DOI:
10.1039/D3MA00736G
(Review Article)
Mater. Adv., 2024,
5, 4025-4054
Biomaterial strategies for regulating the neuroinflammatory response
Received
20th September 2023
, Accepted 7th April 2024
First published on 10th April 2024
Abstract
Injury and disease in the central nervous system (CNS) can result in a dysregulated inflammatory environment that inhibits the repair of functional tissue. Biomaterials present a promising approach to tackle this complex inhibitory environment and modulate the mechanisms involved in neuroinflammation to halt the progression of secondary injury and promote the repair of functional tissue. In this review, we will cover recent advances in biomaterial strategies, including nanoparticles, hydrogels, implantable scaffolds, and neural probe coatings, that have been used to modulate the innate immune response to injury and disease within the CNS. The stages of inflammation following CNS injury and the main inflammatory contributors involved in common neurodegenerative diseases will be discussed, as understanding the inflammatory response to injury and disease is critical for identifying therapeutic targets and designing effective biomaterial-based treatment strategies. Biomaterials and novel composites will then be discussed with an emphasis on strategies that deliver immunomodulatory agents or utilize cell–material interactions to modulate inflammation and promote functional tissue repair. We will explore the application of these biomaterial-based strategies in the context of nanoparticle- and hydrogel-mediated delivery of small molecule drugs and therapeutic proteins to inflamed nervous tissue, implantation of hydrogels and scaffolds to modulate immune cell behavior and guide axon elongation, and neural probe coatings to mitigate glial scarring and enhance signaling at the tissue–device interface. Finally, we will present a future outlook on the growing role of biomaterial-based strategies for immunomodulation in regenerative medicine and neuroengineering applications in the CNS.
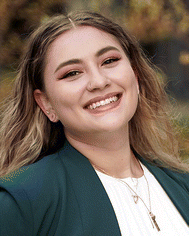
Alycia N. Galindo
| Alycia N. Galindo is a PhD candidate at the University of Oregon's Knight Campus for Accelerating Scientific Impact, working under the supervision of Dr. Marian Hettiaratchi. She holds a Bachelor of Science in Chemical Engineering with a concentration in Bioengineering from the University of New Mexico. During her undergraduate studies, she contributed to the NSF Center for Innovative and Strategic Transformation of Alkane Resources, specializing in fabricating mesoporous silica membranes for dehydrogenation. Currently, her research focuses on affinity-based delivery of neurotrophic factors for treating spinal cord injuries and developing aligned scaffolds for myoblast and neuronal alignment. |
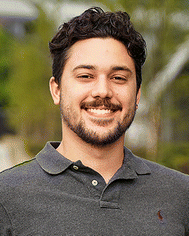
David A. Frey Rubio
| David A. Frey Rubio is a PhD student in the joint bioengineering program at University of Oregon and Oregon State University, conducting research under the guidance of Dr. Marian Hettiaratchi at the Knight Campus for Accelerating Scientific Impact. Holding a Bachelor of Science degree in Biomedical Engineering from the Georgia Institute of Technology (2021), he honed his skills as a lab technician at the Marcus Center for Therapeutic Cell Characterization and Manufacturing before transitioning to the University of Oregon. His current research focuses on innovating affinity-based biomaterial coatings for regulating the neuroinflammatory response towards penetrative neural probes. |
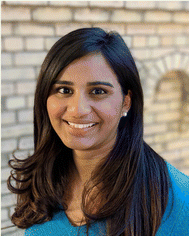
Marian H. Hettiaratchi
| Dr. Marian Hettiaratchi is an Assistant Professor at the Knight Campus for Accelerating Scientific Impact at the University of Oregon. She received a BSc in chemical engineering with a biomedical specialization from the University of Calgary and PhD in biomedical engineering from the Georgia Institute of Technology and Emory University. She was a Natural Sciences and Engineering Research Council of Canada (NSERC) postdoctoral fellow at the University of Toronto. Dr. Hettiaratchi's lab focuses on developing the next generation of affinity-controlled protein delivery vehicles through a combination of polymer chemistry, directed evolution platforms, statistical and bio-transport modeling, and rational protein design. She is the recipient of the NSF CAREER Award, NIH R21 Trailblazer Award, and NIH Maximizing Investigators’ Research Award (MIRA). |
1. Introduction
Neuroinflammation is a process through which the central and peripheral nervous systems combat pathogens, remove extracellular debris, and respond to tissue damage to maintain tissue homeostasis. However, if left unresolved, neuroinflammation will negatively affect neuronal health and result in chronic tissue damage and subsequent loss of function. Consequently, dysregulated neuroinflammation has been identified as a hallmark of many neurodegenerative conditions, including traumatic injury and neurodegenerative disorders such as Alzheimer's disease and multiple sclerosis. Injury to the central nervous system (CNS) can occur from motor vehicle accidents, sports, and falls. Globally, there are 27 million individuals who are currently living with a disability following spinal cord injury (SCI) and approximately 50 million individuals who are affected by a traumatic brain injury (TBI) every year.1–4 Additionally, approximately 15% of the worldwide population is estimated to be currently suffering from some form of neurodegenerative disease with an associated annual cost of approximately $655 billion in the United States alone in 2020.5,6 With an aging global population on the horizon, the prevalence of neuroinflammatory diseases is expected to increase, requiring more research efforts in these areas than ever before.7,8
Since the 1960s, there have been 1200–3000 clinical studies investigating treatments for neuroinflammatory diseases. Combinations of anti-inflammatory drugs,9–11 antioxidants,12–14 corticosteroids,15,16 exercise,17–19 and tailored diets20–24 have been implemented with limited long-term success. While these strategies can help manage disease symptoms, they fail to fully resolve inflammation within the CNS. Our understanding of neuroinflammation is evolving, opening numerous opportunities for new treatment strategies. For example, microglia and astrocytes are critical mediators of neuroinflammation and are the target of many new therapeutics to treat dysregulated immune responses. Biomaterial-based treatment strategies are emerging as a novel approach to address neuroinflammation. Biomaterial-based delivery vehicles such as nanoparticles and hydrogels have shown promise in delivering drugs, proteins, and cells across the intact blood-brain barrier (BBB). Nanoparticles delivered intravenously can extend systemic drug circulation, increasing the likelihood of a therapeutic crossing the BBB and enhancing delivery of therapeutics to target cells in the CNS.25 Conversely, hydrogels are more commonly delivered directly to the site of interest in the CNS for local drug, protein, or cell delivery.26–29 Both hydrogels and nanoparticles can be functionalized with targeting ligands to promote localization within specific environments and modulate cell behavior;30 however, while nanoparticles excel in systemic drug delivery and blood-brain barrier penetration, hydrogels must be locally administered and are thus better suited to treating focal CNS injuries.26,31 Similarly, bioactive cues can be incorporated into tissue engineered scaffolds to guide neural axon elongation, while various surface coatings have been applied on neural probes to enhance neuron signal recording and stimulation at the tissue–device interface.32–34 In this review, we will discuss recent advances in the development of biomaterial strategies for addressing neuroinflammation with a focus on nanoparticles, hydrogels, implantable scaffolds, and neural probe coatings.
2. Background
2.1 Resident cells of the central nervous system
CNS parenchymal tissue is composed of an intricate combination of glycosaminoglycans (e.g., hyaluronic acid), proteoglycans, and glycoproteins (e.g., fibronectin, laminin), but has notably fewer fibrous proteins than muscle or bone (Fig. 1).35–37 This network of extracellular matrix (ECM) molecules provides latching points for neurons to extend and connect to other cells, modulates neural plasticity, and regulates axon regeneration.38 Within this matrix lies a diverse population of support cells called glia, including oligodendrocyte progenitor cells, radial glia, ependymal cells, microglia, oligodendrocytes, and astrocytes. Together, these cells maintain tissue homeostasis by supporting neuron maturation, axon myelination, and nutrient transport, structuring the ECM, and initiating an immune response to foreign bodies such as pathogens, neurotoxins, and implants.39,40
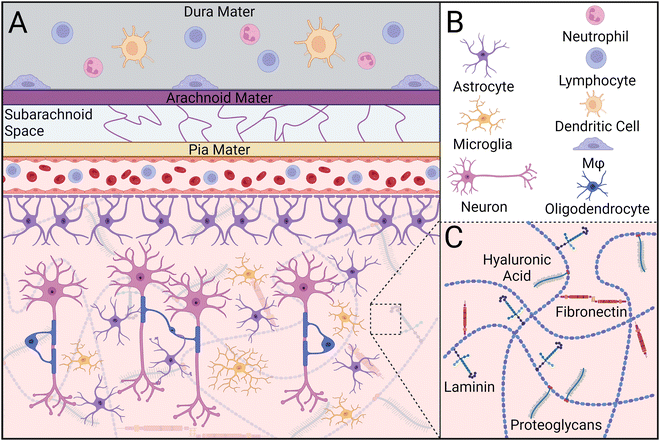 |
| Fig. 1 Composition of central nervous system parenchymal tissue during homeostasis. (A) Peripheral immune cells such as lymphocytes, neutrophils, dendritic cells, and border-associated macrophages (Mφ) reside in the dura mater, contributing to immune surveillance. Astrocytes wrap around neurovasculature, contributing to the integrity and selectivity of the BBB. Astrocytes also facilitate metabolite transport to neighboring glia and neurons. During homeostasis, microglia remain in a branched state, surveying the environment for pathogens, DAMPs, and protein aggregates. Oligodendrocytes myelinate axons and facilitate metabolite transport alongside astrocytes. (B) Different cell types present in CNS tissue. (C) The extracellular matrix in the CNS primarily consists of hyaluronic acid, fibronectin, laminin, and proteoglycans. | |
Microglia are specialized macrophages that serve as the primary innate immune cell population within the CNS (Table 1). In healthy tissue, microglia exist in a “resting” state in which their cytoskeletal protrusions interact with other cells and monitor changes to the local environment.41 Perturbations to parenchymal tissue caused by injury or disease activate microglia, causing alterations in their gene expression and adoption of an amoeboid-like morphology.42,43 Depending on signals perceived in the surrounding environment, stimulated microglia will transition to either a classically activated (M1) or alternatively activated (M2) state, similar to that of macrophages. While M1/M2 nomenclature is typically used to distinguish between pro- and anti-inflammatory phenotypes, respectively, recent studies have shown this system fails to capture the full spectrum of microglial phenotypes.44–46 Collectively, microglia resolve homeostatic disturbances by initiating and orchestrating the inflammatory response, phagocytosing cellular debris, and regulating glial cell behavior.
Table 1 Role of glial cells in neuroinflammation
Cell type |
Role in neuroinflammation |
Microglia |
• Surveillance for pathogen- and damage-associated molecular patterns |
• Remove cellular debris and protein aggregates |
• Facilitate neuronal repair and maturation |
M1-like Microglia |
• Activate local glia via release of pro-inflammatory cytokines |
• Release oxygen and nitrogen reactive species |
• Facilitate immune cell recruitment through chemokine secretion |
M2-like Microglia |
• Remove cellular debris and protein aggregates |
• Neural tissue remodeling |
• Resolve inflammation |
• Promote neurogenesis, angiogenesis, and oligodendrogenesis |
Astrocyte |
• Regulate nutrient transport to neurons |
• Maintain blood-brain barrier selective permeability |
• Modulate neuron synapse plasticity |
• Build the neural extracellular matrix |
A1-like Astrocyte |
• Produce chondroitin sulfate proteoglycans |
• Release oxygen and nitrogen reactive species |
• Release pro-inflammatory cytokines |
A2-like Astrocyte |
• Release neuroprotective factors |
• Resolve inflammation |
• Produce chondroitin sulfate proteoglycans |
Oligodendrocyte |
• Myelinate neuronal axons |
• Regulate nutrient transport to neurons |
• Antigen presentation & T cell activation |
• Regulate microglia activation and peripheral immune cell recruitment |
• Release neuroprotective factors |
Astrocytes are another type of glial cell that make up a large portion of CNS tissue and work in conjunction with microglia to quickly respond to physiological stimuli and pathological perturbations to maintain tissue homeostasis (Table 1). Under healthy conditions, astrocytes interact with neurons and other glia via connexin-based gap junctions.47 These interactions establish large-scale glial networks that regulate neural signal transmission and facilitate nutrient transport. Oligodendrocytes are also included in this network, through which they provide neurotrophic support, deliver metabolites, and myelinate neuronal axons.48 Furthermore, astrocytes can interact with microvessels in the CNS to help maintain endothelial tight junctions within the BBB and blood-spinal cord barrier (BSCB).49 When astrocytes are activated, they undergo significant functional and morphological changes and are typically denoted as A1 and A2 astrocytes, mirroring the widely used macrophage/microglia nomenclature system.
2.2 The role of peripheral immune cells in maintaining tissue homeostasis
CNS parenchyma is segregated from the rest of the body by a series of meningeal layers and the BBB/BSCB. The BBB and BSCB are comprised of tightly packed endothelial cells and protect nervous tissue from many circulating pathogens and neurotoxins.50 Along these tissue borders lie meningeal compartments and perivascular spaces from which bone marrow-derived immune cells can remain in immunological surveillance. This peripheral niche contains several innate and adaptive immune cell populations such as border-associated macrophages (BAMs), dendritic cells, natural killer cells (NKs), T cells, B cells, and neutrophils.51 These cell populations communicate with barrier tissue cells through a series of neurotransmitters, neuropeptides, and cytokines.52 While neurons and glia are the primary residents of CNS parenchyma, recent studies have elaborated on a more active role of peripheral immune cells in maintaining CNS parenchymal homeostasis. Following the onset of injury, the BBB is disrupted, allowing for peripheral immune cell infiltration and inflammatory signaling.53 Innate immune cells can activate glia and facilitate a local tissue response; however, their prolonged presence can be detrimental and is a hallmark of several neurodegenerative diseases characterized by a dysregulated immune response.54–57 Preventing further infiltration of immune cells and managing the inflammatory response is critical for preventing irreparable tissue damage.
2.3 The inflammatory response to central nervous system injury
Trauma to CNS parenchymal tissue by SCI or TBI initiates the death of neurons through apoptosis and necrosis, disrupts the protective BBB/BSCB, and allows peripheral immune cells to infiltrate. Within the damaged tissue environment, local glia and infiltrating immune cells become activated and work together to stabilize the injury site, clear debris, and initiate the healing cascade. While this activity is critical for tissue repair, it can also lead to secondary tissue damage that can prevent timely resolution of neuroinflammation. The inflammatory response to CNS injury is typically broken down into three stages of inflammation, which are each characterized by different time scales, immune cell infiltration rates, protein signaling, and tissue remodeling (Fig. 2). The acute inflammatory phase lasts hours to days after injury, followed by the sub-acute inflammatory phase which lasts up to weeks after injury, and lastly, the chronic inflammatory phase which can last months to years, depending on the severity of the injury. Understanding the cellular and molecular processes that occur throughout each stage of the inflammatory response to CNS injury is critical for designing effective biomaterial-based interventions.
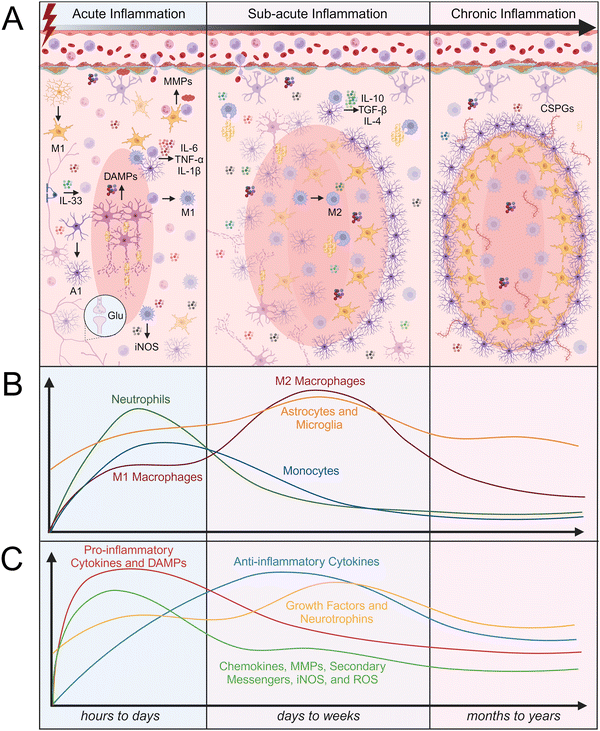 |
| Fig. 2 The stages of the inflammatory response to injury in the central nervous system. The inflammatory response in the CNS can be broken down into the acute inflammatory phase, which lasts hours to days, the sub-acute inflammatory phase, which lasts days to weeks, and chronic inflammatory stage, which lasts months to years. (A) The CNS tissue environment after injury includes an abundance of neurons, glia, and other immune cells as well as a variety of pro- and anti-inflammatory molecules that change throughout the various stages of inflammation. (B) Relative abundance of various immune cells during the stages of inflammation. (C) Relative abundance of pro- and anti-inflammatory cytokines, growth factors, and other biomolecules during the stages of inflammation. Abbreviations: M1 (classically activated macrophages/microglia), M2 (alternatively activated macrophages/microglia), A1 (activated astrocytes), Glu (glutamate), iNOS (inducible nitric oxide synthase), DAMPs (damage-associated molecular patterns), MMPs (matrix metalloproteinases), IL-6 (interleukin-6), TNF-α (tumor necrosis factor-α), IL-1β (interleukin-1β), IL-4 (interleukin-4), IL-10 (interleukin-10), IL-33 (interleukin-33), transforming growth factor-β (TGF-β), CSPGs (chondroitin sulfate proteoglycans). | |
2.3.1 Acute inflammation (hours after injury).
Immediately after traumatic injury to the brain or spinal cord, several events occur that result in the activation of resident glial cells and a rapid influx of peripheral immune cells. Ischemia, edema, and glutamate excitotoxicity begin within the first few hours after injury and initiate local chemokine production to recruit immune cells to the site of injury.58 Small endogenous molecules called damage-associated molecular patterns (DAMPs) are released by damaged and dying cells into the extracellular space. DAMPs activate resident glia and recruit peripheral immune cells, contributing to secondary inflammation, additional tissue damage, and the production of cytokines, inducible nitric oxide synthase (iNOS), and matrix metalloproteinases (MMPs). Cytokines such as interleukin-33 (IL-33), IL-1β, and tumor necrosis factor-α (TNF-α) have all been reported to be released within the first 3 hours after injury.58 IL-33 is an alarmin that is released from damaged oligodendrocytes, causing a cascade of chemokine production from resident astrocytes and microglia that results in the recruitment of neutrophils and monocytes.59–61 iNOS is an enzyme that is produced by infiltrating and activated immune cells and catalyzes the production of nitric oxide, causing neuronal apoptosis. MMPs are peptidases that are produced by neurons, glia, and endothelial cells and degrade the ECM to foster tissue remodeling and reestablish tissue homeostasis. After injury, excess MMP production can cause damage to blood vessels in the perivascular basement membrane.18,61–63 The accumulation of reactive oxygen species (ROS) triggers oxidative stress and disturbs tight junction proteins in the BBB/BSCB, providing an avenue for immune cells to infiltrate. While peripheral immune cells from surrounding tissues infiltrate through the disrupted BBB/BSCB, resident cells including oligodendrocytes, microglia, and astrocytes are activated and continuously release inflammatory signals including cytokines, chemokines, secondary messengers, and ROS.64 Microglia can be activated by cell debris and circulating inflammatory signals to produce inflammatory proteins such as TNF-α, IL-1β, IL-6, and iNOS.64,65 Elevation of such proteins has been detected 6 to 10 hours after injury and peaks within the first day. These cytokines establish a pro-inflammatory environment, resulting in further apoptosis of neurons and glial cells that peaks approximately 8 and 24 hours after injury, respectively. The overall extent of the injury contributes to the amount of pro-inflammatory signals released from injured neural cells, dictating the magnitude of the inflammatory response and secondary tissue damage that occurs. The severity and duration of BBB disruption varies across different pathological conditions and magnitude of trauma. Following injury, defects across the BBB can be large enough to allow entry of circulating serum proteins such as albumin (∼67 kDa).66 During traumatic brain injury, the BBB remains permeable to larger molecular weight proteins (>10 kDa) during the acute phase (<5 hours), while smaller molecules (0.3–10 kDa) may continue to transverse the BBB for several days post-injury.67 Enhanced BBB permeability is usually localized to the site of injury, allowing for the entry of larger therapeutics within an early time frame following the injury.67
The first peripheral immune cells to arrive at the site of injury are neutrophils, which arrive as early as 4 hours and peak 6 to 12 hours after injury.61 Neutrophils release TNF-α, ROS, antimicrobial peptides (AMPs), elastase, and neutrophil extracellular traps (NETs), which cause neuronal cytotoxicity and vascular leakage.61 NETs trap and kill bacteria, but also degrade BBB/BSCB tissue, further compromising its integrity and contributing to the infiltration of additional peripheral immune cells.68 Monocytes are the second and most abundant type of peripheral immune cells that arrive at the site of injury and are first detected within 24 hours after injury.69 Monocytes begin to differentiate at the site of injury into macrophages. Depending on their phenotype, macrophages can play a dual role in early inflammation. The accumulation of M1 macrophages around the lesion increases the production of iNOS, ROS, pro-inflammatory cytokines, and free radicals, resulting in increased neuronal apoptosis.61 Conversely, M2 macrophages phagocytose myelin debris, produce growth factors, and release anti-inflammatory cytokines. The timing of macrophage infiltration and the composition of their extracellular environment influence their differentiation to either an M1 or M2 phenotype.58,61 Microglia can similarly aid in resolving inflammation by expressing anti-inflammatory cytokines such as transforming growth factor-β1 (TGF-β1) and IL-10.58 Many researchers identify IL-10 as an anti-inflammatory cytokine that can halt the production of TNF-α and IL-6; however, IL-10 can also be considered as a pro-inflammatory cytokine that promotes the production of pro-inflammatory cytokines such as interferon-gamma (IFN-γ) from surrounding cells.70
Both astrocytes and microglia are activated within 24 hours after injury and are continuously activated in the first few days following injury. Activated microglia and macrophages are difficult to distinguish because they have similar phenotypes and functions. However, cell surface markers such as ionized calcium binding adaptor molecule 1 (Iba1+), CD206+, CD11b+, and CD45low/high can be used to distinguish microglia and macrophages; low expression of CD45 is indicative of microglia, while high expression of CD45 is indicative of macrophages.71,72 Both macrophages and microglia phagocytose cell debris, which is critical for maintaining tissue homeostasis and promoting tissue repair. Astrocytes and endothelial cells have also been reported to participate in phagocytosing cell debris after CNS injury. Myelin debris contains Nogo-A, myelin-associated glycoprotein (MAG), and ephrin B3, which can induce expression of TNF-α and IL-1β; thus, myelin clearance and subsequent removal of these signals is critical for the resolution of neuroinflammation. The prolonged presence of myelin debris can change the phenotype of macrophages to “foamy” or lipid-laden macrophages, leading to immune cell migration and decreased phagocytosis.61 A1 activated astrocytes are induced by inflammatory signals released from M1 activated microglia, such as TNF-α, IL-1β, and component 1 subcomponent q (C1q).73 The presence of A1 astrocytes can lead to further secretion of inflammatory signals, such as IL-1β, TNF-α, IL-6, ROS, and lipocalin-2.74,75 Conversely, A2 activated astrocytes can promote neuronal survival and tissue repair through the secretion of anti-inflammatory cytokines and neuroprotective growth factors, such as brain-derived neurotrophic factor (BDNF), glial-derived neurotrophic factor (GDNF), fibroblast growth factor-2 (FGF-2), and TGF-β.76–78
