DOI:
10.1039/D3MA00682D
(Review Article)
Mater. Adv., 2024,
5, 1364-1394
Hydrogel-based dressings designed to facilitate wound healing
Received
8th September 2023
, Accepted 18th December 2023
First published on 20th December 2023
Abstract
The escalating prevalence of wounds, particularly chronic wounds, poses significant challenges in terms of treatment management and has led to an increase in healthcare expenditure. Hydrogel-based dressings play a crucial role in wound management due to their unique properties. However, there remains a lack of comprehensive reviews on the use of hydrogel-based dressings for wound healing. This review commences with an overview of the process and characteristics of wound healing. Furthermore, we summarize the various functions of hydrogel-based dressings in promoting wound healing, including adhesive property, antibacterial efficacy, hemostasis, angiogenesis promotion, anti-oxidation, anti-inflammatory effect, self-healing, and conductivity. We further discuss the challenges encountered during the healing of different types of wounds, particularly diabetic wounds, and outline the latest advancements in the application of hydrogel-based dressings in the management of different types of wounds. In addition, we conclude and provide an outlook on the application of hydrogel-based dressings in wound healing, with the aim of further enhancing their ability to promote wound healing.
1. Introduction
The human skin, the largest organ in the body, serves as a remarkable protective barrier that separates the internal environment from the external surroundings. With its multifunctional nature, the skin plays crucial roles in moisturization, thermoregulation, sensory perception, humoral equilibrium, and defense against pathogens.1 However, prolonged exposure to various external factors can impose a significant burden on this vital organ, making it susceptible to a wide range of stimuli. When the integrity of the skin is compromised, such as through the formation of wounds, it becomes a predisposing factor for the development of various diseases.2 The formation of wounds can result in significant discomfort and pain, with severe cases potentially leading to disability.3 In accordance with the duration of wound healing, wounds can be categorized as acute or chronic. Acute wounds are characterized by skin damage resulting from causes such as surgical incisions or bites, and they exhibit the capacity for self-healing through normal and predictable processes, even in the absence of external intervention. The typical healing time for acute wounds is approximately 8 to 12 weeks.4 Chronic wounds, on the other hand, are characterized by an inability to complete the normal healing process within 12 weeks or a prolonged timeframe, often exhibiting recurrent tendencies.5 Common causes of these chronic wounds include untreated or improperly treated acute traumas. Chronic wounds associated with chronic diseases such as diabetes and vascular disorders present a greater challenge in terms of treatment and management, primarily due to the complexity of underlying pathological mechanisms.6,7 Chronic wounds fail to undergo normal healing processes, resulting in prolonged inflammation, insufficient angiogenesis, and severe complications.8 The surge in chronic wounds presents substantial challenges in terms of therapeutic management and contributes to a significant increase in healthcare costs.9 Consequently, the pursuit of straightforward and effective wound treatments remains a formidable challenge, posing significant dilemmas for the healthcare system.
Wound healing is a complex process that involves multiple phases and requires appropriate management, particularly in the case of chronic wounds. Upon the occurrence of a wound, appropriate wound care plays a pivotal role in preventing bacterial infections and expediting the healing process. Bandaging is the most basic treatment in clinic.10 Various treatment modalities are available for wound management, and while invasive surgical interventions like debridement and skin grafting can be effective, they carry risks such as bleeding, tissue injury, and prolonged recovery periods.11 In contrast, wound dressings assume a pivotal role in wound management by offering a protective barrier that safeguards the wound against external infections.12 Furthermore, they serve as scaffolds that facilitate the reorganization of skin cells, thereby fostering the infiltration and integration of host tissues into the wound bed.13,14 The choice of wound dressing can have a significant impact on the wound healing process. Conventional wound dressings, including gauze, cotton, and bandages, are designed to prevent external contamination of the wound. However, they fail to maintain a moist wound environment.15 As exudate solidifies and the wound heals, the dressing adheres to the wound, and the fibers within the dressing become enveloped by new tissue or blood clots. During dressing changes, damage is inflicted on newly formed tissue, causing pain to the patient and impeding the healing process.16 Conventional wound dressings primarily rely on passive protection to prevent external contamination of the wound rather than actively promoting wound healing.17 Moreover, conventional wound dressings lack functionality and specificity, resulting in poor efficacy in treating chronic wounds such as diabetic wounds.18 Hence, there is a necessity to develop novel multifunctional dressings that can provide both physical protection and facilitate wound tissue regeneration for the treatment of skin wounds. Numerous wound dressing materials have been innovated to facilitate the process of wound healing, encompassing semipermeable membranes, semipermeable foams, and hydrocolloids.19,20 However, some of the currently used wound dressings have limitations. For example, they may impede gaseous exchange between the wound and its surroundings, making it difficult for oxygen and nutrients to reach the wound bed. Some dressings are challenging to remove, may not provide adequate protection against microbial infections or maintain sterility, and can potentially induce allergic reactions. Furthermore, they may have limited absorption capacity for wound exudates and fail to maintain an optimal moist environment that is conducive to expedited wound healing.21 In recent years, hydrogels have gained significant attention in the field of wound healing due to their unique properties.
Hydrogels are three-dimensional network structures made up of polymers that are crosslinked chemically or physically (Fig. 1). Physical crosslinking refers to transient crosslinking, usually arising from interactions such as physical entanglement of polymer chains, ionic interactions, electron interactions, hydrogen bonding, etc. These interactions allow the hydrogel to revert to a soluble state when external conditions change. On the other hand, chemical crosslinking involves chemical reactions that create a permanent three-dimensional network through irreversible covalent bonds. Therefore, chemically crosslinked hydrogels possess a stable and permanent structure. They have exceptional hydrophilic (water-loving) properties and remain flexible when in contact with water molecules.22–24 Due to their elevated water content, supple structure, and porous nature, hydrogels bear a remarkable resemblance to living tissues.25 The development of hydrogels as biomaterials can be traced back to 1960, when Wichterle and Lim pioneered their synthesis.26 They created a hydrogel using poly2-hydroxyethyl methacrylate (PHEMA), which was subsequently used as a filler in eye enucleation procedures and as a material for contact lenses. Compared to traditional dressings, hydrogels offer several advantages in wound healing.27 They can alleviate patients' pain, improve the wound microenvironment, combat infections, and facilitate the healing process. Additionally, hydrogels can serve as targeted delivery vehicles for drugs, proteins, or cells.28 The extracellular matrix (ECM), which is a network of molecules surrounding cells, plays a vital role in creating a favorable microenvironment for wound healing by promoting wound re-epithelialization (regrowth of the epidermal layer) and angiogenesis (formation of new blood vessels).29,30 Because hydrogels structurally resemble the ECM, they have gained significant research attention and have wide-ranging applications in the field of biomedical sciences.28,31,32 Recently, there has been a shift in the functionality of hydrogels from singular physical coverage or individual functionalities to combining diverse functionalities. Multifunctional hydrogels have been successfully used in the repair of various types of wounds.33–35 Overall, hydrogels are versatile biomaterials with unique properties that make them suitable for medical applications. Their resemblance to living tissues, ability to retain water, and capacity for targeted drug delivery make them valuable tools in tissue engineering, wound healing, and other biomedical fields. Ongoing research in this area continues to explore and expand the potential uses and functionalities of hydrogels in healthcare.
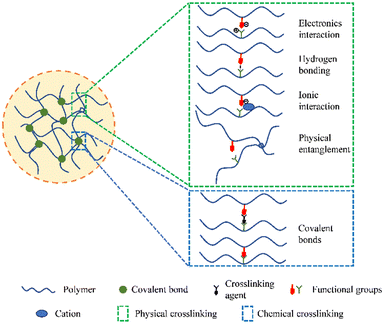 |
| Fig. 1 The structure of hydrogels (physical crosslinking and chemical crosslinking). | |
This article presents a comprehensive review of the research progress regarding the application of hydrogel-based dressings in wound healing. Firstly, we provide an overview of the process and characteristics of wound healing. Secondly, we summarize the various functionalities of hydrogel-based dressings in promoting wound healing, including adhesive property, antibacterial efficacy, hemostasis, angiogenesis promotion, anti-oxidation, anti-inflammatory effect, self-healing property, and conductivity. We also discuss the challenges encountered during the wound healing process, particularly in the context of different wound types (especially diabetic wounds), and outline the latest advancements in the use of hydrogel-based dressings in diverse wound management. Finally, we conclude and provide an outlook on the application of hydrogel-based dressings in wound healing, aiming to further enhance their capacity to promote wound healing.
2. Process and characteristics of wound healing
The wound healing process is a complex mechanism that facilitates the regeneration of damaged skin tissue. The phases of wound healing play a crucial role in determining the appropriate wound dressing for effective wound management. The process of wound healing consists of four sequential phases: hemostasis, inflammation, proliferation, and remodeling, which may also overlap (Fig. 2).36
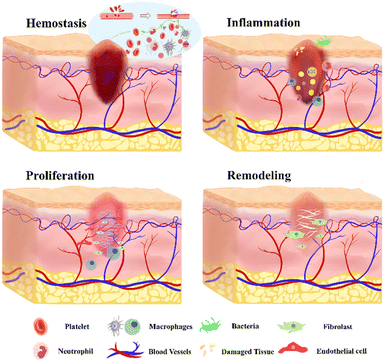 |
| Fig. 2 Process and characteristics of wound healing. | |
The hemostasis phase begins promptly after an injury and progresses rapidly. During this phase, blood vessels constrict, limiting blood flow.37 Platelets aggregate to form a clot, sealing the ruptured vessel wall and stopping the bleeding.27 Blood clots attract various relevant cells to collectively form a protective scab, which covers the wound until blood vessels dilate.38 Coagulation factors significantly contribute to the facilitation of targeted cell infiltration, with a specific emphasis on macrophages.39 In the inflammatory phase, neutrophils, reactive oxygen species (ROS), and proteases released by phagocytic cells clear debris and bacteria, thereby establishing a favorable milieu for the progression of the healing process.40 The injured blood vessels produce transudate, resulting in swelling. Controlled inflammation is beneficial during this phase as it helps prevent infection and manage bleeding.41 The inflammatory response is a complex process influenced by various intrinsic and extrinsic factors.42 Uncontrolled, prolonged, or excessive inflammation can lead to tissue damage and hinder the healing process.43 Different types of innate immune cells play a central role during this phase.44 Neutrophils, serving as the primary responders to epithelial injury, infiltrate the wound tissue, eliminating bacteria, restraining infection, and secreting relevant pro-inflammatory cytokines, consequently promoting fibroblast proliferation and angiogenesis.45,46 Macrophages, on the other hand, serve as master effector cells in tissue repair, demonstrating remarkable versatility and plasticity.47 The transition from inflammation to proliferation is a critical step in the wound healing process.48
The proliferative phase involves the synthesis of connective tissue, including blood vessels and granulation tissues, at the injury site to replace necrotic cells.49 The ECM, comprising proteoglycans, elastin, hyaluronic acid, and collagen, plays a crucial role in the formation of granulation tissue, replacing the original blood clot.50,51 During this phase, there is a noticeable increase in the production of various cells, such as keratinocytes, fibroblasts, and endothelial cells. These cells collectively contribute to wound healing, ECM deposition, and angiogenesis.52 Fibroblasts play a vital role in the conversion of the temporary fibrous matrix rich in fibronectin into a more resilient granulation tissue during the wound healing process.53 Angiogenesis, the process of new blood vessel formation, occurs to meet the demand for various nutrients necessary for tissue proliferation.54 Hypoxia acts as a trigger for angiogenesis, leading to the upregulation of hypoxia-inducible factors (HIFs) and cyclooxygenase 2, thereby inducing increased production of angiogenic factors.55–57 Macrophages significantly contribute to angiogenesis by facilitating microvascular endothelial cell behaviors.58,59 Remodeling and scar formation represent the final phases of wound healing, persisting for up to a year or longer, contingent upon the specific severity of tissue damage and the progress of wound healing.39 An imbalanced equilibrium between ECM synthesis and degradation can result in non-healing wounds or the development of pathological scars.60,61 During this phase, collagen remodeling occurs through crosslinking, leading to a reduction in scar thickness as the wound achieves complete closure.62 Additionally, apoptosis occurs, leading to the removal of cells that played a role in the wound healing process but are no longer needed.63,64
3. Multiple functions of hydrogel-based dressings
Hydrogels were initially utilized as wound dressings to provide physical isolation and create a moist environment. However, with the emergence of challenging non-healing wounds in clinical practice and the increasing demands for effective wound healing, both medical professionals and patients are seeking enhanced performance from hydrogel-based dressings. Consequently, there has been a surge in the development of hydrogel-based dressings with multiple functionalities. In this review, we will provide a comprehensive summary of the various functions of hydrogel-based dressings that contribute to promoting wound healing.
