratio indicates better stability of the catalysts. Therefore, composites are superior to single Mo-doped catalysts, which is conducive to improving the stability of nickel-based materials in future hydrogen production from electrolytic seawater. Therefore, the use of composites is superior to single Mo-doped catalysts, which is conducive to improving the stability of nickel-based materials in future hydrogen production from electrolytic seawater. Furthermore, NiMo-based alloys have been found to bring the ΔGH* in the HER close to zero. Based on this, Zhu et al. synthesized heterogeneous Mo2N/Ni3Mo3N electrocatalysts (Fig. 12b).127 They achieved this by optimizing the ratio of NiMo-based content on a nickel foam substrate and calcining the precursor with the determined optimum molybdenum content under ammonia gas. The electrocatalyst can reach a current density of 500 mA cm−2 in seawater electrolyte at a low overpotential of 123 mV for HER and has a stability of more than 120 h (Fig. 12d). According to Raman analysis, the catalyst can adsorb H2O faster and dissociate OH− faster in the HER, and DFT disclosed that the heterogeneous interfacial structure can reduce the ΔGH* of the catalyst (Fig. 12c). Bu et al. utilized the property that NiMo-based alloys can reduce ΔGH* and prepared NiMo@C3N5 catalysts with a unique heterogeneous structure by using two-dimensional C3N5 as a shell.129 The carbon-based shell effectively protects the catalyst's inner core from seawater poisoning and optimizes the HER pathway. Density functional theory calculations revealed multiple electron transport channels at the interface between the NiMo inner core and the carbon-based shell. This “killing two birds with one stone” property lowers the reaction rate step's energy barrier and reduces the reaction's overpotential. Finally, NiMo@C3N5 Faraday efficiency in seawater was as high as 94.8%. These aforementioned reports suggest that the development of high-performance HER electrocatalysts by doping and interfacial engineering of non-precious metal elements is a feasible strategy.
 |
| Fig. 12 (a) The LSV curves of Mn–NiO–Ni/Ni–F, Pt/C/Ni–F and Ni–F in natural seawater. Reproduced with permission.126 Copyright 2018, Royal Society of Chemistry. (b) Schematic depiction of the synthetic pathways employed for the fabrication of MN-NMN-based catalysts. (c) A computed free energy diagram showcasing the energetics of H2O*, H–OH, and [H + OH]* on various catalysts, including Mo2N, Ni3Mo3N, Mo2N/MoOx, Mo2N–Ni3Mo3N, Ni3Mo3N/MoOx, Mo2N/NiOOH, and Ni3Mo3N/NiOOH. (d) Stability tests of MN-NMN09/NF were conducted using chronoamperometry, maintaining constant current densities of 500 mA cm−2 for 120 hours in an alkaline seawater electrolyte. Reproduced with permission.127 Copyright 2023, Wiley-VCH. Scanning electron micrographics of the (e) Ni-Watts. (f) NiMo alloy. (g) Ni(Nb2O5) composite. (h) Ni(WO3) composite. Reproduced with permission.128 Copyright 2022, Elsevier. | |
Non-precious metal-doped nickel-based electrocatalysts can be used in various methods to enhance the efficiency of HER in diluted seawater. Key efforts include optimizing the structural design of catalysts and managing lattice defects to boost active site density and enhance electron transport rate. Furthermore, the catalytic activity of HER can be improved through surface modification and the inclusion of appropriate heteroatoms or chemicals. When conducting HER in seawater, it is important to evaluate the stability of the catalyst and its capacity to resist contaminants in saltwater to guarantee the catalyst maintains efficient and durable performance in challenging conditions.
3.2.3 Nickel-based phosphide.
In addition to enhancing the intrinsic activity of the OER, nickel-based phosphides also play a crucial role in boosting the intrinsic activity of the reaction during the synthesis of HER catalysts.130 Nickel-based phosphides increase the catalysts' activity and stability and offer abundant active sites for reactant adsorption and electron transfer, as illustrated in Fig. 13a and b.131 Moreover, their excellent chemical stability and corrosion resistance enable them to maintain high activity and longevity throughout extended reaction processes. Furthermore, the rich tunability of nickel-based phosphides allows for precise adjustment of catalyst activity and selectivity by modulating synthesis methods, composition, and structure.70 This tunability enhances the flexibility and options available for catalyst design and optimization.
 |
| Fig. 13 (a) XRD patterns of NFP@NC-300, NFP@NC, and NFP@NC-400 were analyzed. (b) Schematic illustration of the synthesis process of the NFP@NC. Reproduced with permission.131 Copyright 2023, Springer Nature. (c) Schematic illustration of the synthesis of core–shell-structured NiS@LDH/NF. Reproduced with permission.132 Copyright 2023, Wiley-VCH. (d) XPS spectra of Ni 2p were obtained for B,V-Ni2P, V-Ni2P, and Ni2P. Reproduced with permission.133 Copyright 2023, Wiley-VCH. (e) Electrochemical reconstruction of Fe0.01–Ni&Ni0.2Mo0.8N was observed by cyclic voltammetry (CV) scans. (f) A schematic representation of the electrochemical reconstruction process of Fe0.01–Ni&Ni0.2Mo0.8N, transforming into Fe0.01&Mo–NiO, is depicted. Reproduced with permission.48 Copyright 2022, Royal Society of Chemistry. | |
Nickel phosphide has garnered attention among recent HER electrocatalysts for its high electrical conductivity, activity, and tunable electronic configuration. The phosphate layer formed by surface oxidation in seawater repels Cl− ions and mitigates catalyst corrosion effectively.134 Zhao et al. synthesized a B,V-Ni2P electrode by co-doping boron and vanadium phosphide. Vanadium doping enhances the dissociation of water by nickel phosphide, while boron and vanadium co-doping adjusts the electronic configuration of nickel phosphide, promoting interaction with intermediate products and enhancing HER activity.133 In addition, the doping of V also favours the induction of Ni lattice distortion, and according to XPS, the Ni2P binding energy is negatively shifted (Fig. 13d), indicating that its electrons undergo rearrangement. Ultimately, the B,V-Ni2P electrocatalysts only require an overpotential of 162 mV to reach a current density of 100 mA cm−2 in the simulated seawater of 1 M KOH and 0.5 M NaCl, and the nanomorphology of the surfaces has also remained essentially. The nanomorphology of its surface was also basically unchanged in the long-term test, which has good stability.
3.2.4 Nickel-based sulfides.
Similar to nickel phosphides, nickel sulfides also exhibit high chemical stability in seawater electrolysis environments, particularly in chloride-containing seawater where they demonstrate superior corrosion resistance.135 Furthermore, nickel sulfides typically present lower overpotentials in HER, thereby enhancing the efficiency of the HER process.119 This combination of high chemical stability and low overpotential makes nickel sulfides highly effective catalysts for HER in seawater electrolysis.
Ren et al. utilized nickel sulfide (Ni3S2) on nickel foam to design the heterostructure NiS@LDH/NF with OER activity.132 They then phosphorylated it to obtain NiS@FeNiP/NF, which exhibited excellent HER activity (Fig. 13c). During the synthesis, electron transfer from Ni3S2 to the FeNi2P interface facilitated charge redistribution, improving interaction with reaction intermediates. Consequently, the HER activity in alkaline seawater electrolyte was significantly enhanced compared to NiS@LDH/NF. Through a combination of theoretical calculations and experiments, researchers found that NiS@FeNiP/NF, with its superhydrophobic/superhydrophilic surface properties, exhibits excellent H2O adsorption capacity and promotes hydrogen release during the HER. This enables the catalyst to reach 500 mA cm−2 in an alkaline seawater electrolyte, with an overpotential of 237 mV. Fan et al. developed a heterogeneous and corrosion-resistant iron–sulfur-based catalytic electrode, NiS–FeS@IF, using a simplified one-step sulfuration-etching method.135 This catalyst demonstrated a low overpotential of just 322 mV for the HER at a current density of 500 mA cm−2. More importantly, the NiS–FeS@IF electrode sustained industrial-grade high current densities of 1 A cm−2 for over 500 h without significant performance degradation. The distinct and evenly distributed structure of NiS–FeS improves interfacial electron transfer, maximizes the exposure of active sites, and offers efficient routes for quick bubble release and mass transfer. Additionally, this straightforward one-step sulfuration method, distinct from traditional hydrothermal methods, shows promise for large-scale industrial production.
3.2.5 Nickel-based nitrides.
Nickel nitrides, while having lower intrinsic catalytic activity and fewer active sites compared to nickel phosphides and sulfides, offer significant advantages as electrocatalysts for HER. They exhibit excellent mechanical strength and chemical stability, maintaining structural integrity during prolonged operation. Their good corrosion resistance in seawater electrolysis environments enables them to withstand chloride ion corrosion effectively.136 Additionally, nickel nitrides are cost-effective and possess good electrical conductivity, enhancing electron transfer efficiency. Their multifunctionality allows for performance optimization through element doping, adjusting their electronic structure and catalytic properties.138,139 Despite their lower intrinsic activity, these strengths make nickel nitrides promising candidates for HER electrocatalysis.
Based on the property that NiMo-based alloys can reduce ΔGH*, Ning et al. successfully synthesized Fe-doped NiMo-based alloy nitrides Fex–Ni&Ni0.2Mo0.8N on nickel foam.48 These catalysts exhibited excellent HER activity, achieving efficiencies comparable to the highest among reported non-precious metal catalysts. Meanwhile, they found that varying the doping concentration of Fe element can change the nanorod size in the catalyst on the one hand. On the other hand, it will significantly affect its intrinsic HER activity. It is found that Cdl decreases with the increase of Fe doping, which indicates that the increase of Fe doping leads to the decrease of ECSA. In contrast, a small amount of Fe doping does not change the intrinsic activity of the catalyst, and also its electrochemically reconstructed product Fe0.01&Mo–NiO exhibits superior OER performance (Fig. 13e and f). Jin et al. developed a catalyst featuring abundant unsaturated Ni–N bonds, designated as Ni-SN@C, for the HER in alkaline seawater.136 This catalyst exhibited both high activity and stability, achieving a remarkably low overpotential of 23 mV at a current density of 10 mA cm−2, outperforming commercial Pt/C. Moreover, Ni-SN@C demonstrated the ability to generate hydronium ions in high-pH electrolytes, a characteristic similar to that of precious metal catalyst Pt. This study underscores the significant potential of surface modification engineering of transition metals for industrial hydrogen production (Table 2).