2.3.3 Sub-acute inflammation (days to weeks after injury).
The sub-acute phase of inflammation in the CNS occurs in the first few days to weeks following injury and, if left unchecked, can result in the extension of tissue damage beyond the initial injury. Expression of the pro-inflammatory cytokines IL-1β, TNF-α, and IL-6 begins to decrease between 2 to 7 days after injury while anti-inflammatory cytokines IL-10 and TGF-β begin to increase 3 to 7 days after injury.58 In some cases, levels of pro-inflammatory cytokines have been reported to return to baseline around 14 days after injury.58,65 Within 7 to 10 days after the initial injury, astrocytes proliferate quickly around the lesion to begin forming the glial scar. The glial scar separates the damaged tissue from healthy tissue and protects surrounding neurons from the spread of inflammatory signals. Formation of the glial scar is commonly detected by the presence of glial fibrillary acidic protein (GFAP), which is expressed by astrocytes.79 Additionally, chondroitin sulfate proteoglycans (CSPGs) are deposited in and around the injury site by activated astrocytes and function as a major growth-inhibitory component of the glial scar.80 While the glial scar protects neurons from the spread of inflammatory signals, it also prevents neurons from proliferating and extending axons through the injury site, resulting in limited tissue regeneration.79,81 Several cell types form the glial scar. This includes reactive astrocytes and microglia that make up the outer barrier, cells such as ependymal cells, fibroblasts, and pericytes line the inner edges of the scar and interact with reactive astrocytes, and macrophages, neutrophils, and stromal cells that fill the core.4,40,80,82
Neutrophils continue to be present within the injury site up to 2 to 3 days after injury, while the proliferation of other peripheral immune cells such as monocytes peaks slightly later, at 4 to 7 days after injury.69 Resident immune cell and astrocyte activation peaks 3 to 7 days after injury.61 The resolution of inflammation and polarization of macrophages and microglia to M2 phenotypes varies depending on the extent of initial tissue damage and subsequent ischemia. Microglia and macrophages can display both M1 and M2 phenotypic markers throughout the stages of inflammation. Interestingly, in a mouse TBI model, transient M2 microglia first peaked 7 days after injury before differentiating into an M1 phenotype, which peaked 21 to 28 days after injury.65,83 Microglia and astrocytes typically exhibit a low rate of proliferation in healthy tissue but have been reported to proliferate up to months after injury, with populations starting to gradually decline again approximately two weeks after injury.65,79,80
2.3.4 Chronic inflammation (months to years after injury).
A large percentage of individuals who experience a CNS injury develop chronic inflammation that can last several years after injury. In these cases, myelin debris clearance is insufficient after glial scar formation, resulting in the accumulation of “foamy” macrophages and decreased phagocytosis. The accumulation of myelin associated molecules such as Nogo-A and MAG results in neuron retraction and poor axonal regeneration in the tissue adjacent to the injury site. Astrocytes transition into scar-forming astrocytes, and DAMPs remain in the injury environment during the sub-acute and chronic inflammatory phases.4 The glial scar matures weeks to months after injury, during which tissue remodeling and restoration of the BBB/BSCB occurs. Elevated expression of a variety of pro-inflammatory cytokines have also been reported in the later stages of inflammation after TBI and SCI. Deposition of collagen I, fibronectin, and additional ECM components by pericytes, resident immune cells, and stromal cells results in the formation of dense fibrotic tissue that may not be functional.4 The chronic inflammation stage can last years after injury, and the overall mechanisms that result in the secondary progression of neurodegeneration are largely unknown. However, autopsies and positron emission tomography (PET) scans have been used to characterize the chronic inflammatory response in the brain and spinal cord for individuals who have lived years after severe CNS injury. For instance, activated microglia have been found in individuals affected by TBI multiple years after the initial injury.65,84 Additionally, SCI and TBI have been associated with progressive neurodegenerative diseases such as Wallerian degeneration and chronic traumatic inflammatory encephalopathy, respectively. TBI has also been linked to dementia, and many patients experience chronic inflammation in the brain after SCI, indicated by elevated TNF-α levels in the brain and accompanied by symptoms similar to TBI.65,84
2.3.5 Resolution of inflammation.
Although neuroinflammation may be viewed as a negative contributor to injury progression, it is essential for the repair of tissue. The orchestrated infiltration and activation of immune cells and their release of key cytokines are critical to resolving inflammation. The presence of cytokines such as IL-4 or IL-13 can polarize cells including resident glia and peripheral immune cells to their pro-regenerative phenotype. These cells clear cellular debris, protein aggregates, and toxic inflammatory molecules, which is a critical step in the resolution of inflammation. In ideal cases, the increased presence of these immune cells and the release of anti-inflammatory cytokines such as IL-10 and TGF-β can counteract pro-inflammatory molecules to resolve neuroinflammation. An increased presence of pro-regenerative immune cells is more likely to overcome the inflammatory milieu and return to homeostatic levels of immune cells and cytokines. However, in cases where neuroinflammation does not resolve itself, there is often a shift towards pro-inflammatory cells and molecules, resulting in chronic inflammation that is challenging to resolve. Furthermore, the formation of the glial scar and the production of inhibitory molecules such as CSPGs can inhibit the conversion of immune cells to their pro-regenerative phenotypes, preventing the resolution of inflammation.85
2.4 Neuroinflammation during disease
Aggregation of pathological proteins such as amyloid-β and α-synuclein, can promote microglial reactivity.86,87 Prolonged activity of reactive astrocytes and microglia has been identified as a hallmark of several neurodegenerative diseases, including stroke, multiple sclerosis, Alzheimer's disease, and Parkinson's disease.88 The BBB/BCSB prevents the delivery of numerous therapeutic drugs and biomolecules that could be valuable in treating these conditions. Biomaterials can provide a means to circumvent the BBB/BSCB and deliver therapeutics to the site of injury or disease to modulate neuroinflammation. The underlying mechanisms that are involved in each neuroinflammatory condition are summarized here to understand emerging targets for treating inflammation associated with these diseases.
2.4.1 Stroke.
Stroke is the third leading cause of death worldwide, with the majority of strokes caused by blood vessel occlusion and a subsequent ischemic event.89 Although stroke is commonly recognized as a disorder of the blood vessels, the interactions between vascular cells and neural cells, such as neurons and glia, are also an important consideration. Following stroke, activated astrocytes and microglia compromise the integrity of the BBB by producing inflammatory molecules such as DAMPs, ROS, iNOS, MMPs, and pro-inflammatory cytokines. These inflammatory molecules damage glia and endothelial cells, increasing BBB permeability.90,91 MMPs further disrupt the BBB by breaking down tight junction proteins within it. The BBB is a critical barrier that maintains homeostatic levels of immune cells within the CNS tissue, and its disruption facilitates an increase in peripheral immune cell infiltration.50,92 The extent of BBB damage depends on the severity of injury and magnitude of subsequent inflammatory events.93 Microglia are localized centrally within the stroke lesion, and astrocytes are located in and around the lesion.94 Like other cases of neuroinflammation, resident and peripheral immune cells can also resolve inflammation and promote tissue repair by phagocytosing debris, producing anti-inflammatory cytokines, and promoting angiogenesis.89 The severity of the stroke and the degree of BBB damage significantly contribute to the inflammatory response and secondary tissue damage after stroke. Restoration of the BBB allows for the reduction of infiltrating pro-inflammatory cells, creating a favorable environment for subsequent tissue repair.95 Therefore, a large focus of stroke treatments includes accelerating the restoration of the BBB and promoting the production of growth factors to repair damaged tissue.
2.4.2 Multiple sclerosis.
Multiple sclerosis (MS) is a chronic inflammatory disease that is characterized by demyelination, neurodegeneration, and gliosis in the CNS.96 Many researchers have considered MS to be an autoimmune disease in which immune cells infiltrate and attack healthy tissue, causing lesions; however, the exact mechanisms of MS remain unknown. The adaptive and innate immune systems synergistically contribute to chronic neuroinflammation in MS. The most common form of MS is relapsing-remitting multiple sclerosis (RRMS), in which inflammatory attacks on neural tissue continually recur. Macrophages typically reside within lesion sites long-term, while microglia populations only increase during the active phases of RRMS.97 Circulating monocytes infiltrate and differentiate into M1 or M2 macrophage phenotypes. Identification of macrophage phenotypes is valuable for understanding the mechanisms of MS since different phenotypes progress the disease differently.97,98 Monocytes release IL-6, IL-10, IL-12, and TNF-α with the highest levels of IL-6 and IL-12 expression found in untreated MS patients.97 M1 microglia and macrophages express pro-inflammatory cytokines and enhance the adaptive immune response by expressing major histocompatibility complex class II (MHCII), which is a requisite for antigen presentation, ultimately leading to a toxic inflammatory environment.99 Dying and demyelinated neurons produce large quantities of myelin debris in MS patients.100 Residual myelin debris increases inflammation and is commonly considered a hurdle in MS treatment. Shifting innate immune cells to their alternatively activated phenotypes to resolve inflammation and promote phagocytosis is necessary for the treatment of MS.
2.4.3 Glioblastoma.
Gliomas are solid mass tumors arising from the dysregulated activity of glial cells. Glioblastomas are a subtype of highly aggressive gliomas that account for approximately 50% of all malignant tumors in the CNS.101 The tumor microenvironment consists of an intricate mixture of brain tumor cells, tumor-associated macrophages (TAMs), astrocytes, neurons, T cells, NK cells, and neutrophils.102 Genetic alterations and metabolic adaptations of the cancer cells promote activation of TAMs, shifting them to an immunosuppressive M2 phenotype described as “pro-tumor.”103,104 The secretion of cytokines such as IL-6, IL-10, and TGF-β inhibits immune effector cells, upregulates immunosuppressive T regulatory cells, and promotes tumor vascularization.105–107 Ameliorating glioblastoma-associated immunosuppression is necessary for restoring the innate and adaptive immune response. Radiotherapy and chemotherapy are the standard treatment for glioblastoma, but tumor location and off-target side effects pose challenges to this approach.108,109 Thus, treatment strategies that promote pro-inflammatory, anti-cancer activity in tumor-associated immune cells are currently being explored as an alternative treatment strategy for glioblastoma.
2.4.4 Cerebral palsy.
Cerebral palsy is a clinically heterogenous collection of movement disorders arising from neurological dysfunction in the developing brain.110 Neuroinflammation has been identified as a major contributor to the progression of cerebral palsy. Trauma and/or infection within the uterine environment during fetal development results in a release of DAMPs and alarmins, activating innate immune cells through the “Fetal Inflammatory Response Syndrome.”111 Excessive inflammatory signaling within the CNS increases glial and immune cell proliferation, the release of pro-inflammatory cytokines (IL-1β, IL-6, TNF-α, IL-8), and subsequent cell death.112–114
2.4.5 Parkinson's disease.
Parkinson's disease is characterized by the degeneration of dopaminergic neurons and the appearance of motor abnormalities, such as tremors, bradykinesia, rigidity, and postural instability.115 M1 activated microglia play a significant role in Parkinson's disease by increasing the expression of pro-inflammatory cytokines including IL-6 and TNF-α, resulting in the death of dopaminergic neurons, prolonged inflammation, and further degeneration of neural tissue. Microglia have also been reported to release exosomes containing aggregated α-synuclein proteins, which act as a potent chemoattractant of additional immune cells and induce neuronal apoptosis.87 A1 activated astrocyte populations are also elevated in Parkinson's disease, further increasing expression of IL-6 and TNF-α, intercellular adhesion molecule 1 (ICAM1), and ROS in the disease environment. Given the key role of neuroinflammation in Parkinson's disease, therapeutics that reduce the expression of pro-inflammatory cytokines from activated glial cells and work to resolve inflammation are of particular interest.115
3. Biomaterials for modulating neuroinflammation
New insights into the underlying mechanisms of neuroinflammation have prompted the design of novel biomaterials to mitigate the detrimental effects of excessive inflammation and protect surrounding CNS tissue from secondary damage. Recent advances have been made in a variety of biomaterial strategies for modulating neuroinflammation, including nanoparticles with the ability to cross the selectively permeable BBB/BSCB,116–118 hydrogels that enable controlled delivery of immunomodulatory therapeutics,119,120 implantable scaffolds with biophysical cues to enhance cellular alignment and promote a growth-permissive environment for neural regeneration,121–125 and biomaterial coatings to prolong the lifetime of neural probe implants.32,126–128
3.1 Nanoparticles
The delivery of small molecules, proteins, lipids, and nucleic acids has been explored for modulating neuroinflammation. These therapeutic agents may be presented to a patient in either a powdered or liquid phase and may be administered through several routes (intravenous, inhalation, orally, etc.). Once absorbed, these agents pass into systemic circulation until they penetrate a tissue or are cleared by circulating phagocytes.129 The first challenge arises with determining the optimal dose of the drug. Due to short drug half-lives and rapid clearance by the reticuloendothelial system, systemic delivery of therapeutics often results in a low concentration of the drug reaching the target tissue. To overcome this challenge, higher therapeutic doses are typically used, but often result in cytotoxicity and off-target effects. For systemic delivery of therapeutics to the CNS, nanoparticles must bypass the BBB/BSCB before entering parenchymal tissue. This is considered a major obstacle in the field as the highly selective permeability of these barrier tissues makes entry of drugs very difficult. To address these concerns, nanoparticle-based delivery systems have been developed to extend drug half-life and improve their accumulation in target tissues. Nanoparticles can bypass the BBB/BSCB via transcytosis pathways and gaps in disrupted BBB/BSCB tissue (Fig. 3).130 Factors such as zeta potential, surface functionalization, and size determine the pathway of a nanoparticle to the CNS, and thus are important to consider when designing nanoscale delivery vehicles.131,132 Previous studies have shown that particles with a diameter less than 100 nm are more easily transported across the BBB via transcytosis pathways.133,134 Their nanoscale size (1–100 nm) also enhances tissue penetration as the extracellular space within the brain contains a large range of void spaces (40–700 nm) through which the nanoparticles must transverse before reaching the desired location.133,135,136 Similarly, microparticles have been used to deliver drugs systemically; however, the larger size of microparticles (2–2000 μm) can reduce their ability to cross the BBB and travel through neural tissue, given the size constraints of the extracellular space.137 Thus, nanoparticles will be our primary focus for systemic delivery of therapeutics to the CNS.
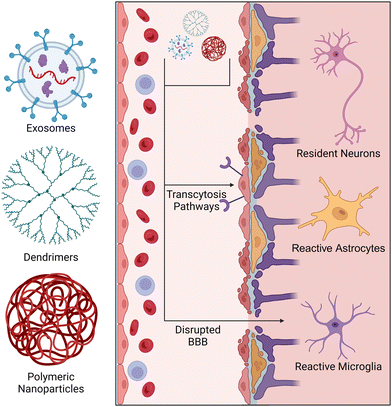 |
| Fig. 3 Nanoparticle-based delivery vehicles and their transport mechanisms through CNS tissue. Nanoparticle delivery vehicles such as exosomes, dendrimers, and polymeric nanoparticles enhance drug delivery across the blood-brain barrier. Nanoparticles may enter the CNS through gaps in the disrupted BBB or via “facilitated transport” pathways, such as carrier-, adsorptive-, and receptor-mediated transcytosis. | |
3.1.1 Exosomes.
Exosomes are nanoscale vesicles released from the outer membrane of cells that facilitate both paracrine and autocrine signaling.138 The payload within these vehicles consists of a diverse collection of proteins, lipids, and nucleic acids that can alter the recipient cell's behavior towards therapeutic or pathological outcomes.139,140 Exosomes have gained traction in recent years as a potential alternative to cell-based therapies as they make it possible to deliver bioactive molecules in a cell-free manner, bypassing concerns related to cell transplantation, including cell viability, potency, and tumorgenicity. Of equal interest are the membrane markers found on these extracellular vesicles. These surface receptors can facilitate vehicle homing to specific cells and tissues, while displaying low immunogenicity, making them ideal candidates for the targeted delivery of therapeutics.141
The general process for manufacturing exosomes consists of three steps: (1) culturing exosome-producing cells (days to weeks), (2) harvesting cell-conditioned media, and (3) several rounds filtration and centrifugation to separate exosomes from cells, debris, and microvesicles. Once isolated, exosome pellets can be reconstituted and stored for later use.123,142–144 The compositions of exosomes are tied to environmental stimuli affecting the secreting cell, which means that exosome composition and release can be primed for specific therapeutic purposes using a variety of conditions. Liu and colleagues demonstrated that culturing bone marrow-derived mesenchymal stem/stromal cells (MSCs) under hypoxic conditions prompted the increased release of exosomes containing a greater quantity of therapeutic microRNAs. Hypoxia-induced MSC-derived exosomes were found to be preferentially uptaken by microglia in vitro and shifted microglia polarization towards an M2 phenotype both in vitro and in a mouse SCI model.145 MSCs have also been stimulated with IFNγ to improve the immunomodulatory capabilities of their exosomes. Exosomes derived from IFNγ-stimulated MSCs have been shown to polarize microglia towards an Iba1+ CD206+ M2 phenotype and inhibit expression of proteins associated with pyroptosis, a highly inflammatory form of programmed cell death.140 IFNγ-stimulated MSC-derived exosomes were systemically delivered in an experimental autoimmune encephalomyelitis mouse model, which mimics the symptoms of MS. Delivered exosomes reduced infiltration of Iba1+ microglia and macrophages and shifted the cells to a branched morphology that is consistent with microglia in a resting state in healthy mice.146
While microglia and infiltrating macrophages have been the primary target for many treatment strategies that seek to ameliorate neuroinflammation, astrocytes have been increasingly implicated in the inflammatory response and have been the focus of several recent studies. MSC-derived exosomes delivered intraventricularly in mice reduced TNF-α and IL-1β expression in lipopolysaccharide-stimulated primary hippocampal astrocytes.143 Exosomes can also be derived from astrocytes themselves. For instance, astrocyte-derived exosomes were found to push microglia towards an M2 state by inhibiting the NF-κB signaling pathway.139 Combining strategies that target both reactive microglia and astrocytes simultaneously may synergistically work to resolve neuroinflammation by prompting the shift of multiple reactive glial cell types towards M2/A2 phenotypes.139
3.1.2 Dendrimers.
Dendrimers are nanoparticles with branched polymer chains that are radially oriented around a symmetric core. The branched structure of dendrimers results in the creation of internal cavities within the nanoparticles, allowing for non-covalent physical entrapment of hydrophobic drugs and their protection from the external environment until reaching their target destination.147–150 Dendrimers can also be functionalized with drugs,151–154 imaging agents,153,155,156 and targeting moieties153,157via covalent attachment, making them effective drug carriers that can be engineered to release their payload in response to target environment stimuli. Dendrimers can both extend drug bioavailability and facilitate the transport of cargo across the disrupted BBB.158 Generation-4 hydroxyl-terminated polyamidoamine (G4-OH-PAMAM) dendrimers have been studied for resolving neuroinflammation as they preferentially target activated microglia.159,160 Colocalization of OH-PAMAM dendrimers in reactive microglia is primarily mediated through endocytosis, pinocytosis, caveolae, and aquaporin channels.161 One such approach focused on inhibiting glutamate carboxypeptidase II (GCPII), a membrane-bound metallopeptidase that is a major contributor to increased extracellular glutamate. Upregulation of GCPII has been observed in activated microglia, contributing to glutamate-mediated excitotoxicity and glial cell dysfunction.162 GCPII inhibitors show potential for regulating neuroinflammation; however, poor bioavailability and tissue penetration hinder this strategy. To overcome this challenge, Zhang and colleagues conjugated 2-(3-Mercaptopropyl) pentanedioic acid, a GCPII inhibitor, to G4-OH-PAMAM dendrimers. They observed improved therapeutic delivery to damaged brain parenchymal tissue with the use of the dendrimers following intravenous delivery in a rabbit cerebral palsy model. Damage severity was positively correlated with greater therapeutic homing and glial cell uptake, resulting in enhanced TGF-β signaling and transition of activated microglia to a ramified state.118 Other GCPII inhibitors such as 2-(phosphonomethyl)-pentanedioic acid have also been conjugated to dendrimers and achieved similar results in MS and cerebral palsy models.117,163
Dendrimer platforms have been used for the delivery of anti-inflammatory drugs. Triamcinolone acetonide is an anti-inflammatory agent that has been delivered intravitreally for clinical treatment of diabetic retinopathy; however, this drug can be cytotoxic and expedite cataract formation.164 Conjugation of triamcinolone acetonide to G4-OH-PAMAM dendrimers resulted in its selective delivery to activated microglia (CD11b+, CD45low) in the eye and inhibited the NF-κB pathway.116 Similarly, G4-OH-PAMAM dendrimers improved the delivery of sinomenine to activated microglia in a rabbit TBI model.165 Significant changes were observed in the expression of TNF-α, IL-10, and IL-1β; however, sinomenine did not affect the secretion of IL-6, IL-4, and TGF-β1.