3.1 Adhesive property
Sutures and staples, while considered the “gold standard” for wound closure, may not be suitable for all types of wounds. These traditional closure methods have drawbacks such as an increased risk of secondary tissue injury and infection, which can impede optimal wound healing.65 As an alternative, adhesive hydrogels have gained increasing attention in the field of wound healing due to their less invasive and more effective nature.66 Presently, an array of adhesive hydrogels have been synthesized with a categorization into nature-inspired adhesive hydrogels and supramolecular-based adhesive hydrogels, aimed at augmenting the interfacial interaction between hydrogels and tissues.67,68 Nature-inspired adhesive hydrogels predominantly emulate structures and constituents observed in mollusks, such as mussels, and certain marine organisms like sandcastle worms. On the other hand, supramolecular-based adhesive hydrogels predominantly rely on mechanisms such as hydrogen bonding, host–guest interactions, or molecular topologies. However, one limitation of adhesive hydrogels is their insufficient mechanical strength, leading to reduced cohesion and restricting their potential applications in wound healing.69 To address this issue, researchers have developed innovative hydrogels with enhanced mechanical performance and adhesive capabilities. Dopamine (DA) is a compound that contains multiple catechol groups, which have been identified as crucial factors in achieving rapid and robust adhesion to wet tissues. In a study, researchers fabricated a gelatin–DA conjugated hydrogel and found that the adhesive properties of the hydrogel were dependent on the DA content, with the highest adhesive value observed at a substitution formula of 46%. The formulation exhibited excellent adhesive performance, measuring approximately 43 kPa (Fig. 3a).70 Shao et al. introduced a novel adhesive hydrogel (CP-Lap hydrogel) composed of chitosan and ε-polylysine (EPL), with modifications involving gallic acid. Due to its dual crosslinking nature, the hydrogel exhibited satisfactory mechanical strength ranging from 150 to 240 kPa, while also demonstrating notable resistance to swelling and degradation. Furthermore, the apparent adhesive strength of CP-Lap hydrogel showed a significant improvement, measuring approximately 30 kPa (Fig. 3b).71 Recently, a novel hydrogel was developed, consisting of catechol-modified oxidized hyaluronic acid, ε-poly-L-lysine, and Fe3+. By synergistically employing the mussel-inspired strategy, dehydration effect, and cohesion enhancement, the hydrogel achieved a remarkably high wet adhesion strength of approximately 78 kPa.72
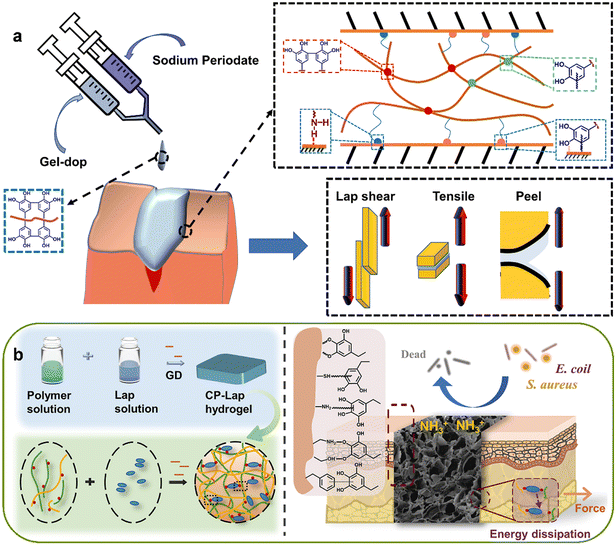 |
| Fig. 3 The adhesive property of hydrogel-based dressings. (a) Schematic figure showing the synthesized Gel-dop conjugate were activated by using sodium periodate chemistry and showing its applicability as tissue adhesive. Reprinted and modified with permission from ref. 70. Copyright 2020 Elsevier. (b) Schematic figure showing LAPONITE® stabilized endogenous antibacterial hydrogel as wet-tissue adhesive. Reprinted with permission from ref. 71. Copyright 2023 Elsevier. | |
Despite the effective wound closure achieved by adhesive hydrogels, their removal can cause pain and potentially interfere with the wound healing process. To tackle this concern, Jiang et al. utilized a protein-polyphenol complexation technique to fabricate an adhesive hydrogel patch, with the objective of establishing a thermoresponsive mechanism that is responsive to the temperature of the human body. The hydrogel's adhesion was intelligently triggered upon contact with warm skin, while painless detachment can be easily achieved by applying an ice bag to the surface of the hydrogel.73 Han et al. devised a two-step procedure to create a polydopamine-clay-polyacrylamide (PDA-clay-PAM) hydrogel with exceptional adhesion and toughness. The process involved the insertion of DA into clay nanosheets and partial oxidation between the layers, resulting in the presence of free catechol groups within the polydopamine-inserted clay nanosheets. Subsequently, acrylamide monomers were introduced and polymerized in situ, yielding a hydrogel with repeatable and durable adhesion. It is noteworthy that this hydrogel had the ability to adhere directly to the surface of human skin without eliciting an inflammatory response in the body. Moreover, it can be easily removed as needed without causing damage to the skin tissue.74 Liu et al. introduced a composite hydrogel named PBOF, formulated using multi-reversible bonds involving polyvinyl alcohol (PVA), borax, oligomeric procyanidin, and ferric ion. This innovative hydrogel demonstrated remarkable properties, including an ultra-stretch ability that was 100 times greater than its initial size, a highly tissue-adhesive strength of 24 kPa, and rapid shape adaptability within 2 minutes. When the removal of the hydrogel-based dressing is required, it can be easily detached within a span of 10 minutes using water as a simple triggering agent.75
3.2 Antibacterial efficacy
In general, when skin tissue is compromised due to various factors, it loses its normal barrier function, rendering the human body susceptible to the invasion of various pathogenic microorganisms from the external environment, including bacteria, fungi, and viruses.76,77 Wound infections caused by various pathogenic microorganisms significantly impede the normal process of wound healing. In severe cases, these infections can further propagate to various organs, leading to severe damage to the overall health of the human body.78 The development of antibacterial hydrogels has shown remarkable efficacy in preventing infection and promoting wound healing. Based on their constituent raw materials, antibacterial agents, and mechanisms of action, antibacterial hydrogels can be classified into three distinct types: (1) inherent antibacterial hydrogel; (2) antibacterial agent-containing hydrogel; and (3) environmental stimulus-responsive antibacterial hydrogel.79
3.2.1 Inherent antibacterial hydrogel.
Hydrogel wound dressings with inherent antibacterial properties can be created using various natural polymer materials. Materials such as chitosan, antimicrobial peptides, organic acids, and plant essential oils are frequently employed in the preparation of antimicrobial hydrogels due to their advantages such as biocompatibility and degradability.80 The presence of functional groups within the molecular structure of natural polymers contributes to their antibacterial activities, which are essential for the construction and efficacy of antibacterial hydrogel wound dressings. As a result, these inherent antibacterial hydrogels can exhibit sustained and persistent antibacterial activity.81 One example of such a hydrogel is the carboxyl-modified cellulosic hydrogel developed by Tavakolian and colleagues. They covalently attached ε-poly-L-lysine, a natural polyamide with antibacterial properties, to the hydrogel through a bioconjugation process. The antibacterial activity of this hydrogel was evaluated against Staphylococcus aureus and Pseudomonas aeruginosa. The results of the antibacterial activity experiment demonstrated significant efficacy, as the antimicrobial hydrogel eradicated approximately 99% of Staphylococcus aureus and Pseudomonas aeruginosa after a 3-hour exposure period (Fig. 4).82 However, it is important to note that hydrogels made from natural antimicrobial agents do possess certain limitations in their application. The antimicrobial action of these hydrogels typically relies on a mechanism that involves the disruption of bacterial cell membranes. While this can be effective, it may result in relatively low sterilization efficiency in some cases. Therefore, it's crucial to consider the specific requirements of the wound and carefully evaluate the antimicrobial efficacy of natural antibacterial hydrogels to ensure their suitability for use in wound dressings. The development of an injectable hydrogel using quaternary ammonium chitosan (QCS) and tannic acid (TA) as the main constituents is a notable advancement. Under physiological conditions, this hydrogel is easily miscible, and the integrity of its structure relies on the dynamic ion bonds and hydrogen bonds formed between QCS and TA constituents.83 Additionally, the incorporation of quaternized chitosan provides the hydrogel with broad-spectrum antibacterial capacity, enhancing its therapeutic potential. The concept of directly incorporating antibacterial moieties within the hydrogel matrix is an interesting approach to enhance the antibacterial characteristics of hydrogels. In a study conducted by Wang et al., they successfully developed stretchable hydrogels with inherent antibacterial properties through copolymerization of zwitterionic monomers with ionic monomers. The zwitterionic moieties contain abundant quaternary ammonium (QA) groups, and additional QA groups were introduced during the copolymerization process. As a result, the hydrogel-based demonstrated exceptional antibacterial efficacy, with a reduction in bacterial growth of over 99%.84
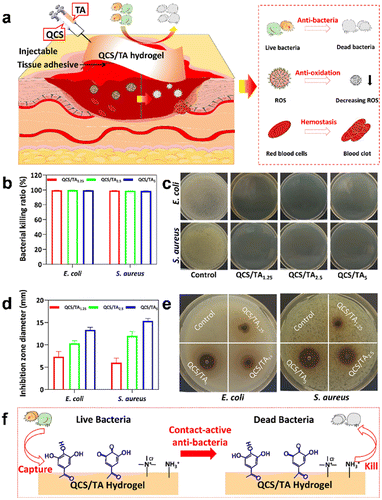 |
| Fig. 4 The antibacterial efficacy of hydrogel-based dressings. (a) QCS/TA hydrogels were Injected into the site of injury. (b) Quantitative statistics of killing ratios and (c) photographs of hydrogels against S. aureus and E. coli in the antibacterial performance test. (d) quantitative statistics and (e) photographs of the inhibition zone against S. aureus and E. coli in the ZOI test. (f) Schematic diagram of the antibacterial mechanism of hydrogels. Reprinted and modified with permission from ref. 82. Copyright 2022 American Chemical Society. | |
3.2.2 Antibacterial agent-containing hydrogel.
An additional effective strategy for addressing bacterial infections involves the incorporation of antibacterial agents into hydrogels, thus conferring exceptional antibacterial properties. Inorganic metal ions (such as Ag, Zn, Au, Cu, and Mg) and metal oxide nanoparticles have been observed to possess a wide range of antibacterial properties.79 The incorporation of inorganic metal ions and metal oxide nanoparticles into hydrogels has been demonstrated to significantly enhance their antimicrobial efficacy. However, concerns regarding the cytotoxicity and biocompatibility of metal-based biomaterials necessitate further comprehensive investigation. Despite these challenges, the development of metal-based composite antibacterial hydrogels remains a prominent and active area of research within the field of antibacterial biomaterials. Jiang et al. conducted a study detailing the fabrication of a multifunctional hydrogel, which incorporated fusiform-like zinc oxide nanorods (brZnO) into carboxymethyl chitosan (CMCS). In this formulation, the brZnO served a dual purpose, acting as both a crosslinker and a nano-filler. The synergistic combination of CMCS matrix and ZnO nanorods exhibited efficient antibacterial effects, while the hydrogel demonstrated controlled and sustained release of Zn2+.85 In addition, the incorporation of antibiotics, synthetic antibacterial agents, and antibacterial peptides within hydrogels can enhance their infection-resistant properties.86–88 Drug-loaded hydrogels have demonstrated superior wound healing capabilities, making them promising candidates for promoting efficient tissue regeneration. Moreover, these hydrogel systems exhibit potential in effectively mitigating bacterial drug resistance.89 The antibiotics frequently incorporated into antibacterial hydrogels can be categorized into aminoglycosides (such as gentamicin and streptomycin), glycopeptides (such as vancomycin), and quinolones (such as ciprofloxacin and levofloxacin).90 For instance, Zhang and colleagues achieved controlled drug delivery by incorporating vancomycin reversibly into a hydrogel synthesized from sodium alginate and citric acid as precursor materials. The resulting vancomycin-loaded hydrogel displayed significant antibacterial activity, particularly in weak acid environments. The combination of citric acid and vancomycin also exhibited a synergistic therapeutic effect, proving advantageous in the treatment of acute infections.91 Prolonged utilization of antibiotics may contribute to the emergence of drug-resistant bacteria, prompting researchers to investigate various synthetic antimicrobial agents as potential alternatives to conventional antibiotics. Hydrogels loaded with synthetic antibacterial agents offer a promising approach for precise drug delivery, providing a viable clinical solution to achieve targeted antibacterial effects and facilitate efficient wound healing. Antimicrobial peptides have been scientifically substantiated for their broad-spectrum antibacterial potential, along with their diverse properties, wide applicability, and reduced propensity to induce resistance mutations in target bacteria, thus presenting significant advantages.92,93 As a result, they represent an effective antimicrobial material to address the challenge of antibiotic resistance. A notable study by Wu et al. involved the preparation of a pH-sensitive hydrogel by integrating DP7 antibacterial peptide and oxidized dextran through reversible covalent bonding, specifically Schiff base linkages. Additionally, ceftazidime was loaded into the pH-sensitive hydrogel. This hydrogel demonstrated a synergistic antibacterial effect, resulting from the combined actions of the antibacterial peptide and the antibiotic. As a result, the hydrogel not only effectively mitigated bacterial resistance but also contributed to a reduction in antibiotic consumption.92
3.2.3 Environmental stimulus-responsive antibacterial hydrogel.
Photothermal agents or photosensitizers offer a promising approach for conferring antibacterial properties by manipulating the local environment upon their introduction. When incorporated into hydrogel wound dressings, these agents enable responsive antibacterial functionality. The therapeutic approaches involving the utilization of photothermal agents and photosensitizers to exert antibacterial effects are respectively designated as photothermal therapy (PTT) and photodynamic therapy (PDT). Moreover, investigators have delved into the advancement of ultrasound-responsive hydrogels, a methodology known as sonodynamic therapy (SDT).
(1) PTT: under sole near-infrared radiation, there is an absence of significant antibacterial effects. However, near-infrared radiation, when employed as an external stimulus, can stimulate photothermal agents to generate substantial heat, resulting in bacterial demise by protein destruction.94 The implementation of PTT in addressing bacterial infections is advantageous in preventing the development of drug-resistant bacteria, as PTT induces physical hyperthermia, causing rapid damage to bacteria.95 Notably, among photothermal agents, inorganic nanomaterials such as noble metals (such as Au, Ag), MoS2, CuS, and innovative two-dimensional materials have garnered considerable attention due to their cost-effectiveness, convenient fabrication processes, remarkable and broad light absorption capabilities, and favorable biocompatibility.79 Nevertheless, the thermal energy produced by photothermal antibacterial agents carries the risk of adverse effects, potentially causing damage to healthy tissues.96 A viable solution involves the strategic design of PTT-based antibacterial agents that exhibit either local or bacterial specificity, thereby enabling precise elimination of pathogenic bacteria while minimizing collateral damage to surrounding tissues.