Table 2 Performance of recently reported nickel-based HER electrocatalysts
Catalysts |
Electrolyte |
Over potential for specific current density |
Stability |
Ref. |
MIL-(IrNiFe)/NF |
1.0 M KOH + seawater |
235 mV@1000 mA cm−2 |
100 h@100 mA cm−2 |
120
|
Pt–Ni@NiMoN/NF |
1.0 M KOH + seawater |
11 mV@10 mA cm−2 |
110 h@400 mA cm−2 |
121
|
Pt-hcp Ni NBs |
1.0 M KOH + seawater |
137 mV@10 mA cm−2 |
130 h@500 mA cm−2 |
122
|
MN-NMN/NF |
1.0 M KOH + seawater |
123 mV@500 mA cm−2 |
120 h@500 mA cm−2 |
126
|
NiMo@C3N5 |
pH = 7.4 seawater |
486 mV@10 mA cm−2 |
10 h@10 mA cm−2 |
129
|
NiS@FeNiP/NF |
1.0 M KOH + seawater |
327 mV@1000 mA cm−2 |
120 h@500 mA cm−2 |
132
|
Fex–Ni&Ni0.2Mo0.8N/NF |
1.0 M KOH + seawater |
69 mV@100 mA cm−2 |
80 h@425 mA cm−2 |
48
|
B,V-Ni2P |
1.0 M KOH +0.5 M NaCl |
162 mV@100 mA cm−2 |
100 h@500 mA cm−2 |
133
|
NiS–FeS@IF |
1.0 M KOH +0.5 M NaCl |
322 mV@500 mA cm−2 |
500 h@1 A cm−2 |
136
|
Ni-SN@C |
1.0 M KOH + seawater |
23 mV@10 mA cm−2 |
24 h@10 mA cm−2 |
137
|
4. Anti-corrosion strategies for nickel-based materials
In contrast to freshwater electrolysis, seawater electrolysis faces electrode corrosion due to the corrosive nature of chloride ions present in seawater. Researchers have explored various strategies to enhance the stability and prolong the service life of nickel-based materials in this challenging environment.35,140 Apart from leveraging the inherent corrosion resistance of nickel-based materials, efforts have been made to target the development of high-activity catalysts for seawater electrolysis at low overpotential conditions, focusing on improving OER selectivity and reducing the competitive CER.141 Recent studies have concentrated on enhancing the corrosion resistance of nickel-based electrocatalyst materials through doping with corrosion-resistant ions, utilizing electrostatic repulsion, and incorporating protective layers.142,143
4.1 Electrostatic repulsion strategy
Construction of polyanionic systems on catalyst surfaces or intercalations, shielding the corrosive effect of chloride ions by electrostatic repulsion and thus increasing the stability of the catalyst is a common corrosion resistance strategy (Fig. 14a).144,147 Zhang et al. investigated the impediment to Cl− migration using different ionic intercalations between NiFe-LDH intercalations through DFT calculations (Fig. 14c).146 The results showed that the PO43− intercalation had the strongest rejection of chloride ions, where the migration energy barrier of chloride ions was 2.91 eV (Fig. 14d), which was higher than that of its control CO32− (2.57 eV) and OH− intercalation (0.86 eV). As a result, a strategy to intercalate PO43− groups in NiFe-LDH is proposed, where PO43− carrying a large negative charge can facilitate an electrostatic repulsion strategy to prevent chloride ions from passing through the NiFe-LDH intercalation, preventing the nickel matrix from being corroded (Fig. 14e–g). A series of electrochemical tests revealed that the corrosion resistance life of PO43− inserted NiFe-LDH was extended by more than one hundred times compared to pristine NiFe-LDH. Based on the same principle, NF/Ni3N@NiFe-PA constructed by Li et al. also has good corrosion resistance. The researchers quantified the concentration of Cl− by ion chromatography, and NF/Ni3N@NiFe-PA only adsorbed 0.11 mm Cl−, which is lower than the original NF/Ni3N (0.26 mm Cl−) (Fig. 14b).145 The phosphate-rich surface of the catalyst prevents chloride ion corrosion through electrostatic repulsion. Additionally, the multilayer structure of corrosion-resistant Ni3N and in situ-generated NiFeOOH enhances corrosion inhibition. Besides phosphate groups, sulfur-containing, carbon-containing, and other anionic groups are also used to improve corrosion resistance based on electrostatic repulsion principle.99,148 Kang et al. reported a RuMoNi electrocatalyst with in situ-grown MoSO42− that preferentially adsorbs at the anode and repels chloride ions during electrolysis, which can be operated continuously for 3000 h at a current density of 500 mA cm−2 and is 100% selective for OER in a 1 M KOH + simulated seawater electrolyte.38
 |
| Fig. 14 (a) A visual representation is provided to demonstrate the implementation of double-polyamide thin-film composite (TFC) membranes in seawater electrolysis. Reproduced with permission.144 Copyright 2024, American Chemical Society. (b) The electrocatalytic performance for the OER of different samples, including NF, NF/Ni(OH)2, NF/Ni3N, NF/Ni3N@PA, NF/Ni3N@NiFe-PA, NF/PA, and NF/NiFe-PA, has been evaluated in alkaline simulated seawater (1 M KOH + 0.5 M NaCl). A summary of the overpotentials required for achieving current densities of 50, 100, and 200 mA cm−2 for each sample is provided. Reproduced with permission.145 Copyright 2023, Wiley-VCH. (c) The structure of NiFe-LDH supported on Ni foam can be visually depicted through a schematic illustration. (d) Energetic barriers to Cl− migration in PO43−/NiFe-LDH. (e) Schematic representation of PO43− against Cl− corrosion in PO43−/NiFe-LDH environment. (f) Schematic representation of Cl− crossing NiFe-LDH without any ionic intercalation. (g) Energy barrier for Cl− migration in NiFe-LDH without ionic intercalation. Reproduced with permission.146 Copyright 2022, Wiley-VCH. | |
4.2 Adding corrosion inhibitors to the electrolyte
The addition of corrosion inhibitors to the electrolyte can serve two main purposes: promoting the exclusion of chloride ions by the polyanion in the electrolyte or facilitating a reaction between a specific component in the electrolyte and the catalyst surface.149 This reaction can lead to forming a passivation layer on the catalyst surface, thereby enhancing its corrosion resistance. Ma et al. exploited this strategy by adding sulphate to the electrolyte to improve catalyst corrosion resistance, and the catalysts were stable for 3–5 times longer than when reacting in a seawater electrolyte without SO42− (Fig. 15a–c).150 Recently, Hiroki et al.152 added a borate/phosphate hybrid additive to an alkaline seawater electrolyte, where borate is used as a buffering reagent to maintain the stability of the electrolyte pH. In contrast, phosphate can form a passivation layer with Ni on the catalyst at a pH of 9.2, preventing the catalyst from dissolving due to corrosion (Fig. 15e and f). Researchers used redox probes and Operando XAS analysis to investigate the catalyst's corrosion protection mechanism at non-extreme pH levels. They ruled out electrostatic repulsion as a factor in the enhanced corrosion resistance, demonstrating that increased stability is due to surface complex formation on the Ni group and passivation. Tang et al. found that a decrease in pH around the anode during the electrolysis of seawater accelerated the corrosion of the electrode material by chloride ions, and that this corrosion behaviour increased rapidly with increasing current density.151 The researchers classified the results of electrochemical testing of the materials at different pH and current densities and found that the electrode materials can undergo a stable oxygen precipitation reaction in the blue, low-current-density, high-pH region, while the electrodes dissolve violently in the red, high-current-density, low-pH region (Fig. 15d). This finding draws attention to the fact that, in addition to a corrosion-resistant strategy for the catalyst, the construction of a long-lasting electrolyte/electrolyte interfacial microenvironment is an important factor in determining the stability of electrolytic seawater.153
 |
| Fig. 15 (a) Visual representations of electrolyte systems above the electrode surface obtained from classical molecular dynamics simulations. (b) Comparison of the electrochemical performance for OER on NiFe-LDH nanoarrays supported on NF in electrolytes with and without SO42−, presented as LSV curves. (c) In different electrolytes, the LSV curves of NiFe-LDH/NF with iR compensation for overall water splitting are obtained. Reproduced with permission.150 Copyright 2021, Wiley-VCH. (d) The figure is a schematic representation of the degradation behaviour of the model electrode at different local pH values. Reproduced with permission.151 Copyright 2024, American Chemical Society. (e) CV curves of NiFeOx in K-borate and K-borate/phosphate electrolytes in KCl. (f) CV curves of NiFeOx in K-borate and K-borate/phosphate electrolytes in KBr.152 Copyright 2024, Royal Society of Chemistry. | |
4.3 Doped corrosion-resistant ions
Doping the catalyst with corrosion-resistant ions is another effective method to extend its service life. Incorporating metal ions with good corrosion resistance into the catalyst's structure enhanced its ability to resist corrosion by chloride ions.154 These corrosion-resistant metal ions not only improve the surface redox activity of the catalyst but also help reduce the corrosive impact of chlorides on the catalyst.155,156 Ou et al. uniformly doped Mo into Ni3S2 to obtain Mo–Ni3S2/NMF (Fig. 16c). Electrochemical measurements and DFT calculations revealed that Mo doping increased the binding energy with *OH and decreased the binding energy with *Cl on the catalyst surface, thereby enhancing corrosion resistance (Fig. 16a and b).157 Meanwhile, the in situ-grown SOxn− also improves the corrosion resistance of the catalyst through the principle of electrostatic repulsion, which can maintain the stability of 160 h at 1.53 V. Rare earth elements have good chemical stability and corrosion resistance, and the doping of appropriate amount of rare earth ions in the catalyst can improve its corrosion resistance. Recently, Yao et al. doped Ce on NiFe LDH, and Ce generated a protective layer of CeO2 to prevent chloride ions from penetrating the catalytic layer during the electrolysis of seawater.83 The electrocatalyst can work stably for 500 h at a current density of 1000 mA cm−2, a finding that points the way to exploring Ce-doped LDH. Multi-component catalysts, such as high-entropy alloys, offer enhanced corrosion resistance through the coordination of each metal component, compared to single mono or binary metal catalysts.159 Bian et al. used a one-step electrodeposition method to construct a high-entropy alloy multi-component catalyst NiFeCuCoCe HEA on a nickel foam substrate. Further, they enhanced the corrosion resistance of the material through the in situ growth of various hydroxyl oxides on the surface of the catalyst by modification (Fig. 16d–f).158 Characterizing the surface of the catalyst after the OER by XPS, the researchers found that there was no significant reconstruction of the surface of the samples used for a long period of time, demonstrating the good corrosion resistance of the catalyst. This is attributed to the incorporation of NiCuCe corrosion-resistant elements and the formation of a dense layered structure on the catalyst surface during modification, both of which work in tandem to prevent the corrosion of chloride ions.