Dendrimers can also be engineered to induce anti-tumor behavior in TAMs. Triptolide is a STAT3 inhibitor with promising anti-cancer properties that are overshadowed by its poor aqueous solubility and potential for systemic toxicity. Conjugation of triptolide to G4-OH-PAMAM dendrimers increased its water solubility by 500-fold.166 Triptolide release was triggered in the glioblastoma microenvironment by the pH- and esterase-sensitive nature of the conjugating linker. This delivery platform not only facilitated targeted delivery of triptolide to TAMs, but also reduced overall off-target activity as indicated by lower levels of markers associated with hepatic toxicity.166
Dendrimer size was found to be a major factor contributing to how long the nanoparticles remained in circulation and distributed within tumor tissue.167 One group found that G6-OH-PAMAM dendrimers exhibited a 10-fold higher accumulation within tumor tissue after 24 hours and 2–3 fold higher tumor specificity compared to a generation-4 dendrimers. These nanoparticles were approximately 6.7 nm in diameter, which is small enough to penetrate the BBB around the tumor (<20 nm) yet large enough to avoid glomerular filtration and subsequent renal clearance (>6 nm).167 This was further explored by conjugating N-acetyl-L-cysteine to a G6-OH-PAMAM dendrimer.168N-Acetyl-L-cysteine is known for its anti-inflammatory and anti-oxidant properties, yet suffers from non-specific binding to circulating plasma proteins and low accumulation in the CNS. For these reasons, systemic delivery of N-acetyl-L-cysteine could greatly benefit from nanoparticle strategies. In this study, dendrimer-mediated delivery of N-acetyl-L-cysteine was localized to M1 microglia and promoted their return to a quiescent state. This decrease in the pro-inflammatory microglia population improved motor score and survival rate in a rabbit cerebral palsy model. Collectively, these studies highlight dendrimers as versatile platforms capable of enhancing the bioavailability of loaded drugs, diffusing across the extracellular space, and colocalizing with reactive glia.
3.1.3 Polymeric nanoparticles.
Polymeric nanoparticles can be fabricated from a wide variety of synthetic and natural polymer combinations, such as poly(ethylene glycol) (PEG), poly(lactic-co-glycolic acid) (PLGA), poly(caprolactone) (PCL), chitosan, and alginate.169–171 They can be tuned to degrade at different rates, modified with target-specific ligands,172 and made responsive to environmental stimuli, such as proteases, ROS, and pH.173–175 Polymeric nanoparticles typically possess a high drug loading capacity and can protect their cargo from the harsh environment of the body until it reaches the site of interest, both increasing drug bioavailability and reducing the dose required to make a drug effective. The ability of polymeric nanoparticles to shield their cargo makes them ideal candidates for transporting therapeutics across the BBB, motivating their growing application towards addressing neuroinflammation.
Nanoparticles that can cross the BBB and accumulate in astrocytes have been synthesized from a biodegradable block copolymer consisting of PLGA and PEG functionalized with a terminal lipophilic triphenylphosphonium cation.176 These nanoparticles were loaded with antioxidant and anti-inflammatory agents and then delivered intravenously, resulting in a decrease in ROS from reactive astrocytes in a rodent brain injury model. These same nanoparticles were applied in a different study to facilitate the delivery of antiretroviral therapeutics in HIV-infected mice.177 HIV infection can elicit a neuroinflammatory response from microglia and border-associated immune cells.178,179 Antiretroviral therapeutics are used to manage viral load, but have a limited ability to cross the BBB when administered freely.180 In this case, nanoparticle delivery facilitated antiretroviral therapy accumulation in the brain, leading to a decrease in p24, a distinctive HIV antigen. In a different study, epigallocatechin-3-gallate, a potent antioxidant,181–183 was delivered to a mouse epilepsy model using PEGylated PLGA nanoparticles.184 Release of the antioxidant agent was facilitated by nanoparticle degradation, while PEGylation masked the nanoparticles from the immune system, effectively increasing the half-life of the nanoparticles. Prolonged release of epigallocatechin-3-gallate from PEGylated-PLGA nanoparticles significantly decreased seizure rate and intensity in the mice. Furthermore, immunohistochemical results revealed lower activity by Iba1+ microglia and GFAP+ astrocytes. Polymeric encapsulation of antiepileptic therapeutics has also been reported using naturally-derived polymers such as chitosan.185
Polymeric nanoparticles can also be functionalized with targeting ligands. Amphiphilic macromolecule-based nanoparticles have been developed with a tunable binding affinity for CD36, a class B scavenger receptor that mediates microglial activation.186 Optimization of the alkyl side chain length in the polymer core increased binding of the nanoparticles to CD36, inhibiting microglial interactions with α-synuclein aggregates, which are known to propagate inflammatory signaling in Parkinson's disease.87,186 In another study, chitosan nanocarriers were modified with fucoidan,187 a sulfated amphiphilic polysaccharide that is known to target P-selectin,188 a cell adhesion molecule used to recruit macrophages to an inflammatory environment.189,190 Chitosan is particularly responsive to acidic environments, promoting accumulation of chitosan nanoparticles in acidic inflammatory tissue sites.186
Semiconducting polymer nanoparticles contain polymers with large π-conjugated rigid backbones. These conjugated polymers can efficiently produce heat or singlet oxygen when exposed to near-infrared light, allowing for activation of heat- and oxygen-responsive therapeutics.191,192 Semiconducting polymer nanoparticles can leverage the minimally invasive nature of near-infrared irradiation to achieve spatiotemporal control over the delivery and activation of therapeutics. For example, controlled release of the anti-inflammatory agent curcumin has been achieved using these nanoparticles, reducing ROS and intracellular calcium in mouse microglia while also inhibiting amyloid-β aggregation.193
3.2 Hydrogels
Hydrogels are water-swollen polymeric networks that are promising delivery vehicles for a variety of cells, proteins, and drugs. Hydrogels are preferred for soft tissue applications due to their intrinsically lower mechanical stiffnesses and can come in different sizes including bulk gels, microgels, and nanogels.194–196 Hydrogels can be surgically implanted, administered intravenously,194 intranasally,195,197–199 or injected locally to the site of injury.200–203 In the CNS, injectable hydrogels are preferable because they can be injected in a minimally invasive manner through the delicate BBB/BBSB with minimal damage, enabling the local delivery of high doses of immunomodulatory therapeutics. Naturally-derived polymers commonly used to fabricate hydrogels for the CNS include hyaluronic acid (HA), alginate, chitosan, gelatin, laminin, fibrin, elastin, and self-assembling peptides.200,204 Hydrogels can also be made of synthetic polymers such as PEG, PLGA, polyurethane, and poly(N-isopropylacrylamide) (PNIPAAm).200 A hydrogel that closely matches the mechanical and biochemical properties of the CNS can minimize glial scar formation and facilitate effective tissue repair. For instance, hydrogel stiffnesses should match that of brain and spinal cord tissue, reported to range from 100–2000 Pa.204,205 Furthermore, the addition of specific peptide sequences or nanoparticles that promote cell–material interactions and respond to pH, temperature, or electromagnetic stimuli can also be added to a variety of natural and synthetic hydrogels to increase their functionality in the CNS.200 Here, we will discuss the recent advances in hydrogels that have been used to deliver proteins, cytokines, and anti-inflammatory molecules to modulate the innate inflammatory response after injury or disease in the CNS.
HA is one the most common biopolymers that has been investigated for neural tissue engineering. HA is an abundant ECM molecule in the CNS produced by neurons and astrocytes.194 HA interacts with several cellular receptors, including the CD44 receptor, receptor for hyaluronan-mediated motility (RHAMM), and toll-like receptors (TLRs) 2 and 4 on neurons and glial cells to promote cell migration, proliferation, and differentiation.204,208–210 The effects of HA on the inflammatory response in the CNS are dependent on its molecular weight. High molecular weight HA (>1000 kDa) can reduce glial scar formation, hinder astrocyte activation, and decrease the production of pro-inflammatory cytokines, whereas low molecular weight HA (<500 kDa) can elicit pro-inflammatory astrocyte proliferation and cytokine production.208,211–213 HA is a particularly attractive biopolymer for hydrogels for CNS regeneration, as it can be easily modified to enable tunable crosslinking and incorporate peptides for cell adhesion and material degradation. HA can also be easily modified with different functional groups or peptides to produce hydrogels that can modulate inflammation while simultaneously promoting neuronal regeneration. HA-containing hydrogels have been used to deliver cells, neurotrophins, and other growth factors. Wang et al. developed an injectable, dual enzymatically crosslinked HA hydrogel to deliver nerve growth factor (NGF) and bone marrow-derived MSCs to a mouse model of TBI.214 HA was modified with tyramine to enable dual-enzymatic crosslinking with galactose oxidase and horseradish peroxide. MSCs and NGF were encapsulated within the hydrogel prior to crosslinking.215 The co-delivery of MSCs and NGF reduced IL-6 production, inhibited neuronal apoptosis, and polarized macrophages and microglia towards an alternatively-activated M2 phenotype. It has also been suggested that MSCs can promote neural regeneration by secreting cytokines and proteins that are associated with neural tissue repair.216
Hyaluronic acid-methylcellulose (HAMC) hydrogels are frequently used in neural tissue engineering applications for both cell and protein delivery.30,217–219 This blend of two polymers is physically crosslinked through hydrophobic interactions and is shear-thinning, which enables facile injection through a fine-gauge needle, and inverse thermal gelling, which enables gelation at body temperature.220 Recently, a HAMC hydrogel was modified with the peptide sequence KAFAK and the neurotrophic factor BDNF.30 KAFAK is an immunomodulatory peptide that can suppress the expression of the pro-inflammatory cytokines IL-6, IL-1β, and TNF-α.221 BDNF is a potent neurotrophic factor that can enhance the survival of neurons and axonal regeneration. The co-functionalized hydrogel reduced the expression of TNF-α, IL-1β, and IL-6 and promoted functional recovery after SCI (Fig. 4(A)). This hydrogel also increased the expression of IL-10, which can have anti-inflammatory effects. Interestingly, the HAMC hydrogel alone reduced levels of TNF-α, however less significantly, indicating that the hydrogel itself had an anti-inflammatory effect. One potential mechanism of this effect is that high molecular weight HA (>1000 kDa) can interact with the CD44 cellular receptor on resident and peripheral immune cells to decrease the expression of inflammatory cytokines, leading to a more favorable environment for tissue repair.208,210 In addition to regulating inflammatory cytokines, this hydrogel was capable of promoting neuronal survival, increasing axonal regeneration, and decreasing glial scar formation.
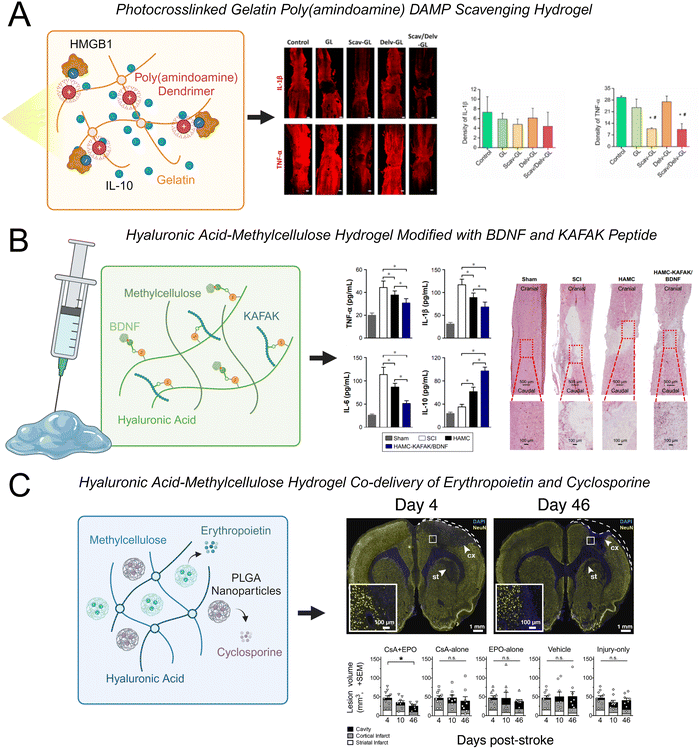 |
| Fig. 4 Examples of recent advances in hydrogels to modulate CNS inflammation. (A) A photo-crosslinked gelatin hydrogel modified with cationic charged poly(amindoamine) dendrimers simultaneously scavenged HMGB1 and released IL-10 to reduce expression of TNF-α and IL-1β in spinal cord tissue.206 (B) A hyaluronic acid-methylcellulose (HAMC) hydrogel modified with brain derived growth factor (BDNF) and the immunomodulatory peptide KAFAK reduced expression of TNF-α, IL-1β, and IL-6 and increased expression of IL-10 in spinal cord tissue.30 (C) A HAMC hydrogel loaded with poly(lactic-co-glycolic acid) (PLGA) nanoparticles for the co-delivery of erythropoietin and cyclosporine reduced lesion volume after stroke injury.207 | |
Other natural biopolymers including alginate, chitosan, gelatin, and combinations of such polymers have also been used to fabricate hydrogels for CNS applications. A fascinating approach is using hydrogels to sequester inflammatory molecules and subsequently halt the progression of downstream inflammatory responses. Cationic polymers such as PAMAM dendrimers can bind to negatively charged DAMPs, reducing the inflammatory response associated with their release and promoting the polarization of macrophages and microglia to an M2 phenotype. A photo-crosslinked gelatin hydrogel was modified with PAMAM dendrimers and loaded with IL-10.206 This hydrogel effectively sequestered high mobility group box 1 (HMGB1), which is an anionic charged DAMP that triggers TLR-4 activation and stimulates neuroinflammation and cell apoptosis through the NF-κB signaling pathway. The DAMP-scavenging/IL-10-releasing gelatin hydrogel reduced TNF-α and IL-1β levels and glial scar formation, while enhancing neural regeneration and functional recovery in a SCI model (Fig. 4(B)).
Hydrogels made from synthetic polymers such as PLGA, PNIPAAm, polyurethane, and PEG can also be used to treat inflammation in the CNS. A thermosensitive hydrogel made from a triblock copolymer (PLGA–PEG–PLGA) was used to deliver baricitinib, which is an FDA-approved inhibitor of the JAK2/STAT3 pathway that is clinically used to treat autoimmune diseases such as rheumatoid arthritis.222 The phosphorylation of JAK2 and STAT3 was significantly increased after SCI compared to uninjured tissue, motivating the use of this pathway as a therapeutic target.222 When delivered in a rat SCI model, the PLGA–PEG–PLGA hydrogel released 80% of the loaded baricitinib within 3 days after injury, during the acute inflammation stage, which is when JAK2/STAT3 expression peaks. Baricitinib successfully inhibited the JAK2/STAT3 signaling pathway in vivo, decreasing the levels of iNOS and TNF-α and reducing cell apoptosis. Follow-up in vitro studies suggested that baricitinib reduced SCI inflammation by inhibiting the classical activation of microglia.222 The JAK2/STAT3 pathway is suggested to be upstream of the mTOR signaling pathway involved in microglia and macrophage polarization, making it a promising approach for regulating immune cell polarization.
Hydrogels can also be loaded with peptides to enhance cell–material interactions, nanoparticles that can control the release of anti-inflammatory therapeutics, or nanoparticles that are responsive to specific stimuli. For instance, PLGA nanoparticles have been used for sustained release of anti-inflammatory drugs and growth factors218,223,224 and are regularly loaded into HAMC hydrogels for the sustained delivery of anti-inflammatory molecules for stroke treatment.207,224 A HAMC hydrogel loaded with PLGA nanoparticles was used for the co-delivery of cyclosporine and erythropoietin to modulate the inflammatory response after stroke.207 Cyclosporine is an immunosuppressant that is used to treat autoimmune diseases and has the ability to stimulate stem cells in the brain by creating a growth-permissive environment. Erythropoietin can promote neuronal regeneration and increase levels of circulating red blood cells. Cyclosporine and erythropoietin co-delivered within the acute phase of inflammation after stroke injury have been shown to synergistically enhance neuroprotection. Sustained co-delivery of cyclosporine and erythropoietin using a HAMC hydrogel containing PLGA nanoparticles successfully enhanced neuronal regeneration, increased neuronal plasticity, and decreased the size of the stroke lesion in the sub-acute phase of a rat ischemic stroke model (Fig. 4(C)). Thus, the delivery of drug-loaded nanoparticles within a HAMC hydrogel presents a viable platform for the sustained co-delivery of multiple therapeutics.
The same composite HAMC hydrogel containing PLGA nanoparticles has also been used to control the release of the neuroprotective peptide pituitary adenylate cyclase-activating poly peptide (PACAP) after stroke.224 PACAP has immunomodulatory effects and can increase expression of neurotrophins, promote neuronal survival, and polarize microglia towards an M2 phenotype. PLGA nanoparticles were terminated with a carboxylic acid to control their negative surface charge and promote binding to the positively charged PACAP peptide. Tuning the surface charge of the nanoparticles and the pH-responsive carboxylic acid ends on PLGA successfully controlled the release of PACAP. The effect of nanoparticle surface charge on PACAP bioactivity was evaluated by measuring the release of IL-6 from primary astrocytes in vitro. Expression of IL-6, downstream of PAC1 receptor activation, was inhibited by PACAP and was significantly reduced with PLGA nanoparticles carrying a −24 mV charge at day 10 compared to fresh PACAP. The PLGA-HAMC composite biomaterial successfully sustained the release of PACAP for 10 days in a rat stroke model, providing an avenue for tunable delivery of peptide and protein therapeutics for treating neuroinflammation.
When delivering therapeutics for clinical applications, it is important to consider how the delivery vehicle will affect the pharmacokinetics of the loaded therapeutic. Drug loading is subject to several factors such as drug solubility and size interactions with the delivery vehicle. Nanoparticles can extend drug bioavailability and transport therapeutics across the BBB, yet most nanoparticles suffer from low drug loading (∼10% of total vehicle mass).133,225,226 Renal clearance, circulating monocytes, and complement system protein interactions may limit the circulatory time of nanoparticles and effective therapeutic duration.227–230 Hydrogels typically have a greater capacity for loading hydrophilic drugs, because the drug can be absorbed into the water-swollen hydrogel network. Delivery of drugs to the target tissue will primarily be dictated by the size of the drug and the size of the hydrogel pores.231 Most small molecule drugs are much smaller than the pores of a hydrogel, resulting in rapid burst release and clearance from the target tissue.232,233 Furthermore, hydrogels have a limited ability to deliver hydrophobic drugs that are poorly soluble in aqueous media.234 Hydrogels are instead ideal for delivering proteins and cells, which can be better entrapped within the polymer network and released in a more sustained manner upon matrix degradation.27,29,235 The different benefits and drawbacks of nanoparticles and hydrogels has led to the use of combinations of these two delivery vehicles to leverage the advantages of both delivery platforms.224
Hydrogels have been fabricated with the addition of gold nanoparticles to achieve electroconductive characteristics. Specifically, hydrogels that use nanocrosslinkers, such as carbon-based nanomaterials or liposomes, to impart self-healing and responsive properties can be mixed with gold nanoparticles for regenerative medicine applications.220 A self-healing chitosan derivative hydrogel containing dialdehyde polyurethane nanocrosslinkers and gold nanoparticles was recently evaluated as a treatment for Parkinson's disease.221 This hydrogel reduced neurodegeneration and improved motor functional recovery in a rat model of Parkinson's disease. The polyurethane nanocrosslinkers and gold nanoparticles were suggested to be responsible for scavenging ROS, a key regulator of inflammation in Parkinson's disease.221 Polyurethane also exhibited immunomodulatory effects by shifting macrophages from an M1 to M2 phenotype. Altogether, hydrogels are a versatile platform to deliver drugs, cells, and proteins that have the potential to reduce neuroinflammation and improve functional recovery following CNS injury and disease.
3.3 Implantable scaffolds
Injuries to the CNS such as stroke, TBI, and SCI can result in the loss of large volumes of functional tissue. In these cases, bulk implantable scaffolds can be advantageous due to their ability to fill cavities formed after injury, provide mechanical support, and guide cell migration and elongation of axons through their pores during neural regeneration. Scaffolds that have mechanical, biophysical, and biochemical characteristics similar to CNS tissue can provide a habitable environment for both transplanted and host cells. Furthermore, scaffolds with electroconductive properties can promote proliferation, differentiation, and regeneration of neuronal cells by transmitting electrical signals to surrounding cells in the brain and spinal cord.236 In the spinal cord, implantable scaffolds that can provide biophysical cues that guide anisotropic elongation and alignment of axons through the lesion are especially attractive, as they increase the likelihood of promoting robust new synaptic formations and functional recovery.
Implantable scaffolds are produced through numerous methods including templated scaffolds fabricated by casting or injection molding, cryogenic processes such as freeze-drying or ice-templating,34,237,238 additive manufacturing techniques that extrude fibrous polymers or viscoelastic hydrogels,239,240 microfluidic devices that can deposit polymeric materials in a precise structure,241,242 or by the decellularization of tissues.243,244 Depending on the method used for producing these scaffolds, scaffolds can exhibit a fibrous, multichannel, or microporous structure that allows for the support of a neural network.245 Additive manufacturing or deposition of polymeric fibers on a nano- or micro- level can be used to create scaffolds that can have controlled porosity and orientation for guiding cellular alignment. Bioprinting viscoelastic hydrogels provides a convenient method for loading exosomes, cells, nanoparticles, and proteins for implantation directly into the lesion. Freeze-drying polymers can enhance scaffold mechanical strength and provide tunable porosities with appropriate biophysical cues for cell infiltration from surrounding CNS tissue. Implantable scaffolds can be made from a variety of polymers that are commonly used to make hydrogels and can also extend to water-insoluble polymers such as silk fibrin, PCL, and PLGA.
Scaffolds implanted in the spinal cord lesion after injury are commonly used to guide axonal regeneration, bridge synaptic formations, and promote functional recovery. Anisotropic channels can provide biophysical cues to facilitate the migration of transplanted and endogenous cells and elongation of axons through the spinal cord lesion. Aligned collagen scaffolds are popularly used to achieve this goal. Recently, Zou et al. used an aligned collagen sponge to promote differentiation and neurite outgrowth of human fetal brain neural stem cells (NSCs) and fetal spinal cord NSCs for the treatment of SCI (Fig. 5(A)).121 Both spinal cord and brain NSCs delivered in the collagen scaffolds reduced pro-inflammatory IL-1β and IL-6 secretion and expression of CD68, a cell surface marker for activated microglia/macrophages. However, IL-1β expression levels were significantly lower in the collagen scaffold containing spinal cord-derived NSCs. Furthermore, the collagen scaffold containing spinal cord-derived NSCs induced neurite outgrowth, increased the number of mature neurons, and promoted axonal myelination and synapse formation, demonstrating that spinal cord-derived NSCs were overall more effective than brain-derived NSCs for reducing inflammation and promoting neuronal regeneration after SCI.