(2) PDT: PDT represents a minimally invasive approach employing light-responsive photosensitizers and appropriate excitation sources to generate ROS. The resulting ROS, formed through an oxidative process, exhibit the capability to detrimentally affect surrounding biomolecules such as proteins, lipids, and nucleic acids, thereby leading to the eradication of pathogenic microorganisms.97,98 Typically, photosensitizers fall into two primary classifications: organic and inorganic photosensitizers. Organic photosensitizers predominantly encompass macrocyclic compounds such as porphyrins, phthalocyanines, indocyanine dyes, phenothiazines, and photosensitizers exhibiting aggregation-induced luminescence properties. Inorganic-based photosensitizers consist of ZnO, TiO2, and graphene quantum dots (GQDs).97 However, owing to the diminished stability of photosensitizers in physiological conditions, coupled with their low water solubility and photostability, the majority of photosensitizers encounter challenges in persisting at the defect site for repeated photodynamic stimulation.99 To surmount this constraint, photosensitizers are integrated into diverse carriers such as hydrogels. Zheng and colleagues have developed an innovative hydrogel platform that utilizes atomically precise gold nanoclusters-embedded natural polysaccharide carrageenan for single near-infrared light-triggered PTT and PDT.100 In this hydrogel-based dressing, the incorporation of Au25Capt18 as a single-component phototherapeutic agent exhibited significant thermal effects and generated singlet oxygen (1O2) upon irradiation with a single near-infrared light source. This unique combination allows for the rapid elimination of bacteria.
(3) SDT: sonosensitizers refer to pharmaceutical agents necessitating ultrasound activation for its efficacy.101 Ultrasound possesses the capability to penetrate deeply within the body, facilitating SDT in effectively targeting bacteria located deeply within an organism.102 The ultrasound-responsive hydrogel takes advantage of the non-invasive, safe, and cost-effective characteristics of ultrasound technology. Ultrasound has the ability to notably enhance the permeability of internal and external membranes, decrease the fluidity of bacterial cell membranes, and induce membrane depolarization, resulting in effective bacterial eradication.103 Porphyrin derivatives stand as the most extensively investigated sonosensitizers in research.104 Additional organic dyes, such as methylene blue, emodin, and acridine orange, have been employed as sonosensitizers in SDT.105 Due to its piezoelectric effect, barium titanate (BaTiO3) has witnessed further advancement in its potential application as a highly efficient sonosensitizer in sonodynamic antibacterial therapy.106,107 Utilizing ultrasound-triggered piezocatalytic therapy, Liu et al. developed a multifunctional hydrogel incorporating BaTiO3 nanoparticles. When exposed to ultrasonic vibrations, these embedded nanoparticles promptly generated ROS as a result of the substantial built-in electric field. This remarkable characteristic imparts the hydrogel with exceptional antibacterial efficacy.108 In addition to the aforementioned stimuli-responsive approaches, other stimulus-response modalities, such as pH, thermal, and salt, have also been extensively investigated and have shown promising results.109–111
3.3 Hemostasis
Hemostasis is a crucial process in wound healing that involves the formation of blood clots to stop bleeding from injured blood vessels. However, in cases of severe trauma or surgical procedures, excessive and uncontrolled bleeding can overwhelm the body's natural hemostatic capacity, leading to significant blood loss and impaired wound healing. Challenging scenarios of wound bleeding frequently occur in real-life situations, imposing stringent requirements for the development of hemostatic hydrogels capable of effectively addressing these complexities.112–114 Presently, natural polymers, synthetic polymers, inorganic compounds, and metal-based materials are utilized in the formulation of hemostatic hydrogels.115 The common natural polymer hemostatic materials are polysaccharide and collagen.116,117 The main polysaccharide hemostatic materials are chitosan, cellulose, hyaluronic acid and alginate. Synthetic polymers made by physical or chemical methods are easier to functionalize than natural polymers, such as self-assembling peptides, polyethylene glycol, and polycaprolactone.118–120 The inorganic materials suitable for hemostasis predominantly encompass zeolite, mesoporous silica, and graphene.121–123 Metal ions such as Ag, Zn and Cu can also be effectively used in the preparation of hemostatic materials.115 Liu et al. introduced a study describing the development of an injectable thermogelling chitosan/glycerophosphate formulation enriched with dihydrocaffeic acid (DHCA) to enhance the gel-forming capacity and tissue adherence properties.124 By integrating DHCA, the researchers achieved a significant reduction in gelation time, surpassing the duration of non-composite hydrogels by more than double, particularly at approximately 37 °C. Notably, the composite hydrogel exhibited a tissue adhesive strength more than two-fold higher than both non-composite hydrogels and previously reported formulations. Moreover, these thermosensitive hydrogels displayed significant in vitro effects in reducing the coagulation time of whole blood. Subsequent in vivo experiments conducted on rat models of hepatic hemorrhage and tail amputation revealed that the application of these thermosensitive hydrogels resulted in substantial improvements in hemostasis time and reduced blood loss. Recently, a self-gelling hemostasis powder has been developed, comprising a combination of polyacrylic acid, polyacrylamide, and quaternate chitosan. Upon contact with water, the powder rapidly fused, forming a stable hydrogel within an exceptionally short duration (<0.25 minutes). In vivo hemostatic evaluations demonstrated the superior performance of this hemostatic powder compared to commercially available products, specifically chitosan powder. Additionally, the hemostatic efficacy of this powder was comparable to that of hemostatic zeolite, as evidenced by the significant reduction in bleeding volume and hemostatic time (Fig. 5a).112 Researchers have also demonstrated the effectiveness of a biomaterial composed of oxidized alginate and oxidized dextran in combination with polyamidoamine dendrimer amine. This biomaterial enabled simultaneous electrostatic and covalent interactions with tissue, resulting in enhanced adhesion. Importantly, experiments conducted on both rabbit and pig models have demonstrated the exceptional ability of this biomaterial to withstand supraphysiological pressures (approximately 300 mmHg) and effectively prevent bleeding (Fig. 5b).125 To address the pressing medical challenges posed by visceral rupture, a multifunctional hydrogel-based wound dressing has been developed, harnessing the collective advantages of dynamic covalent bonds, metal–catechol chelation, and hydrogen bonds for multi-crosslinking. The efficacy of this dressing in promoting hemostasis has been demonstrated through experimentation using a rat ventricular puncture model, highlighting its potent hemostatic capability.126
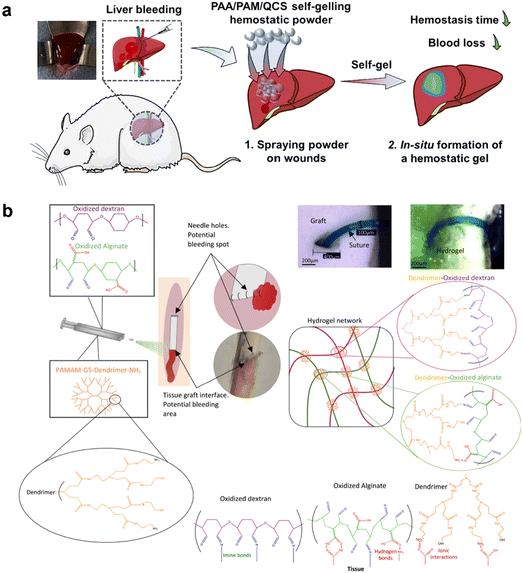 |
| Fig. 5 The hemostatic property of hydrogel-based dressings. (a) Schematic figure showing a self-gelling powder based on polyacrylic acid/polyacrylamide/quaternate chitosan for rapid hemostasis. Reprinted and modified with permission from ref. 112. Copyright 2023 Elsevier. (b) Sealant composition and interactions with tissue and ePTFE graft. Reprinted and modified with permission from ref. 125. Copyright 2022 Wiley-VCH. | |
3.4 Angiogenesis promotion
In the context of wound healing, nutrition and oxygen supply through blood vessels are essential for the cells involved in the healing process.127,128 Angiogenesis, the formation of new blood vessels, and revascularization play a critical role in accelerating wound healing, particularly in chronic wounds.129 Natural-origin hydrogels can serve as fundamental elements in pro-angiogenic systems and can be categorized into three major classes: (i) polysaccharide-based, (ii) protein-based, and (iii) derived from cellularized tissues.130 In certain instances, specific chemical constituents such as collagen and hyaluronic acid have demonstrated the potential to impart angiogenic capabilities to hydrogels. In order to enhance and promote wound angiogenesis, a variety of pro-angiogenic materials, such as various pro-angiogenic growth factors, copper ions and VEGF-mimicking peptide, can be incorporated into the hydrogel.131–133 Li et al. successfully devised a novel silica-based nanocomposite hydrogel scaffold, denoted as PABC, which incorporated polyethylene glycol diacrylate as the framework and incorporated bioactive glass nanoparticles containing copper and sodium alginate. In vitro investigations demonstrated that this novel hydrogel formulation exhibited significant enhancements in the viability, proliferative capacity, and angiogenic potential of endothelial progenitor cells. Importantly, in vivo studies indicated that this hydrogel formulation facilitated the generation and regeneration of vascular networks in diabetic wounds. This process was achieved through the upregulation of hypoxia-inducible factor-1α/vascular endothelial growth factor (HIF-1α/VEGF) expression and collagen matrix deposition, leading to accelerated wound healing and enhanced regeneration of skin tissue.134 In another study conducted by Jang et al., a gelatin methacrylate hydrogel incorporating a VEGF-mimicking peptide was formulated using a three-dimensional bio-printer. The hydrogel patches exhibited controlled release of the VEGF peptide, which facilitated the accelerated process of angiogenesis and promoted wound healing at the application site (Fig. 6).133
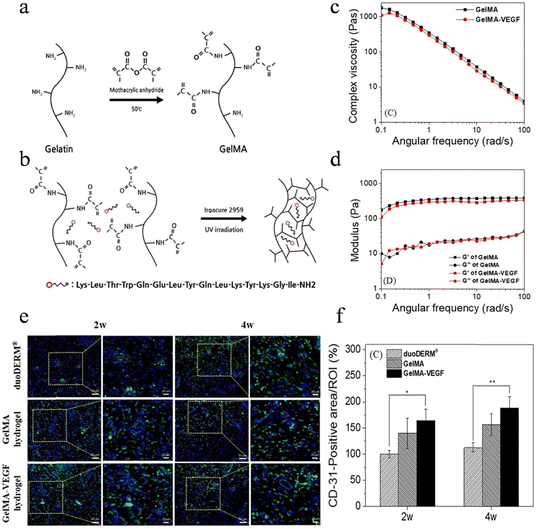 |
| Fig. 6 The angiogenesis promotion of hydrogel-based dressings. (a) Reaction of MA and gelatin. (b) Fabrication of crosslinked hydrogel network of GelMA included VEGF peptide. (c) Complex viscosity and (d) storage modulus (G′) and loss modulus (G′′). It was tested at 20 °C with 35 mm plate and plate geometry. (e) IF analysis of wounds harvested from pig at 2 and 4 weeks to assess the expression of vascular markers CD31. (f) Quantitative analysis of CD31. Reprinted and modified with permission under a Creative Commons Attribution 4.0 International License from ref. 133. Copyright 2021 IOP Publishing. | |
Recently, extracellular vesicles (EVs), also known as exosomes, derived from various cell types have emerged as a pivotal component of cell-free therapies, demonstrating significant potential in the field of wound healing.135,136 These EVs offer advantages over cell-based therapies by mitigating immunological risks and improving the homing efficiency of transplanted cells. They promote rapid wound healing through mechanisms involving angiogenesis promotion and inflammation modulation.135–138 Yang et al. conducted a study where they encapsulated exosomes derived from human umbilical cord mesenchymal stem cells (hUCMSC-exos) within a thermosensitive hydrogel called Pluronic F-127 (PF-127). They used a full-thickness wound model in diabetic rats and found that the combination of PF-127 hydrogel with hUCMSC-exos led to increased production of CD31 and upregulation of VEGF expression. This intervention promoted vascularization at the wound site, resulting in the acceleration of the wound healing process.139
Additionally, previous studies have highlighted the potential benefits of the “hot spring effect” in wound healing. The therapeutic benefits of hot springs are attributed to mild thermal stimulation and the promotion of angiogenesis mediated by the presence of metal ions.140 Sheng et al. developed a bioactive photothermal hydrogel by integrating fayalite and N,O-carboxymethyl chitosan. This hydrogel released bioactive ions and had inherent heating properties, creating a localized hot ion environment within the wound area. Through in vivo experiments, the hot spring-mimetic hydrogel formulation has been shown to effectively stimulate angiogenesis in wound sites.141
3.5 Anti-oxidation
Oxidative stress has been observed to have a detrimental impact on the wound healing process. ROS, such as hydrogen peroxide (H2O2), superoxide (O2˙−), the hydroxyl radical (˙OH), and 1O2, are not only strong oxidants but also important regulators of wound healing.142 Celluar signalling requires low levels of ROS especially angiogenesis, which is one of the necessary conditions for wound healing. However, an excessive presence of ROS in wounds disrupts the balance of the oxidative/antioxidant system, resulting in oxidative stress.143 Additionally, the overexpression of ROS induces oxidative damage to various biomolecules (such as protein inactivation, lipid peroxidation, DNA damage), mitochondrial dysfunction, endoplasmic reticulum stress.144 There is a certain degree of cytotoxicity associated with ROS overexpression, which can damage cells involved in wound healing like fibroblasts and vascular endothelial cells, also negatively affect the reconstruction of the ECM and the regeneration of blood vessels, ultimately impacting tissue regeneration.145 Accordingly, the inclusion of antioxidant properties in wound healing hydrogel-based dressings is crucial to address the excessive presence of ROS, thereby improving the oxidative microenvironment of the wound and promoting rapid wound healing. There are two primary methods for preparing antioxidant hydrogels: direct incorporation of antioxidants into the hydrogel matrix or coupling of antioxidant materials with the polymers that form the hydrogel.146 Current research suggests that the majority of hydrogels used for wound healing are loaded with antioxidants. However, researchers have also begun exploring hydrogels constructed from polymers modified with antioxidative materials, as the stability of antioxidants is a concern.143
Hydrogels have emerged as promising intelligent drug delivery systems that protect bioactive molecules from degradation.147 In the context of cutaneous wound healing, hydrogel systems loaded with antioxidants have been extensively used and have shown positive outcomes. Natural antioxidants can be categorized into thiol compounds, non-thiol compounds, vitamins, and various enzymes. In vivo, the major antioxidants consist of antioxidant enzymes and non-enzymatic antioxidants. These antioxidants exhibit strong antioxidative potential and effectively scavenge free radicals within organisms, thereby mitigating oxidative stress.143,148,149 Notably, polyphenols, predominantly found in plant-derived foods, have been extensively investigated due to their remarkable antioxidant properties.150,151 Plant polyphenols constitute a category of secondary metabolites characterized by a polyphenolic structure widely distributed in plants. In a strict sense, plant polyphenols encompass solely tannins; however, in a broader context, they also encompass small-molecule phenols, including anthocyanins, catechins, resveratrol, quercetin, gallic acid, ellagic acid, arbutin, and other natural phenols.143 Resveratrol is non-flavonoid polyphenol compound that has the ability to anti-inflammation and antioxidant. Li et al. reported the development of a natural silk fibroin-gelatin hydrogel-based dressing loaded with resveratrol, which was subsequently employed for wound healing applications.152 Yu and colleagues formulated a CMCS-plantamajoside hydrogel comprising plantamajoside, a natural Chinese herbal medicine known for its antioxidation capabilities. This hydrogel effectively diminished oxidative stress indicators and exhibited enhanced wound healing, as evaluated through an in vivo burn wound model.153 TA, a naturally occurring plant-derived polyphenolic molecule, exhibits biocompatibility and possesses antioxidative and anti-inflammatory characteristics, rendering it a valuable natural resource for the preparation and modification of biomaterials. By harnessing the favorable interactions between fibroin protein and TA, Jing et al. introduced a multifunctional hydrogel. The incorporation of TA imparted notable antioxidative activity to the hydrogel, as demonstrated by in vivo experiments, which highlighted the hydrogel's potential in promoting wound healing.154
Single antioxidant drug encapsulation alone may present challenges such as inconsistent performance and irregular release profiles. In recent years, the integration of nanomaterials into hydrogel networks has emerged as a promising strategy for developing antioxidant hydrogels, attracting growing attention from researchers.155 Ma et al. reported the development of an oxidized alginate/carboxylmethyl chitosan hydrogel augmented with antioxidative and anti-inflammatory properties by incorporating silver nanoparticle-coated epigallocatechin gallate (EGCG) nanoparticles. EGCG, the primary active component of tea polyphenols, is well-known for its antioxidative and anti-inflammatory characteristics. In this study, the ABTS and DPPH radical scavenging rates were quantified, and their magnitudes showed a direct correlation with the concentration of EGCG nanoparticles.156 In another study, Xu et al. introduced a multifunctional wound dressing called the Alg-PDA-Se hydrogel for infected wounds. This hydrogel incorporated low doses of selenium nanoparticles, as quantitatively analyzed, and exhibited remarkable ROS scavenging capabilities, effectively alleviating oxidative stress. In vivo experiments also demonstrated its synergistic effects against Staphylococcus aureus and Escherichia coli, promoting the healing of bacterial-infected wounds (Fig. 7).157 Recently, an ultrasound-assisted nanozyme hydrogel spray was developed by incorporating various components such as hyaluronic acid-encapsulated l-arginine, ultrasmall gold nanoparticles, and Cu1.6O nanoparticles co-loaded with phosphorus-doped graphitic carbon nitride nanosheets. Nanoenzymes, characterized by their enzymatic activity, have gained significant attention for the treatment of diabetic wounds due to their ability to regulate redox homeostasis and mitigate ROS-dependent inflammation. In vitro and in vivo experiments demonstrated that nanoenzyme hydrogel sprays can be activated within the diabetic wound environment, effectively eliminating ROS, alleviating inflammation, mitigating hypoxia, reducing blood glucose levels, promoting angiogenesis, and eliminating pathogens. Consequently, these effects significantly expedite the healing process of diabetic wounds.158 Collectively, these studies underscored the potential of diverse hydrogel-based strategies that incorporate antioxidants and nanoparticles to enhance wound healing, mitigate oxidative stress, and alleviate inflammation.