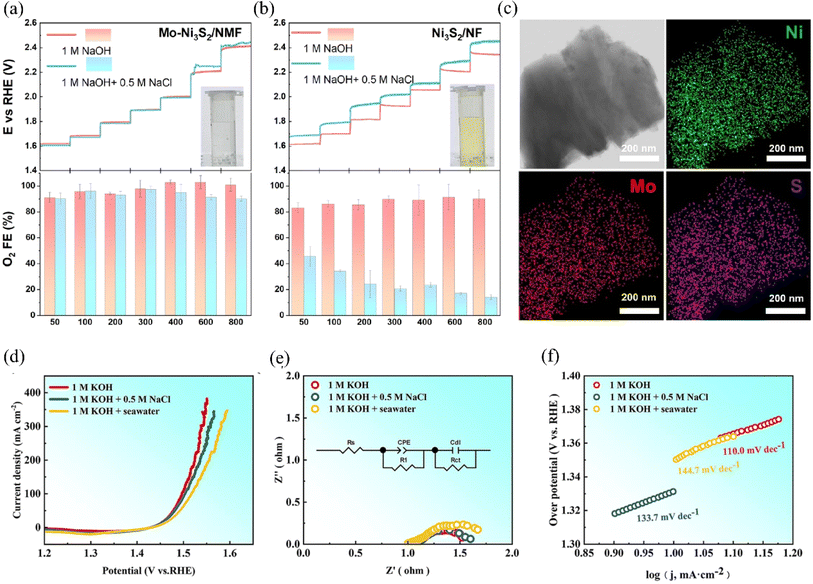 |
| Fig. 16 Anodic activity CP tests without iR compensation and corresponding O2 Faraday electrical efficiency measurements were performed on (a) Mo–Ni3S2/NMF and (b) Ni3S2/NF in N2-saturated 1.0 M NaOH electrolytes containing 0.5 M NaCl and no NaCl, respectively, at currents from 50 to 800 mA. The hold time for each step was set to 300 s. The inset in the figure shows a photograph of the electrolyte after iodide titration. (c) The HAADF-STEM image and elemental mapping of Mo–Ni3S2/NMF are shown. Reproduced with permission.157 Copyright 2023, Elsevier. OER performance of HEA-NCFCC/NF@EA in 1.0 M KOH, 1.0M KOH + 0.5 M NaCl and alkaline seawater (d) polarization curves; (e) electrochemical impedance spectroscopy; (f) Tafel plots;158 Copyright 2023, Elsevier. | |
5. Conclusions and outlook
Seawater, as an abundant renewable resource on the planet, provides a high-quality source of direct electrolysis for hydrogen production. This method enables the production of hydrogen and freedom from dependence on other fossil fuels, making it an essential means of contributing to the goal of carbon neutrality by 2060.160 The process of direct electrolysis of seawater for hydrogen production has the unique advantage of not only avoiding pollutants and greenhouse gases, but also serving as a sustainable source of hydrogen energy. However, the existence of expensive production costs of precious metal catalysts and the challenge of corrosion of catalysts by seawater make direct electrolysis of seawater unattainable for large-scale commercial applications. Facing this background, the research progress for modifying non-precious-metal nickel-based electrolyte materials and the corrosion-resistant strategy of nickel-based catalysts in seawater have attracted much attention in recent years.
This review provides an overview of the subject, summarising the latest research advances and strategies. The principle of seawater electrolysis, the basis for judging electrocatalyst activity, and the pathways reported in recent years to improve the catalytic activity and stability of OER and HER in seawater electrolysis with modified nickel-based electrolyte materials are explained. Meanwhile, effective corrosion resistance strategies in recent years are summarised to study catalyst corrosion in seawater environments. These research results point the way to the further development and commercial application of direct electrolysis of seawater for hydrogen production. By reducing catalyst costs, improving catalytic activity and stability, and designing corrosion-resistant nickel-based catalysts, we are expected to overcome the current technological limitations and promote the large-scale application of direct electrolysis of seawater for hydrogen production, which will make a significant contribution to the achievement of sustainable energy development and the goal of carbon neutrality.
Although nickel-based catalysts have achieved electrochemical activity comparable to commercial noble metal-based catalysts through modification, the current technology is still unable to meet the demand for large-scale commercial applications.161,162 The main reasons for this status quo are twofold. Firstly, these modified nickel-based catalysts are still in the laboratory stage, with low yields for single synthesis and time-consuming preparation processes, making it impossible to achieve batch production.163 Secondly, it is important to note that despite the improvements achieved by doping the catalyst with corrosion-resistant ions, there are still challenges in the stability of these modified catalysts during high-current, long-duration seawater-catalyzed electrolysis. Further research is required to address these issues effectively. In the future, efforts can be focused on enhancing the electrochemical performance of nickel-based catalysts to a level where they can be utilized commercially.164,165
5.1 Further exploration of corrosion resistance strategies
In the future, to further promote the commercialisation of electrolytic seawater, researchers should continue to gain a deeper understanding of the mechanism of electrolytic seawater as well as propose corrosion-resistant strategies. For example, corrosion-resistant materials and new synthesis pathways can be selected.35 Materials with good corrosion resistance are sought as growth substrates for electrocatalysts. These materials can include corrosion-resistant metals or alloys and carbon-based materials with excellent corrosion resistance.166 In addition, modifying and protecting the surface of the electrocatalyst can enhance its corrosion resistance. For example, suitable surface coatings, films or encapsulating materials are used to block corrosive substances in seawater from coming into direct contact with the catalyst. Surface modification can also improve the stability of the electrocatalyst by changing the surface chemistry or forming a protective oxide layer, for example. By optimizing the characteristics of the catalyst in terms of nanostructure as well as crystal morphology, it is also an excellent way to improve its corrosion resistance. For example, reasonable control of the lattice defects and pore structure of the catalyst can enhance its corrosion resistance and improve the catalytic activity. It is worth noting that regulating the environmental conditions of the catalytic reaction, such as controlling the pH value and temperature of the electrolyte, can optimize the corrosion resistance of the electrocatalyst. Appropriate electrolyte composition and concentration are reasonably selected to avoid the corrosion of the catalyst by excessive pH and ion concentration. Finally, advanced monitoring and analysis techniques, such as in situ spectroscopy and electrochemical testing, are used to track and evaluate the performance of the electrocatalyst in a corrosive environment in real-time. This will help to understand the corrosion mechanism and provide guidance for optimising the design and regulation of electrocatalysts.
5.2 Developing more efficient and scaleable synthesis methods
Optimization of the synthesis conditions can be carried out to find the best reaction conditions by precisely controlling the synthesis conditions such as reaction temperature, pressure, catalyst concentration, and the amount of additives used. Reasonable synthesis conditions can increase the reaction rate and yield, thus improving the preparation efficiency of nickel-based catalysts.167 At the same time, through the improvement and optimization of the synthesis route, the intermediate reaction steps and energy consumption can be reduced, and the reaction conversion and catalyst yield can be increased. Process simulation techniques and reaction engineering principles can be used to guide the optimal design of synthetic routes. Some existing novel synthetic methods, such as microfluidic synthesis, sol–gel method, hydrothermal synthesis, etc., can replace the traditional synthetic methods.163 Further study of these novel synthetic methods can provide higher reaction control and higher reaction rates, thus increasing the yield of nickel-based catalysts.
5.3 Improvement of catalyst stability
To improve the stability of the catalyst, we can adopt a series of strategies. Firstly, choose catalyst support materials reasonably, such as carbon materials with good chemical stability and conductivity, oxides, or stable high surface area materials. Second, enhance the compatibility of the catalyst with the electrolyte through surface modification and functionalisation to reduce the reaction with impurities in seawater and improve the stability of the catalyst.168 At the same time, the surface structure and composition of the catalyst are optimized to increase the number and activity of active sites, and active site engineering is carried out to improve catalytic activity and stability. In addition, the mass transfer performance and stability of the catalysts are enhanced by designing and optimizing the electrode structure of the catalysts, including morphology, pore structure, and pore size. In addition, the conductivity performance of the catalyst can be improved by doping or modulating the electronic structure of the catalyst.169 Finally, a suitable electrolyte formulation is selected, and stabilizers, buffers, or additives are added to optimise the electrolyte and improve the stability of the catalyst in seawater. The combined application of these strategies can effectively improve the stability of modified nickel-based catalysts to withstand high currents and prolonged catalytic electrolysis reactions in seawater environments and promote the commercial application of seawater direct electrolysis for hydrogen production technology. This will provide important support for the development of sustainable energy and the achievement of carbon neutrality.
Data availability
The data used to support the review are included within the article.
Conflicts of interest
There are no conflicts to declare.
Acknowledgements
Supports of the National Natural Science Foundation of China (21902021, 21908017), the Fundamental Research Funds for the Central Universities (DUT22LK09), the Open Foundation of Key Laboratory of Industrial Ecology and Environmental Engineering, MOE (KLIEEE-21-02), the Foundation of State Key Laboratory of High-efficiency Utilization of Coal and Green Chemical Engineering (2022-K70), and the Hefei Advanced Computing Center for this work are gratefully acknowledged.
References
- D. S. Kaufman and E. Broadman, Revisiting the Holocene global temperature conundrum, Nature, 2023, 614, 425–435 CrossRef CAS PubMed.
- T. R. Karl, A. Arguez, B. Y. Huang, J. H. Lawrimore, J. R. McMahon, M. J. Menne, T. C. Peterson, R. S. Vose and H. M. Zhang, Possible artifacts of data biases in the recent global surface warming hiatus, Science, 2015, 348, 1469–1472 CrossRef CAS PubMed.
- Z. Xie, B. Han, Y. Sun, B. L. Su, J. Yang, X. Bao and M. He, Green carbon science for carbon neutrality, Natl. Sci. Rev., 2023, 10, nwad225 CrossRef PubMed.
- T. Jiang, X. He, B. Su, P. H. Havea, K. Wei, Z. W. Kundzewicz and D. Liu, COP 28: Challenge of coping with climate crisis, Innovation, 2024, 5, 100559 Search PubMed.
- K. Sanderson, COP28 climate summit signals the end of fossil fuels — but is it enough?, Nature, 2023, 624(7992), 484–485 CrossRef CAS PubMed.
- H. The, Lancet Planetary, COP28 reflections, Lancet, 2024, 8, e1 Search PubMed.
- R. Li, Y. Li, P. Yang, P. Ren, D. Wang, X. Lu, R. Xu, Y. Li, J. Xue, J. Zhang, M. An, J. Ma, B. Wang, H. Liu and S. Dou, Synergistic interface engineering and structural optimization of non-noble metal telluride-nitride electrocatalysts for sustainably overall seawater electrolysis, Appl. Catal., B, 2022, 318, 121834 CrossRef CAS.