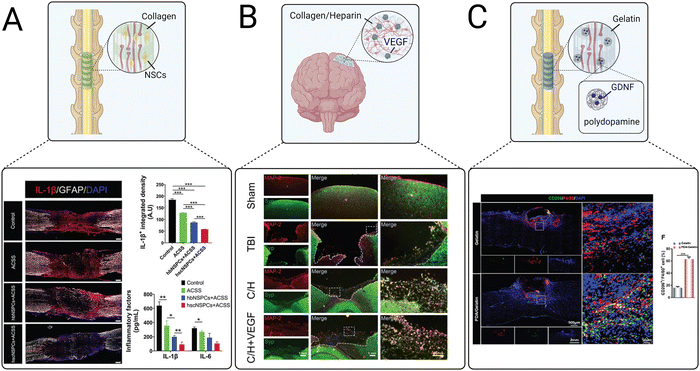 |
| Fig. 5 Examples of recent advances in implantable scaffolds to modulate CNS inflammation. (A) An aligned collagen scaffold loaded with neural stem cells (NSCs) decreased the expression of pro-inflammatory cytokines including IL-1β and IL-6.121 (B) A collagen/heparin scaffold for the repair of brain tissue after TBI promoted synaptic formations as indicated by staining of microtubule associated protein 2 (MAP-2) and synaptophysin (Syn).246 (C) An aligned gelatin scaffold containing glial cell line-derived neurotrophic factor (GDNF)-loaded polydopamine nanoparticles polarized macrophage and microglia to M2 phenotypes after SCI as indicated by CD206 and F40/F80 staining.247 | |
Implantable scaffolds fabricated using synthetic polymers present opportunities for investigating the use of cell–material interactions for modulating inflammation, as functional groups can easily be modified in polymer synthesis to tune material properties. The degradation of PLGA produces an acidic environment which increases inflammation; thus, modifying the degradation rate and products of PLGA could modulate the immune response.248 This idea motivated the evaluation of increasing PLGA content and varying lactic acid and glycolic acid ratios within a polyurethane scaffold with varying soft and hard segments that contain PEG, PCL, PLGA, L-lysine, ethyl ester diisocyanate, and 1,3-propanediol to further adjust the degradation rate of the scaffold, the acidity of its degradation products, and the subsequent immune response.249–251 The synthetic water-soluble polymer was fabricated by waterborne polyurethane emulsion, crosslinked using UV light, and freeze-dried. Increasing PLGA content of bulk scaffolds resulted in largely anti-inflammatory effects, including reduced levels of nitric oxide, decreased expression of TNF-α, and increased levels of IL-10 in vitro. PLGA containing equal amounts of lactic acid and glycolic acid resulted in the highest expression of anti-inflammatory cytokines. These results also demonstrated the potential of PLGA to polarize microglia to an M2 phenotype.248 This phenomenon was suggested to be caused by immunomodulatory effects of PLGA in addition to the scaffold's mechanical stiffness and degradation rate, further supporting the use of PLGA for implantable scaffolds.
Exosome-loaded 3D-printed scaffolds offer mechanical support while delivering biochemical cues to modulate neuroinflammation and promote tissue regeneration. A 3D-printed collagen/silk fibroin scaffold loaded with exosomes derived from MSCs pretreated with FGF-2 was used to treat TBI in a canine model.122 Both FGF-2-pretreated and untreated exosomes reduced lesion size, apoptosis of neurons, and glial scar formation, while also promoting neuronal regeneration, myelination, synapse formation, and angiogenesis. The least neuronal apoptosis was observed with FGF-2-pretreated exosomes. Exosome delivery also reduced IL-6 and TNF-α expression and increased IL-10 expression. Notably, the same research group has evaluated several variations of stimulated stromal cells and exosomes delivered via 3D printed scaffolds. Hypoxic-preconditioned MSCs have been delivered with the same collagen/silk scaffold, while collagen/chitosan 3D-printed scaffolds have been used for the delivery of exosomes derived from insulin-like growth factor (IGF)-1-pretreated NSCs and BDNF-stimulated MSCs for TBI treatment.124,125,252,253 It will be interesting to compare the overall effectiveness of using exosomes from stimulated MSCs, NSCs, and stromal cells as immunomodulatory treatments in the CNS over the coming years.
Implantable scaffolds have also been used as delivery vehicles for proteins. A freeze-dried collagen/heparin scaffold loaded with vascular endothelial growth factor (VEGF) was delivered to a rat model of TBI (Fig. 5(B)).246 This scaffold had been previously evaluated for the treatment of SCI and resulted in significant functional recovery, motivating its use for treating other CNS injuries. The collagen/heparin scaffold provided mechanical support for cells infiltrating from the surrounding brain tissue and successfully sustained the release of VEGF over 27 days. The VEGF-loaded collagen/heparin scaffold promoted angiogenesis, improved neuronal survival, relieved edema, and reduced lesion size in a rat TBI model. Interestingly, scaffolds with and without VEGF both significantly decreased levels of the inflammatory cytokines IL-1β and IL-6. The success of this scaffold in both the spinal cord and the brain demonstrates its versatility for treating multiple types of CNS injuries.
Combination strategies of multiple types of biomaterials have also been explored. Ma et al. evaluated a multi-component gelatin scaffold loaded with polydopamine nanoparticles that released GDNF for the treatment of SCI (Fig. 5(C)).247 Polydopamine is used in many regenerative medicine applications for its ability to scavenge ROS, reduce inflammation, and polarize macrophages and microglia towards an M2 phenotype.254–256 The gelatin scaffold reduced ROS and promoted M2 microglia polarization, as demonstrated by the downregulation of iNOS and increased expression of CD206 in vitro. When implanted in a mouse model of SCI, M2 polarization of microglia was significantly increased around the SCI lesion, TNF-α and IL-1β levels were decreased, and IL-10 and TGF-β levels were increased, suggesting an overall positive effect of the scaffold on resolving inflammation. Furthermore, the encapsulation of GDNF into the polydopamine nanoparticles reduced neuronal apoptosis and scar formation and promoted neuronal survival and axonal regeneration. Staining for vGluT1, a synaptic maker, revealed the successful formation of new synapses. Overall, the scaffold provided dynamic biochemical and biophysical cues to successfully promote M2 polarization, modulate cytokine expression, and promote synapse formation, confirming the utility of combinatorial therapeutic strategies to simultaneously modulate the inflammatory response and provide neurotrophic support.
3.4 Neural probe coatings
In addition to promoting repair after tissue injury and disease, biomaterials can also aid in the integration of implants for the CNS. Neural probes interface with neural tissue to stimulate and/or record electrical activity on the single-neuron level through changes in the membrane potentials of surrounding neurons. The use of neural probes has enhanced our understanding of neural connectivity, enabled the development of neuroprosthetics, and advanced treatment of neurological disorders. To measure neural activity with high spatiotemporal resolution, probes are typically inserted directly into parenchymal tissue, compromising the BBB.257–259 The resulting tissue damage leads to the activation of local glia and initiates a foreign body response.260,261 Additionally, the brain goes through a range of micromotions during daily activity, which induces mechanical stresses between the implant and surrounding tissue, resulting in further tissue damage and inflammation.262,263 Ultimately, probes intended to directly interface with neural tissue are slowly segregated from the CNS as glial scar tissue encapsulates the electrode shank.264 Thus, regulating neuroinflammatory signaling and glial reactivity to the implant is critical for ensuring the long-term reliability of implanted probes. Two approaches exist in choosing materials for the fabrication of neural probes and their coatings. Materials that support protein and cell adhesion may be used to promote the growth of neurons in direct contact with the neural probes to improve signal detection, or nonfouling materials that resist protein and cell adhesion may be used to try to minimize the foreign body response and shield the probes from damage. Both approaches aim to minimize glial scar formation and maximize the long-term functionality of the neural probes.
Graphene is a popular material for neural probe fabrication as its mechanical flexibility, chemical inertness, and high electrical conductivity allow it to effectively interface with neural tissue (Fig. 6(A)). Graphene can encourage neuronal adhesion, axonal sprouting, and neural stem cell differentiation.268,269 Graphene outperforms other materials typically used in electrode interfaces, including parylene-C, polyimide, and diamond, because it results in reduced glial scar formation and limits the retraction of neurons from the area immediately surrounding the implant site. The tissue adjacent to graphene neural probes typically contains higher neuron density, average quantity and length of neurites, and total neural outgrowth than neural probes made from other materials.32 Application of graphene to silicon probes resulted in a significant decrease of glial cell proliferation, accompanied by a greater number of neural soma and processes around the implant compared to uncoated devices.32 Despite these advantages, previous studies have raised concerns over graphene's suitability for in vivo applications given its susceptibility to enzymatic oxidation by phagocyte-secreted peroxidases.270–272 This mechanism for degradation poses a challenge for graphene-coated probes, as infiltrating immune cells will cause surface stripping and eventual failure of the device. To prevent potential device failure and particle bioaccumulation, strategies to protect the graphene coating layer of neural probes from degradation will be critical.
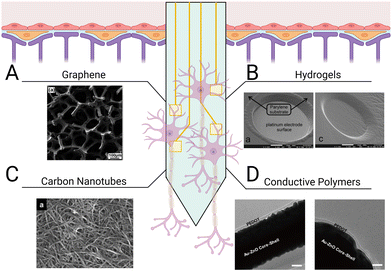 |
| Fig. 6 Different types of coatings for improving neural probe integration. Electrode shanks may be coated with (A) graphene monolayers to support neuronal attachment,265 (B) hydrogels with tunable mechanical properties and conjugated ligands for mimicking CNS parenchymal tissue,33 (C) carbon nanotubes for lower electrode impedance and increased charge transfer,266 and (D) conductive polymers, such as PEDOT, to enhance communication across the tissue–electrode interface.267 | |
HA thin films for neural probe coatings are of particular interest, because HA is a primary component of CNS parenchymal ECM.208 Combining HA thin films with poly-L-lysine (PLL), a positively charged amino acid polymer, improves neural adhesion via electrostatic interactions with the negatively charged cell membrane.273–275 This thin film composite has been used to coat intracortical electrodes. Following 7 weeks of in vivo recording, histological staining revealed a slight decrease in the number of Iba-1+ microglia and GFAP+ astrocytes around the implant site.126 When deposited on graphene monolayers, the HA/PLL coating improved neuronal adhesion and outgrowth in vitro over 14 days. The physical characteristics of the HA/PLL coating on graphene monolayers were also investigated using Raman spectroscopy and atomic force microscopy. The HA/PLL coating was found to adhere to the graphene monolayer without significantly affecting its crystalline quality. Together, this work demonstrates the potential of HA/PLL coatings to facilitate neural probe integration; however, whether HA/PLL coatings can protect graphene from enzymatic oxidation remains to be explored.
Hydrogels are promising materials for coating neural probes because they are cytocompatible, display tunable mechanical properties, and can be easily functionalized with stimuli-responsive crosslinkers or cell-adhesive peptides to enhance probe integration (Fig. 6(B)). PEG hydrogels are commonly used to shield implants from detection by the immune system due to their resistance to protein and cell adhesion.276 Spencer et al. used PEG-dimethacrylate hydrogel coatings to reduce micromotion-induced stress within implant sites in rats.277 They evaluated the effect of shank size on the immune response by implanting glass capillaries of varying sizes (150, 200, and 400 μm diameter) and found that increasing the diameter of the shanks increased recruitment of reactive astrocytes and macrophages, increased BBB permeability, and decreased neuronal density within a 100 μm radius of the implant in a rodent brain. Uncoated 150 μm diameter capillaries were then compared to capillaries coated with varying thicknesses (150, 200, 400 μm) of 10% w/v 8000 kDa PEG-dimethacrylate. Hydrogel coatings reduced strain in vivo following perpendicular and axial probe displacement, subsequently decreasing trauma at the implant site. Furthermore, reduced GFAP staining confirmed hydrogel coatings ameliorated astrocyte reactivity 8 weeks post implantation. However, swelling of the PEG-dimethacrylate in vivo also resulted in a larger implant diameter, increasing BBB permeability, macrophage recruitment, and neuron retreat from the site. While the soft interface of the shanks protected the site from micromotions, reduced neuron density over a 100 μm radius could hinder device efficacy. In another study, a different approach was used, in which a dual-layered microfluidic system consisting of a gelatin methacrylate-PEG hydrogel microchannel encased by a thin, porous polydimethylsiloxane membrane was implanted.278 The dual-layered system was attached to a metal probe while dry so that it was stable enough to allow implantation without delamination of the coating. Once inserted, the composite hydrogel swelled, releasing encapsulated IL-4 and dexamethasone, which increased M2 macrophages and decreased TNF-α and IL-6 secretion. Platforms such as these present opportunities to explore co-delivery of anti-inflammatory cytokines (IL-13, IL-4), inflammatory cytokine receptor antagonists (IL-1 receptor antagonist), and neurotrophic factors to promote neuronal survival while simultaneously regulating the glial response.
While hydrogel coatings are typically not electroconductive and do not contribute to neuron-electrode communication, hydrogels that contain conductive polymers present the opportunity to improve neural signal detection while also maintaining the advantages of a mechanically and biochemically tunable coating. Conductive polymer hydrogels can promote neuron differentiation, reduce astrocyte reactivity, and are generally cytocompatible.279,280 Conductive hydrogels may also help enhance several electrode properties such as electrical conductivity (the ability to conduct current in a given solution), impedance (resistance of the electrode to alternating current, in which low impedance is associated with greater signal quality), cut-off frequency (frequency limit from which signal noise may be removed), and charge storage capacity (the capacity of the device for storing electricity). Cui et al. designed a transparent, stretchable hydrogel electrode consisting of PEGylated silk fibroin and the conductive polymer poly(3,4-ethylenedioxythiophene):polystyrene sulfonate (PEDOT:SS).127 Silk fibroin was first crosslinked using PEG diglycidyl ether, enabling control over the mechanical properties and degradation kinetics of the hydrogel. PEGylated silk fibroin was poured over a thin film of PEDOT:SS prior to the implant interfacing with rat neurovasculature. This conductive polymer hydrogel could both record and stimulate neural activity across the rat cortex. Similarly, PEDOT:SS was used as a conducting polymer within the synthetic copolymer hydrogel, P(DMAA-co-5%MABP-co-2,5%SSNa), to enhance the functionality of iridium oxide electrodes.128 Dimethylacrylamide (DMAA) functions as the polymer backbone of the hydrogel while sodium 4-styrenesulfonate (SSNa) acts as an anion for PEDOT deposition. Methacryloyloxy benzophenone (MABP) is an ultraviolet light-reactive molecule that can form covalent attachments with 4-(3-triethoxysilyl)propoxybenzophenone silane. Photolithographic patterning methods were used to control the covalent attachment of the conductive polymer hydrogel to the electrode surface. Covalent attachment of the conductive polymer hydrogel to iridium oxide electrodes improved charge storage capacity by 2.5-fold, lowered cut-off frequency, and reduced signal impedance. When this material was incubated with the neuroblastoma cell-line SH-SY5Y, no cytotoxic effects or significant changes in material stability were observed. In another study, PEDOT was used to coat a gold-coated, ZnO nanowire microelectrode array (Fig. 6(D)).267 ZnO nanowires can help lower the impedance values of the device by increasing the overall effective electrode surface area. The nanowires themselves are flexible, yet ZnO has poor conductivity. Coating the nanowires with gold can improve conductivity, while supplementing with PEDOT can provide a biologically active interface with the neural tissue. Taken together, these studies demonstrate that conductive polymer hydrogels are a promising class of materials for facilitating tissue–probe integration and enhancing signal sensitivity.
The rigidity of neural probes is a double-edged sword. On one hand, shank stiffness greater than that of neural tissue is necessary for proper insertion. On the other hand, the mechanical mismatch between the probes and the tissue can lead to secondary trauma from the micromotions of the device. Carbon nanotubes are suitable for neural probe fabrication as they are highly elastic yet mechanically strong (Fig. 6(C)). The structure of carbon nanotubes prevents the release of toxic degradation products, and their innate electrical conductivity allows them to replace commonly used metals as electrodes. When compared to a platinum electrode array, single-walled carbon nanotube electrode arrays did not exhibit appreciable damage and displayed significantly less impedance following 2 months of implantation in rat cortex.281 Furthermore, long-term implantation of carbon nanotubes did not significantly increase the presence of reactive microglia or astrocytes or change neuron density. In a different application, carbon nanotube fibers were used as a soft cortical electrode compatible with magnetic resonance imaging (MRI) techniques.282 The carbon nanotube fiber microelectrodes displayed a 9 times lower impedance than the platinum/iridium electrodes used in the study. Two hours post-implantation, the carbon nanotube fiber electrodes recorded precise, single-unit spike activity with a high signal-to-noise ratio. In addition to not interfering with MRI, the carbon nanotube fibers reduced both GFAP and Iba-1 signal around the implant site and maintained higher neuronal density than the platinum/iridium probes. In contrast, the neuron loss zone around the platinum/iridium probes continued to increase in size, indicating progressive neurodegeneration. Carbon nanotube fiber electrodes continued to detect single-neuron recordings for up to 4–5 months, suggesting stable long-term reliability.
4. Future outlook
The successful application of hydrogels in the CNS for the modulation of neuroinflammation has prompted their advancement to clinical trials. Recently, a self-assembled peptide hydrogel based on the short peptide RADA16, which contains four repeating units of the “RADA” peptide sequence, advanced to clinical trials for the treatment of SCI with an expected completion date in 2025.283 This hydrogel has demonstrated a promising ability to support stem cell viability and promote neural regeneration after SCI in animal models and is currently being investigated in clinical trials for the delivery of a heterogeneous collection of cells from the stromal vascular fraction of adipose tissue.283,284 Additionally, intranasal administration of APH-1105 nanoparticles is currently in clinical trials for the treatment of Alzheimer's disease and is expected to be completed in 2024.285 APH-1105 is a modulator of the enzyme α-secretase, which cleaves pathological amyloid precursor proteins to a soluble product that is easily cleared from the brain and avoids accumulation of amyloid plaques.199 Intranasal administration of nanoparticles bypasses the BBB and enables transport directly to the brain; this strategy has also been used to deliver other therapeutics such as rivastigmine and insulin.
Several recent advances have been made in hydrogel research that we expect will translate into valuable therapeutic strategies for managing neuroinflammation in the coming years. Hydrogels that can scavenge pro-inflammatory molecules are emerging as a novel strategy to reduce inflammation in many tissues. The addition of a variety of functional groups, peptides, and charged polymers to hydrogels can confer the ability to scavenge and remove pro-inflammatory molecules. For example, material modifications using gallic acid, polyurethane, PAMAM dendrimers, and polyethylenimine have been recently used to scavenge pro-inflammatory molecules, such as ROS and DAMPs.119,206,286 Nanocrosslinked hydrogels, which are made from nanoclays, nanoparticles, or liposomes, are a novel class of hydrogels that combine the injectability and self-healing properties of dynamic covalent crosslinked hydrogels with the versatility of nanoscale materials. By changing the crosslinkers employed, these hydrogels can be engineered to degrade in response to a variety of stimuli, including light, heat, pH, and electromagnetism. Some recent examples of this class of materials include dialdehyde polyurethane nanocrosslinkers and graphene oxide, which has been used to modulate microglia polarization and promote angiogenesis and tissue regeneration after SCI.287
Emerging developments to enhance temporal control over biomaterial structure and the delivery of proteins and other biomolecules may also have applications in mitigating neuroinflammation, which is an innately time-dependent process. Photodynamic hydrogels, which use light to trigger changes in material structure or the release of cargo, present a versatile approach to enable spatiotemporal control over biomaterial behavior. Current methods for fabricating photodynamic hydrogels are limited to the use of high-energy ultraviolet light (<400 nm), which exhibits low tissue penetration.288–291 A recent investigation by Rapp and DeForest reports the use of three photolabile crosslinkers, expanding the compatibility of photocleavable crosslinkers to a greater spectrum of visible and infrared light.289 PEG-based hydrogels showcased rapid, orthogonal degradation in response to red (617 nm), green (530 nm), and blue (405 nm) light. The multiplexed degradation of hydrogel constructs creates new opportunities for triggered, spatiotemporal release of multiple bioactive agents from a single hydrogel. This approach could also potentially be reversed for finer control in biofabrication processes, paving the way for light-mediated gelation of deep tissue implants or gradient patterning of cell-supporting peptides or conjugated therapeutics, as well as differential photocrosslinking of various polymers into a single platform. As the selection of wavelength-selective crosslinkers grows, we can expect to see more complex hydrogel-based platforms for studying pathophysiological phenomena and controlling the release of bioactive agents. This approach may have utility in SCI specifically, in which the staggered delivery of multiple therapeutics has been shown to improve tissue repair and functional recovery.292
Novel strategies are also being employed to improve the ability of implantable scaffolds to align axons within damaged neural tissue, including the implantation of prevascularized and conductive scaffolds. Aligned, prevascularized scaffolds can recruit neural cells to the site of injury and promote vascular infiltration, aiding in tissue regeneration.293–295 Additionally, several aligned collagen sponge scaffolds are now making their way into clinical trials for axonal alignment and spinal cord tissue repair after SCI. These include a study completed in 2021 that evaluated an aligned collagen sponge scaffold for the treatment of acute SCI, a study that assessed the effectiveness of an aligned collagen sponge combined with epidural electrical stimulation, and a third study that evaluated the NeuroRegen Scaffold™ loaded with bone marrow mononuclear cells compared to traditional intradural decompression and adhesiolysis treatment.296–298 Lastly, an aligned scaffold fabricated from PLGA-b-PLL called Neuro-Spinal Scaffold™ is currently in clinical trials for treating anatomically complete SCI with an expected completion date of 2028.299
Enhancing the tissue-electrode interface and mitigating the foreign body response to neural probes is a major priority in the neuroelectronic field. Biohybrid neural interfaces, also known as “living electrodes,” are an emerging concept in which neural probes contain engineered networks of neurons or materials that encourage host neurons to grow into the implant to facilitate a seamless tissue–electrode interface and improve signal recording. A study by Cullen et al. reported the design of hydrogel microconduits for supporting neuron outgrowth and unidirectional axon elongation.300 While the motivation of the work was originally to reconnect damaged neural tracts, these microtissue constructs could also be used to stimulate target neurons. This proof-of-concept was later reported by Adewole et al., in a study where engineered neuronal networks were implanted into the male rat cortex.301 The self-contained nature of these living electrodes facilitated synaptogenesis with target neurons, as indicated by sustained neuronal activity and signal detection over a 5-week period. This work suggests that living electrodes could be used to overcome the challenge of diminishing functionality observed with inorganic neural probes; however, longitudinal studies are still needed to confirm long-term construct integration.