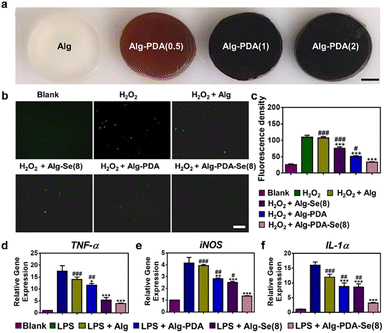 |
| Fig. 7 The anti-oxidation property of hydrogel-based dressings. (a) Optical images of the Alg hydrogel modified with different dopamine concentrations. Scale bar: 2 mm. (b) Intracellular ROS-scavenging performance of Alg, Alg-PDA, Alg-Se(8), and Alg-PDA-Se(8). Scale bar: 50 μm. (c) Quantitative result of ROS scavenging ability, n = 5. (d) Expression of pro-inflammatory factors TNF-α and iNOS and IL-1α in macrophages (RAW264.7) after being treated with different hydrogels by RT-PCR. Reprinted and modified with permission from ref. 157. Copyright 2023 American Chemical Society. | |
In addition to hydrogels laden with various antioxidants, researchers in recent years have explored the utilization of antioxidant materials to modify polymers for the construction of self-antioxidative hydrogels. The majority of these self-antioxidative hydrogel matrices involve modified polyphenols.143,159 The preparation of chitosan hydrogels modified with dihydrocaffeic acid manifests notable antioxidative activity, exhibiting a promotional effect on the healing of chronic inflammatory wounds.109,160 Zhu et al. identified a peptide derived from laminin with inherent antioxidative properties. Conjugation of this peptide with poly(polyethylene glycol cocitric acid-co-N-isopropylacrylamide) results in the formation of a hydrogel dressing, which can be utilized to facilitate wound healing. This peptide-hydrogel system demonstrated excellent antioxidative effects without the necessity for additional biological or pharmacological factors, thereby accelerating tissue regeneration in diabetic wounds.161
3.6 Anti-inflammatory effect
Wound healing is a complex process that can be particularly challenging in the case of chronic wounds, such as diabetic ulcers and severe burn injuries. In these situations, various factors can disrupt the regulation of inflammation, leading to delays in wound healing.162,163 Although insufficient wound inflammation is not commonly observed, excessive inflammation is nearly ubiquitous in chronic wounds, impeding the normal tissue repair of the wound.164 Consequently, addressing excessive inflammation is crucial for facilitating the wound healing process. As a result, extensive research has been conducted by investigators in the field of anti-inflammatory hydrogels, yielding significant achievements. The classification of hydrogel dressings with anti-inflammatory properties is determined by the precise mechanisms through which they exert their anti-inflammatory activities. These mechanisms include scavenging excessive ROS, sequestering chemotactic factors, and promoting the polarization of macrophages.165
(1) Scavenging excessive ROS: the derivatives of oxygen, referred to as ROS, have been recognized to play a beneficial role in promoting wound healing at low concentrations.166 However, certain factors present in wounds, such as hyperglycemia and severe infections, can cause persistent infiltration of inflammatory cells like neutrophils and macrophages, leading to the production of excessive and harmful ROS. Oxidative stress, characterized by an imbalance between ROS production and the body's antioxidant defense system, contributes to a robust inflammatory response that sustains the pro-inflammatory phenotype of macrophages and impedes wound healing, particularly in chronic wounds.167–169 Alleviating oxidative stress can significantly mitigate inflammatory responses and expedite the rapid healing of wounds. The application of antioxidant hydrogel-based dressings in wound healing has been elucidated in the anti-oxidation property section.
(2) Sequestering chemotactic factors: chemotactic factors play a crucial role in all stages of wound healing and can significantly impact the wound healing process. However, the presence of excessive chemotactic factors at the wound site can lead to unfavorable outcomes in wound healing. Therefore, therapeutic strategies aimed at targeting the excessive pro-inflammatory chemotactic factors at the wound site have shown promise in improving the inflammatory condition and promoting wound healing.170–172 Potential approaches for targeting chemotactic factors encompass monoclonal antibodies, small-molecule antagonists, and glycosaminoglycans (GAGs) that disrupt the distribution of chemotactic factors.173 Among these strategies, GAG-based anti-inflammatory hydrogels stand out as prominent in the field of wound healing therapeutics. The formulation of hydrogels involves the flexible utilization of diverse biomimetic materials, guided by the interaction principles between GAGs and chemotactic factors. This enables the hydrogels to capture excessive pro-inflammatory chemotactic factors in wounds, thereby facilitating the process of wound healing. A novel hydrogel was developed based on end-functionalized star-shaped polyethylene glycol (starPEG) and GAG heparin derivatives. This hydrogel was designed to effectively sequester chemotactic factors. In a study involving patients with chronic venous leg ulcers, this hydrogel demonstrated the ability to efficiently remove inflammation-associated chemotactic factors from the wound fluid. Additionally, the hydrogel reduced the migratory activity of monocytes and polymorphonuclear neutrophils. Furthermore, experimental results using a wound delayed healing model in db/db mice showed the beneficial effect of this hydrogel in alleviating the inflammatory response.174
(3) Promoting the polarization of macrophages: chronic wound healing impairment is closely associated with disruptions in the immune microenvironment, which is characterized by sustained elevation of inflammatory mediators and abnormal innate and adaptive immune responses.175 Given the critical role of immune cells in each stage of wound healing, the regulation of immune responses offers a highly promising strategy for effective wound management. Immune cell reprogramming has emerged as a widely adopted approach for modulating immune reactions and has been successfully implemented in wound management.176 This approach often involves manipulating macrophage polarization, facilitating antigen-specific differentiation of T cells into T follicular helper cells, and inducing pro-inflammatory activation markers on dendritic cells (DCs).177 Given the critical role of excessive inflammation in chronic wounds, manipulating macrophage attachment and polarization to enhance inflammation resolution has become a prominent approach in wound treatment. Maintaining a balanced pro-inflammatory/anti-inflammatory phenotype ratio during the wound healing process is recognized as a critical factor in modulating inflammatory reactions and transitioning from inflammation to proliferation.178–180 Macrophages, based on their roles in wound healing, can be categorized into pro-inflammatory macrophages (M1 macrophages) and anti-inflammatory macrophages (M2 macrophages). The polarization of macrophages is highly dependent on the microenvironment of the wound, and detrimental factors present at the wound site, such as hyperglycemia and infection, can impede the polarization of M1 macrophages towards M2 macrophages. In this scenario, the wound remains in the inflammatory phase, which can adversely affect the proliferation of epithelial tissues, deposition of collagen, and angiogenesis, thereby impeding the normal process of wound healing.181–183 Finding ways to promote the polarization of M1 macrophages towards M2 macrophages in the wound becomes a challenging issue that needs to be addressed. In recent years, a spectrum of dressings has been specifically engineered to modulate the microenvironment of chronic wounds, with the objective of fostering macrophage polarization during the latter phases of wound healing. Notably, hydrogel dressings have emerged as a focal point of interest in this domain. These hydrogels can be meticulously designed to immunomodulate chronic wounds by administering bioactive molecules such as antimicrobial agents, immunomodulatory components, growth factors, genetic material, and cellular components. This targeted delivery aims to stimulate the transition of M1 macrophages to the M2 phenotype, thereby expediting the overall wound healing process.177 In the study conducted by Sheng et al., a water-soluble phosphocreatine-grafted methacryloyl chitosan (CSMP) was synthesized using a one-step lyophilization method. Subsequently, the CSMP hydrogel was fabricated through a photocrosslinking process. The results demonstrated that the CSMP hydrogel may exert inhibitory effects on M1 macrophage polarization through the NF-κB signaling pathway. Moreover, in vivo research findings demonstrated that this hydrogel significantly enhanced the wound healing process and reduced the levels of associated inflammatory factors.184 Wei et al. devised a multifunctional hydrogel incorporating three polyphenols, namely TA, oligomeric proanthocyanidins, and EGCG. In vitro experimental outcomes demonstrated the hydrogel's capability to effectively scavenge hydroxyl and ABTS+ radicals, exhibiting potent antioxidant properties. In a mouse model, this hydrogel promoted M2 macrophage polarization and cytokine-mediated anti-inflammatory effects, thereby accelerating wound healing (Fig. 8).185 The ion products derived from bioactive glass have been proven to promote the polarization of macrophages towards the M2 phenotype, leading to an increased secretion of anti-inflammatory growth factors by these cells.186,187 The study conducted by Zhu et al. investigated the effects of bioactive glass/sodium alginate hydrogel, demonstrating its ability to induce polarization of macrophages towards the M2 phenotype and enhance the expression of anti-inflammatory genes.188 Exosomes derived from M2 macrophages (M2-Exos) carry essential regulatory factors that drive macrophage polarization towards the M2 phenotype. These vesicles have been harnessed to induce the reprogramming of M1 macrophages into the M2 subtype, effectively contributing to wound management.189 Biodegradable and hydrolytically degradable PEG hydrogels (Exogels) have been designed and developed for sustained release of exosomes. The Exogels were utilized to encapsulate M2-Exos, aiming to optimize their therapeutic impact in the context of wound healing. The localized presence of M2-Exos facilitated a successful local transition from M1 to M2 macrophages, sustaining this transition for a period surpassing 6 days. Subsequently, this prolonged effect leads to augmented therapeutic outcomes, encompassing accelerated wound closure and elevated healing quality, as demonstrated in an animal model for cutaneous wound healing.190 In addition to a plethora of biological constituents, several immunomodulatory small molecules, such as IL-10, resolvin D1, lipoxin A4, and catechol, have been investigated for their potential in wound healing.191–193 These molecules demonstrate various effects on different stages of the wound healing process, notably by influencing inflammatory reactions through the polarization of anti-inflammatory phenotypes, augmenting antigen-specific CD4+ T cell responses, and downregulating the expression of IL-1β. Sok et al. conducted an investigation into the characteristics of a PEG-based hydrogel. This hydrogel exhibited the capability of targeted release of aspirin-triggered resolvin-D1, a specialized pro-resolving lipid mediator, in conjunction with the binding of recombinant human IL-10. The experimental findings demonstrated that this approach successfully recruited and re-educated mononuclear phagocytes within the hydrogel. Notably, the hydrogel facilitated the localization of immune-suppressive subsets, including CD206+ macrophages (M2a/c) and IL-10-expressing DCs. These results provide compelling evidence for the potential of combined delivery strategies in recruiting regenerative cell subsets that contribute to addressing complications associated with wound healing.194
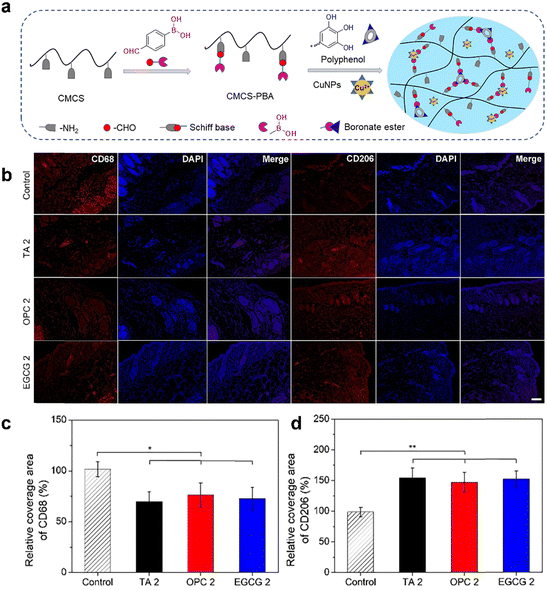 |
| Fig. 8 The anti-inflammatory effect of hydrogel-based dressings. (a) Schematic illustration of preparing polyphenol-crosslinked hydrogels. (b) Representative immunofluorescence staining of CD68 and CD206 on Day 7. Quantified data of the relative area percentage that covered by (c) CD68 and (d) CD206, respectively. Reprinted and modified with permission from ref. 185. Copyright 2022 Elsevier B.V. | |
3.7 Self-healing
There is a greater demand for mechanical durability when using hydrogel-based dressings over an extended period. The mechanical forces generated by human activities can damage the integrity of the hydrogel structure, thereby affecting the reparative efficacy of the hydrogel-based dressings.195 However, self-healing hydrogels possess the unique ability to restore both the structural and functional integrity. Unlike traditional hydrogels, self-healing hydrogels exhibit reversible dynamic linkages within their network. As a result, when the network is damaged, self-healing hydrogels can effectively restore their original structure without compromising their functionality.196,197 Consequently, self-healing hydrogels hold significant potential for applications in biomedical fields, particularly in wound dressings. Self-healing hydrogels can be classified into two categories based on their healing mechanisms: chemical covalent cross-linking hydrogels and physical noncovalent cross-linking hydrogels.