- Y. Li, H. Xu, P. Yang, R. Li, D. Wang, P. Ren, S. Ji, X. Lu, F. Meng, J. Zhang and M. An, Interfacial engineering induced highly efficient CoNiP@NiFe layered double hydroxides bifunctional electrocatalyst for water splitting, Mater. Today Energy, 2022, 25, 100975 CrossRef CAS.
- R. Li, Y. Li, P. Yang, P. Ren, D. Wang, X. Lu, H. Zhang, Z. Zhang, P. Yan, J. Zhang, M. An, B. Wang, H. Liu and S. Dou, Key Roles of Interfacial OH- ion Distribution on Proton Coupled Electron Transfer Kinetics Toward Urea Oxidation Reaction, Small, 2023, 19, e2302151 CrossRef PubMed.
- R. Li, P. Ren, P. Yang, Y. Li, D. Wang, X. Lu, H. Zhang, F. Meng, X. Peng, B. Yuan, B. Wang, J. Zhang, M. An and G. Wu, VOx-doped CoP catalysts with synergistic dual-active configuration for boosting hydrogen evolution kinetics, Nano Energy, 2024, 126, 109613 CrossRef CAS.
- P. Nikolaidis and A. Poullikkas, A comparative overview of hydrogen production processes, Renewable Sustainable Energy Rev., 2017, 67, 597–611 CrossRef CAS.
- A. I. Osman, N. Mehta, A. M. Elgarahy, M. Hefny, A. Al-Hinai, A. H. Al-Muhtaseb and D. W. Rooney, Hydrogen production, storage, utilisation and environmental impacts: a review, Environ. Chem. Lett., 2022, 20, 153–188 CrossRef CAS.
- H. Yang, M. Driess and P. W. Menezes, Self-Supported Electrocatalysts for Practical Water Electrolysis, Adv. Energy Mater., 2021, 11, 2102074 CrossRef CAS.
- W. He, X. Li, C. Tang, S. Zhou, X. Lu, W. Li, X. Li, X. Zeng, P. Dong, Y. Zhang and Q. Zhang, Materials Design and System Innovation for Direct and Indirect Seawater Electrolysis, ACS Nano, 2023, 17, 22227–22239 CrossRef CAS PubMed.
- Q. Fu, J. Han, X. Wang, P. Xu, T. Yao, J. Zhong, W. Zhong, S. Liu, T. Gao, Z. Zhang, L. Xu and B. Song, 2D Transition Metal Dichalcogenides: Design, Modulation, and Challenges in Electrocatalysis, Adv. Mater., 2021, 33, e1907818 CrossRef PubMed.
- Y. Zheng, Y. Jiao, Y. Zhu, L. H. Li, Y. Han, Y. Chen, M. Jaroniec and S. Z. Qiao, High Electrocatalytic Hydrogen Evolution Activity of an Anomalous Ruthenium Catalyst, J. Am. Chem. Soc., 2016, 138, 16174–16181 CrossRef CAS PubMed.
- C. Wang, Q. Zhang, B. Yan, B. You, J. Zheng, L. Feng, C. Zhang, S. Jiang, W. Chen and S. He, Facet Engineering of Advanced Electrocatalysts Toward Hydrogen/Oxygen Evolution Reactions, Nano-Micro Lett., 2023, 15, 52 CrossRef CAS PubMed.
- Y. Wang, M. Wang, Y. Yang, D. Kong, C. Meng, D. Zhang, H. Hu and M. Wu, Potential technology for seawater electrolysis: Anion-exchange membrane water electrolysis, Chem Catal., 2023, 3, 100643 CrossRef CAS.
- Z. Y. Yu, Y. Duan, X. Y. Feng, X. Yu, M. R. Gao and S. H. Yu, Clean and Affordable Hydrogen Fuel from Alkaline Water Splitting: Past, Recent Progress, and Future Prospects, Adv. Mater., 2021, 33, e2007100 CrossRef PubMed.
- A. Badreldin, A. El Ghenymy, A.-R. Al-Zubi, A. Ashour, N. Hassan, A. Prakash, M. Kozusznik, D. V. Esposito, S. U. I. Solim and A. Abdel-Wahab, Stepwise strategies for overcoming limitations of membraneless electrolysis for direct seawater electrolysis, J. Power Sources, 2024, 593, 233991 CrossRef CAS.
- Y. Dong and S. Komarneni, Strategies to Develop Earth-Abundant Heterogeneous Oxygen Evolution Reaction Catalysts for pH-Neutral or pH-Near-Neutral Electrolytes, Small Methods, 2021, 5, e2000719 CrossRef PubMed.
- M. Maril, J. L. Delplancke, N. Cisternas, P. Tobosque, Y. Maril and C. Carrasco, Critical aspects in the development of anodes for use in seawater electrolysis, Int. J. Hydrogen Energy, 2022, 47, 3532–3549 CrossRef CAS.
- Z. Xiao, J. Wang, H. Lu, Y. Qian, Q. Zhang, A. Tang and H. Yang, Hierarchical Co/MoNi heterostructure grown on monocrystalline CoNiMoOx nanorods with robust bifunctionality for hydrazine oxidation-assisted energy-saving hydrogen evolution, J. Mater. Chem. A, 2023, 11, 15749–15759 RSC.
- F. Lu, M. Zhou, Y. Zhou and X. Zeng, First-Row Transition Metal Based Catalysts for the Oxygen Evolution Reaction under Alkaline Conditions: Basic Principles and Recent Advances, Small, 2017, 13, 1701931 CrossRef PubMed.
- N. T. Suen, S. F. Hung, Q. Quan, N. Zhang, Y. J. Xu and H. M. Chen, Electrocatalysis for the oxygen evolution reaction: recent development and future perspectives, Chem. Soc. Rev., 2017, 46, 337–365 RSC.
- F. Dionigi, T. Reier, Z. Pawolek, M. Gliech and P. Strasser, Design Criteria, Operating Conditions, and Nickel-Iron Hydroxide Catalyst Materials for Selective Seawater Electrolysis, ChemSusChem, 2016, 9, 962–972 CrossRef CAS PubMed.
- X. Ren, X. Dong, Z. Wu, J. Cao, X. Yang, J. Hao, L. Liu, G. Wu and A. Liu, Theoretical study of Au–NX–C catalysts for H2O2 electrosynthesis via two-electron oxygen reduction reaction, Catal. Sci. Technol., 2024, 14, 2226–2234 RSC.
- X. Ren, X. Dong, L. Liu, J. Hao, H. Zhu, A. Liu and G. Wu, Research
progress of electrocatalysts for the preparation of H2O2 by electrocatalytic oxygen reduction reaction, SusMat, 2023, 3, 442–470 CrossRef CAS.
- H. Zhu, X. Ren, X. Yang, X. Liang, A. Liu and G. Wu, Fe-based catalysts for nitrogen reduction toward ammonia electrosynthesis under ambient conditions, SusMat, 2022, 2, 214–242 CrossRef CAS.
- A. Liu, X. Liang, X. Ren, W. Guan, M. Gao, Y. Yang, Q. Yang, L. Gao, Y. Li and T. Ma, Recent Progress in MXene-Based Materials: Potential High-Performance Electrocatalysts, Adv. Funct. Mater., 2020, 30, 2003437 CrossRef CAS.
- J. Guo, Y. Zheng, Z. Hu, C. Zheng, J. Mao, K. Du, M. Jaroniec, S.-Z. Qiao and T. Ling, Direct seawater electrolysis by adjusting the local reaction environment of a catalyst, Nat. Energy, 2023, 8(3), 264–272 CAS.
- Y. Zheng, W. Geng, S. Xiao, T. Ma, C. Cheng, Y. Liao, Z. Zeng, S. Li and C. Zhao, Interfacial Ir-V Direct Metal Bonding Enhanced Hydrogen Evolution Activity in Vanadium Oxides Supported Catalysts, Angew Chem. Int. Ed. Engl., 2024, e202406427 Search PubMed.
- D. Zhang, Y. Shi, J. Yin and J. Lai, Recent Advances for Seawater Hydrogen Evolution, ChemCatChem, 2024, e202301305 CrossRef CAS.
- F. Dingenen and S. W. Verbruggen, Tapping hydrogen fuel from the ocean: A review on photocatalytic, photoelectrochemical and electrolytic splitting of seawater, Renewable Sustainable Energy Rev., 2021, 142, 110866 CrossRef CAS.
- J. Dong, C. Yu, H. Wang, L. Chen, H. Huang, Y. Han, Q. Wei and J. Qiu, A robust & weak-nucleophilicity electrocatalyst with an inert response for chlorine ion oxidation in large-current seawater electrolysis, J. Energy Chem., 2024, 90, 486–495 CrossRef CAS.
- S. Zhang, Y. Wang, S. Li, Z. Wang, H. Chen, L. Yi, X. Chen, Q. Yang, W. Xu, A. Wang and Z. Lu, Concerning the stability of seawater electrolysis: a corrosion mechanism study of halide on Ni-based anode, Nat. Commun., 2023, 14, 4822 CrossRef CAS PubMed.
- H. M. Zhang, L. H. Zuo, J. K. Li, S. F. Zhang, J. X. Guo, X. P. Li, G. Liu, P. Wang and J. F. Sun, Research and strategies for efficient electrocatalysts towards anodic oxygen evolution reaction in seawater electrolysis system, J. Mater. Sci. Technol., 2024, 187, 123–140 CrossRef.
- X. Kang, F. Yang, Z. Zhang, H. Liu, S. Ge, S. Hu, S. Li, Y. Luo, Q. Yu, Z. Liu, Q. Wang, W. Ren, C. Sun, H. M. Cheng and B. Liu, A corrosion-resistant RuMoNi catalyst for efficient and long-lasting seawater oxidation and anion exchange membrane electrolyzer, Nat. Commun., 2023, 14, 3607 CrossRef CAS PubMed.
- L. Li, B. Wang, G. W. Zhang, G. Yang, T. Yang, S. Yang and S. C. Yang, Electrochemically Modifying the Electronic Structure of IrO Nanoparticles for Overall Electrochemical Water Splitting with Extensive Adaptability, Adv. Energy Mater., 2020, 10, 2001600 CrossRef CAS.
- F. H. Zhang, L. Yu, L. B. Wu, D. Luo and Z. F. Ren, Rational design of oxygen evolution reaction catalysts for seawater electrolysis, Trends Chem., 2021, 3, 485–498 CrossRef CAS.
- Z. Zhao, J. P. Sun and X. C. Meng, Recent advances in transition metal-based electrocatalysts for seawater electrolysis, Int. J. Energy Res., 2022, 46, 17952–17975 CrossRef CAS.