5. Conclusion
A dysregulated neuroinflammatory response is a hallmark of traumatic injury and several neurodegenerative diseases. While injury and disease onset in the CNS may result from a myriad of causes, unresolved neuroinflammation from any of these causes can result in secondary injury, worsening tissue damage. Biomaterials are emerging as a promising therapeutic approach for resolving dysregulated inflammatory responses. Ultimately, we expect that the recent advances in a variety of biomaterials described within this review will lead to viable strategies for treating injury and disease in the CNS. As the population affected by CNS injury and disease grows in the future, it will be critical to continue expanding our understanding of the pathological mechanisms of neuroinflammation to be able to use these new insights to design better biomaterials for regenerative medicine and neuroengineering applications in the CNS.
Conflicts of interest
There are no conflicts to declare.
Acknowledgements
We are grateful for funding from the National Institutes of Health (R21-EB032112) and the National Science Foundation CAREER award (2237240). Figures were created with BioRender.com.
References
- D. Sandean, Management of acute spinal cord injury: A summary of the evidence pertaining to the acute management, operative and non-operative management, World J. Orthop., 2020, 11(12), 573–583 CrossRef PubMed.
- Report to Congress: Traumatic Brain Injury in the United States | Concussion|Traumatic Brain Injury | CDC Injury Center [Internet]. 2019 [cited 2023 Sep 10]. Available from: https://www.cdc.gov/traumaticbraininjury/pubs/tbi_report_to_congress.html.
- TBI Data | Concussion | Traumatic Brain Injury | CDC Injury Center [Internet]. 2023 [cited 2023 Sep 10]. Available from: https://www.cdc.gov/traumaticbraininjury/data/index.html.
- E. J. Bradbury and E. R. Burnside, Moving beyond the glial scar for spinal cord repair, Nat. Commun., 2019, 10(1), 3879 CrossRef PubMed.
-
K. E. Thorpe, A. I. Levey and J. U. S. Thomas BURDEN OF Neurodegenerative Disease. 2021. Available from: https://www.fightchronicdisease.org/sites/default/files/May%202021%20Neurodegenerative%20Disease%20Burden%20on%20US%20-%20FINAL%20.pdf.
- J. Van Schependom and M. D’haeseleer, Advances in Neurodegenerative Diseases, J. Clin. Med., 2023, 12(5), 1709 CrossRef PubMed.
- J. C. Zheng and S. Chen, Translational Neurodegeneration in the era of fast growing international brain research, Transl. Neurodegener., 2022, 11(1), 1, DOI:10.1186/s40035-021-00276-9.
- Y. Hou, X. Dan, M. Babbar, Y. Wei, S. G. Hasselbalch and D. L. Croteau,
et al., Ageing as a risk factor for neurodegenerative disease, Nat. Rev. Neurol., 2019, 15(10), 565–581 CrossRef PubMed.
- G. Margetts, S. Kleidonas, N. S. Zaibi, M. S. Zaibi and K. D. Edwards, Evidence for anti-inflammatory effects and modulation of neurotransmitter metabolism by Salvia officinalis L, BMC Complement Med Ther., 2022, 22, 131 CrossRef CAS PubMed.
- J. E. Park, Y. H. Leem, J. S. Park, D. Y. Kim, J. L. Kang and H. S. Kim, Anti-Inflammatory and Neuroprotective Mechanisms of GTS-21, an α7 Nicotinic Acetylcholine Receptor Agonist, in Neuroinflammation and Parkinson's Disease Mouse Models, Int. J. Mol. Sci., 2022, 23(8), 4420 CrossRef CAS PubMed.
- S. Rom, V. Zuluaga-Ramirez, N. L. Reichenbach, M. A. Erickson, M. Winfield and S. Gajghate,
et al., Secoisolariciresinol diglucoside is a blood-brain barrier protective and anti-inflammatory agent: implications for neuroinflammation, J. Neuroinflammation, 2018, 15(1), 25 CrossRef PubMed.
- Y. Zhao, J. Zhang, Y. Zheng, Y. Zhang, X. J. Zhang and H. Wang,
et al., NAD+ improves cognitive function and reduces neuroinflammation by ameliorating mitochondrial damage and decreasing ROS production in chronic cerebral hypoperfusion models through Sirt1/PGC-1α pathway, J. Neuroinflammation, 2021, 18, 207 CrossRef CAS PubMed.
- D. Zhang, B. Jing, Z. N. Chen, X. Li, H. M. Shi and Y. C. Zheng,
et al., Ferulic acid alleviates sciatica by inhibiting neuroinflammation and promoting nerve repair via the TLR4/NF-κB pathway, CNS Neurosci. Ther., 2023, 29(4), 1000–1011 CrossRef CAS PubMed.
- S. Mani, R. Dubey, I. C. Lai, M. A. Babu, S. Tyagi and G. Swargiary,
et al., Oxidative Stress and Natural Antioxidants: Back and Forth in the Neurological Mechanisms of Alzheimer's Disease, J. Alzheimer's Dis., 2023, 96(3), 877–912 CAS.
- Y. Takahashi, N. Morimoto, M. Takamiya, M. Morimoto, S. Mino and S. Kuramoto,
et al., Successful treatment with corticosteroids for refractory deterioration of cryptococcal meningitis with an increasing cryptococcal antigen titer, J. Clin. Neurosci., 2020, 72, 455–457 CrossRef CAS PubMed.
- S. Alkabie and A. Budhram, Prolonged Corticosteroids Without Maintenance Immunotherapy for Treatment of Anti-LGI1 Encephalitis: Analysis of Outcomes and Relapse Rate, Neurol. Neuroimmunol. Neuroinflamm., 2023, 10(3), e200115 CrossRef PubMed.
- M. Wang, H. Zhang, J. Liang, J. Huang and N. Chen, Exercise suppresses neuroinflammation for alleviating Alzheimer's disease, J. Neuroinflammation, 2023, 20, 76 CrossRef PubMed.
- Y. Zhang, P. Zhang, X. Shen, S. Tian, Y. Wu and Y. Zhu,
et al., Early Exercise Protects the Blood-Brain Barrier from Ischemic Brain Injury via the Regulation of MMP-9 and Occludin in Rats, Int. J. Mol. Sci., 2013, 14(6), 11096–11112 CrossRef PubMed.
- A. Jacob, L. Nina Peralta, D. Pegues, K. Okamura, A. Chang and D. McSkimming,
et al., Exercise alleviates symptoms of CNS lupus, Brain Res., 2021, 1765, 147478 CrossRef CAS PubMed.
- Y. Tao, S. X. Leng and H. Zhang, Ketogenic Diet: An Effective Treatment Approach for Neurodegenerative Diseases, Curr. Neuropharmacol., 2022, 20(12), 2303–2319 CrossRef CAS PubMed.
- Z. Jiang, X. Yin, M. Wang, T. Chen, Y. Wang and Z. Gao,
et al., Effects of Ketogenic Diet on Neuroinflammation in Neurodegenerative Diseases, Aging Dis., 2022, 13(4), 1146–1165 CrossRef PubMed.
- Y. Xu, C. Jiang, J. Wu, P. Liu, X. Deng and Y. Zhang,
et al., Ketogenic diet ameliorates cognitive impairment and neuroinflammation in a mouse model of Alzheimer's disease, CNS Neurosci. Ther., 2022, 28(4), 580–592 CrossRef CAS PubMed.
- J. Jiang, H. Pan, F. Shen, Y. Tan and S. Chen, Ketogenic diet alleviates cognitive dysfunction and neuroinflammation in APP/PS1 mice via the Nrf2/HO-1 and NF-κB signaling pathways, Neural Regener. Res., 2023, 18(12), 2767–2772 CrossRef CAS PubMed.
- C. Li, J. Pan, P. Sun, S. Wang, S. Wang and W. Feng,
et al., Ketogenic Diet Alleviates Hypoglycemia-Induced Neuroinflammation via Modulation the Gut Microbiota in Mice, Mol. Nutr. Food Res., 2023, 67(11), e2200711 CrossRef PubMed.
- P. Moscariello, D. Y. W. Ng, M. Jansen, T. Weil, H. J. Luhmann and J. Hedrich, Brain Delivery of Multifunctional Dendrimer Protein Bioconjugates, Adv. Sci., 2018, 5(5), 1700897 CrossRef PubMed.
- E. Bellotti, A. L. Schilling, S. R. Little and P. Decuzzi, Injectable thermoresponsive hydrogels as drug delivery system for the treatment of central nervous system disorders: A review, J. Controlled Release, 2021, 329, 16–35 CrossRef CAS PubMed.
- Z. Wei, J. Zhao, Y. M. Chen, P. Zhang and Q. Zhang, Self-healing polysaccharide-based hydrogels as injectable carriers for neural stem cells, Sci. Rep., 2016, 6(1), 37841 CrossRef CAS PubMed.
- Z. Z. Khaing, N. K. Agrawal, J. H. Park, S. Xin, G. C. Plumton and K. H. Lee,
et al., Localized and sustained release of brain-derived neurotrophic factor from injectable hydrogel/microparticle composites fosters spinal learning after spinal cord injury, J. Mater. Chem. B, 2016, 4(47), 7560–7571 RSC.
- J. Piantino, J. A. Burdick, D. Goldberg, R. Langer and L. I. Benowitz, An injectable, biodegradable hydrogel for trophic factor delivery enhances axonal rewiring and improves performance after spinal cord injury, Exp. Neurol., 2006, 201(2), 359–367 CrossRef CAS PubMed.
- Z. He, H. Zang, L. Zhu, K. Huang, T. Yi and S. Zhang,
et al., An anti-inflammatory peptide and brain-derived neurotrophic factor-modified hyaluronan-methylcellulose hydrogel promotes nerve regeneration in rats with spinal cord injury, Int. J. Nanomed., 2019, 14, 721–732 CrossRef PubMed.
- R. B. Shultz and Y. Zhong, Hydrogel-based local drug delivery strategies for spinal cord repair, Neural Regener. Res., 2020, 16(2), 247–253 Search PubMed.
- A. Bourrier, P. Shkorbatova, M. Bonizzato, E. Rey, Q. Barraud and G. Courtine,
et al., Monolayer Graphene Coating of Intracortical Probes for Long-Lasting Neural Activity Monitoring, Adv. Healthcare Mater., 2019, 8(18), 1801331 CrossRef PubMed.
- C. D. Lee, S. A. Hara, L. Yu, J. T. W. Kuo, B. J. Kim and T. Hoang,
et al., Matrigel coatings for Parylene sheath neural probes, J. Biomed. Mater. Res., Part B, 2016, 104(2), 357–368 CrossRef CAS PubMed.
- N. L. Francis, P. M. Hunger, A. E. Donius, B. W. Riblett, A. Zavaliangos and U. G. K. Wegst,
et al., An ice-templated, linearly aligned chitosan–alginate scaffold for neural tissue engineering, J. Biomed. Mater. Res., Part A, 2013, 101(12), 3493–3503 CrossRef PubMed.
- A. Dityatev, M. Schachner and P. Sonderegger, The dual role of the extracellular matrix in synaptic plasticity and homeostasis, Nat. Rev. Neurosci., 2010, 11(11), 735–746 CrossRef CAS PubMed.
- I. Song and A. Dityatev, Crosstalk between glia, extracellular matrix and neurons, Brain Res. Bull., 2018, 136, 101–108 CrossRef CAS PubMed.
- E. R. Burnside and E. J. Bradbury, Review: Manipulating the extracellular
matrix and its role in brain and spinal cord plasticity and repair, Neuropathol. Appl. Neurobiol., 2014, 40(1), 26–59 CrossRef CAS PubMed.
- D. Lam, H. A. Enright, J. Cadena, S. K. G. Peters, A. P. Sales and J. J. Osburn,
et al., Tissue-specific extracellular matrix accelerates the formation of neural networks and communities in a neuron-glia co-culture on a multi-electrode array, Sci. Rep., 2019, 9(1), 4159 CrossRef PubMed.
- N. J. Allen and D. A. Lyons, Glia as architects of central nervous system formation and function, Science, 2018, 362(6411), 181–185 CrossRef CAS PubMed.
- S. Deng, L. Gan, C. Liu, T. Xu, S. Zhou and Y. Guo,
et al., Roles of Ependymal Cells in the Physiology and Pathology of the Central Nervous System, Aging Dis., 2023, 14(2), 468–483 Search PubMed.
- A. Nimmerjahn, F. Kirchhoff and F. Helmchen, Resting microglial cells are highly dynamic surveillants of brain parenchyma in vivo, Science, 2005, 308(5726), 1314–1318 CrossRef CAS PubMed.
- T. R. F. Green, S. M. Murphy, M. P. Moreno-Montano, E. Audinat and R. K. Rowe, Reactive morphology of dividing microglia following kainic acid administration, Front. Neurosci., 2022, 16, 972138 CrossRef PubMed.
- Y. Fujita and T. Yamashita, Alterations in Chromatin Structure and Function in the Microglia, Front. Cell Dev. Biol., 2021, 8, 626541 CrossRef PubMed.
- Y. L. Tan, Y. Yuan and L. Tian, Microglial regional heterogeneity and its role in the brain, Mol. Psychiatry, 2020, 25(2), 351–367 CrossRef PubMed.
- R. M. Ransohoff, A polarizing question: do M1 and M2 microglia exist?, Nat. Neurosci., 2016, 19(8), 987–991 CrossRef CAS PubMed.
- C. C. Kim, M. C. Nakamura and C. L. Hsieh, Brain trauma elicits non-canonical macrophage activation states, J. Neuroinflammation, 2016, 13(1), 117 CrossRef PubMed.
- H. R. Peng, Y. K. Zhang and J. W. Zhou, The Structure and Function of Glial Networks: Beyond the Neuronal Connections, Neurosci. Bull., 2023, 39(3), 531–540 CrossRef PubMed.
- I. Molina-Gonzalez, R. K. Holloway, Z. Jiwaji, O. Dando, S. A. Kent and K. Emelianova,
et al., Astrocyte–oligodendrocyte interaction regulates central nervous system regeneration, Nat. Commun., 2023, 14(1), 3372 CrossRef CAS PubMed.
- B. P. Heithoff, K. K. George, A. N. Phares, I. A. Zuidhoek, C. Munoz-Ballester and S. Robel, Astrocytes are necessary for blood-brain barrier maintenance in the adult mouse brain, Glia, 2021, 69(2), 436–472 CrossRef CAS PubMed.
- H. Kadry, B. Noorani and L. Cucullo, A blood–brain barrier overview on structure, function, impairment, and biomarkers of integrity, Fluids Barriers CNS, 2020, 17, 69 CrossRef PubMed.
- G. Castellani, T. Croese, J. M. Peralta Ramos and M. Schwartz, Transforming the understanding of brain immunity, Science, 2023, 380(6640), eabo7649 CrossRef CAS PubMed.
- J. Rustenhoven, A. Drieu, T. Mamuladze, K. A. de Lima, T. Dykstra and M. Wall,
et al., Functional characterization of the dural sinuses as a neuroimmune interface, Cell, 2021, 184(4), 1000–1016 CrossRef CAS PubMed.
- Y. Huang, S. Chen, Y. Luo and Z. Han, Crosstalk between Inflammation and the BBB in Stroke, Curr. Neuropharmacol., 2020, 18(12), 1227–1236 CrossRef CAS PubMed.
- S. Garofalo, G. Cocozza, G. Bernardini, J. Savage, M. Raspa and E. Aronica,
et al., Blocking immune cell infiltration of the central nervous system to tame Neuroinflammation in Amyotrophic lateral sclerosis, Brain, Behav., Immun., 2022, 105, 1–14 CrossRef CAS PubMed.
- G. P. Williams, A. M. Schonhoff, A. Jurkuvenaite, N. J. Gallups, D. G. Standaert and A. S. Harms, CD4 T cells mediate
brain inflammation and neurodegeneration in a mouse model of Parkinson's disease. Brain, J. Neurol., 2021, 144(7), 2047–2059 Search PubMed.
- D. Gate, N. Saligrama, O. Leventhal, A. C. Yang, M. S. Unger and J. Middeldorp,
et al., Clonally expanded CD8 T cells patrol the cerebrospinal fluid in Alzheimer's disease, Nature, 2020, 577(7790), 399–404 CrossRef CAS PubMed.
- T. H. Khanh Vu, H. Chen, L. Pan, K. S. Cho, D. Doesburg and E. F. Thee,
et al., CD4+ T-Cell Responses Mediate Progressive Neurodegeneration in Experimental Ischemic Retinopathy, Am. J. Pathol., 2020, 190(8), 1723–1734 CrossRef CAS PubMed.
- D. J. Hellenbrand, C. M. Quinn, Z. J. Piper, C. N. Morehouse, J. A. Fixel and A. S. Hanna, Inflammation after spinal cord injury: a review of the critical timeline of signaling cues and cellular infiltration, J. Neuroinflammation, 2021, 18(1), 284 CrossRef CAS PubMed.
- S. P. Gadani, J. T. Walsh, I. Smirnov, J. Zheng and J. Kipnis, The Glia-Derived Alarmin IL-33 Orchestrates the Immune Response and Promotes Recovery following CNS Injury, Neuron, 2015, 85(4), 703–709 CrossRef CAS PubMed.
- Y. Pomeshchik, I. Kidin, P. Korhonen, E. Savchenko, M. Jaronen and S. Lehtonen,
et al., Interleukin-33 treatment reduces secondary injury and improves functional recovery after contusion spinal cord injury, Brain, Behav., Immun., 2015, 44, 68–81 CrossRef CAS PubMed.
- A. F. M. Salvador and J. Kipnis, Immune response after central nervous system injury, Semin. Immunol., 2022, 59, 101629 CrossRef CAS PubMed.
- M. Chaturvedi and L. Kaczmarek, MMP-9 Inhibition: a Therapeutic Strategy in Ischemic Stroke, Mol. Neurobiol., 2014, 49(1), 563–573 CrossRef CAS PubMed.
- J. Y. Lee, H. Y. Choi, H. J. Ahn, B. G. Ju and T. Y. Yune, Matrix Metalloproteinase-3 Promotes Early Blood–Spinal Cord Barrier Disruption and Hemorrhage and Impairs Long-Term Neurological Recovery after Spinal Cord Injury, Am. J. Pathol., 2014, 184(11), 2985–3000 CrossRef CAS PubMed.
- D. DiSabato, N. Quan and J. P. Godbout, Neuroinflammation: The Devil is in the Details, J. Neurochem., 2016, 139(Suppl 2), 136–153 CrossRef CAS PubMed.
- D. W. Simon, M. J. McGeachy, H. Bayır, R. S. B. Clark, D. J. Loane and P. M. Kochanek, The far-reaching scope of neuroinflammation after traumatic brain injury, Nat. Rev. Neurol., 2017, 13(3), 171–191 CrossRef PubMed.
- S. M. LeVine, Albumin and multiple sclerosis, BMC Neurol., 2016, 16, 47 CrossRef PubMed.
- M. D. Habgood, N. Bye, K. M. Dziegielewska, C. J. Ek, M. A. Lane and A. Potter,
et al., Changes in blood-brain barrier permeability to large and small molecules following traumatic brain injury in mice, Eur. J. Neurosci., 2007, 25(1), 231–238 CrossRef CAS PubMed.
- A. Shafqat, A. Noor Eddin, G. Adi, M. Al-Rimawi, S. Abdul Rab and M. Abu-Shaar,
et al., Neutrophil extracellular traps in central nervous system pathologies: A mini review, Front. Med., 2023, 10, 1083242 CrossRef PubMed.
- M. Javdani and A. Barzegar-Bafrouei, The Key Role of Macrophages and Monocytes in Spinal Cord Injury: Development of Novel Therapeutic Approaches, Neurosci. J., 2020, 8(4), 90–102 Search PubMed.
- R. A. Saxton, N. Tsutsumi, L. L. Su, G. C. Abhiraman, K. Mohan and L. T. Henneberg,
et al., Structure-based decoupling of the pro- and anti-inflammatory functions of interleukin-10, Science, 2021, 371(6535), eabc8433 CrossRef CAS PubMed.
- A. M. Jurga, M. Paleczna and K. Z. Kuter, Overview of General and Discriminating Markers of Differential Microglia Phenotypes, Front. Cell. Neurosci., 2020, 14, 198 CrossRef PubMed.
- K. Ohsawa, Y. Imai, Y. Sasaki and S. Kohsaka, Microglia/macrophage-specific protein Iba1 binds to fimbrin and enhances its actin-bundling activity, J. Neurochem., 2004, 88(4), 844–856 CrossRef CAS PubMed.
- S. A. Liddelow, K. A. Guttenplan, L. E. Clarke, F. C. Bennett, C. J. Bohlen and L. Schirmer,
et al., Neurotoxic reactive astrocytes are induced by activated microglia, Nature, 2017, 541(7638), 481–487 CrossRef CAS PubMed.
- W. Ng and S. Y. Ng, Remodeling of astrocyte secretome in amyotrophic lateral sclerosis: uncovering novel targets to combat astrocyte-mediated toxicity, Transl. Neurodegener., 2022, 11(1), 54 CrossRef CAS PubMed.
- F. Bi, C. Huang, J. Tong, G. Qiu, B. Huang and Q. Wu,
et al., Reactive astrocytes secrete lcn2 to promote neuron death, Proc. Natl. Acad. Sci. U. S. A., 2013, 110(10), 4069–4074 CrossRef CAS PubMed.
- A. Becerra-Calixto and G. P. Cardona-Gómez, The Role of Astrocytes in Neuroprotection after Brain Stroke: Potential in Cell Therapy, Front. Mol. Neurosci., 2017, 10, 88 Search PubMed.
- L. P. Diniz, V. Tortelli, I. Matias, J. Morgado, A. P. B. Araujo and H. M. Melo,
et al., Astrocyte Transforming Growth Factor Beta 1 Protects Synapses against Aβ Oligomers in Alzheimer's Disease Model, J. Neurosci., 2017, 37(28), 6797–6809 CrossRef CAS PubMed.