(1) Chemical covalent cross-linking hydrogels: chemical covalent interactions, such as Diels–Alder reactions, disulfide linkages, acylhydrazone bonds, and imine bonds, are the reversible bonds formed in these self-healing hydrogels.198 Among them, the reversible imine bond, also known as a Schiff base, is a dynamic covalent bond formed through the condensation of an amine and an aldehyde group. The imine bond is consistently employed as the dynamic crosslinker in the production of self-healing hydrogels with dynamic properties.199,200 Amino containing polymers, such as chitosan, gelatin, and polyetherimide, can be prepared as dynamic hydrogels through the formation of imine bonds with aldehyde-functionalized polymers.160,201,202 The presence of this chemical bond plays a pivotal role in the self-healing process of hydrogels, as it enables the occurrence of breakage and reformation within the network of the hydrogel.203,204 Cui et al. developed an antibacterial hydrogel for facilitating the effective healing of wounds infected by Staphylococcus aureus. The presence of dynamic imine bonds in hydrogels, based on quaternized chitosan and PEG derivative (dialdehyde terminated PEG), imparts self-healing and self-adaptive capabilities to the material. These unique capabilities enabled the hydrogel to effectively conform to irregular wound surfaces and enhanced the safety and efficacy of drug delivery.205 In another study by Chen et al., a novel hemostatic hydrogel was developed by cross-linking inorganic polyphosphate conjugated with poly(aspartic acid) hydrazide and PEO90dialdehyde. Leveraging the dynamic nature of acylhydrazone bonds, the hydrogel demonstrated the ability to repair cracks generated under external forces. Moreover, the hydrogel exhibited excellent biocompatibility, tissue adhesion, and remarkable hemostatic properties (Fig. 9).206 The thermal reversibility of the Diels–Alder cycloaddition, involving a conjugated diene and a dienophile, enables its dissociation upon heating and establishment of a new equilibrium. This reaction is widely favored for its favorable thermal reversibility characteristics and the simplicity of its reaction conditions. Shao et al. presented a rapid self-healing nanocomposite hydrogel composed of cellulose nanocrystals (CNCs) and polymer chains of PEG. Fourier-transform infrared spectroscopy confirmed the presence of a covalent Diels–Alder click reaction between CNCs and PEGs, which exhibited excellent thermal reversibility. The results demonstrated that this hydrogel exhibited excellent self-healing capability, reaching up to 78%.207
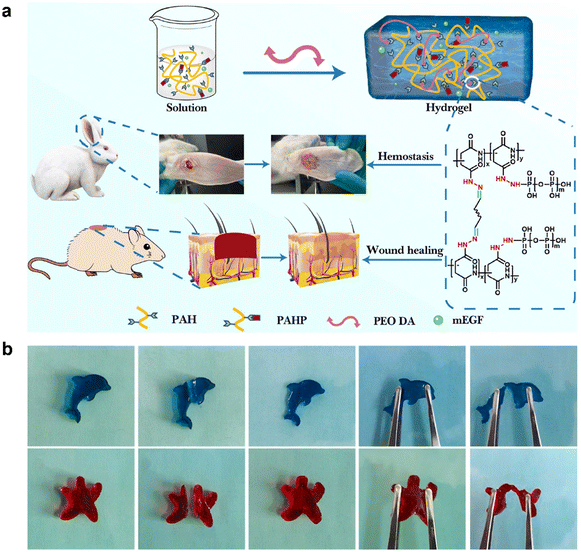 |
| Fig. 9 The self-healing property of hydrogel-based dressings. (a) PAHP and PEO DA cross-linked hydrogel were used for rapid hemostasis of rabbit ear arterial and wound repair in mice. (b) Self-healing of dolphin and starfish (PAH/PEO90 DA hydrogel). Reprinted and modified with permission from ref. 206. Copyright 2022 Elsevier B.V. | |
(2) Physical noncovalent cross-linking hydrogels: physical noncovalent interactions, such as hydrogen bonds, hydrophobic associations, π–π stacking, and host–guest interactions, can also be utilized.198 Hydrogen bonding is a crucial physical interaction involved in the formation of secondary and tertiary structures in biological systems. This intermolecular interaction is induced by the protonation of polar functional groups, which imparts the ideal characteristics of self-healing to the wound dressing material.208,209 Several polymers, including polyvinyl alcohol, polyethyleneimine, polyvinylpyrrolidone, hyaluronic acid, and chitosan, have garnered extensive attention in the preparation of self-healing hydrogels through hydrogen-bond crosslinking.200,210 Yu et al. fabricated a self-healing hydrogel-based dressing using humic acid and polyvinylpyrrolidone as materials. In this hydrogel, humic acid acted as a crosslinker, forming hydrogen bonds with polyvinylpyrrolidone. The dynamic reversibility of the hydrogen bonds formed between humic acid and polyvinylpyrrolidone endowed the hydrogel with remarkable self-healing properties. In the event of significant deformation, the integrity of the internal structure of the hydrogel may be compromised; however, it rapidly reverts back to its original intact state autonomously.210 The π–π stacking interaction, a noncovalent interaction, specifically refers to interactions involving aromatic groups that possess π bonds.211,212 Han et al. successfully prepared a double-layer hydrogel composed of an adhesive layer and a tough layer through in situ polymerization. The tough layer was enhanced by the addition of polystyrene particles, improving the gel's deformability. On the other hand, the adhesive layer achieved the adhesion of various interfaces by incorporating PDA nanoparticles. Furthermore, the double-layer gels exhibited enhanced self-healing properties due to the physical cross-linking of hydrophobic associations in the ductile layer and the physical interactions of hydrogen bonding and π–π stacking in the adhesive layer.213
3.8 Conductivity
Previous studies have confirmed the capability of electroactive materials to influence the behavior of electrically excitable cells involved in wound healing, such as fibroblasts and keratinocytes.214 Consequently, the use of materials with conductive properties holds great potential for enhancing critical aspects of the wound healing process. Furthermore, the application of conductive materials in wound healing has demonstrated the ability to mitigate oxidative damage by reducing excessive levels of ROS. Recognizing these beneficial effects, an increasing number of researchers have proposed incorporating conductive materials into hydrogel dressings with the aim of improving the wound healing process.215–217 Conductive hydrogels have emerged as promising scaffold materials for tissue engineering of electroactive tissues. Conductive hydrogels can serve as bridges between normal and damaged tissues, facilitating the transmission of electrical signals between the tissues.218–220 Based on the specific conductive component used, conductive hydrogels can be classified into five distinct groups: conductive polymers, carbon nanomaterials, noble metal nanocrystals, ionic conductive hydrogels, and other variations.220
Exemplifying this trend, conductive hydrogels based on carbon by straightforwardly incorporating CNTs or graphene into hydrogel matrices. Furthermore, the efficacy of these materials in facilitating tissue repair has been empirically validated.221 CNTs are allotropes of carbon characterized by a nanostructured tubular form, exhibiting a length-to-diameter ratio exceeding 1
000
000.222 Introducing CNTs into wound healing processes holds the potential to mediate electrical signals in the wound area, facilitating and expediting wound healing by promoting cell migration and blood flow. Liang et al. presented a study demonstrating the incorporation of CNTs into wound dressings. In this study, a layer of PDA was initially used to coat the CNTs’ surface, reducing the strong hydrophobic interactions typically associated with them. The modified CNTs were then integrated into hydrogels, showcasing a positive correlation between the concentration of CNTs and the conductive properties of the resulting hydrogel. In vivo experiments conducted in mice demonstrated that these hydrogels exhibited desirable antimicrobial, antioxidant, and adhesive properties, effectively promoting wound healing processes.217 Graphene, an electrically conductive two-dimensional material, possesses several desirable properties including biocompatibility, high surface area, thermal conductivity, and electrical conductivity. Its high specific surface area allows for potential enhancement of the biological activity of hydrogels through improved interactions between biomolecules, cells, and organisms upon its incorporation. The study conducted by Zhang et al. focused on the development of a graphene hybrid supramolecular hydrogel (GS hydrogel) specifically intended for wound dressing applications.223 In animal experiments, the GS hydrogel exhibited remarkable efficacy in accelerating the wound healing process. It facilitated microbial clearance, promoted collagen deposition, and stimulated blood vessel formation, all of which are crucial for effective wound healing. Additionally, the GS hydrogel showed significant antibacterial activity against methicillin-resistant Staphylococcus aureus (MRSA), which is a concerning antimicrobial-resistant bacterium. Wu et al. developed a conductive antibacterial hydrogel that exhibited the sustained release of the pro-angiogenic drug deferoxamine. This hydrogel facilitated the generation of HIF-1α and VEGF, thereby promoting angiogenesis. Significantly, it is noteworthy that the hydrogel, incorporating polyaniline, facilitated the transmission of electrical signals to the skin. This property could synergistically combine with electrical stimulation therapy to promote the healing of diabetic-infected wounds.224 Wang et al. conducted a research study aimed at enhancing wound management through the introduction of a flexible electrical patch integrated with conductive hydrogel electrodes. The hydrogel was developed by incorporating silver nanowires (AgNW) and methacrylated alginate (MAA). The selection of AgNW as the electrode material was motivated by its antibacterial properties, while MAA was chosen for its clinical suitability in promoting wound healing. Meticulous optimization of the hydrogel formulation was carried out to ensure its compatibility with medical-grade patches, facilitating personalized wound treatment. In vitro investigations demonstrated that the application of electrical stimulation led to increased secretion of growth factors, along with enhanced cell proliferation and migration capabilities. In vivo experiments further supported the accelerated wound healing effects associated with the implementation of this patch (Fig. 10).225
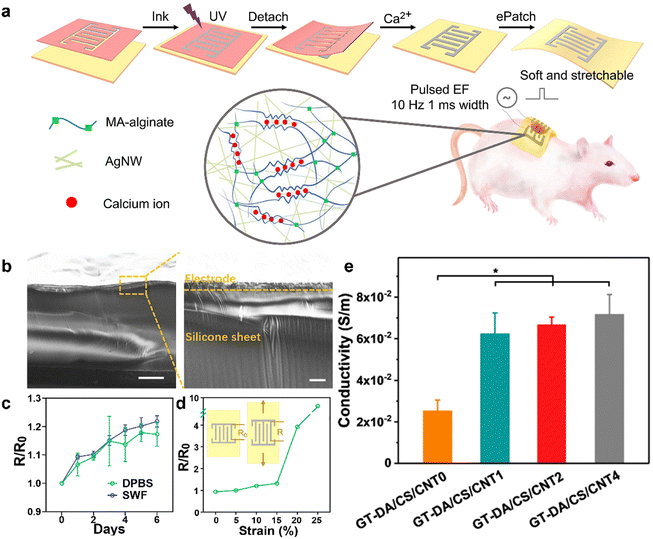 |
| Fig. 10 The conductivity of hydrogel-based dressings. (a) Schematics of the ePatch fabrication, AgNW-MAA ink formula and the double-crosslinked network. (b) SEM images of a cross-section of the fabricated electrode. The right image showed the magnified section of the left one. Scale bars represent 100 and 10 μm for left and right images, respectively. (c) Change of electrode resistance after soaking in DPBS. Ratios of resistances at different time points (R) to time 0 (R0) were calculated. (d) Resistance changes under strain ranging from 0 to 25%. (e) Conductivity of the GT-DA/CS/CNT hydrogels. Reprinted and modified with permission from ref. 225. Copyright 2022 Elsevier. | |
4. Application of hydrogel-based dressings in different types of wounds
Cutaneous wounds can be classified into different types, namely acute wounds and chronic wounds, each with distinct characteristics. The unique features of each wound type emphasize the need for specific hydrogel-based dressings that are tailored to address their specific requirements. In this section, we will explore the challenges encountered during the wound healing process across different wound types and provide an overview of the recent advancements in the application of hydrogel-based dressings for diverse wound management (Table 1). Moreover, an increasing number of researchers have endeavored to develop various hydrogel dressings capable of monitoring the wound healing process, and we will conduct a comprehensive review of these novel hydrogels as well.