- M. Abedi, S. Rezaee and S. Shahrokhian, Designing core-shell heterostructure arrays based on snowflake NiCoFe-LTH shelled over W2N-WC nanowires as an advanced bi-functional electrocatalyst for boosting alkaline water/seawater electrolysis, J. Colloid Interface Sci., 2024, 666, 307–321 CrossRef CAS PubMed.
- S. J. Liu, S. J. Ren, R. T. Gao, X. H. Liu and L. Wang, Atomically embedded Ag on transition metal hydroxides triggers the lattice oxygen towards sustained seawater electrolysis, Nano Energy, 2022, 98, 107212 CrossRef CAS.
- B. L. Jiang, J. Y. Li, Y. Y. Cui, S. J. Shi, N. Jiang and J. Guan, Controlled growth of highly active NiMoN(100)-decorated porous N- doped carbon nanotubes on carbon cloth as efficient electrodes for alkaline media and seawater electrolysis, J. Alloys Compd., 2023, 958, 170371 CrossRef CAS.
- R. Yang, L. Mei, Z. Lin, Y. Fan, J. Lim, J. Guo, Y. Liu, H. S. Shin, D. Voiry, Q. Lu, J. Li and Z. Zeng, Intercalation in 2D materials and in situ studies, Nat. Rev. Chem, 2024, 8, 410–432 CrossRef CAS PubMed.
- L. M. Hong, B. Li, C. D. Jing, Z. H. Zhuang, Y. J. Zhang, H. B. Huang, Q. Q. Jiang and J. G. Tang, Recent advances on cationic vacancy engineering application of oxygen evolution reaction electrocatalyst in seawater, J. Environ. Chem. Eng., 2024, 12, 111946 CrossRef CAS.
- Y. Chen, X. Q. Jiang, Y. D. Li, J. Zeng and H. P. Liang, Construction of S-modified Amorphous Fe(OH)3 on NiSe Nanowires as Bifunctional Electrocatalysts for Efficient Seawater Splitting, ACS Appl. Nano Mater., 2024, 7, 3960–3967 CrossRef.
- W. Liu, W. Liu, T. Hou, J. Ding, Z. Wang, R. Yin, X. San, L. Feng, J. Luo and X. Liu, Coupling Co-Ni phosphides for energy-saving alkaline seawater splitting, Nano Res., 2024, 17, 4797–4806 CrossRef CAS.
- S. Gopalakrishnan, V. Saranya, G. Anandha babu, S. Harish, E. Senthil Kumar and M. Navaneethan, Heterogeneous bimetallic oxysulfide nanostructure (Ni-Co) as hybrid bifunctional electrocatalyst for sustainable overall alkaline simulated seawater splitting, J. Alloys Compd., 2023, 965, 171124 CrossRef CAS.
- G. Wang, T. Xiang, X. Ren, L. Zhang and C. Chen, Transition metal-based electrocatalysts for hydrogen production from seawater: A review, Int. J. Hydrogen Energy, 2024, 73, 775–790 CrossRef CAS.
- F. Zhang, L. Yu, L. Wu, D. Luo and Z. Ren, Rational design of oxygen evolution reaction catalysts for seawater electrolysis, Trends Chem., 2021, 3, 485–498 CrossRef CAS.
- H. Saada, B. Fabre, G. Loget and G. Benoit, Is Direct Seawater Splitting Realistic with Conventional Electrolyzer Technologies?, ACS Energy Lett., 2024, 3351–3368 CrossRef CAS.
- H. Jin, J. Xu, H. Liu, H. Shen, H. Yu, M. Jaroniec, Y. Zheng and S. Z. Qiao, Emerging materials and technologies for electrocatalytic seawater splitting, Sci. Adv., 2023, 9, eadi7755 CrossRef CAS PubMed.
- H. Sun, Z. Yan, F. Liu, W. Xu, F. Cheng and J. Chen, Self-Supported Transition-Metal-Based Electrocatalysts for Hydrogen and Oxygen Evolution, Adv. Mater., 2020, 32, e1806326 CrossRef PubMed.
- C. Hu, L. Zhang and J. Gong, Recent progress made in the mechanism comprehension and design of electrocatalysts for alkaline water splitting, Energy Environ. Sci., 2019, 12, 2620–2645 RSC.
- C. L. Hu, L. Zhang and J. L. Gong, Recent progress made in the mechanism comprehension and design of electrocatalysts for alkaline water splitting, Energy Environ. Sci., 2019, 12, 2620–2645 RSC.
- Y. Zheng, Y. Jiao, A. Vasileff and S. Z. Qiao, The Hydrogen Evolution Reaction in Alkaline Solution: From Theory, Single Crystal Models, to Practical Electrocatalysts, Angew Chem. Int. Ed. Engl., 2018, 57, 7568–7579 CrossRef CAS PubMed.
- D. C. Chen, Z. W. Chen, X. X. Zhang, Z. L. Lu, S. Xiao, B. B. Xiao and C. V. Singh, Exploring single atom catalysts of transition-metal doped phosphorus carbide monolayer for HER: A first-principles study, J. Energy Chem., 2021, 52, 155–162 CrossRef CAS.
- A. Parra-Puerto, K. L. Ng, K. Fahy, A. E. Goode, M. P. Ryan and A. Kucernak, Supported Transition Metal Phosphides: Activity Survey for HER, ORR, OER, and Corrosion Resistance in Acid and Alkaline Electrolytes, ACS Catal., 2019, 9, 11515–11529 CrossRef CAS.
- W. X. Meng, J. Wang, D. Zhang, J. Xu, F. M. Guo, Y. J. Zhang, R. Pang, A. Y. Cao and Y. Y. Shang, Defective Amorphous Carbon-Coated Carbon Nanotube-Loaded Ruthenium Nanoparticles as Efficient Electrocatalysts for Hydrogen Production, Small Struct., 2023, 4, 2300098 CrossRef CAS.
- J. Song, C. Wei, Z. F. Huang, C. Liu, L. Zeng, X. Wang and Z. J. Xu, A review on fundamentals for designing oxygen evolution electrocatalysts, Chem. Soc. Rev., 2020, 49, 2196–2214 RSC.
- J. S. Yoo, X. Rong, Y. Liu and A. M. Kolpak, Role of Lattice Oxygen Participation in Understanding Trends in the Oxygen Evolution Reaction on Perovskites, ACS Catal., 2018, 8, 4628–4636 CrossRef CAS.
- H. Liu, W. Shen, H. Jin, J. Xu, P. Xi, J. Dong, Y. Zheng and S. Z. Qiao, High-Performance Alkaline Seawater Electrolysis with Anomalous Chloride Promoted Oxygen Evolution Reaction, Angew Chem. Int. Ed. Engl., 2023, 62, e202311674 CrossRef CAS PubMed.
- S. Y. Feng, P. Rao, Y. H. Yu, J. Li, P. L. Deng, Z. Y. Kang, S. L. Wang, Z. P. Miao, Y. J. Shen, X. L. Tian and Z. F. Wu, Self-assembled heterojunction CoSe2@CoO catalysts for efficient seawater electrolysis, Electrochim. Acta, 2023, 463, 142870 CrossRef CAS.
- Y. Shi and B. Zhang, Correction: Recent advances in transition metal phosphide nanomaterials: synthesis and applications in hydrogen evolution reaction, Chem. Soc. Rev., 2016, 45, 1781 RSC.
- P. A. Kempler and A. C. Nielander, Reliable reporting of Faradaic efficiencies for electrocatalysis research, Nat. Commun., 2023, 14, 1158 CrossRef CAS PubMed.
- T. Cui, X. J. Zhai, L. L. Guo, J. Q. Chi, Y. Zhang, J. W. Zhu, X. M. Sun and L. Wang, Controllable synthesis of a self-assembled ultralow Ru, Ni-doped Fe2O3 lily as a bifunctional electrocatalyst for large-current-density alkaline seawater electrolysis, Chin. J. Catal., 2022, 43, 2202–2211 CrossRef CAS.
- T. Wu, E. Song, S. Zhang, M. Luo, C. Zhao, W. Zhao, J. Liu and F. Huang, Engineering Metallic Heterostructure Based on Ni3N and 2M-MoS2 for Alkaline Water Electrolysis with Industry-Compatible Current Density and Stability, Adv. Mater., 2022, 34, e2108505 CrossRef PubMed.
- W. L. Liang, M. Y. Zhou, X. Y. Lin, J. C. Xu, P. Y. Dong, Z. C. Le, M. Z. Yang, J. Chen, F. Y. Xie, N. Wang, Y. S. Jin and H. Meng, Nickel-doped tungsten oxide promotes stable and efficient hydrogen evolution in seawater, Appl. Catal., B, 2023, 325, 122397 CrossRef CAS.
- H.-Y. Wang, J.-T. Ren, L. Wang, M.-L. Sun, H.-M. Yang, X.-W. Lv and Z.-Y. Yuan, Synergistically enhanced activity and stability of bifunctional nickel phosphide/sulfide heterointerface electrodes for direct alkaline seawater electrolysis, J. Energy Chem., 2022, 75, 66–73 CrossRef CAS.
- A. K. Tareen, G. S. Priyanga, K. Khan, E. Pervaiz, T. Thomas and M. Yang, Nickel-Based Transition Metal Nitride Electrocatalysts for the Oxygen Evolution Reaction, ChemSusChem, 2019, 12, 3941–3954 CrossRef CAS PubMed.
- L. Li, X. Cao, J. Huo, J. Qu, W. Chen, C. Liu, Y. Zhao, H. Liu and G. Wang, High valence metals engineering strategies of Fe/Co/Ni-based catalysts for boosted OER electrocatalysis, J. Energy Chem., 2023, 76, 195–213 CrossRef CAS.
- N. Hales, T. J. Schmidt and E. Fabbri, Reversible and irreversible transformations of Ni-based electrocatalysts during the oxygen evolution reaction, Curr. Opin. Electrochem., 2023, 38, 101231 CrossRef CAS.
- H. X. Wang, M. Y. Cui, G. L. Fu, J. Y. Zhang, X. Y. Ding, I. Azaceta, M. Bugnet, D. M. Kepaptsoglou, V. K. Lazarov, V. A. D. O'Shea, F. E. Oropeza and K. H. L. Zhang, Vertically aligned Ni/NiO nanocomposites with abundant oxygen deficient hetero-interfaces for enhanced overall water splitting, Sci. China: Chem., 2022, 65, 1885–1894 CrossRef CAS.
- H. Khadijeh, A. Kumar, A. R. Jadhav, O. Moradlou, A. Z. Moshfegh and H. Lee, Nanorod Array-Based Hierarchical NiO Microspheres as a Bifunctional Electrocatalyst for a Selective and Corrosion-Resistance Seawater Photo/Electrolysis System, ACS Catal., 2023, 13, 5516–5528 CrossRef.