- E. Cekanaviciute, H. K. Dietrich, R. C. Axtell, A. M. Williams, R. Egusquiza and K. M. Wai,
et al., Astrocytic TGF-β Signaling Limits Inflammation and Reduces Neuronal Damage during Central Nervous System Toxoplasma Infection, J. Immunol., 2014, 193(1), 139–149 CrossRef CAS PubMed.
- Y. Zhou, A. Shao, Y. Yao, S. Tu, Y. Deng and J. Zhang, Dual roles of astrocytes in plasticity and reconstruction after traumatic brain injury, Cell Commun. Signaling, 2020, 18(1), 62 CrossRef PubMed.
- T. Yang, Y. Dai, G. Chen and S. Cui, Dissecting the Dual Role of the Glial Scar and Scar-Forming Astrocytes in Spinal Cord Injury, Front. Cell. Neurosci., 2020, 14, 78 CrossRef CAS PubMed.
- X. He, Y. Li, B. Deng, A. Lin, G. Zhang and M. Ma,
et al., The PI3K/AKT signalling pathway in inflammation, cell death and glial scar formation after traumatic spinal cord injury: Mechanisms and therapeutic opportunities, Cell Proliferation, 2022, 55(9), e13275 CrossRef CAS PubMed.
- K. L. Adams and V. Gallo, The diversity and disparity of the glial scar, Nat. Neurosci., 2018, 21(1), 9–15 CrossRef CAS PubMed.
- G. Wang, J. Zhang, X. Hu, L. Zhang, L. Mao and X. Jiang,
et al., Microglia/macrophage polarization dynamics in white matter after traumatic brain injury, J. Cereb. Blood Flow Metab., 2013, 33(12), 1864–1874 CrossRef CAS PubMed.
- A. I. Faden, J. Wu, B. A. Stoica and D. J. Loane, Progressive inflammation-mediated neurodegeneration after traumatic brain or spinal cord injury, Br. J. Pharmacol., 2016, 173(4), 681–691 CrossRef CAS PubMed.
- I. Francos-Quijorna, M. Sánchez-Petidier, E. R. Burnside, S. R. Badea, A. Torres-Espin and L. Marshall,
et al., Chondroitin sulfate proteoglycans prevent immune cell phenotypic conversion and inflammation resolution via TLR4 in rodent models of spinal cord injury, Nat. Commun., 2022, 13(1), 2933 CrossRef CAS PubMed.
- T. Heurtaux, A. Michelucci, S. Losciuto, C. Gallotti, P. Felten and G. Dorban,
et al., Microglial activation depends on beta-amyloid conformation: role of the formylpeptide receptor 2, J. Neurochem., 2010, 114(2), 576–586 CrossRef CAS PubMed.
- X. Su, K. A. Maguire-Zeiss, R. Giuliano, L. Prifti, K. Venkatesh and H. J. Federoff, Synuclein activates microglia in a model of Parkinson's Disease, Neurobiol. Aging, 2008, 29(11), 1690–1701 CrossRef CAS PubMed.
- S. Hickman, S. Izzy, P. Sen, L. Morsett and J. El Khoury, Microglia in neurodegeneration, Nat. Neurosci., 2018, 21(10), 1359–1369 CrossRef CAS PubMed.
- R. L. Jayaraj, S. Azimullah, R. Beiram, F. Y. Jalal and G. A. Rosenberg, Neuroinflammation: friend and foe for ischemic stroke, J. Neuroinflammation, 2019, 16(1), 142 CrossRef PubMed.
- Y. Guo, L. Dong, A. Gong, J. Zhang, L. Jing and T. Ding,
et al., Damage to the blood-brain barrier and activation of neuroinflammation by focal cerebral ischemia under hyperglycemic condition, Int. J. Mol. Med., 2021, 48(1), 142 CrossRef CAS PubMed.
- X. Hu, T. M. De Silva, J. Chen and F. M. Faraci, Cerebral Vascular Disease and Neurovascular Injury in Ischemic Stroke, Circ. Res., 2017, 120(3), 449–471 CrossRef CAS PubMed.
- L. Marchetti and B. Engelhardt, Immune cell trafficking across the blood-brain barrier in the absence and presence of neuroinflammation, Vasc. Biol., 2020, 2(1), H1–H18 CAS.
- S. Bernardo-Castro, J. A. Sousa, A. Brás, C. Cecília, B. Rodrigues and L. Almendra,
et al., Pathophysiology of Blood–Brain Barrier Permeability Throughout the Different Stages of Ischemic Stroke and Its Implication on Hemorrhagic Transformation and Recovery, Front. Neurol., 2020, 11, 594672 CrossRef PubMed.
- M. Fang, L. Zhong, X. Jin, R. Cui, W. Yang and S. Gao,
et al., Effect of Inflammation on the Process of Stroke Rehabilitation and Poststroke Depression, Front. Psychiatry, 2019, 10, 184 CrossRef PubMed.
- H. J. Audebert, M. M. Rott, T. Eck and R. L. Haberl, Systemic Inflammatory Response Depends on Initial Stroke Severity but Is Attenuated by Successful Thrombolysis, Stroke, 2004, 35(9), 2128–2133 CrossRef PubMed.
- S. L. Hauser and B. A. C. Cree, Treatment of Multiple Sclerosis: A Review, Am. J. Med., 2020, 133(12), 1380–1390 CrossRef CAS PubMed.
- S. Haase and R. A. Linker, Inflammation in multiple sclerosis, Ther. Adv. Neurol. Disord., 2021, 14, 17562864211007687 CAS.
- M. Carstensen, T. Christensen, M. Stilund, H. J. Møller, E. L. Petersen and T. Petersen, Activated monocytes and markers of inflammation in newly diagnosed multiple sclerosis, Immunol. Cell Biol., 2020, 98(7), 549–562 CrossRef CAS PubMed.
- E. O’Loughlin, C. Madore, H. Lassmann and O. Butovsky, Microglial Phenotypes and Functions in Multiple Sclerosis, Cold Spring Harbor Perspect. Med., 2018, 8(2), a028993 CrossRef PubMed.
- F. Cignarella, F. Filipello, B. Bollman, C. Cantoni, A. Locca and R. Mikesell,
et al., TREM2 activation on microglia promotes myelin debris clearance and remyelination in a model of multiple sclerosis, Acta Neuropathol., 2020, 140(4), 513–534 CrossRef CAS PubMed.
- Q. T. Ostrom, M. Price, C. Neff, G. Cioffi, K. A. Waite and C. Kruchko,
et al., CBTRUS Statistical Report: Primary Brain and Other Central Nervous System Tumors Diagnosed in the United States in 2015–2019, Neuro Oncol., 2022, 24(Suppl 5), v1–95 CAS.
- K. Gabrusiewicz, B. Rodriguez, J. Wei, Y. Hashimoto, L. M. Healy and S. N. Maiti,
et al., Glioblastoma-infiltrated innate immune cells resemble M0 macrophage phenotype, JCI Insight, 2016, 1(2), e85841 CrossRef PubMed.
- X. Ma, H. Zhu, L. Cheng, X. Chen, K. Shu and S. Zhang, Targeting FGL2 in glioma immunosuppression and malignant progression, Front. Oncol., 2022, 12, 1004700 CrossRef CAS PubMed.
- A. X. Chen, R. D. Gartrell, J. Zhao, P. S. Upadhyayula, W. Zhao and J. Yuan,
et al., Single-cell characterization of macrophages in glioblastoma reveals MARCO as a mesenchymal pro-tumor marker, Genome Med., 2021, 13, 88 CrossRef CAS PubMed.
- P. Peng, H. Zhu, D. Liu, Z. Chen, X. Zhang and Z. Guo,
et al., TGFBI secreted by tumor-associated macrophages promotes glioblastoma stem cell-driven tumor growth via integrin αvβ5-Src-Stat3 signaling, Theranostics, 2022, 12(9), 4221–4236 CrossRef CAS PubMed.
- Q. Liu, G. Li, R. Li, J. Shen, Q. He and L. Deng,
et al., IL-6 promotion of glioblastoma cell invasion and angiogenesis in U251 and T98G cell lines, J. Neurooncol., 2010, 100(2), 165–176 CrossRef CAS PubMed.
- V. M. Ravi, N. Neidert, P. Will, K. Joseph, J. P. Maier and J. Kückelhaus,
et al., T-cell dysfunction in the glioblastoma microenvironment is mediated by myeloid cells releasing interleukin-10, Nat. Commun., 2022, 13(1), 925 CrossRef CAS PubMed.
- E. P. Sulman, N. Ismaila, T. S. Armstrong, C. Tsien, T. T. Batchelor and T. Cloughesy,
et al., Radiation Therapy for Glioblastoma: American Society of Clinical Oncology Clinical Practice Guideline Endorsement of the American Society for Radiation Oncology Guideline, J. Clin. Oncol., 2017, 35(3), 361–369 CrossRef CAS PubMed.
-
S. Manrique-Guzmán, T. Herrada-Pineda and F. Revilla-Pacheco, Surgical Management of Glioblastoma. in Glioblastoma, ed. S. De Vleeschouwer, Codon Publications, Brisbane (AU), 2017, Available from: https://www.ncbi.nlm.nih.gov/books/NBK469999/ Search PubMed.
- K. Vitrikas, H. Dalton and D. Breish, Cerebral Palsy:An Overview, Am. Fam. Physician, 2020, 101(4), 213–220 Search PubMed.
- E. Jung, R. Romero, L. Yeo, R. Diaz-Primera, J. Marin-Concha and R. Para,
et al., The fetal inflammatory response syndrome: the origins of a concept, pathophysiology, diagnosis, and obstetrical implications, Semin. Fetal Neonatal Med., 2020, 25(4), 101146 CrossRef PubMed.
- R. Kölliker-Frers, L. Udovin, M. Otero-Losada, T. Kobiec, M. I. Herrera and J. Palacios,
et al., Neuroinflammation: An Integrating Overview of Reactive-Neuroimmune Cell Interactions in Health and Disease, Mediators Inflammation, 2021, 2021, 9999146 Search PubMed.
- G. H. Kim, J. E. Kim, S. J. Rhie and S. Yoon, The Role of Oxidative Stress in Neurodegenerative Diseases, Exp. Neurobiol., 2015, 24(4), 325–340 CrossRef PubMed.
- P. M. Liy, N. N. A. Puzi, S. Jose and S. Vidyadaran, Nitric oxide modulation in neuroinflammation and the role of mesenchymal stem cells, Exp. Biol. Med., 2021, 246(22), 2399–2406 CrossRef CAS PubMed.
- I. Pajares M, A. Rojo, G. Manda, L. Boscá and A. Cuadrado, Inflammation in Parkinson's Disease: Mechanisms and Therapeutic Implications, Cells, 2020, 9(7), 1687 CrossRef PubMed.
- H. Cho, S. P. Kambhampati, M. J. Lai, L. Zhou, G. Lee and Y. Xie,
et al., Dendrimer-Triamcinolone Acetonide Reduces Neuroinflammation, Pathological Angiogenesis, and Neuroretinal Dysfunction in Ischemic Retinopathy, Adv. Ther., 2021, 4(2), 2000181 CrossRef CAS PubMed.
- K. R. Hollinger, A. Sharma, C. Tallon, L. Lovell, A. G. Thomas and X. Zhu,
et al., Dendrimer-2PMPA selectively blocks upregulated microglial GCPII activity and improves cognition in a mouse model of multiple sclerosis, Nanotheranostics, 2022, 6(2), 126–142 CrossRef PubMed.
- F. Zhang, Z. Zhang, J. Alt, S. P. Kambhampati, A. Sharma and S. Singh,
et al., Dendrimer-enabled targeted delivery attenuates glutamate excitotoxicity and improves motor function in a rabbit model of cerebral palsy, J. Controlled Release, 2023, 358, 27–42 CrossRef CAS PubMed.
- D. Zhang, Y. Ren, Y. He, R. Chang, S. Guo and S. Ma,
et al., In situ forming and biocompatible hyaluronic acid hydrogel with reactive oxygen species-scavenging activity to improve traumatic brain injury repair by suppressing oxidative stress and neuroinflammation, Mater. Today Bio, 2022, 15, 100278 CrossRef CAS PubMed.
- A. Albashari, Y. He, Y. Zhang, J. Ali, F. Lin and Z. Zheng,
et al., Thermosensitive bFGF-Modified Hydrogel with Dental Pulp Stem Cells on Neuroinflammation of Spinal Cord Injury, ACS Omega, 2020, 5(26), 16064–16075 CrossRef CAS PubMed.
- Y. Zou, D. Ma, H. Shen, Y. Zhao, B. Xu and Y. Fan,
et al., Aligned collagen scaffold combination with human spinal cord-derived neural stem cells to improve spinal cord injury repair, Biomater. Sci., 2020, 8(18), 5145–5156 RSC.
- X. Liu, G. Zhang, P. Wei, L. Hao, L. Zhong and K. Zhong,
et al., 3D-printed collagen/silk fibroin/secretome derived from bFGF-pretreated HUCMSCs scaffolds enhanced therapeutic ability in canines traumatic brain injury model, Front. Bioeng. Biotechnol., 2022, 10, 995099 CrossRef PubMed.
- X. Liu, J. Wang, P. Wang, L. Zhong, S. Wang and Q. Feng,
et al., Hypoxia-pretreated mesenchymal stem cell-derived exosomes-loaded low-temperature extrusion 3D-printed implants for neural regeneration after traumatic brain injury in canines, Front. Bioeng. Biotechnol., 2022, 10, 1025138 CrossRef PubMed.
- X. Y. Liu, Y. H. Feng, Q. B. Feng, J. Y. Zhang, L. Zhong and P. Liu,
et al., Low-temperature 3D-printed collagen/chitosan scaffolds loaded with exosomes derived from neural stem cells pretreated with insulin growth factor-1 enhance neural regeneration after traumatic brain injury, Neural Regener. Res., 2023, 18(9), 1990–1998 CAS.
- X. Liu, J. Zhang, X. Cheng, P. Liu, Q. Feng and S. Wang,
et al., Integrated printed BDNF-stimulated HUCMSCs-derived exosomes/collagen/chitosan biological scaffolds with 3D printing technology promoted the remodelling of neural networks after traumatic brain injury, Regener. Biomater., 2023, 10, rbac085 CrossRef CAS PubMed.
- A. Bourrier, A. Szarpak-Jankowska, F. Veliev, R. Olarte-Hernandez, P. Shkorbatova and M. Bonizzato,
et al., Introducing a biomimetic coating for graphene neuroelectronics: towardin-vivoapplications, Biomed. Phys. Eng. Express, 2020, 7(1), 015006 CrossRef PubMed.
- Y. Cui, F. Zhang, G. Chen, L. Yao, N. Zhang and Z. Liu,
et al., A Stretchable and Transparent Electrode Based on PEGylated Silk Fibroin for In Vivo Dual-Modal Neural-Vascular Activity Probing, Adv. Mater., 2021, 33(34), e2100221 CrossRef PubMed.
- C. Kleber, M. Bruns, K. Lienkamp, J. Rühe and M. Asplund, An interpenetrating, microstructurable and covalently attached conducting polymer hydrogel for neural interfaces, Acta Biomater., 2017, 58, 365–375 CrossRef CAS PubMed.
- M. A. Dobrovolskaia, P. Aggarwal, J. B. Hall and S. E. McNeil, Preclinical Studies To Understand Nanoparticle Interaction with the Immune System and Its Potential Effects on Nanoparticle Biodistribution, Mol. Pharmaceutics, 2008, 5(4), 487–495 CrossRef CAS PubMed.
- Y. Zhou, Z. Peng, E. S. Seven and R. M. Leblanc, Crossing the blood-brain barrier with nanoparticles, J. Controlled Release, 2018, 270, 290–303 CrossRef CAS PubMed.
- S. Honary and F. Zahir, Effect of Zeta Potential on the Properties of Nano-Drug Delivery Systems – A Review (Part 1), Trop. J. Pharm. Res., 2013, 12(2), 255–264 Search PubMed.
- N. Ma, C. Ma, C. Li, T. Wang, Y. Tang and H. Wang,
et al., Influence of nanoparticle shape, size, and surface functionalization on cellular uptake, J. Nanosci. Nanotechnol., 2013, 13(10), 6485–6498 CrossRef CAS PubMed.
- S. M. Lombardo, M. Schneider, A. E. Türeli and N. Günday Türeli, Key for crossing the BBB with nanoparticles: the rational design, Beilstein J. Nanotechnol., 2020, 11, 866–883 CrossRef CAS PubMed.
- S. Zhang, J. Li, G. Lykotrafitis, G. Bao and S. Suresh, Size-Dependent Endocytosis of Nanoparticles, Adv. Mater., 2009, 21, 419–424 CrossRef CAS PubMed.
- A. G. Godin, J. A. Varela, Z. Gao, N. Danné, J. P. Dupuis and B. Lounis,
et al., Single-nanotube tracking reveals the nanoscale organization of the extracellular space in the live brain, Nat. Nanotechnol., 2017, 12(3), 238–243 CrossRef CAS PubMed.
- C. Nicholson and S. Hrabětová, Brain Extracellular Space: The Final Frontier of Neuroscience, Biophys. J., 2017, 113(10), 2133–2142 CrossRef CAS PubMed.
- M. N. Singh, K. S. Y. Hemant, M. Ram and H. G. Shivakumar, Microencapsulation: A promising technique for controlled drug delivery, Res. Pharm. Sci., 2010, 5(2), 65–77 CAS.
- S. Weng, Q. L. Lai, J. Wang, L. Zhuang, L. Cheng and Y. Mo,
et al., The Role of Exosomes as Mediators of Neuroinflammation in the Pathogenesis and Treatment of Alzheimer's Disease, Front. Aging Neurosci., 2022, 14, 899944 CrossRef CAS PubMed.
- X. Long, X. Yao, Q. Jiang, Y. Yang, X. He and W. Tian,
et al., Astrocyte-derived exosomes enriched with miR-873a-5p inhibit neuroinflammation via microglia phenotype modulation after traumatic brain injury, J. Neuroinflammation, 2020, 17(1), 89 CrossRef CAS PubMed.
- X. Liu, M. Zhang, H. Liu, R. Zhu, H. He and Y. Zhou,
et al., Bone marrow mesenchymal stem cell-derived exosomes attenuate cerebral ischemia-reperfusion injury-induced neuroinflammation and pyroptosis by modulating microglia M1/M2 phenotypes, Exp. Neurol., 2021, 341, 113700 CrossRef CAS PubMed.
- K. Ekström, R. Crescitelli, H. I. Pétursson, J. Johansson, C. Lässer and R. Olofsson Bagge, Characterization of surface markers on extracellular vesicles isolated from lymphatic exudate from patients with breast cancer, BMC Cancer, 2022, 22(1), 50 CrossRef PubMed.
- M. Riazifar, M. R. Mohammadi, E. J. Pone, A. Yeri, C. Lässer and A. I. Segaliny,
et al., Stem Cell-Derived Exosomes as Nanotherapeutics for Autoimmune and Neurodegenerative Disorders, ACS Nano, 2019, 13(6), 6670–6688 CrossRef CAS PubMed.
- P. Xian, Y. Hei, R. Wang, T. Wang, J. Yang and J. Li,
et al., Mesenchymal stem cell-derived exosomes as a nanotherapeutic agent for amelioration of inflammation-induced astrocyte alterations in mice, Theranostics, 2019, 9(20), 5956–5975 CrossRef CAS PubMed.
- X. Long, X. Yao, Q. Jiang, Y. Yang, X. He and W. Tian,
et al., Astrocyte-derived exosomes enriched with miR-873a-5p inhibit neuroinflammation via microglia phenotype modulation after traumatic brain injury, J. Neuroinflammation, 2020, 17, 89 CrossRef CAS PubMed.
- W. Liu, Y. Rong, J. Wang, Z. Zhou, X. Ge and C. Ji,
et al., Exosome-shuttled miR-216a-5p from hypoxic preconditioned mesenchymal stem cells repair traumatic spinal cord injury by shifting microglial M1/M2 polarization, J. Neuroinflammation, 2020, 17(1), 47 CrossRef CAS PubMed.
- M. Riazifar, M. R. Mohammadi, E. J. Pone, A. Yeri, C. Lässer and A. I. Segaliny,
et al., Stem Cell-Derived Exosomes as Nanotherapeutics for Autoimmune and Neurodegenerative Disorders, ACS Nano, 2019, 13(6), 6670–6688 CrossRef CAS PubMed.
- Y. Cheng, Z. Xu, M. Ma and T. Xu, Dendrimers as drug carriers: applications in different routes of drug administration, J. Pharm. Sci., 2008, 97(1), 123–143 CrossRef CAS PubMed.
- S. Choudhary, L. Gupta, S. Rani, K. Dave and U. Gupta, Impact of Dendrimers on Solubility of Hydrophobic Drug Molecules, Front. Pharmacol., 2017, 8, 261 CrossRef PubMed.
- F. Najafi, M. Salami-Kalajahi, H. Roghani-Mamaqani and A. Kahaie-Khosrowshahi, A comparative study on solubility improvement of tetracycline and dexamethasone by poly(propylene imine) and polyamidoamine dendrimers: An insight into cytotoxicity and cell proliferation, J. Biomed. Mater. Res., Part A, 2020, 108(3), 485–495 CrossRef CAS PubMed.
- H. Namazi and M. Adeli, Dendrimers of citric acid and poly (ethylene glycol) as the new drug-delivery agents, Biomaterials, 2005, 26(10), 1175–1183 CrossRef CAS PubMed.
- R. S. Navath, Y. E. Kurtoglu, B. Wang, S. Kannan, R. Romero and R. M. Kannan, Dendrimer-drug conjugates for tailored intracellular drug release based on glutathione levels, Bioconjugate Chem., 2008, 19(12), 2446–2455 CrossRef CAS PubMed.
- Y. E. Kurtoglu, M. K. Mishra, S. Kannan and R. M. Kannan, Drug release characteristics of PAMAM dendrimer-drug conjugates with different linkers, Int. J. Pharm., 2010, 384(1–2), 189–194 CrossRef CAS PubMed.
- I. J. Majoros, A. Myc, T. Thomas, C. B. Mehta and J. R. Baker, PAMAM dendrimer-based multifunctional conjugate for cancer therapy: synthesis, characterization, and functionality, Biomacromolecules, 2006, 7(2), 572–579 CrossRef CAS PubMed.