Table 1 Etiology of different types of wounds and functional requirements of hydrogel dressings
Wound type |
Etiology |
Functional requirements |
Hydrogel dressings (example) |
Acute wound |
Incisional wound |
Surgical incisions, burns, bites, deep lacerations, or abrasions |
Adhesive property, Antibacterial efficacy, hemostasis, self-healing property |
PEGSD/GTU,231 QCS-PA@Fe232 |
Excisional wound |
Adhesive property, antibacterial efficacy, hemostasis, angiogenesis promotion, anti-oxidation, anti-inflammatory effect, self-healing property, conductivity |
HA-DA/rGO,234 PB-EPL/TA@BC,235 μGeld particles236 |
Chronic wound |
Diabetic wound |
Inadequate primary care, bacterial infections, diabetes, or vascular disorders |
Adhesive property, antibacterial efficacy, hemostasis, angiogenesis promotion, anti-oxidation, anti-inflammatory effect, self-healing property, conductivity, oxygen supply, glucose decreases |
OxOBand,260 HAMA-PBA/catechol,271 CBP/GMs@Cel&INS,267 FEMI,280 BP/CS-bFGF291 |
4.1 Acute wound
An acute wound refers to a skin injury caused by surgical incisions, burns, bites, deep lacerations, or abrasions.226 Acute wounds have the inherent ability to heal spontaneously through a well-organized and predictable process, even without external interventions.4 The typical healing time for acute wounds is approximately 8 to 12 weeks. During the healing process, acute wounds exhibit low levels of bacteria, inflammatory cytokines, proteases, and reactive oxygen species. Additionally, they maintain an intact functional matrix, along with heightened mitotic activity and the proliferative capacity of cells.7,227 When cultivating fibroblasts from acute wounds, it was observed that they exhibited a heightened level of mitotic activity.228,229 Researchers have investigated the impact of acute wound fluid from split-thickness skin graft patients on the in vitro growth of human dermal fibroblasts and umbilical vein endothelial cells. The findings revealed a stimulating effect of acute wound fluid on the growth of both cell types, indicating that the molecular environment of acute wounds promotes cellular proliferation.229 In scientific studies, the safety and effectiveness of wound dressings in promoting the healing of acute wounds are often assessed by creating incisional or excisional models on the dorsal area of rats or pigs.
The incisional model is a commonly used experimental method where surgical wounds are created on the skin of animals, typically rats or pigs, using sharp surgical blades. This approach allows for the controlled creation of skin incisions with minimal accompanying tissue damage. However, conventional suturing methods used to close these wounds have drawbacks such as secondary tissue damage, increased risk of infection, and discomfort during suture removal.230 In contrast to sutures, hydrogel-based dressings have emerged as a less invasive and more effective alternative for wound healing. In order to promptly close incision wounds, achieve effective hemostasis, and prevent wound infections, it is essential to utilize hydrogel dressings with adhesive properties, antibacterial efficacy, hemostatic capabilities, and self-healing properties to facilitate the repair of the incision wound. Zhao et al. devised a novel injectable self-healing hydrogel adhesive. This hydrogel adhesive was composed of two components: catechol-Fe3+ coordination cross-linked poly(glycerol sebacate)-co-poly(ethylene glycol)-g-catechol and quadruple hydrogen bonding cross-linked ureido-pyrimidinone modified gelatin. In in vivo experiments, this hydrogel demonstrated notable hemostatic properties for treating skin trauma and showed high efficacy in combatting methicillin-resistant MRSA infections. Moreover, compared to medical glue and surgical sutures, the hydrogel adhesive exhibited superior wound closure and healing capabilities for skin incisions.231 Another group of researchers, Liang et al., developed adhesive hydrogels using a dual-dynamic-bond cross-linking approach. These hydrogels include Fe3+, protocatechualdehyde (PA) containing catechol and aldehyde groups, and QCS with the aim of facilitating the closure of skin incisions. The dual dynamic crosslinking strategy involved pH-sensitive coordination bonds (catechol-Fe) and dynamic Schiff base bonds, which can undergo reversible breaking and reformation. This strategy imparted excellent self-healing properties to the hydrogel-based dressing, enabling it to undergo self-healing and on-demand dissolution or removal. Additionally, these hydrogels possessed injectability, favorable biocompatibility, antibacterial activity, multifunctional adhesiveness, and hemostatic properties. The positive effects of these hydrogels on wound closure and post-wound-closure care have been demonstrated through in vivo evaluations using a rat skin incision model (Fig. 11).232
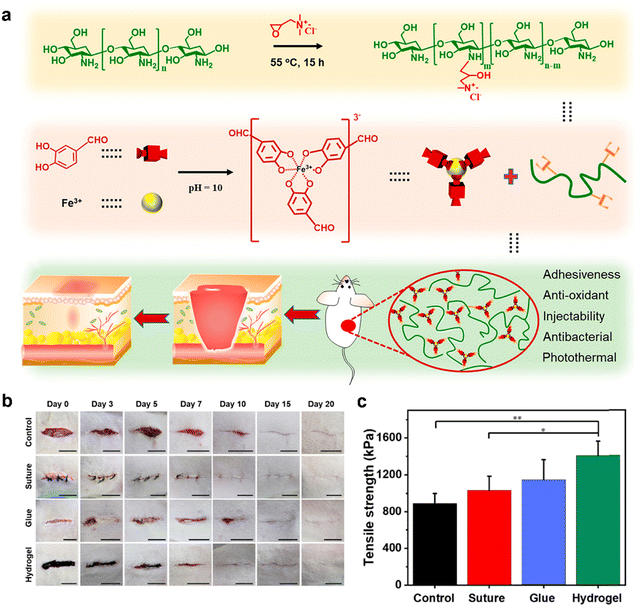 |
| Fig. 11 Hydrogel-based dressings for acute wound healing. (a) Schematic illustration for the preparation and application of the dual-dynamic-bond cross-linked adhesive hydrogel. (b) Representative images of the incisional skin wounds treated by surgical sutures, biomedical glue, and adhesive hydrogel, as well as the skin incisions without treatment at determined times. Scale bar: 1 cm. (c) Tensile strength of the healed skin incision. Reprinted and modified with permission from ref. 232. Copyright 2021 American Chemical Society. | |
In the establishment of full-thickness excisional wound models, researchers commonly use blades or punches to remove the epidermal, dermal, and subcutaneous fat layers, creating a clearly delineated full-thickness skin excision wound model. However, this method exposes deep tissues, which can lead to potential complications such as deep infections, fluid exudation, and damage to blood vessels and nerves, thereby complicating the wound healing process.233 To address complications associated with excisional wounds, hydrogel dressings must possess a variety of functions while meeting fundamental requirements. These functions include adhesive properties, antibacterial efficacy, hemostatic capabilities, promotion of angiogenesis, anti-oxidative features, anti-inflammatory effects, self-healing, and conductivity. However, the application of hydrogels in the healing of full-thickness excision wounds still faces certain limitations, including limited capacity to manage exudate, the challenge of incorporating and preserving the bioactivity of loaded bioactive substances, and low skin adhesion. To enhance the repair of full-thickness excision wounds and address potential complications, Liang et al. developed a series of adhesive, hemostatic, antioxidant, conductive, photothermal, and antibacterial hydrogels. These hydrogels were fabricated by utilizing hyaluronic acid-graft-DA and reduced graphene oxide (rGO) through a H2O2/HPR system. In a murine full-thickness skin excision wound model, the hydrogels demonstrated the ability to upregulate the expression of CD31, leading to a significant enhancement of vascularization. Furthermore, these hydrogels showed remarkable improvements in granulation tissue thickness and collagen deposition. As a result, they exhibited enhanced wound healing and superior therapeutic effectiveness compared to commercially available Tegaderm membranes.234 Yi et al. developed a novel hydrogel-based wound dressing by incorporating TA and EPL modified bacterial cellulose into a PVA and borax matrix. This hydrogel demonstrated excellent adhesion, strong antibacterial properties, favorable biocompatibility, and potent hemostatic effects. Moreover, it accelerated the healing of full-thickness skin excision wounds by reducing inflammation and promoting collagen deposition compared to commercially available Tegaderm membranes.235 However, the application of hydrogels is limited in highly exudative wounds due to their limited liquid absorption capacity. To overcome this constraint, Panwar et al. introduced dehydrated microgel particles (μGeld) capable of rapid swelling and interconnection, leading to the formation of an integrated hydrogel upon exposure to fluids. These microgel particles, derived from carboxymethylated starch and cellulose, efficiently absorbed fluid and released silver nanoparticles to effectively control infections. This hydrogel can effectively regulate wound exudate and create a moist environment. In a murine full-thickness wound model, this hydrogel significantly enhanced wound healing.236 Another promising substance for promoting skin regeneration is Substance P (SP). However, the application of SP is hindered by its poor stability and susceptibility to oxidative reactions during production, transportation, and storage. To address this limitation, Li et al. developed an SP-conjugated chitosan hydrochloride hydrogel (CSCl-SP) that exhibited an enhanced capacity for repairing full-thickness skin defects. CSCl-SP served as a stable and controlled release system for SP. In vitro experiments showed that CSCl-SP enhanced the proliferation, migration, and synthesis of human umbilical vein endothelial cells (HUVECs) and fibroblasts. Furthermore, it upregulated genes and proteins associated with angiogenesis. Additionally, CSCl-SP significantly promoted axonal growth in Neuro2a cells. In vivo experiments demonstrated that CSCl-SP notably accelerated vascularization, ECM deposition and remodeling, and nerve regeneration. These collective effects ultimately led to a rapid and effective healing of full-thickness skin defects.237
4.2 Chronic wound
Chronic wounds are characterized by their failure to progress through the normal healing process within a timeframe of 12 weeks or longer, and they often exhibit recurring occurrences. These wounds face obstacles in their healing stages due to physiological conditions, such as bacterial infections and associated pathologies.5 There are several factors that can complicate the typical course of wound healing, including inadequate primary care, bacterial infections, diabetes, and vascular disorders. Chronic wounds encompass a variety of conditions, such as venous ulcers, diabetic foot ulcers, arterial ulcers, ischemic ulcers, and pressure ulcers. Some of these wounds may initially be triggered by traumatic events, similar to acute wounds.238,239 The presence of these associated factors hinders the healing process, reduces the quality of healing, and prolongs the overall healing duration. Chronic wounds impose significant physical and psychosocial stress on patients, leading to persistent pain and discomfort.240 Due to the accessibility of diabetic wound models, research on chronic wounds has predominantly focused on diabetic wounds. In this section, we will review the advancements in the application of hydrogels in diabetic wound healing. Diabetes mellitus (DM) is a chronic medical condition characterized by persistently high blood sugar levels due to insufficient insulin production or utilization in the body. While injuries can occur in various parts of the body, diabetic patients often experience leg and foot ulcers. These ulcers pose significant challenges for healing due to impaired circulation, which makes them susceptible to infections and increases the risk of amputation. An increasing number of researchers contend that the healing of diabetic wounds is intricately linked to the physiological and pathological aspects of diabetes.241,242 Diabetes affects a series of intrinsic pathophysiological changes within tissues and cells responsible for repair and regeneration, consequently impacting wound healing processes.243 The systemic hyperglycemia characteristic of diabetes has the potential to induce protein glycosylation, resulting in the upregulation of pro-inflammatory cytokines, peroxide-induced damage, and alterations in the ECM.244 The substantial accumulation of ROS in diabetic wounds is known to evoke a vigorous inflammatory response by impeding the migration of endogenous stem cells, phagocytes, and macrophages.245,246 Elevated blood glucose levels can also suppress the proliferation and differentiation of cells associated with wound repair (such as fibroblasts, keratinocytes, and endothelial cells), thereby impeding angiogenesis and re-epithelialization.241,244,247 Upon culturing fibroblasts derived from chronic wounds, it was observed that their mitotic capacity was markedly diminished.228,248 The presence of chronic wound exudate has been observed to inhibit the proliferation of fibroblasts and keratinocytes. Additionally, cytokine and growth factor receptors, such as type II TGF-β receptor, may be downregulated or functionally impaired in the context of chronic wounds.248–250 Due to the presence of elevated blood glucose, abnormal blood lipids, insulin resistance, and excessive inflammation in diabetic wounds, oxidative stress may occur, consequently resulting in impaired functionality and apoptosis of nerve cells within the wound site.244,245,251 Neuropathy precipitates the loss and alteration of protective sensation, leading to aberrant biomechanical loading, tissue damage, and ultimately, the development of peripheral skin ulcers.243,244,251 Particularly noteworthy is the heightened susceptibility of diabetic patients with compromised immune function to external infections.252,253 Bacterial infections are the most common hindrance to the wound healing process. The presence of bacteria in infected wounds can lead to cell death and tissue necrosis, resulting in increased and prolonged inflammation. Additionally, necrotic tissue obstructs the growth of new tissue into the wound and provides a favorable environment for bacterial growth, intensifying the severity of the infection and creating a vicious cycle.240,254 In the presence of an exacerbated infection, macrophages produce higher levels of ROS to combat the invading pathogens.255–257 Excessive production of ROS leads to the generation of various pro-inflammatory cytokines, disruption of ECM, and oxidative damage. These factors prolong the existence of M1 macrophages and impede their polarization process towards M2 macrophages.243 Moreover, excessive ROS can cause damage to normal cells and tissues, leading to malnutrition, impaired vascular regeneration, hypoxia, and neural disturbances. These factors eventually contribute to persistent inflammation and the failure of chronic wounds to heal.246,258 To address detrimental factors influencing the wound healing process in diabetic patients and to facilitate the wound healing, diverse innovative multifunctional hydrogel dressings necessitate development. These hydrogel dressings should encompass adhesive properties, antibacterial efficacy, hemostatic capabilities, promotion of angiogenesis, anti-oxidative features, anti-inflammatory effects, self-healing, conductivity, oxygen supply, and glucose reduction.