- H. T. Qian, J. Wei, C. C. Yu, F. Tang, W. Jiang, D. S. Xia and L. Gan, In Situ Quantification of the Active Sites, Turnover Frequency, and Stability of Ni-Fe (Oxy)hydroxides for the Oxygen Evolution Reaction, ACS Catal., 2022, 12, 14280–14289 CrossRef CAS.
- D. N. Zhang, H. Cheng, X. Y. Hao, Q. Sun, T. Y. Zhang, X. W. Xu, Z. L. Ma, T. Yang, J. Ding, X. Q. Liu, M. Yang and X. L. Huang, Stable Seawater Oxidation at High-Salinity Conditions Promoted by Low Iron-Doped Non-Noble-Metal Electrocatalysts, ACS Catal., 2023, 13, 15581–15590 CrossRef CAS.
- C. Debabrata, H. Jaromir, B. Tomas, P. Martin and B. Karel, Optimization of synthesis of the nickel-cobalt oxide based anode electrocatalyst and of the related membrane-electrode assembly for alkaline water electrolysis, J. Power Sources, 2017, 347, 247–258 CrossRef.
- T. Haq and Y. Haik, NiOx–FeOx Nanoclusters Anchored on g-C3N4 Sheets for Selective Seawater Oxidation with High Corrosion Resistance, ACS Sustain. Chem. Eng., 2022, 10, 6622–6632 CrossRef CAS.
- Z. He, J. Zhang, Z. Gong, H. Lei, D. Zhou, N. Zhang, W. Mai, S. Zhao and Y. Chen, Activating lattice oxygen in NiFe-based (oxy)hydroxide for water electrolysis, Nat. Commun., 2022, 13, 2191 CrossRef CAS PubMed.
- Y. Q. Wang, S. Tao, H. Lin, G. P. Wang, K. N. Zhao, R. M. Cai, K. W. Tao, C. X. Zhang, M. Z. Sun, J. Hu, B. L. Huang and S. H. Yang, Atomically targeting NiFe LDH to create multivacancies for OER catalysis with a small organic anchor, Nano Energy, 2021, 81, 105606 CrossRef CAS.
- M. H. Wang, Z. X. Lou, X. Wu, Y. Liu, J. Y. Zhao, K. Z. Sun, W. X. Li, J. Chen, H. Y. Yuan, M. Zhu, S. Dai, P. F. Liu and H. G. Yang, Operando High-Valence Cr-Modified NiFe Hydroxides for Water Oxidation, Small, 2022, 18, e2200303 CrossRef PubMed.
- Y. C. Yao, S. J. Sun, H. Zhang, Z. X. Li, C. X. Yang, Z. W. Cai, X. He, K. Dong, Y. L. Luo, Y. Wang, Y. C. Ren, Q. Liu, D. D. Zheng, W. H. Zhuang, B. Tang, X. P. Sun and W. C. Hu, Enhancing the stability of NiFe-layered double hydroxide nanosheet array for alkaline seawater oxidation by Ce doping, J. Energy Chem., 2024, 91, 306–312 CrossRef CAS.
- H. D. Qi, K. Huang, F. H. K. Pan, R. T. Ma, C. Lian, H. L. Liu and J. Hu, Boosting Direct Seawater Electrolysis through Intercalation Engineering of Layered Double Hydroxides, Ind. Eng. Chem. Res., 2023, 62, 19674–19682 CrossRef CAS.
- S. Dresp, T. N. Thanh, M. Klingenhof, S. Brückner, P. Hauke and P. Strasser, Efficient direct seawater electrolysers using selective alkaline NiFe-LDH as OER catalyst in asymmetric electrolyte feeds, Energy Environ. Sci., 2020, 13, 1725–1729 RSC.
- J. Chen, X. Shi, S. Feng, J. Li, X. Gao, X. Wu, K. Li, A. Qi, C. You and X. Tian, Design of highly active and durable oxygen evolution catalyst with intrinsic chlorine inhibition property for seawater electrolysis, Nano Mater. Sci., 2023 DOI:10.1016/j.nanoms.2023.10.003.
- Z. Zhang, K. Ye, H. Du and X. Li, In situ electrodeposition synthesis of CoP@NiFe LDH heterostructure as high-performance electrocatalyst for enhanced seawater electrolysis, Int. J. Hydrogen Energy, 2024, 62, 722–731 CrossRef CAS.
- Y. Xia, L. Guo, J. Zhu, J. Tang, Z. Li, X. Liu, J. Chi and L. Wang, Manipulating electronic structure of nickel phosphide via asymmetric coordination interaction for anion-exchange membrane based seawater electrolysis, Appl. Catal., B, 2024, 351, 123995 CrossRef CAS.
- X. Liu, Q. Yu, X. Qu, X. Wang, J. Chi and L. Wang, Manipulating Electron Redistribution in Ni(2) P for Enhanced Alkaline Seawater Electrolysis, Adv. Mater., 2024, 36, e2307395 CrossRef PubMed.
- Y. Yu, X. Chen, J. Li, Y. Xiao, X. Shi, P. Rao, P. Deng, H. Wen and X. Tian, Ni-based heterostructure with protective phosphide layer to enhance the oxygen evolution reaction for the seawater electrolysis, Int. J. Hydrogen Energy, 2024, 51, 1373–1380 CrossRef CAS.
- S. J. Lv, Y. Deng, Q. Liu, Z. Q. Fu, X. B. Liu, M. H. Wang, Z. Y. Xiao, B. Li and L. Wang, Carbon-quantum-dots-involved Fe/Co/Ni phosphide open nanotubes for high effective seawater electrocatalytic decomposition, Appl. Catal., B, 2023, 326, 122403 CrossRef CAS.
- H. Zhao, M. Liu, X. Q. Du and X. S. Zhang, Construction of nickel stannum based sulfide as efficient electrocatalysts for freshwater, seawater and urea oxidation, Int. J. Hydrogen Energy, 2024, 58, 117–127 CrossRef CAS.
- L. Yu, Q. Zhu, S. Song, B. McElhenny, D. Wang, C. Wu, Z. Qin, J. Bao, Y. Yu, S. Chen and Z. Ren, Non-noble metal-nitride based electrocatalysts for high-performance alkaline seawater electrolysis, Nat. Commun., 2019, 10, 5106 CrossRef PubMed.
- C. Fu, W. Hao, J. Fan, Q. Zhang, Y. Guo, J. Fan, Z. Chen and G. Li, Fabrication of Ultra-Durable and Flexible NiP(x) -Based Electrode toward High-Efficient Alkaline Seawater Splitting at Industrial Grade Current Density, Small, 2023, 19, e2205689 CrossRef PubMed.
- L. Yang, Y. Zhao, L. Zhu and D. Xia, Rational construction of grille structured P-CoZnO-Cu2SeS/NF composite electrocatalyst for boosting seawater electrolysis and corrosion resistance, Appl. Surf. Sci., 2023, 631, 157541 CrossRef CAS.
- Y. Song, X. Zhang, Z. Xiao, Y. Wang, P. Yi, M. Huang and L. Zhang, Coupled amorphous NiFeP/crystalline Ni3S2 nanosheets enables accelerated reaction kinetics for high current density seawater electrolysis, Appl. Catal., B, 2024, 352, 124028 CrossRef CAS.
- S. W. Wu, S. Q. Liu, X. H. Tan, W. Y. Zhang, K. Cadien and Z. Li, Ni3S2-embedded NiFe LDH porous nanosheets with abundant heterointerfaces for high-current water electrolysis, Chem. Eng. J., 2022, 442, 136105 CrossRef CAS.
- C. Z. Wang, M. Zhu, Z. Cao, P. Zhu, Y. Cao, X. Y. Xu, C. Xu and Z. Y. Yin, Heterogeneous bimetallic sulfides based seawater electrolysis towards stable industrial-level large current density, Appl. Catal., B, 2021, 291, 120071 CrossRef CAS.
- Y. J. Sun, S. Kang, K. M. Kim, S. Mhin, J. C. Kim, S. J. Kim, E. Enkhtuvshin, S. Choi and H. Han, Sulfur-incorporated nickel-iron layered double hydroxides for effective oxygen evolution reaction in seawater, Appl. Surf. Sci., 2021, 568, 150965 CrossRef.
- X. Luo, P. Ji, P. Wang, X. Tan, L. Chen and S. Mu, Spherical Ni3S2/Fe-NiPx Magic Cube with Ultrahigh Water/Seawater Oxidation Efficiency, Adv. Sci., 2022, 9, e2104846 CrossRef PubMed.
- M. A. N. Manar, B. Z. Mohamed, M. E.-B. Haitham, G. M. Gehad and E. E.-K. Mohamed, Two-dimensional nickel cyano-bridged coordination polymer thermally derived potent electrocatalysts for alkaline hydrogen evolution reaction, J. Mater. Chem. A, 2023, 11, 24261–24271 RSC.
- X. Wang, G. Liu, D. Zhang, S. Han, J. Yin, J. Jiang, W. Wang and Z. Li, N-doped carbon sheets supported P-Fe3O4-MoO2 for freshwater and seawater electrolysis, J. Colloid Interface Sci., 2023, 652, 1217–1227 CrossRef CAS PubMed.
- B. R. Wang, M. J. Lu, D. Chen, Q. Zhang, W. W. Wang, Y. T. Kang, Z. X. Fang, G. S. Pang and S. H. Feng, NixFeyN@C microsheet arrays on Ni foam as an efficient and durable electrocatalyst for electrolytic splitting of alkaline seawater, J. Mater. Chem. A, 2021, 9, 13562–13569 RSC.
- Y. Q. Zhao, B. Jin, A. Vasileff, Y. Jiao and S. Z. Qiao, Interfacial nickel nitride/sulfide as a bifunctional electrode for highly efficient overall water/seawater electrolysis, J. Mater. Chem. A, 2019, 7, 8117–8121 RSC.
- H. Sun, J. Sun, Y. Song, Y. Zhang, Y. Qiu, M. Sun, X. Tian, C. Li, Z. Lv and L. Zhang, Nickel-Cobalt Hydrogen Phosphate on Nickel Nitride Supported on Nickel Foam for Alkaline Seawater Electrolysis, ACS Appl. Mater. Interfaces, 2022, 14, 22061–22070 CrossRef CAS PubMed.
- L. Lin, S. Piao, Y. Choi, L. Lyu, H. Hong, D. Kim, J. Lee, W. Zhang and Y. Piao, Nanostructured Transition Metal Nitrides as Emerging Electrocatalysts for Water Electrolysis: Status and Challenges, EnergyChem, 2022, 4, 100072 CrossRef CAS.