- S. P. Kuruvilla, G. Tiruchinapally, A. C. Crouch, M. E. H. ElSayed and J. M. Greve, Dendrimer-doxorubicin conjugates exhibit improved anticancer activity and reduce doxorubicin-induced cardiotoxicity in a murine hepatocellular carcinoma model, PLoS One, 2017, 12(8), e0181944 CrossRef PubMed.
- M. T. McMahon and J. W. M. Bulte, Two decades of dendrimers as versatile MRI agents: a tale with and without metals, Wiley Interdiscip. Rev.: Nanomed. Nanobiotechnol., 2018, 10(3), e1496 Search PubMed.
- L. Zhao, J. Zhu, Y. Cheng, Z. Xiong, Y. Tang and L. Guo,
et al., Chlorotoxin-Conjugated Multifunctional Dendrimers Labeled with Radionuclide 131I for Single Photon Emission Computed Tomography Imaging and Radiotherapy of Gliomas, ACS Appl. Mater. Interfaces, 2015, 7(35), 19798–19808 CrossRef CAS PubMed.
- R. Sharma, K. Liaw, A. Sharma, A. Jimenez, M. Chang and S. Salazar,
et al., Glycosylation of PAMAM Dendrimers Significantly Improves Tumor Macrophage Targeting and Specificity in Glioblastoma, J. Controlled Release, 2021, 337, 179–192 CrossRef CAS PubMed.
-
A. Chauhan, B. Anton and M. K. Singh, Pharmaceutical Applications of Dendrimers, ed. Chauhan A. and Kulhari H., Elsevier, 2019, ch. 3, pp. 59–92 Search PubMed.
- A. Shi, E. Khoury, N. Sah, A. Fowler, K. Liaw and A. Sharma,
et al., Characterization of microglial phagocytosis and dendrimer nanoparticle uptake in a neonatal rabbit model of cerebral palsy, Precis Nanomedicine, 2021, 4(4), 840–850 Search PubMed.
- H. Dai, R. S. Navath, B. Balakrishnan, B. R. Guru, M. K. Mishra and R. Romero,
et al., Intrinsic targeting of inflammatory cells in the brain by polyamidoamine dendrimers upon subarachnoid administration, Nanomedicine, 2010, 5(9), 1317–1329 CrossRef CAS PubMed.
- Y. Alnasser, S. P. Kambhampati, E. Nance, L. Rajbhandari, S. Shrestha and A. Venkatesan,
et al., Preferential and Increased Uptake of Hydroxyl-Terminated PAMAM Dendrimers by Activated Microglia in Rabbit Brain Mixed Glial Culture, Molecules, 2018, 23(5), 1025 CrossRef PubMed.
- S. Yang, D. Datta, W. Elizabeth, A. Duque, Y. M. Morozov and J. Arellano,
et al., Inhibition of glutamate-carboxypeptidase-II in dorsolateral prefrontal cortex: potential therapeutic target for neuroinflammatory cognitive disorders, Mol. Psychiatry, 2022, 27(10), 4252–4263 CrossRef CAS PubMed.
- N. Sah, Z. Zhang, A. Chime, A. Fowler, A. Mendez-Trendler and A. Sharma,
et al., Dendrimer conjugated glutamate carboxypeptidase II inhibitor restores microglial changes in a rabbit model of cerebral palsy, Dev. Neurosci., 2023, 45, 268–275 CrossRef CAS PubMed.
- O. Cekiç, S. Chang, J. J. Tseng, Y. Akar, G. R. Barile and W. M. Schiff, Cataract progression after intravitreal triamcinolone injection, Am. J. Ophthalmol., 2005, 139(6), 993–998 CrossRef PubMed.
- R. Sharma, S. P. Kambhampati, Z. Zhang, A. Sharma, S. Chen and E. I. Duh,
et al., Dendrimer mediated targeted delivery of sinomenine for the treatment of acute neuroinflammation in traumatic brain injury, J. Controlled Release, 2020, 323, 361–375 CrossRef CAS PubMed.
- K. Liaw, R. Sharma, A. Sharma, S. Salazar, S. Appiani La Rosa and R. M. Kannan, Systemic dendrimer delivery of triptolide to tumor-associated macrophages improves anti-tumor efficacy and reduces systemic toxicity in glioblastoma, J. Controlled Release, 2021, 329, 434–444 CrossRef CAS PubMed.
- K. Liaw, F. Zhang, A. Mangraviti, S. Kannan, B. Tyler and R. M. Kannan, Dendrimer size effects on the selective brain tumor targeting in orthotopic tumor models upon systemic administration, Bioeng. Transl. Med., 2020, 5(2), e10160 CrossRef CAS PubMed.
- Z. Zhang, Y. A. Lin, S. Y. Kim, L. Su, J. Liu and R. M. Kannan,
et al., Systemic dendrimer-drug nanomedicines for long-term treatment of mild-moderate cerebral palsy in a rabbit model, J. Neuroinflammation, 2020, 17, 319 CrossRef CAS PubMed.
- S. Fan, Y. Zheng, X. Liu, W. Fang, X. Chen and W. Liao,
et al., Curcumin-loaded PLGA-PEG nanoparticles conjugated with B6 peptide for potential use in Alzheimer's disease, Drug Delivery, 2018, 25(1), 1091–1102 CrossRef CAS PubMed.
- I. Schlachet, H. Moshe Halamish and A. Sosnik, Mixed Amphiphilic Polymeric Nanoparticles of Chitosan, Poly(vinyl alcohol) and Poly(methyl methacrylate) for Intranasal Drug Delivery: A Preliminary In Vivo Study, Molecules, 2020, 25(19), 4496 CrossRef CAS PubMed.
- R. Grillo, N. F. S. de Melo, D. R. de Araújo, E. de Paula, A. H. Rosa and L. F. Fraceto, Polymeric alginate nanoparticles containing the local anesthetic bupivacaine, J. Drug Targeting, 2010, 18(9), 688–699 CrossRef CAS PubMed.
- C. M. J. Hu, L. Zhang, S. Aryal, C. Cheung, R. H. Fang and L. Zhang, Erythrocyte membrane-camouflaged polymeric nanoparticles as a biomimetic delivery platform, Proc. Natl. Acad. Sci. U. S. A., 2011, 108(27), 10980–10985 CrossRef CAS PubMed.
- F. Fang, Y. Ni, H. Yu, H. Yin, F. Yang and C. Li,
et al., Inflammatory endothelium-targeted and cathepsin responsive nanoparticles are effective against atherosclerosis, Theranostics, 2022, 12(9), 4200–4220 CrossRef CAS PubMed.
- L. Palanikumar, S. Al-Hosani, M. Kalmouni, V. P. Nguyen, L. Ali and R. Pasricha,
et al., pH-responsive high stability polymeric nanoparticles for targeted delivery of anticancer therapeutics, Commun. Biol., 2020, 3(1), 1–17 CrossRef PubMed.
- L. Xu, M. Zhao, W. Gao, Y. Yang, J. Zhang and Y. Pu,
et al., Polymeric nanoparticles responsive to intracellular ROS for anticancer drug delivery, Colloids Surf., B, 2019, 181, 252–260 CrossRef CAS PubMed.
- B. Surnar, U. Basu, B. Banik, A. Ahmad, B. Marples and N. Kolishetti,
et al., Nanotechnology-mediated crossing of two impermeable membranes to modulate the stars of the neurovascular unit for neuroprotection, Proc. Natl. Acad. Sci. U. S. A., 2018, 115(52), E12333–E12342 CrossRef CAS PubMed.
- B. Surnar, A. S. Shah, M. Park, A. A. Kalathil, M. Z. Kamran and R. R. Jaime,
et al., Brain-Accumulating Nanoparticles for Assisting Astrocytes to Reduce Human Immunodeficiency Virus and Drug Abuse-Induced Neuroinflammation and Oxidative Stress, ACS Nano, 2021, 15(10), 15741–15753 CrossRef CAS PubMed.
- H. Akiyama, S. Jalloh, S. Park, M. Lei, G. Mostoslavsky and S. Gummuluru, Expression of HIV-1 Intron-Containing RNA in Microglia Induces Inflammatory Responses, J. Virol., 2021, 95(5), e01386-20, DOI:10.1128/JVI.01386-20.
- H. Peng, L. Sun, B. Jia, X. Lan, B. Zhu and Y. Wu,
et al., HIV-1-infected and immune-activated macrophages induce astrocytic differentiation of human cortical neural progenitor cells via the STAT3 pathway, PLoS One, 2011, 6(5), e19439 CrossRef CAS PubMed.
- M. Hoenigl, A. Chaillon, D. J. Moore, S. R. Morris, S. R. Mehta and S. Gianella,
et al., Rapid HIV Viral Load Suppression in those Initiating Antiretroviral Therapy at First Visit after HIV Diagnosis, Sci. Rep., 2016, 6, 32947 CrossRef CAS PubMed.
- H. S. Kim, M. J. Quon and J. A. Kim, New insights into the mechanisms of polyphenols beyond antioxidant properties; lessons from the green tea polyphenol, epigallocatechin 3-gallate, Redox Biol., 2014, 2, 187–195 CrossRef CAS PubMed.
- A. Chowdhury, J. Sarkar, T. Chakraborti, P. K. Pramanik and S. Chakraborti, Protective role of epigallocatechin-3-gallate in health and disease: A perspective, Biomed. Pharmacother., 2016, 78, 50–59 CrossRef CAS PubMed.
- R. de la Torre, S. de Sola, G. Hernandez, M. Farré, J. Pujol and J. Rodriguez,
et al., Safety and efficacy of cognitive training plus epigallocatechin-3-gallate in young adults with Down's syndrome (TESDAD): a double-blind, randomised, placebo-controlled, phase 2 trial, Lancet Neurol., 2016, 15(8), 801–810 CrossRef CAS PubMed.
- A. Cano, M. Ettcheto, M. Espina, C. Auladell, A. C. Calpena and J. Folch,
et al., Epigallocatechin-3-gallate loaded PEGylated-PLGA nanoparticles: A new anti-seizure strategy for temporal lobe epilepsy. Nanomedicine, Nanotechnol. Biol. Med., 2018, 14(4), 1073–1085 CrossRef CAS PubMed.
- A. Firdous, S. Sarwar, F. A. Shah, S. Tabasum, A. Zeb and H. Nadeem,
et al., Contribution of Attenuation of TNF-α and NF-κB in the Anti-Epileptic, Anti-Apoptotic and Neuroprotective Potential of Rosa webbiana Fruit and Its Chitosan Encapsulation, Molecules, 2021, 26(8), 2347 CrossRef CAS PubMed.
- N. Zhao, N. L. Francis, S. Song, V. Kholodovych, H. R. Calvelli and C. L. Hoop,
et al., CD36-Binding Amphiphilic Nanoparticles for Attenuation of Alpha Synuclein-Induced Microglial Activation, Adv. NanoBiomed Res., 2022, 2(6), 2100120 CrossRef CAS PubMed.
- T. M. Don, W. J. Chang, P. R. Jheng, Y. C. Huang and E. Y. Chuang, Curcumin-laden dual-targeting fucoidan/chitosan nanocarriers for inhibiting brain inflammation via intranasal delivery, Int. J. Biol. Macromol., 2021, 181, 835–846 CrossRef CAS PubMed.
- M. Jafari, V. Sriram, Z. Xu, G. M. Harris and J. Y. Lee, Fucoidan-Doxorubicin Nanoparticles Targeting P-Selectin for Effective Breast Cancer Therapy, Carbohydr. Polym., 2020, 249, 116837 CrossRef CAS PubMed.
- E. Yeini, P. Ofek, S. Pozzi, N. Albeck, D. Ben-Shushan and G. Tiram,
et al., P-selectin axis plays a key role in microglia immunophenotype and glioblastoma progression, Nat. Commun., 2021, 12(1), 1912 CrossRef CAS PubMed.
- U. M. Chandrasekharan, M. Siemionow, M. Unsal, L. Yang, E. Poptic and J. Bohn,
et al., Tumor necrosis factor α (TNF-α) receptor-II is required for TNF-α–induced leukocyte-endothelial interaction in vivo, Blood, 2007, 109(5), 1938–1944 CrossRef CAS PubMed.
- J. Li and K. Pu, Semiconducting Polymer Nanomaterials as Near-Infrared Photoactivatable Protherapeutics for Cancer, Acc. Chem. Res., 2020, 53(4), 752–762 CrossRef CAS PubMed.
- N. S. Rejinold, G. Choi and J. H. Choy, Recent Developments on Semiconducting Polymer Nanoparticles as Smart Photo-Therapeutic Agents for Cancer Treatments—A Review, Polymers, 2021, 13(6), 981 CrossRef CAS PubMed.
- B. Li, N. Li, L. Chen, S. Ren, D. Gao and H. Geng,
et al., Alleviating Neuroinflammation through Photothermal Conjugated Polymer Nanoparticles by Regulating Reactive Oxygen Species and Ca2+ Signaling, ACS Appl. Mater. Interfaces, 2022, 14(43), 48416–48425 CrossRef CAS PubMed.
- X. Li, X. Xu, M. Xu, Z. Geng, P. Ji and Y. Liu, Hydrogel systems for targeted cancer therapy, Front. Bioeng. Biotechnol., 2023, 11, 1140436 CrossRef PubMed.
- Y. Zhang, Z. Zou, S. Liu, S. Miao and H. Liu, Nanogels as Novel Nanocarrier Systems for Efficient Delivery of CNS Therapeutics, Front. Bioeng. Biotechnol., 2022, 10, 954470 CrossRef PubMed.
- C. S. A. de Lima, T. S. Balogh, J. P. R. O. Varca, G. H. C. Varca, A. B. Lugão, A. Camacho-Cruz, E. Bucio and S. S. Kadlubowski,
et al., An Updated Review of Macro, Micro, and Nanostructured Hydrogels for Biomedical and Pharmaceutical Applications, Pharmaceutics, 2020, 12(10), 970 CrossRef CAS PubMed.
- S. Al Harthi, S. E. Alavi, M. A. Radwan, M. M. El Khatib and I. A. AlSarra, Nasal delivery of donepezil HCl-loaded hydrogels for the treatment of Alzheimer's disease, Sci. Rep., 2019, 9(1), 9563 CrossRef PubMed.
- Q. S. Wang, K. Li, L. N. Gao, Y. Zhang, K. M. Lin and Y. L. Cui, Intranasal delivery of berberine via in situ thermoresponsive hydrogels with non-invasive therapy exhibits better antidepressant-like effects, Biomater. Sci., 2020, 8(10), 2853–2865 RSC.
- R. Taléns-Visconti, J. V. de Julián-Ortiz, O. Vila-Busó, O. Diez-Sales and A. Nácher, Intranasal Drug Administration in Alzheimer-Type Dementia: Towards Clinical Applications, Pharmaceutics, 2023, 15(5), 1399 CrossRef PubMed.
- E. Hasanzadeh, A. Seifalian, A. Mellati, J. Saremi, S. Asadpour and S. E. Enderami,
et al., Injectable hydrogels in central nervous system: Unique and novel platforms for promoting extracellular matrix remodeling and tissue engineering, Mater. Today Bio, 2023, 20, 100614 CrossRef CAS PubMed.
- G. Jensen, J. L. Holloway and S. E. Stabenfeldt, Hyaluronic Acid Biomaterials for Central Nervous System Regenerative Medicine, Cells, 2020, 9(9), E2113 CrossRef PubMed.
- E. Bellotti, A. L. Schilling, S. R. Little and P. Decuzzi, Injectable thermoresponsive hydrogels as drug delivery system for the treatment of central nervous system disorders: A review, J. Controlled Release, 2021, 329, 16–35 CrossRef CAS PubMed.
- Q. Li, X. Shao, X. Dai, Q. Guo, B. Yuan and Y. Liu,
et al., Recent trends in the development of hydrogel therapeutics for the treatment of central nervous system disorders, NPG Asia Mater., 2022, 14(1), 1–14 CrossRef.
- D. W. Nelson and R. J. Gilbert, Extracellular Matrix-Mimetic Hydrogels for Treating Neural Tissue Injury: A Focus on Fibrin, Hyaluronic Acid, and Elastin-Like Polypeptide Hydrogels, Adv. Healthcare Mater., 2021, 10(22), e2101329 CrossRef PubMed.
- P. Hen Lin, Q. Dong and S. Yian Chew, Injectable hydrogels in stroke and spinal cord injury treatment: a review on hydrogel materials, cell–matrix interactions and glial involvement, Mater. Adv., 2021, 2(8), 2561–2583 RSC.
- H. Shen, B. Xu, C. Yang, W. Xue, Z. You and X. Wu,
et al., A DAMP-scavenging, IL-10-releasing hydrogel promotes neural regeneration and motor function recovery after spinal cord injury, Biomaterials, 2022, 280, 121279 CrossRef CAS PubMed.
- A. Tuladhar, J. M. Obermeyer, S. L. Payne, R. C. W. Siu, S. Zand and C. M. Morshead,
et al., Injectable hydrogel enables local and sustained co-delivery to the brain: Two clinically approved biomolecules, cyclosporine and erythropoietin, accelerate functional recovery in rat model of stroke, Biomaterials, 2020, 235, 119794 CrossRef CAS PubMed.
- G. Jensen, J. L. Holloway and S. E. Stabenfeldt, Hyaluronic Acid Biomaterials for Central Nervous System Regenerative Medicine, Cells, 2020, 9(9), 2113 CrossRef CAS PubMed.
- P. Casini, I. Nardi and M. Ori, RHAMM mRNA expression in proliferating and migrating cells of the developing central nervous system, Gene Expression Patterns, 2010, 10(2), 93–97 CrossRef CAS PubMed.
- Z. Z. Khaing and S. K. Seidlits, Hyaluronic acid and neural stem cells: implications for biomaterial design, J. Mater. Chem. B, 2015, 3(40), 7850–7866 RSC.
- D. V. Chistyakov, A. A. Astakhova, N. V. Azbukina, S. V. Goriainov, V. V. Chistyakov and M. G. Sergeeva, High and Low Molecular Weight Hyaluronic Acid Differentially Influences Oxylipins Synthesis in Course of Neuroinflammation, Int. J. Mol. Sci., 2019, 20(16), 3894 CrossRef CAS PubMed.
- Z. Z. Khaing, B. D. Milman, J. E. Vanscoy, S. K. Seidlits, R. J. Grill and C. E. Schmidt, High molecular weight hyaluronic acid limits astrocyte activation and scar formation after spinal cord injury, J. Neural Eng., 2011, 8(4), 046033 CrossRef PubMed.
- S. Salathia, M. R. Gigliobianco, C. Casadidio, P. Di Martino and R. Censi, Hyaluronic Acid-Based Nanosystems for CD44 Mediated Anti-Inflammatory and Antinociceptive Activity, Int. J. Mol. Sci., 2023, 24(8), 7286 CrossRef CAS PubMed.
- L. Wang, J. Li, D. Zhang, S. Ma, J. Zhang and F. Gao,
et al., Dual-enzymatically crosslinked and injectable hyaluronic acid hydrogels for potential application in tissue engineering, RSC Adv., 2020, 10(5), 2870–2876 RSC.
- L. Wang, D. Zhang, Y. Ren, S. Guo, J. Li and S. Ma,
et al., Injectable hyaluronic acid hydrogel loaded with BMSC and NGF for traumatic brain injury treatment, Mater. Today Bio, 2022, 13, 100201 CrossRef CAS PubMed.
- C. W. Zeng, Multipotent Mesenchymal Stem Cell-Based Therapies for Spinal Cord Injury: Current Progress and Future Prospects, Biology, 2023, 12(5), 653 CrossRef CAS PubMed.
- V. Delplace, A. J. Pickering, M. H. Hettiaratchi, S. Zhao, T. Kivijärvi and M. S. Shoichet, Inverse electron-demand Diels–Alder methylcellulose hydrogels enable the Co-delivery of chondroitinase ABC and neural progenitor cells, Biomacromolecules, 2020, 21(6), 2421–2431 CrossRef CAS PubMed.
- M. T. Ho, C. J. Teal and M. S. Shoichet, A hyaluronan/methylcellulose-based hydrogel for local cell and biomolecule delivery to the central nervous system, Brain Res. Bull., 2019, 148, 46–54 CrossRef CAS PubMed.
- M. M. Pakulska, K. Vulic and M. S. Shoichet, Affinity-based release of chondroitinase ABC from a modified methylcellulose hydrogel, J. Controlled Release, 2013, 171(1), 11–16 CrossRef CAS PubMed.
- R. Y. Tam, M. J. Cooke and M. S. Shoichet, A covalently modified hydrogel blend of hyaluronan–methyl cellulose with peptides and growth factors influences neural stem/progenitor cell fate, J. Mater. Chem., 2012, 22(37), 19402–19411 RSC.
- R. L. Bartlett, S. Sharma and A. Panitch, Cell-penetrating peptides released from thermosensitive nanoparticles suppress pro-inflammatory cytokine response by specifically targeting inflamed cartilage explants, Nanomedicine, 2013, 9(3), 419–427 CrossRef CAS PubMed.
- X. Q. Zheng, J. F. Huang, J. L. Lin, Y. X. Zhu, M. Q. Wang and M. L. Guo,
et al., Controlled release of baricitinib from a thermos-responsive hydrogel system inhibits inflammation by suppressing JAK2/STAT3 pathway in acute spinal cord injury, Colloids Surf., B, 2021, 199, 111532 CrossRef CAS PubMed.
- A. Cunha, A. Gaubert, L. Latxague and B. Dehay, PLGA-Based Nanoparticles for Neuroprotective Drug Delivery in Neurodegenerative Diseases, Pharmaceutics, 2021, 13(7), 1042 CrossRef CAS PubMed.
- E. Ho, Y. Deng, D. Akbar, K. Da, M. Létourneau and C. M. Morshead,
et al., Tunable Surface Charge Enables the Electrostatic Adsorption-Controlled Release of Neuroprotective Peptides from a Hydrogel–Nanoparticle Drug Delivery System, ACS Appl. Mater. Interfaces, 2023, 15(1), 91–105 CrossRef CAS PubMed.
- Y. Liu, G. Yang, S. Jin, L. Xu and C. X. Zhao, Development of High-Drug-Loading Nanoparticles, ChemPlusChem, 2020, 85(9), 2143–2157 CrossRef CAS PubMed.