During the inflammatory phase of wound healing in diabetic patients, there is often an excessive inflammatory response, which hampers the progression of healing. Therefore, it is essential to minimize unnecessary inflammation in order to facilitate wound healing. To address this issue, Shah and colleagues conducted a study aimed at developing an injectable hydrogel composed of hyaluronic acid and Pullulan, with the inclusion of curcumin (Cur) for its potent antioxidant properties.259 This hydrogel demonstrated a dual function of inhibiting various inflammatory cells and promoting collagen deposition and epithelialization of wounds. As a result, it exhibited a synergistic effect in enhancing the healing of diabetic wounds. In another study by Shiekh and colleagues, they introduced a novel wound dressing called OxOBand, which incorporated exosomes carrying oxygen-releasing antioxidants. The dressing consisted of antioxidant polyurethane cryogels known for their high porosity and sustained oxygen-releasing capabilities. Additionally, the dressing was enriched with exosomes derived from adipose-derived stem cells (ADSCs). The hydrogel demonstrated effective mitigation of oxidative stress, thereby reducing the extent of inflammation, while also promoting epithelialization. Consequently, it significantly enhanced the healing process of diabetic wounds (Fig. 12).260 Furthermore, Zhu et al. synthesized a novel hydrogel called GelMA/SFMA/MSN-RES/PDEVs. This hydrogel was created by combining methacrylated gelatin (GelMA) and silk fibroin methacrylate (SFMA). To enhance the release kinetics of the hydrogel, mesoporous silica nanoparticles (MSNs) were introduced, and subsequently, resveratrol (RES) and platelet-derived EVs (PDEVs) were incorporated into the hydrogel. The hydrogel demonstrated the ability to downregulate pro-inflammatory cytokine expression while upregulating anti-inflammatory factors, resulting in the reduction of inflammation and oxidative stress in the wound microenvironment. Moreover, the hydrogel exhibited the capacity to promote angiogenesis, facilitating rapid healing of diabetic wounds.261 Facilitating the polarization of M1 macrophages to the M2 phenotype has been demonstrated as an effective strategy to suppress inflammation.183 In recent years, an increasing number of researchers have applied this approach to diabetic wound healing.262–264 Jiao et al. developed a hydrogel using PF-127 and sodium ascorbyl phosphate (SAP) as materials, with Wharton's jelly mesenchymal stem cells (WJMSC) encapsulated within it. This hydrogel was applied to diabetic wounds, and the results demonstrated its ability to reduce M1 macrophages and increase M2 macrophages, thereby promoting macrophage transformation. Furthermore, the hydrogel facilitated cell proliferation and angiogenesis, synergistically enhancing the rapid healing of diabetic wounds.265
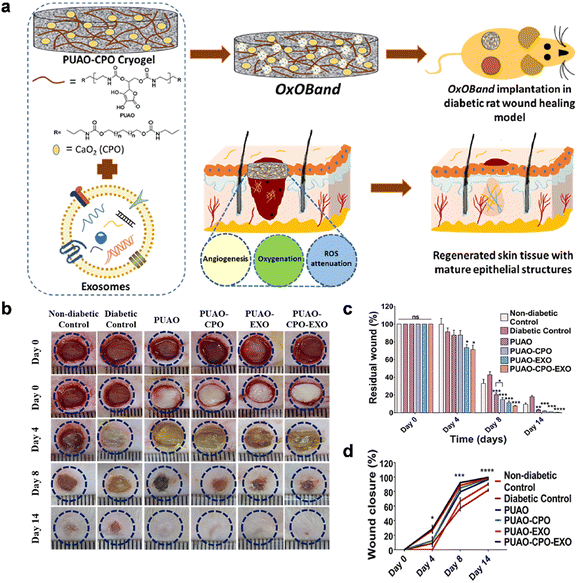 |
| Fig. 12 Hydrogel-based dressings for diabetic wound healing. (a) Schematic representation of the formation of OxOBand from oxygen releasing antioxidant PUAO-CPO cryogels with adipose-derived stem cells exosomes. (b) Representative images of wounds immediately after wounding and at day 0, day 4, day 8 and day 14 post-wounding. The blue circle represents the wound area on day 0 and is overlaid on the image at different days. (c) Quantification of the residual wound after day 4, day 8 and day 14. (d) Rate of wound closure showing a faster rate of closure in exosome treated and oxygen releasing scaffolds in comparison to diabetic as well as non-diabetic control. Reprinted and modified with permission from ref. 260. Copyright 2020 Elsevier. | |
Elevated blood glucose and elevated levels of ROS in the wound environment can impede normal wound healing through complex mechanisms. Elevated blood glucose significantly impedes the healing of diabetic wounds, as it hinders the provision of nutrients and oxygen to cells. Consequently, researchers have endeavored to ameliorate the local wound environment by employing hydrogels loaded with glucose oxidase and insulin, aiming to reduce the blood glucose levels around the wound site effectively.266,267 Developing hydrogels with responsive functionality towards glucose and/or ROS to facilitate diabetic wound healing has been a significant challenge.268–270 Xu and colleagues conducted chemical modifications to hyaluronic acid methacrylate (HAMA) by introducing phenylboronic acid (PBA) moieties, resulting in the creation of a glucose-responsive HA derivative called HAMA-PBA. Subsequently, a glucose-responsive hydrogel dressing, HAMA-PBA/catechol (HMPC), was synthesized by forming boronic ester bonds between HAMA-PBA and catechol. This hydrogel exhibited glucose-responsive behavior and released catechol, displaying significant antioxidant properties. It effectively scavenged intracellular ROS and protected cells against oxidative stress damage. In vitro and in vivo experiments confirmed that HMPC hydrogel significantly alleviated inflammatory responses, enhanced the wound healing process in diabetic wounds.271 Hypoxia is recognized as a key contributing factor to impaired healing in diabetic wounds.272 Pu et al. developed a novel system for promoting wound healing in diabetic wounds by combining MnO2 nanosheets and a dual-network hydrogel composed of silk fibroin and carboxymethyl cellulose (CMC). The MnO2 nanosheets, encapsulated within the hydrogel, have the ability to catalyze excessive ROS in the wound environment, converting them into essential oxygen. This process helped alleviate oxidative stress, promote angiogenesis, and mitigate inflammation in vivo. The system demonstrated superior potential in enhancing the healing of diabetic wounds when compared to commercially available dressings.273 Similarly, Xu et al. introduced a multifunctional hydrogel loaded with platelet-rich plasma (PRP) and possessing dual-responsiveness to both ROS and glucose. This hydrogel exhibited accelerated degradation in conditions of oxidative stress and/or hyperglycemia, leading to the release of various cytokines derived from activated blood platelets. This dynamic response enabled the rapid resolution of inflammation, polarization of macrophages toward the anti-inflammatory M2 phenotype, enhanced migration and proliferation of fibroblasts, and increased angiogenesis in diabetic wounds. Overall, this novel hydrogel demonstrated high efficiency in promoting healing of diabetic wounds.274
The treatment of infected diabetic ulcers requires a combined approach involving antimicrobial therapy and tissue repair. In recent years, a multitude of hydrogel-based dressings endowed with antimicrobial properties have been developed for the treatment of diabetic wounds.275–277 Liu et al. created an injectable and self-healing hydrogel by combining chitosan with Ag+ and Cu2+. The chitosan chains were cross-linked with these metal ions through specific chemical interactions, resulting in the formation of the CS–Ag–Cu hydrogel. This hydrogel enabled the sustained release of Ag+ and Cu2+, providing antimicrobial activity and promoting vascularization. In in vivo experiments, the CS–Ag–Cu hydrogel significantly accelerated tissue repair in diabetic infected wounds, demonstrating its effectiveness in enhancing the healing process.278 Qin et al. developed a dual-layered adhesive film composed of sodium alginate/carboxymethyl chitosan (SA/CMCS). The core of the film contained a small intestine submucosa (SIS) hydrogel composite dressing loaded with SA-basic fibroblast growth factor (SA-bFGF) microspheres. Additionally, the film was integrated with a graphene oxide (GO)-based antisense transformation system. The SIS-based hydrogel stimulated vascularization, collagen deposition, and immune regulation, facilitating diabetic wound healing. The GO-based transformation system exhibited effective antibacterial activity in the treatment of diabetic infected wounds through post-transformation regulation. Furthermore, the SA/CMCS film provided stable adhesion to the wound, creating a moist wound environment that facilitated effective wound recovery.279 Wang et al. developed an injectable multifunctional hydrogel called FEMI hydrogel to tackle multidrug-resistant (MDR) bacterial infections in diabetic wounds. The FEMI hydrogel was formulated by incorporating EPL and MnO2 nanosheets with a “nanoknife-like” structure. This combination endowed the hydrogel with exceptional antimicrobial properties against MDR bacteria. Additionally, the hydrogel improved the oxidative-damaged microenvironment commonly observed in diabetic wounds. By utilizing a pH/redox dual-responsive mechanism, the hydrogel achieved sustained and spatiotemporal insulin release, thereby regulating blood glucose levels. In vivo experiments demonstrated the positive effect of the FEMI hydrogel on promoting the healing of diabetic wounds with MDR bacterial infections.280 Biofilm formation by bacteria on infected wounds is a significant challenge that impedes the effectiveness of conventional treatments. To address this issue, researchers have successfully developed various novel and effective antimicrobial hydrogels.281–283 Cai et al. developed a eucalyptus essential oil nanoemulsion hydrogel specifically for this purpose. In vitro experiments demonstrated that the hydrogel displayed strong antimicrobial activity and inhibited biofilm formation. Moreover, in vivo experiments further confirmed its effectiveness in promoting the healing of infected wounds.284
Impaired wound vascularization is a significant contributing factor to the delayed healing of diabetic wounds. Stimulating angiogenesis in these wounds is crucial to supply essential nutrients and oxygen to the cells involved in the healing process, thereby playing a vital role in diabetic wound healing.129,285 One effective strategy to enhance angiogenesis is the controlled release of angiogenic growth factors, such as VEGF.286 Freudenberg et al. developed a VEGF-releasing hydrogel, where VEGF was integrated and protected within the supportive starPEG-heparin hydrogel matrix. This approach facilitated neovascularization in diabetic wounds, resulting in accelerated healing.285 Moreover, promising approaches involve the delivery of drugs or stem cells that induce the upregulation of angiogenesis-associated genes.287,288 Growth factors have gained substantial attention in the field of drug delivery due to their pivotal role in promoting wound healing. However, the high presence of proteases in diabetic wounds poses a challenge as they can degrade endogenous growth factors and their receptors.289 Hence, a crucial focus for researchers is the development of strategies to deliver growth factors while preserving their activity. The hydrogel system offers a means to maintain the bioactivity of growth factors, facilitating their delivery to diabetic wounds and promoting angiogenesis at the wound site.290 Hao et al. proposed a CMCS hydrogel that incorporated fibroblast growth factor. Experimental findings revealed that the hydrogel promoted neovascularization in diabetic wounds by upregulating the expression of CD31 and CD34, thereby enhancing the wound healing process.291 Additionally, certain small molecule drugs have been encapsulated within hydrogels to promote angiogenesis.292 Shao et al. synthesized a hydrogel by utilizing 3-carboxyl-4-fluorophenylboronic acid-grafted quaternized chitosan and PVA as precursors. The hydrogel was designed to incorporate gelatin microspheres loaded with the pro-angiogenic drug desferrioxamine. In vivo experiments demonstrated that this hydrogel upregulated the expression of HIF-1 and VEGF, leading to accelerated angiogenesis and enhanced collagen deposition, ultimately accelerating the healing process of diabetic wounds.293 Mesenchymal stem cells (MSCs) have the potential to secrete various growth factors, such as bFGF and TGF-b1, which can promote diabetic wound healing. However, their survival rate is often low, and when directly applied to the wound site, the survival rate after 24 hours can sometimes be less than 10%.294,295 Incorporating MSCs into hydrogels has proven to be an effective approach to promote angiogenesis at the wound site, thereby facilitating rapid healing of diabetic wounds.296–298 Furthermore, the concurrent application of adipose-derived stem cells (ASCs) and hydrogels has shown substantial potential in aiding the healing of diabetic wounds.299,300 In recent years, exosomes have emerged as a prominent area of research in the biomedical field, and the use of exosomes derived from different stem cell sources for angiogenesis has shown particularly promising results. Previous studies have demonstrated that delivering exosomes through hydrogels can effectively stimulate neovascularization in diabetic wounds, thereby facilitating the wound healing process.301,302
4.3 Wound monitoring