- S. Gopalakrishnan, G. Anandha babu, S. Harish, E. S. Kumar and M. Navaneethan, Interface engineering of heterogeneous NiMn layered double hydroxide/vertically aligned NiCo2S4 nanosheet as highly efficient hybrid electrocatalyst for overall seawater splitting, Chemosphere, 2024, 350, 141016 CrossRef CAS PubMed.
- J. Zhou, J. Liang, Z. Fan, W. Tan, X. Sun, R. Ding, P. Gao and Y. Zhang, NiFe2O4/Ni2P Mott-Schottky heterojunction boosts the oxygen evolution reaction in alkaline water/natural seawater, Int. J. Hydrogen Energy, 2024, 51, 770–778 CrossRef CAS.
- Y. Huang, J. R. Han, H. B. Wang, L. H. Liu and H. Y. Liang, Multiple metallic dopants in nickel nanoparticles for electrocatalytic oxygen evolution, Prog. Nat. Sci.: Mater. Int., 2023, 33, 67–73 CrossRef CAS.
- D. Wu, D. Chen, J. Zhu and S. Mu, Ultralow Ru Incorporated Amorphous Cobalt-Based Oxides for High-Current-Density Overall Water Splitting in Alkaline and Seawater Media, Small, 2021, 17, e2102777 CrossRef PubMed.
- S. H. Wang, P. Yang, X. F. Sun, H. L. Xing, J. Hu, P. Chen, Z. T. Cui, W. K. Zhu and Z. J. Ma, Synthesis of 3D heterostructure Co-doped Fe2P electrocatalyst for overall seawater electrolysis, Appl. Catal., B, 2021, 297, 120386 CrossRef CAS.
- C. Q. Huang, Q. C. Zhou, L. Yu, D. S. Duan, T. Y. Cao, S. H. Qiu, Z. Z. Wang, J. Guo, Y. X. Xie, L. P. Li and Y. Yu, Functional Bimetal Co-Modification for Boosting Large-Current-Density Seawater Electrolysis by Inhibiting Adsorption of Chloride Ions, Adv. Energy Mater., 2023, 13, 2301475 CrossRef CAS.
- L. Shao, X. D. Han, L. Shi, T. Z. Wang, Y. S. Zhang, Z. Q. Jiang, Z. X. Yin, X. R. Zheng, J. H. Li, X. P. Han and Y. D. Deng, In Situ Generation of Molybdate-Modulated Nickel-Iron Oxide Electrodes with High Corrosion Resistance for Efficient Seawater Electrolysis, Adv. Energy Mater., 2024, 14, 2303261 CrossRef CAS.
- C. Xu, J. Zhao, Z. Zhao, W. Zhang and X. Wang, Surface reconstruction in amorphous CoFe-based hydroxides/crystalline phosphide heterostructure for accelerated saline water electrolysis, J. Colloid Interface Sci., 2024, 659, 821–832 CrossRef PubMed.
- L. Ji, C. Lv, Z. Chen, Z. Huang and C. Zhang, Nickel-Based (Photo)Electrocatalysts for Hydrogen Production, Adv. Mater., 2018, 30, e1705653 CrossRef PubMed.
- B. Guo, X. Wen, L. Xu, X. Ren, S. Niu, R. YangCheng, G. Ma, J. Zhang, Y. Guo, P. Xu and S. Li, Noble Metal Phosphides: Robust Electrocatalysts toward Hydrogen Evolution Reaction, Small Methods, 2023, e2301469 CrossRef PubMed.
- S. H. Ye, W. Xiong, P. Liao, L. R. Zheng, X. Z. Ren, C. X. He, Q. L. Zhang and J. H. Liu, Removing the barrier to water dissociation on single-atom Pt sites decorated with a CoP mesoporous nanosheet array to achieve improved hydrogen evolution, J. Mater. Chem. A, 2020, 8, 11246–11254 RSC.
- W. L. Yu, Z. Chen, Y. L. Fu, W. P. Xiao, B. Dong, Y. M. Chai, Z. X. Wu and L. Wang, Superb All-pH Hydrogen Evolution Performances Powered by Ultralow Pt-Decorated Hierarchical Ni-Mo Porous Microcolumns, Adv. Funct. Mater., 2023, 33, 2210855 CrossRef CAS.
- L. Wang, H. Tao, Y. Liu, Y. Chen, Z. Chen, X. Yang, B. Yang, Z. Li, Q. Dai, C. Lian, L. Lei and Y. Hou, Multiscale modulation of ultra-small ruthenium anchored on bimetallic sulfides for seawater electrolysis at ampere-level current density, Nano Energy, 2024, 124, 109481 CrossRef CAS.
- X. J. Zhai, Q. P. Yu, G. S. Liu, J. L. Bi, Y. Zhang, J. Q. Chi, J. P. Lai, B. Yang and L. Wang, Hierarchical microsphere MOF arrays with ultralow Ir doping for efficient hydrogen evolution coupled with hydrazine oxidation in seawater, J. Mater. Chem. A, 2021, 9, 27424–27433 RSC.
- H. S. Hu, Z. R. Zhang, Y. W. Zhang, T. Thomas, H. Y. Du, K. K. Huang, J. P. Attfield and M. H. Yang, An ultra-low Pt metal nitride electrocatalyst for sustainable seawater hydrogen production, Energy Environ. Sci., 2023, 16, 4584–4592 RSC.
- J. Su, Q. Wang, M. Fang, Y. Wang, J. Ke, Q. Shao and J. Lu, Metastable Hexagonal-Phase Nickel with Ultralow Pt Content for an Efficient Alkaline/Seawater Hydrogen Evolution Reaction, ACS Appl. Mater. Interfaces, 2023, 51160–51169 CrossRef CAS PubMed.
- J. Na, H. Yu, S. Jia, J. Chi, K. Lv, T. Li, Y. Zhao, Y. Zhao, H. Zhang and Z. Shao, Electrochemical reconstruction of non-noble metal-based heterostructure nanorod arrays electrodes for highly stable anion exchange membrane seawater electrolysis, J. Energy Chem., 2024, 91, 370–382 CrossRef CAS.
- J. Jiang, Y. Tian, J. Zhang, C. Zhang and L. Ai, Metallic Cu-incorporated NiFe layered double hydroxide nanosheets enabling energy-saving hydrogen generation from chlorine-free seawater electrolysis coupled with sulfion upcycling, Fuel, 2024, 367, 131506 CrossRef CAS.
- X. H. Wu, J. S. Qiu and Z. Y. Wang, Rare-Earth Doping Transitional Metal Phosphide for Efficient Hydrogen Evolution in Natural Seawater, Small Struct., 2023, 4, 2200268 CrossRef CAS.
- X. Y. Lu, J. Pan, E. Lovell, T. H. Tan, Y. H. Ng and R. Amal, A sea-change: manganese doped nickel/nickel oxide electrocatalysts for hydrogen generation from seawater, Energy Environ. Sci., 2018, 11, 1898–1910 RSC.
- Z. X. Zhu, L. Luo, Y. X. He, M. Mushtaq, J. Q. Li, H. Yang, Z. Khanam, J. Qu, Z. M. Wang and M. S. Balogun, High-Performance Alkaline Freshwater and Seawater Hydrogen Catalysis by Sword-Head Structured Mo2N-Ni3Mo3N Tunable Interstitial Compound Electrocatalysts, Adv. Funct. Mater., 2023, 34, 2306061 CrossRef.
- N. B. Ysea, V. B. Llorente, A. Loiacono, L. L. Marquez, L. Diaz, G. I. Lacconi and E. A. Franceschini, Critical insights from alloys and composites of Ni-based electrocatalysts for HER on NaCl electrolyte, J. Alloys Compd., 2022, 915, 165352 CrossRef CAS.
- X. M. Bu, X. Y. Liang, Y. Bu, Q. Quan, Y. Meng, Z. X. Lai, W. Wang, C. T. Liu, J. Lu, C. M. L. C. Wu and J. Ho, NiMo@CN heterostructures with multiple electronic transmission channels for highly efficient hydrogen evolution from alkaline electrolytes and seawater, Chem. Eng. J., 2022, 438, 135379 CrossRef CAS.
- Z. H. Ge, B. Fu, J. P. Zhao, X. Li, B. Ma and Y. T. Chen, A review of the electrocatalysts on hydrogen evolution reaction with an emphasis on Fe, Co and Ni-based phosphides, J. Mater. Sci., 2020, 55, 14081–14104 CrossRef CAS.
- H. Zhang, Y. L. Wang, B. Zhang, S. L. Zhang, Y. R. Ma, Z. X. Wu, Y. J. Zhu, F. S. Liu, Z. Y. Xiao and L. Wang, Interwoven N-doped carbon nanotubes with capped Ni-doped FeP as double-functional electrocatalysts for overall seawater electrolysis, Sci. China Mater., 2023, 66, 4630–4638 CrossRef CAS.
- J. T. Ren, L. Chen, W. W. Tian, X. L. Song, Q. H. Kong, H. Y. Wang and Z. Y. Yuan, Rational Synthesis of Core-Shell-Structured Nickel Sulfide-Based Nanostructures for Efficient Seawater Electrolysis, Small, 2023, 19, e2300194 CrossRef PubMed.
- T. Zhao, S. Wang, C. Jia, C. Rong, Z. Su, K. Dastafkan, Q. Zhang and C. Zhao, Cooperative Boron and Vanadium Doping of Nickel Phosphides for Hydrogen Evolution in Alkaline and Anion Exchange Membrane Water/Seawater Electrolyzers, Small, 2023, 19, e2208076 CrossRef PubMed.
- Y. Zou, M. Jin, D. Zhu and Y. J. Tang, Surface Adsorption of Amorphous Phosphate on RuNi-Doped Molybdate for the Hydrogen Evolution Reaction, Inorg. Chem., 2023, 62, 15757–15765 CrossRef CAS PubMed.
- W. Xu, T. Ma, H. Chen, D. Pan, Z. Wang, S. Zhang, P. Zhang, S. Bao, Q. Yang, L. Zhou, Z. Tian, S. Dai and Z. Lu, Scalable Fabrication of Cu2S@NiS@Ni/NiMo Hybrid Cathode for High-Performance Seawater Electrolysis, Adv. Funct. Mater., 2023, 33, 2302263 CrossRef CAS.
- J. Fan, X. Ma, J. Xia, L. Zhang, Q. Bi and W. Hao, Corrosion resistance and earth-abundance FeS-based heterojunction catalyst for seawater splitting at industrial grade density, J. Colloid Interface Sci., 2024, 657, 393–401 CrossRef CAS PubMed.
- H. Jin, X. Wang, C. Tang, A. Vasileff, L. Li, A. Slattery and S. Z. Qiao, Stable and Highly Efficient Hydrogen Evolution from Seawater Enabled by an Unsaturated Nickel Surface Nitride, Adv. Mater., 2021, 33, e2007508 CrossRef PubMed.