- S. Shen, Y. Wu, Y. Liu and D. Wu, High drug-loading nanomedicines: progress, current status, and prospects, Int. J. Nanomed., 2017, 12, 4085–4109 CrossRef CAS PubMed.
- H. S. Choi, W. Liu, P. Misra, E. Tanaka, J. P. Zimmer and B. I. Ipe,
et al., Renal Clearance of Nanoparticles, Nat. Biotechnol., 2007, 25(10), 1165–1170 CrossRef CAS PubMed.
- M. Settles, M. Etzrodt, K. Kosanke, M. Schiemann, A. Zimmermann and R. Meier,
et al., Different Capacity of Monocyte Subsets to Phagocytose Iron-Oxide Nanoparticles, PLoS One, 2011, 6(10), e25197 CrossRef CAS PubMed.
- B. S. Zolnik, Á. González-Fernández, N. Sadrieh and M. A. Dobrovolskaia, Nanoparticles and the Immune System, Endocrinology, 2010, 151(2), 458–465 CrossRef CAS PubMed.
- Z. Wang, E. D. Hood, J. Nong, J. Ding, O. A. Marcos-Contreras and P. M. Glassman,
et al., Combating complement's deleterious effects on nanomedicine by conjugating complement regulatory proteins to nanoparticles, Adv. Mater., 2022, 34(8), e2107070 CrossRef PubMed.
- J. Li and D. J. Mooney, Designing hydrogels for controlled drug delivery, Nat. Rev. Mater., 2016, 1(12), 16071 CrossRef CAS PubMed.
- X. Huang and C. S. Brazel, On the importance and mechanisms of burst release in matrix-controlled drug delivery systems, J. Controlled Release, 2001, 73(2–3), 121–136 CrossRef CAS PubMed.
- Y. Chehreghanianzabi, R. Barua, T. Shi, S. Yurgelevic, G. Auner and D. C. Markel,
et al., Comparing the release of erythromycin and vancomycin from calcium polyphosphate hydrogel using different drug loading methods, J. Biomed. Mater. Res., Part B, 2020, 108(2), 475–483 CrossRef CAS PubMed.
- M. McKenzie, D. Betts, A. Suh, K. Bui, L. D. Kim and H. Cho, Hydrogel-Based Drug Delivery Systems for Poorly Water-Soluble Drugs, Molecules, 2015, 20(11), 20397–20408 CrossRef CAS PubMed.
- E. Ansorena, P. De Berdt, B. Ucakar, T. Simón-Yarza, D. Jacobs and O. Schakman,
et al., Injectable alginate hydrogel loaded with GDNF promotes functional recovery in a hemisection model of spinal cord injury, Int. J. Pharm., 2013, 455(1), 148–158 CrossRef CAS PubMed.
- F. Xue, T. Liu, X. Liu, K. Chen, L. Duan and G. Gao, Electroconductive and porous graphene-xanthan gum gel scaffold for spinal cord regeneration, Eur. Polym. J., 2022, 173, 111225 CrossRef CAS.
-
I. Dryg, Modulating Neuroinflammation with Porous Templated Scaffolds, PhD thesis, University of Washington, 2019.
- Ice-Templated Scaffolds with Microridged Pores Direct DRG Neurite Growth – Riblett – 2012 – Advanced Functional Materials – Wiley Online Library [Internet]. [cited 2024 Feb 9]. Available from: https://onlinelibrary.wiley.com/doi/10.1002/adfm.201201323.
- K. A. Tran, B. J. DeOre, D. Ikejiani, K. Means, L. S. Paone and L. De Marchi,
et al., Matching mechanical heterogeneity of the native spinal cord augments axon infiltration in 3D-printed scaffolds, Biomaterials, 2023, 295, 122061 CrossRef CAS PubMed.
- K. K. Moncal, R. S. Tigli Aydın, K. P. Godzik, T. M. Acri, D. N. Heo and E. Rizk,
et al., Controlled Co-delivery of pPDGF-B and pBMP-2 from intraoperatively bioprinted bone constructs improves the repair of calvarial defects in rats, Biomaterials, 2022, 281, 121333 CrossRef CAS PubMed.
- W. Zhuge, H. Liu, W. Wang and J. Wang, Microfluidic bioscaffolds for regenerative engineering, Eng. Regen., 2022, 3(1), 110–120 Search PubMed.
- M. Zhao, H. Liu, X. Zhang, H. Wang, T. Tao and J. Qin, A flexible microfluidic strategy to generate grooved microfibers for guiding cell alignment, Biomater. Sci., 2021, 9(14), 4880–4890 RSC.
- Y. H. Ma, H. J. Shi, Q. S. Wei, Q. W. Deng, J. H. Sun and Z. Liu,
et al., Developing a mechanically matched decellularized spinal cord scaffold for the in situ matrix-based neural repair of spinal cord injury, Biomaterials, 2021, 279, 121192 CrossRef CAS PubMed.
- A. E. C. Granato, E. F. da Cruz, D. M. Rodrigues-Junior, A. C. Mosini, H. Ulrich and B. V. M. Rodrigues,
et al., A novel decellularization method to produce brain scaffolds, Tissue Cell, 2020, 67, 101412 CrossRef CAS PubMed.
- J. P. M. Sousa, E. Stratakis, J. Mano and P. A. A. P. Marques, Anisotropic 3D scaffolds for spinal cord guided repair: Current concepts, Biomater. Adv., 2023, 148, 213353 CrossRef CAS PubMed.
- J. Zhang, X. Liu, K. Ma, M. Chen, H. Xu and X. Niu,
et al., Collagen/heparin scaffold combined with vascular endothelial growth factor promotes the repair of neurological function in rats with traumatic brain injury, Biomater. Sci., 2021, 9(3), 745–764 RSC.
- J. Ma, J. Li, X. Wang, M. Li, W. Teng and Z. Tao,
et al., GDNF-Loaded Polydopamine Nanoparticles-Based Anisotropic Scaffolds Promote Spinal Cord Repair by Modulating Inhibitory Microenvironment, Adv. Healthcare Mater., 2023, 12(8), 2202377 CrossRef CAS PubMed.
- S. Ma, X. Feng, F. Liu, B. Wang, H. Zhang and X. Niu, The pro-inflammatory response of macrophages regulated by acid degradation products of poly(lactide-co-glycolide) nanoparticles, Eng. Life Sci., 2021, 21(10), 709–720 CrossRef CAS PubMed.
- G. Zhang, A. Zhen, J. Chen, B. Du, F. Luo and J. Li,
et al., In Vitro Effects of Waterborne Polyurethane 3D Scaffolds Containing Poly(lactic-co-glycolic acid)s of Different Lactic Acid/Glycolic Acid Ratios on the Inflammatory Response, Polymers, 2023, 15(7), 1786 CrossRef CAS PubMed.
- B. Du, H. Yin, Y. Chen, W. Lin, Y. Wang and D. Zhao,
et al., A waterborne polyurethane 3D scaffold containing PLGA with a controllable degradation rate and an anti-inflammatory effect for potential applications in neural tissue repair, J. Mater. Chem. B, 2020, 8(20), 4434–4446 RSC.
- H. Hao, J. Shao, Y. Deng, S. He, F. Luo and Y. Wu,
et al., Synthesis and characterization of biodegradable lysine-based waterborne polyurethane for soft tissue engineering applications, Biomater. Sci., 2016, 4(11), 1682–1690 RSC.
- X. Liu, J. Wang, P. Wang, L. Zhong, S. Wang and Q. Feng,
et al., Hypoxia-pretreated mesenchymal stem cell-derived exosomes-loaded low-temperature extrusion 3D-printed implants for neural regeneration after traumatic brain injury in canines, Front. Bioeng. Biotechnol., 2022, 10, 1025138 CrossRef PubMed.
- X. Liu, G. Zhang, P. Wei, L. Zhong, Y. Chen and J. Zhang,
et al., Three-dimensional-printed collagen/chitosan/secretome derived from HUCMSCs scaffolds for efficient neural network reconstruction in canines with traumatic brain injury, Regener. Biomater., 2022, 9, rbac043 CrossRef CAS PubMed.
- Y. Li, L. Yang, Y. Hou, Z. Zhang, M. Chen and M. Wang,
et al., Polydopamine-mediated graphene oxide and nanohydroxyapatite-incorporated conductive scaffold with an immunomodulatory ability accelerates periodontal bone regeneration in diabetes, Bioact. Mater., 2022, 18, 213–227 CAS.
- Z. Liu, Q. Deng, G. Qin, J. Yang, H. Zhang and J. Ren,
et al., Biomarker-activated multifunctional lysosome-targeting chimeras mediated selective degradation of extracellular amyloid fibrils, Chem, 2023, 9(7), 2016–2038 CAS.
- T. T. Zhu, H. Wang, H. W. Gu, L. S. Ju, X. M. Wu and W. T. Pan,
et al., Melanin-like polydopamine nanoparticles mediating anti-inflammatory and rescuing synaptic loss for inflammatory depression therapy, J. Nanobiotechnol., 2023, 21(1), 52 CrossRef CAS PubMed.
- R. J. Vetter, J. C. Williams, J. F. Hetke, E. A. Nunamaker and D. R. Kipke, Chronic neural recording using silicon-substrate microelectrode arrays implanted in cerebral cortex, IEEE Trans. Biomed. Eng., 2004, 51(6), 896–904 CrossRef PubMed.
- A. G. P. Schjetnan and A. Luczak, Recording Large-scale Neuronal Ensembles with Silicon Probes in the Anesthetized Rat, J. Visualized Exp., 2011, 56, 3282 Search PubMed.
- F. Barz, V. Trouillet, O. Paul and P. Ruther, CMOS-Compatible, Flexible, Intracortical Neural Probes, IEEE Trans. Biomed. Eng., 2020, 67(5), 1366–1376 Search PubMed.
- K. Joseph, M. Kirsch, M. Johnston, C. Münkel, T. Stieglitz and C. A. Haas,
et al., Transcriptional characterization of the glial response due to chronic neural implantation of flexible microprobes, Biomaterials, 2021, 279, 121230 CrossRef CAS PubMed.
- H. C. Lee, J. Gaire, S. W. Currlin, M. D. McDermott, K. Park and K. J. Otto, Foreign Body Response to Intracortical Microelectrodes Is Not Altered with Dip-Coating of Polyethylene Glycol (PEG), Front. Neurosci., 2017, 11, 513 CrossRef PubMed.
- N. Sharafkhani, A. Z. Kouzani, S. D. Adams, J. M. Long, G. Lissorgues and L. Rousseau,
et al., Neural tissue-microelectrode interaction: Brain micromotion, electrical impedance, and flexible microelectrode insertion, J. Neurosci. Methods, 2022, 365, 109388 CrossRef PubMed.
- J. Duncan, A. Sridharan, S. S. Kumar, D. Iradukunda and J. Muthuswamy, Biomechanical micromotion at the neural interface modulates intracellular membrane potentials in vivo, J. Neural Eng., 2021, 18(4), 045010 CrossRef PubMed.
- S. Song, L. N. Druschel, E. R. Chan and J. R. Capadona, Differential expression of genes involved in the chronic response to intracortical microelectrodes, Acta Biomater., 2023, 169, 348–362 CrossRef CAS PubMed.
- X. Dong, Y. Ma, G. Zhu, Y. Huang, J. Wang and M. B. Chan-Park,
et al., Synthesis of graphene–carbon nanotube hybrid foam and its use as a novel three-dimensional electrode for electrochemical sensing, J. Mater. Chem., 2012, 22(33), 17044 RSC.
- G. G. Wallace, J. Chen, D. Li, S. E. Moulton and J. M. Razal, Nanostructured carbon electrodes, J. Mater. Chem., 2010, 20(18), 3553 RSC.
- M. Ryu, J. H. Yang, Y. Ahn, M. Sim, K. H. Lee and K. Kim,
et al., Enhancement of Interface Characteristics of Neural Probe Based on Graphene, ZnO Nanowires, and Conducting Polymer PEDOT, ACS Appl. Mater. Interfaces, 2017, 9(12), 10577–10586 CrossRef CAS PubMed.
- O. Akhavan and E. Ghaderi, The use of graphene in the self-organized differentiation of human neural stem cells into neurons under pulsed laser stimulation, J. Mater. Chem. B, 2014, 2(34), 5602–5611 RSC.
- N. P. Pampaloni, M. Lottner, M. Giugliano, A. Matruglio, F. D’Amico and M. Prato,
et al., Single-layer graphene modulates neuronal communication and augments membrane ion currents, Nat. Nanotechnol., 2018, 13(8), 755–764 CrossRef CAS PubMed.
- C. M. Girish, A. Sasidharan, G. S. Gowd, S. Nair and M. Koyakutty, Confocal Raman imaging study showing macrophage mediated biodegradation of graphene in vivo, Adv. Healthcare Mater., 2013, 2(11), 1489–1500 CrossRef CAS PubMed.
- R. Kurapati, C. Martìn, V. Palermo, Y. Nishina and A. Bianco, Biodegradation of graphene materials catalyzed by human eosinophil peroxidase, Faraday Discuss., 2021, 227, 189–203 RSC.
- R. Kurapati, J. Russier, M. A. Squillaci, E. Treossi, C. Ménard-Moyon and A. E. Del Rio-Castillo,
et al., Dispersibility-Dependent Biodegradation of Graphene Oxide by Myeloperoxidase, Small, 2015, 11(32), 3985–3994 CrossRef CAS PubMed.
- A. Khademhosseini, K. Y. Suh, J. M. Yang, G. Eng, J. Yeh and S. Levenberg,
et al., Layer-by-layer deposition of hyaluronic acid and poly-L-lysine for patterned cell co-cultures, Biomaterials, 2004, 25(17), 3583–3592 CrossRef CAS PubMed.
- D. Mazia, G. Schatten and W. Sale, Adhesion of cells to surfaces coated with polylysine. Applications to electron microscopy, J. Cell Biol., 1975, 66(1), 198–200 CrossRef CAS PubMed.
- A. Faussner, M. M. Deininger, C. Weber and S. Steffens, Direct addition of poly-lysine or poly-ethylenimine to the medium: A simple alternative to plate pre-coating, PLoS One, 2022, 17(7), e0260173 CrossRef CAS PubMed.
- A. Gökaltun, Y. B. Kang (Abraham), M. L. Yarmush, O. B. Usta and A. Asatekin, Simple Surface Modification of Poly(dimethylsiloxane) via Surface Segregating Smart Polymers for Biomicrofluidics, Sci. Rep., 2019, 9(1), 7377 CrossRef PubMed.
- K. C. Spencer, J. C. Sy, K. B. Ramadi, A. M. Graybiel, R. Langer and M. J. Cima, Characterization of Mechanically Matched Hydrogel Coatings to Improve the Biocompatibility of Neural Implants, Sci. Rep., 2017, 7(1), 1952 CrossRef PubMed.
- L. Frey, P. Bandaru, Y. S. Zhang, K. O’Kelly, A. Khademhosseini and S. R. Shin, A Dual-layered Microfluidic System for Long-term Controlled In Situ Delivery of Multiple Anti-inflammatory Factors for Chronic Neural Applications, Adv. Funct. Mater., 2018, 28(12), 1702009 CrossRef PubMed.
- E. Stewart, N. R. Kobayashi, M. J. Higgins, A. F. Quigley, S. Jamali and S. E. Moulton,
et al., Electrical stimulation using conductive polymer polypyrrole promotes differentiation of human neural stem cells: a biocompatible platform for translational neural tissue engineering, Tissue Eng., Part C, 2015, 21(4), 385–393 CrossRef CAS PubMed.
- K. H. Sun, Z. Liu, C. Liu, T. Yu, T. Shang and C. Huang,
et al., Evaluation of in vitro and in vivo biocompatibility of a myo-inositol hexakisphosphate gelated polyaniline hydrogel in a rat model, Sci. Rep., 2016, 6, 23931 CrossRef CAS PubMed.
- L. Pavone, S. Moyanova, F. Mastroiacovo, L. Fazi, C. Busceti and A. Gaglione,
et al., Chronic neural interfacing with cerebral cortex using single-walled carbon nanotube-polymer grids, J. Neural Eng., 2020, 17(3), 036032 CrossRef PubMed.
- L. Lu, X. Fu, Y. Liew, Y. Zhang, S. Zhao and Z. Xu,
et al., Soft and MRI Compatible Neural Electrodes from Carbon Nanotube Fibers, Nano Lett., 2019, 19(3), 1577–1586 CrossRef CAS PubMed.
-
H. Zhu Safety and Feasibility of Stromal Vascular Fraction (SVF) Combined With Functional Self-assembling Peptide Nanofiber Hydrogels in the Treatment of Spinal Cord Injury [Internet]. clinicaltrials.gov, 2023 Jul [cited 2023 Sep 1]. Report No.: NCT05967325, Available from: https://clinicaltrials.gov/study/NCT05967325.
- H. Zhai, J. Zhou, J. Xu, X. Sun, Y. Xu and X. Qiu,
et al., Mechanically strengthened hybrid peptide-polyester hydrogel and potential applications in spinal cord injury repair, Biomed. Mater., 2020, 15(5), 055031 CrossRef CAS PubMed.
- Aphios. Safety, Tolerability and Efficacy Assessment of Intranasal Nanoparticles of APH-1105, A Novel Alpha Secretase Modulator For Mild to Moderate Cognitive Impairment Due to Alzheimer's Disease(AD) [Internet]. clinicaltrials.gov, 2021 Jul [cited 2023 Sep 12], Report No.: NCT03806478, Available from: https://clinicaltrials.gov/study/NCT03806478.
- X. Huang, D. He, Z. Pan, G. Luo and J. Deng, Reactive-oxygen-species-scavenging nanomaterials for resolving inflammation, Mater. Today Bio, 2021, 11, 100124 CrossRef CAS PubMed.
- G. Agarwal, A. Roy, H. Kumar and A. Srivastava, Graphene-collagen cryogel controls neuroinflammation and fosters accelerated axonal regeneration in spinal cord injury, Biomater. Adv., 2022, 139, 212971 CrossRef CAS PubMed.
- T. L. Rapp and C. A. DeForest, Visible Light-Responsive Dynamic Biomaterials: Going Deeper and Triggering More, Adv. Healthcare Mater., 2020, 9(7), e1901553 CrossRef PubMed.
- T. L. Rapp and C. A. DeForest, Tricolor visible wavelength-selective photodegradable hydrogel biomaterials, Nat. Commun., 2023, 14(1), 5250 CrossRef CAS PubMed.
- M. Clement, G. Daniel and M. Trelles, Optimising the design of a broad-band light source for the treatment of skin, J. Cosmet. Laser Ther., 2005, 7(3–4), 177–189 CrossRef PubMed.
- C. Ash, M. Dubec, K. Donne and T. Bashford, Effect of wavelength and beam width on penetration in light-tissue interaction using computational methods, Lasers Med. Sci., 2017, 32(8), 1909–1918 CrossRef PubMed.
- M. A. Anderson, T. M. O’Shea, J. E. Burda, Y. Ao, S. L. Barlatey and A. M. Bernstein,
et al., Required growth facilitators propel axon regeneration across complete spinal cord injury, Nature, 2018, 561(7723), 396–400 CrossRef CAS PubMed.
- J. Zhong, J. Xu, S. Lu, Z. Wang, Y. Zheng and Q. Tang,
et al., A Prevascularization Strategy Using Novel Fibrous Porous Silk Scaffolds for Tissue Regeneration in Mice with Spinal Cord Injury, Stem Cells Dev., 2020, 29(9), 615–624 CrossRef CAS PubMed.
- S. Guo, I. Redenski, S. Landau, A. Szklanny, U. Merdler and S. Levenberg, Prevascularized Scaffolds Bearing Human Dental Pulp Stem Cells for Treating Complete Spinal Cord Injury, Adv. Healthcare Mater., 2020, 9(20), e2000974 CrossRef PubMed.
- K. A. Tran, P. P. Partyka, Y. Jin, J. Bouyer, I. Fischer and P. A. Galie, Vascularization of self-assembled peptide scaffolds for spinal cord injury repair, Acta Biomater., 2020, 104, 76–84 CrossRef CAS PubMed.
-
J. Dai Safety and Efficacy of Functional Neural Regeneration Collagen Scaffold Transplantation in Complete (AISA) Acute Spinal Cord Injury Patients [Internet]. clinicaltrials.gov, 2020 Dec [cited 2023 Sep 1]. Report No.: NCT02510365, Available from: https://clinicaltrials.gov/study/NCT02510365.
-
J. Dai Safety and Efficacy of Functional Neural Regeneration Collagen Scaffold Transplantation Combined With Epidural Electrical Stimulation for Spinal Cord Injury Repair [Internet], clinicaltrials.gov, 2019 Oct [cited 2023 Sep 1]. Report No.: NCT03966794, Available from: https://clinicaltrials.gov/study/NCT03966794.
-
J. Dai NeuroRegen ScaffoldTM With Bone Marrow Mononuclear Cells Transplantation vs. Intradural Decompression and Adhesiolysis in Patients With Chronic Spinal Cord Injury [Internet], clinicaltrials.gov, 2020 Dec [cited 2023 Sep 1], Report No.: NCT02688062, Available from: https://clinicaltrials.gov/study/NCT02688062.
-
InVivo Therapeutics. Randomized, Controlled, Single-blind Study of Probable Benefit of the Neuro-Spinal ScaffoldTM for Safety and Neurologic Recovery in Subjects With Complete Thoracic AIS A Spinal Cord Injury as Compared to Standard of Care [Internet], clinicaltrials.gov; 2022 Jun [cited 2023 Sep 1], Report No.: NCT03762655, Available from: https://clinicaltrials.gov/study/NCT03762655.
- D. K. Cullen, M. D. Tang-Schomer, L. A. Struzyna, A. R. Patel, V. E. Johnson and J. A. Wolf,
et al., Microtissue Engineered Constructs with Living Axons for Targeted Nervous System Reconstruction, Tissue Eng., Part A, 2012, 18(21–22), 2280–2289 CrossRef CAS PubMed.
- D. O. Adewole, L. A. Struzyna, J. C. Burrell, J. P. Harris, A. D. Nemes and D. Petrov,
et al., Development of optically controlled “living electrodes” with long-projecting axon tracts for a synaptic brain–machine interface, Sci. Adv., 2021, 7(4), eaay5347 CrossRef CAS PubMed.
Footnote |
† These authors contributed equally to this work. |
|
This journal is © The Royal Society of Chemistry 2024 |