- A. Badreldin, A. Nabeeh, Z. K. Ghouri, J. Abed, N. Wang, Y. Wubulikasimu, K. Youssef, D. Kumar, M. K. Stodolny, K. Elsaid, E. H. Sargent and A. Abdel-Wahab, Early Transition-Metal-Based Binary Oxide/Nitride for Efficient Electrocatalytic Hydrogen Evolution from Saline Water in Different pH Environments, ACS Appl. Mater. Interfaces, 2021, 13, 53702–53716 CrossRef CAS PubMed.
- X. Wang, X. Zhang, Y. Xu, H. Song, X. Min, Z. Tang, C. Pi, J. Li, B. Gao, Y. Zheng, X. Peng, P. K. Chu and K. Huo, Heterojunction Mo-based binary and ternary nitride catalysts with Pt-like activity for the hydrogen evolution reaction, Chem. Eng. J., 2023, 470, 144370 CrossRef CAS.
- R. Y. Fan, X. Y. Zhang, N. Yu, F. G. Wang, H. Y. Zhao, X. Liu, Q. X. Lv, D. P. Liu, Y. M. Chai and B. Dong, Rapid “self-healing” behavior induced by chloride anions to renew the Fe-Ni(oxy)hydroxide surface for long-term alkaline seawater electrolysis, Inorg. Chem. Front., 2022, 9, 4216–4224 RSC.
- J. W. Koster, S. A. Tornoe, N. P. Kobayashi and D. C. Potts, Explicitly controlling electrical current density overpowers the kinetics of the chlorine evolution reaction and increases the hydrogen production during seawater electrolysis, Int. J. Hydrogen Energy, 2023, 48, 4994–5000 CrossRef CAS.
- H. Tian and J. Ge, High-efficiency direct seawater electrolysis enabled by electrode microenvironment regulation, Chem Catal., 2023, 3, 100580 CrossRef CAS.
- H.-M. Zhang and J. Li, Strategies for overcoming seawater adverse effects on cathodic hydrogen evolution reaction electrocatalysts, Fuel, 2024, 367, 131505 CrossRef CAS.
- X. Zhou, R. F. Taylor, L. Shi, C. Xie, B. Bian and B. E. Logan, Reducing Chloride Ion Permeation during Seawater Electrolysis Using Double-Polyamide Thin-Film Composite Membranes, Environ. Sci. Technol., 2024, 58, 391–399 CrossRef CAS PubMed.
- P. Li, S. Zhao, Y. Q. Huang, Q. H. Huang, B. J. Xi, X. G. An and S. L. Xiong, Corrosion Resistant Multilayered Electrode Comprising Ni3N Nanoarray Overcoated with NiFe-Phytate Complex for Boosted Oxygen Evolution in Seawater Electrolysis, Adv. Energy Mater., 2024, 14, 2303360 CrossRef CAS.
- B. Zhang, S. Liu, S. Zhang, Y. Cao, H. Wang, C. Han and J. Sun, High Corrosion Resistance of NiFe-Layered Double Hydroxide Catalyst for Stable Seawater Electrolysis Promoted by Phosphate Intercalation, Small, 2022, 18, e2203852 CrossRef PubMed.
- C. C. Li, B. Zhu, Z. X. Liu, J. T. Zhao, R. R. Meng, L. S. Zhang and Z. G. Chen, Polyelectrolyte-based photothermal hydrogel with low evaporation enthalpy for solar-driven salt-tolerant desalination, Chem. Eng. J., 2022, 431, 134224 CrossRef CAS.
- Y. C. Li, X. Y. Wu, J. P. Wang, H. X. Wei, S. Y. Zhang, S. L. Zhu, Z. Y. Li, S. L. Wu, H. Jiang and Y. Q. Liang, Sandwich structured Ni3S2-MoS2-Ni3S2@Ni foam electrode as a stable bifunctional electrocatalyst for highly sustained overall seawater splitting, Electrochim. Acta, 2021, 390, 138833 CrossRef CAS.
- X. Lyu and A. Serov, Cutting-edge methods for amplifying the oxygen evolution reaction during seawater electrolysis: a brief synopsis, Ind. Chem. Mater., 2023, 1, 475–485 RSC.
- T. Ma, W. Xu, B. Li, X. Chen, J. Zhao, S. Wan, K. Jiang, S. Zhang, Z. Wang, Z. Tian, Z. Lu and L. Chen, The Critical Role of Additive Sulfate for Stable Alkaline Seawater Oxidation on Nickel-Based Electrodes, Angew Chem. Int. Ed. Engl., 2021, 60, 22740–22744 CrossRef CAS PubMed.
- M. Tang, K. Du, R. Yu, H. Shi, P. Wang, Y. Guo, Q. Wei, H. Yin and D. Wang, Microzone-Acidification-Driven Degradation Mechanism of the NiFe-Based Anode in Seawater Electrolysis, ACS Appl. Mater. Interfaces, 2024, 16, 3260–3269 CrossRef CAS PubMed.
- H. Komiya, K. Obata, T. Honma and K. Takanabe, Dynamic stabilization of nickel-based oxygen evolution electrocatalysts in the presence of chloride ions using a phosphate additive, J. Mater. Chem. A, 2024, 12, 3513–3522 RSC.
- T. T. He, Q. F. Liu, H. F. Fan, Y. Yang, H. T. Wang, S. Z. Zhang, R. X. Che and E. R. Wang, Exploring the effect of ion concentrations on the electrode activity and stability for direct alkaline seawater electrolysis, Int. J. Hydrogen Energy, 2023, 48, 19385–19395 CrossRef CAS.
- S. A. Patil, A. C. Khot, V. D. Chavan, I. Rabani, D.-k. Kim, J. Jung, H. Im and N. K. Shrestha, Electrostatically robust CoFeOF nanosheet against chloride for green-H2 production in alkaline seawater electrolysis, Chem. Eng. J., 2024, 480, 146545 CrossRef CAS.
- L. J. Yang, D. Lu, L. J. Zhu and D. H. Xia, Construction of Mo doped CoMoCH-Cu2SeS/NF composite electrocatalyst with high catalytic activity and corrosion resistance in seawater electrolysis: A case study on cleaner energy, J. Cleaner Prod., 2023, 413, 137462 CrossRef CAS.
- S. Ge, X. Shen, J. Gao, K. Ma, H. Zhao, R. Fu, C. Feng, Y. Zhao, Q. Jiao and H. Li, Synergy of Mo doping and heterostructures in FeCo2S4@Mo-NiCo LDH/NF as durable and corrosion-resistance bifunctional electrocatalyst towards seawater electrolysis at industrial current density, Chem. Eng. J., 2024, 485, 150161 CrossRef CAS.
- W. Ou, W. Zhang, H. Qin, W. Zhou, Y. Tang and Q. Gao, Enhancing anti-chlorine corrosion of Ni3S2 by Mo-doping for mimic seawater electrolysis, J. Colloid Interface Sci., 2024, 655, 852–862 CrossRef CAS PubMed.
- H. W. Bian, P. Qi, G. W. Xie, X. Liu, D. Zhang and P. Wang, HEA-NiFeCuCoCe/NF through ultra-fast electrochemical self-reconstruction with high catalytic activity and corrosion resistance for seawater electrolysis, Chem. Eng. J., 2023, 477, 147286 CrossRef CAS.
- Y. Z. Shi, B. Yang and P. K. Liaw, Corrosion-Resistant High-Entropy Alloys: A Review, Metals, 2017, 7, 43 CrossRef.
- R. Ortiz-Imedio, D. G. Caglayan, A. Ortiz, H. Heinrichs, M. Robinius, D. Stolten and I. Ortiz, Power-to-Ships: Future electricity and hydrogen demands for shipping on the Atlantic coast of Europe in 2050, Energy, 2021, 228, 120660 CrossRef.
- D. Y. Li, L. L. Liao, H. Q. Zhou, Y. Zhao, F. M. Cai, J. S. Zeng, F. Liu, H. Wu, D. S. Tang and F. Yu, Highly active non-noble electrocatalyst from Co2P/Ni2P nanohybrids for pH-universal hydrogen evolution reaction, Mater. Today Phys., 2021, 16, 100314 CrossRef CAS.
- F. Shiokawa, A. Asilah Haji Tajuddin, T. Ohto, Y. Yu, T. Fujita, H. Tanimoto, Z. Xi, S. Jeong and Y. Ito, Durable high-entropy non-noble metal anodes for neutral seawater electrolysis, Chem. Eng. J., 2024, 479, 147862 CrossRef CAS.
- N. Wen, Y. Xia, H. Wang, D. Zhang, H. Wang, X. Wang, X. Jiao and D. Chen, Large-Scale Synthesis of Spinel NixMn3-xO4 Solid Solution Immobilized with Iridium Single Atoms for Efficient Alkaline Seawater Electrolysis, Adv. Sci., 2022, 9, e2200529 CrossRef PubMed.
- Q. Zhou, L. L. Liao, H. Q. Zhou, D. Y. Li, D. S. Tang and F. Yu, Innovative strategies in design of transition metal-based catalysts for large-current-density alkaline water/seawater electrolysis, Mater. Today Phys., 2022, 26, 100727 CrossRef CAS.
- Y. X. Zhao, Q. Y. Sun, C. F. Zhang, F. S. Liu, L. Wang and G. R. Xu, Self-supported electrocatalysts for high-current-density water/seawater electrolysis, J. Alloys Compd., 2023, 968, 172286 CrossRef CAS.
- A. Mishra, H. Park, F. El-Mellouhi and D. Suk Han, Seawater electrolysis for hydrogen production: Technological advancements and future perspectives, Fuel, 2024, 361, 130636 CrossRef CAS.
- M. J. Liang, R. Karthick, Q. Wei, J. H. Dai, Z. S. Jiang, X. C. Chen, T. Z. Oo, S. H. Aung and F. M. Chen, The progress and prospect of the solar-driven photoelectrochemical desalination, Renewable Sustainable Energy Rev., 2022, 155, 111864 CrossRef CAS.
- S. Zhang, W. Xu, H. Chen, Q. Yang, H. Liu, S. Bao, Z. Tian, E. Slavcheva and Z. Lu, Progress in Anode Stability Improvement for Seawater Electrolysis to Produce Hydrogen, Adv. Mater., 2024, e2311322 CrossRef PubMed.
- W. Zheng, L. Y. S. Lee and K. Y. Wong, Improving the performance stability of direct seawater electrolysis: from catalyst design to electrode engineering, Nanoscale, 2021, 13, 15177–15187 RSC.
|
This journal is © The Royal Society of Chemistry 2024 |