The dynamic monitoring of physiological parameters and the real-time quantitative and qualitative assessment of wound healing play a crucial role in enhancing the effectiveness of wound treatment, conducting clinical experiments, and expanding treatment options for wound dressings. Consequently, researchers have proposed the development of multifunctional hydrogel-based dressings to enable comprehensive monitoring of various parameters in wound healing, including pH levels, pressure, infection status, temperature, and glucose levels.303–307 A novel method has been developed for the in situ biosynthesis of functional conjugated polymers within artificial hydrogels, utilizing the biological microenvironment. This approach allowed for the real-time monitoring of wound infection and the inhibition of bacteria. Specifically, a readily polymerizable derivative of aniline dimer, named N-(3-sulfopropyl) p-aminodiphenylamine (SPA), was strategically polymerized in situ to form a polySPA (PSPA) polymer within a calcium alginate hydrogel. This polymerization process was initiated by the catalytic activity of H2O2, which is overexpressed in infected wounds, resulting in the generation of hydroxyl radicals. The presence of preloaded horseradish peroxidase (HRP) facilitated this catalytic reaction. The resulting PSPA polymer exhibited remarkable near-infrared absorption properties, enabling its utilization in the real-time monitoring of H2O2 through visual observation and the analysis of photoacoustic signals. Additionally, the near-infrared light-mediated photothermal effect of PSPA was employed to effectively inhibit bacterial growth (Fig. 13a–c).308 Jiang et al. developed a temperature-responsive hydrogel wound dressing using a polymer-based approach. The hydrogel comprised of polyacrylic acid (PAA)-grafted poly(N-isopropylacrylamide), vinyl-based polyacrylamide, and silver nanowires. To enable wireless transmission of temperature changes, the hydrogel matrix was ingeniously connected to a Bluetooth module, allowing data transfer to a smart device. This innovative design combined conductive hydrogel dressings with wireless transmission technology, successfully achieving real-time wireless monitoring of wound temperature. This novel dressing holds promise for early detection of signs of infection during the wound healing process.309 Zong et al. developed multifunctional chitosan hydrogels using a polymerized ionic liquid and a near-infrared fluorescent probe known as PIL-CS. These hydrogels had the ability to visualize wound pH in real-time through near-infrared fluorescence imaging. By monitoring changes in pH, the hydrogel could provide insights into the healing process of diabetic wounds. The hydrogel also demonstrated pH-responsive drug release, specifically for antioxidants that can eliminate ROS. This drug release capability could further promote the healing process of diabetic wounds by reducing oxidative stress (Fig. 13d).310 On the other hand, Liu et al. created a highly transparent conductive hydrogel patch by incorporating poly(tannic acid)-doped polypyrrole nanofibrils within a poly(acrylamide-acrylated adenine) polymer matrix. This patch had the ability to indirectly monitor glucose levels at the wound site. By analyzing changes in glucose levels, the hydrogel patch could provide information on the individual's blood glucose levels, which is crucial for diabetic patients. Additionally, the hydrogel patch offered timely feedback on the individual's level of physical activity. This capability could be useful in managing diabetes and providing personalized care based on glucose levels and activity levels.311 Patients suffering from pressure ulcers often experience limited mobility, making them highly susceptible to secondary pressure injuries and wound infections. In order to address this challenge, Li et al. developed a novel hydrogel-based dressing using a composite of PVA/acrylamide-ionic liquid. This innovative dressing leveraged the inherent antibacterial properties and electrical conductivity of imidazolidine ionic liquids. Remarkably, the hydrogel-based dressing exhibited a high sensitivity to pressure stimuli. Moreover, it demonstrated real-time responsiveness, consistent signal output, and exceptional mechanical characteristics. By implementing this dressing, extensive monitoring of human movement on a large scale became possible, enabling the prompt transmission of wound pressure information to healthcare providers. Consequently, the dressing played a critical role in preventing the occurrence of secondary pressure injuries.312
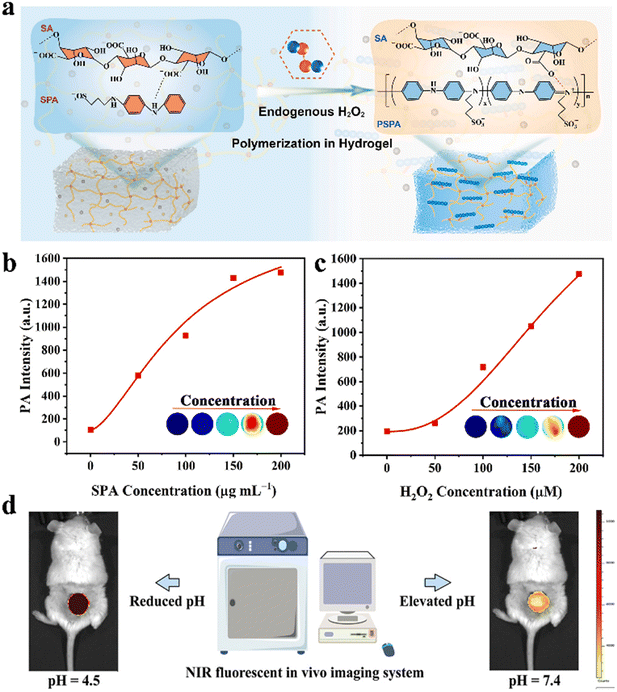 |
| Fig. 13 The wound monitoring of hydrogel-based dressings. (a) Schematic illustration of H2O2-activated in situ polymerization of SPA in the CA hydrogel. PA intensity of the CA@SPA-HRP hydrogel in the presence of 100 μM H2O2 and different concentrations of SPA (b) as well as in the presence of different concentrations of H2O2 and 100 μg mL−1 SPA (c). Reprinted and modified with permission from ref. 308. Copyright 2022 Elsevier. (d) Real-time imaging schematic of NIR fluorescent hydrogel PIL-CS on in vivo diabetic mice. Reprinted with permission from ref. 310. Copyright 2023 Wiley-VCH. | |
5. Conclusions and future perspectives
Wound healing, especially in the context of chronic wounds, is a complex and multi-step process, posing challenges to the development of therapeutic approaches for wound management. This has driven researchers to delve deeper into the underlying mechanisms of wound healing, leading to the exploration of new treatment modalities aimed at achieving optimal wound healing outcomes. Despite extensive research into wound treatment strategies and increased understanding of wound healing, managing cutaneous wounds, especially chronic wounds, remains a significant challenge in the field of wound care. While various therapeutic strategies have emerged, none have proven universally effective in fully healing all types of wounds.
In recent years, hydrogels have gained considerable attention in the literature due to their diverse advantages. Several hydrogel-based dressings, possessing distinct functionalities, have been investigated for their potential in wound healing, resulting in remarkable achievements. Therefore, this article aims to discuss the intricacies and characteristics of wound healing processes, followed by a comprehensive overview of the enhanced functionalities of hydrogel-based dressings and the strategies employed to achieve these functionalities. Lastly, the challenges encountered during the healing progression of different wound types, particularly diabetic wounds, are examined, culminating in a summary of the advancements in hydrogel-based dressing applications in diverse wound management scenarios.
The utilization of multifunctional hydrogel-based dressings to enhance wound healing stands as a prominent domain for future investigation. Despite the notable progress achieved in current research endeavors, there remains a multitude of significant issues that warrant exploration in the context of forthcoming clinical applications (Fig. 14). First, given the varied characteristics of distinct wound types, the mechanisms underlying their impaired healing vary, thus precluding a one-size-fits-all hydrogel-based dressing for all wound types. Additionally, patients exhibit varying reactivity to hydrogel-based dressings, with personalized hydrogel-based dressings remaining in a nascent stage. Moreover, wound healing constitutes a multifaceted and sequential physiological progression, wherein each stage entails distinct functional requisites for wound dressings. To date, researchers have not devised hydrogel-based dressings that comprehensively cater to the diverse requirements of all phases of wound healing. Consequently, the development of individualized dressings tailored to the unique wounds of diverse patients holds profound clinical significance. To realize this objective, hydrogel-based dressings must possess the capacity to monitor and acquire precise wound-specific information, thus facilitating the formulation of pertinent hydrogel constructs. With the evolution of medical technology and further probing into wound healing dynamics, there is a prospect that hydrogel materials will be capable of real-time monitoring of wound conditions, adeptly executing requisite functionalities, and adroitly responding to alterations within the wound environment, thereby ushering in a paradigm of authentic personalized management. Second, despite the evident merits associated with the application of multifunctional hydrogels, their utilization remains predominantly at the foundational stage of animal experimentation, with a notable dearth of extensive clinical studies to substantiate their therapeutic efficacy and safety. Currently, researchers predominantly opt for establishing wound models in rats or mice; however, there exist disparities between rodent and human skin. For instance, rodent skin tends to exhibit contraction during the healing process, potentially introducing variability into experimental outcomes. Although large animals (such as pig) and humans exhibit many similarities in skin morphology and characteristics, practicality and accessibility issues have limited their widespread use for establishing wound models. In the future, it is crucial to place increased emphasis on rigorous clinical investigations to validate and complement existing research findings. Furthermore, existing research lacks in-depth exploration, as many studies have only concluded that hydrogel-based dressings promote wound healing by facilitating the resolution of inflammation, angiogenesis, collagen deposition, bacterial inhibition, among other factors. However, these studies often lack comprehensive investigations into the specific underlying mechanisms. Therefore, future research endeavors should delve deeper into elucidating the intricate mechanisms through which hydrogel-based dressings facilitate wound healing. Such efforts are essential to establish a robust foundation for their secure and effective clinical application. Moreover, researchers have combined hydrogels with various other techniques, such as nanomaterials, exosomes, stem cells, 3D bioprinting technology, and cold atmospheric plasma therapy, to enhance wound healing outcomes.313–317 Although the synergistic application of these methods has shown promising results, a more comprehensive investigation into their safety, efficacy, and underlying mechanisms is necessary. Additionally, these innovative approaches unavoidably introduce increased production costs, thereby hindering their widespread clinical adoption. Therefore, in the future, it is imperative to optimize manufacturing processes and formulations to design composite hydrogel-based dressings that are not only safer and more efficient but also cost-effective, addressing the need for improved wound management strategies.
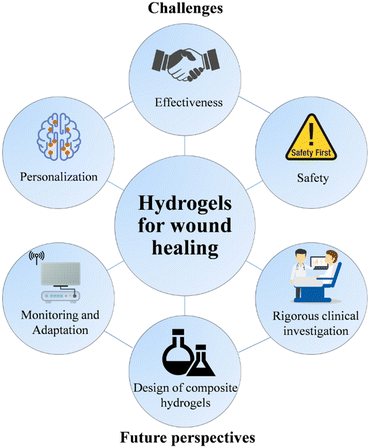 |
| Fig. 14 Current challenges and future perspectives of hydrogel dressings for wound healing. | |
Conflicts of interest
The authors declare no conflict of interest.
Acknowledgements
This work was financially supported by National Natural Science Foundation of China (22372001), Starting Fund for Scientific Research of High-Level Talents, Anhui Agricultural University (rc382108), the Key Research and Development Plan of Anhui Province (2022e07020037), Health Research Project of Anhui Province (AHWJ2022b014), the Hefei Independent Innovation Policy “Borrowed and Subsidized” Project (J2020Y02), Postgraduate Innovation Research and Practice Program of Anhui Medical University (YJS20230120), Scientific Research Project of Anhui Higher Education Institutions (2022AH051191) and Innovation and entrepreneurship training program for college students, Anhui Agricultural University (X202310364209).
References
- A. K. Dąbrowska, F. Spano, S. Derler, C. Adlhart, N. D. Spencer and R. M. Rossi, Sking Res. Technol., 2018, 24, 165–174 CrossRef PubMed.
- H. Mei, P. Yao, S. Wang, N. Li, T. Zhu, X. Chen, M. Yang, S. Zhuo, S. Chen, J. M. Wang, H. Wang, D. Xie, Y. Wu and Y. Le, Toxicol. Sci, 2017, 159, 327–338 CrossRef CAS PubMed.
- Y. Niu, Q. Li, Y. Ding, L. Dong and C. Wang, Adv. Drug Delivery Rev., 2019, 146, 190–208 CrossRef CAS PubMed.
- C. Dai, S. Shih and A. Khachemoune, J. Dermatol. Treat., 2020, 31, 639–648 CrossRef CAS PubMed.
- S. Bowers and E. Franco, Am. Fam. Physician, 2020, 101, 159–166 Search PubMed.
- J. G. Powers, C. Higham, K. Broussard and T. J. Phillips, J. Am. Acad. Dermatol., 2016, 74, 607–625 CrossRef PubMed ; quiz 625–606.
- L. M. Morton and T. J. Phillips, J. Am. Acad. Dermatol., 2016, 74, 589–605 CrossRef PubMed ; quiz 605–586.
- L. Qi, C. Zhang, B. Wang, J. Yin and S. Yan, Macromol. Biosci., 2022, 22, e2100475 CrossRef PubMed.
- S. R. Nussbaum, M. J. Carter, C. E. Fife, J. DaVanzo, R. Haught, M. Nusgart and D. Cartwright, Value Health, 2018, 21, 27–32 CrossRef PubMed.
- I. M. Balsa and W. T. Culp, Vet. Clin. North Am. Small Anim. Pract., 2015, 45, 1049–1065 CrossRef PubMed.
- M. Y. Ou, P. C. Tan, Y. Xie, K. Liu, Y. M. Gao, X. S. Yang, S. B. Zhou and Q. F. Li, Theranostics, 2022, 12, 5470–5487 CrossRef CAS PubMed.
- D. Simões, S. P. Miguel, M. P. Ribeiro, P. Coutinho, A. G. Mendonça and I. J. Correia, Eur. J. Pharm. Biopharm., 2018, 127, 130–141 CrossRef PubMed.
- A. Weisel, R. Cohen, J. A. Spector and Y. Sapir-Lekhovitser, J. Tissue Eng. Regener. Med., 2022, 16, 1173–1183 CrossRef CAS PubMed.
- C. W. Hicks, G. Q. Zhang, J. K. Canner, N. Mathioudakis, D. Coon, R. L. Sherman and C. J. Abularrage, Plast. Reconstr. Surg., 2020, 146, 893–902 CrossRef CAS PubMed.
- S. T. Haque, S. K. Saha, M. E. Haque and N. Biswas, Biomater. Sci., 2021, 9, 7705–7747 RSC.
- S. Dhivya, V. V. Padma and E. Santhini, Biomedicine, 2015, 5, 22 CrossRef PubMed.
- A. Dart, M. Bhave and P. Kingshott, Macromol. Biosci., 2019, 19, e1800488 CrossRef PubMed.
- V. Brumberg, T. Astrelina, T. Malivanova and A. Samoilov, Biomedicines, 2021, 9, 1235 CrossRef CAS PubMed.
- M. L. Costa, J. Achten, R. Knight, M. E. Png, J. Bruce, S. Dutton, J. Madan, K. Vadher, M. Dritsaki, J. Masters, L. Spoors, M. Campolier, N. Parsons, M. Fernandez, S. Jones, R. Grant and J. Nanchahal, Health Technol. Assess., 2020, 24, 1–86 Search PubMed.
- C. D. Weller, V. Team and G. Sussman, Front. Pharmacol., 2020, 11, 155 CrossRef CAS PubMed.
- M. G. Mehrabani, R. Karimian, R. Rakhshaei, F. Pakdel, H. Eslami, V. Fakhrzadeh, M. Rahimi, R. Salehi and H. S. Kafil, Int. J. Biol. Macromol., 2018, 116, 966–976 CrossRef CAS PubMed.
- J. Zhu, R. Hou, M. Liu, L. Wang, W. Chen, Y. Sun, W. Wei and S. Ye, Mater. Today Sustain., 2022, 18, 100125 CrossRef.
- M. E. Aljghami, S. Saboor and S. Amini-Nik, Ann. Biomed. Eng., 2019, 47, 659–675 CrossRef PubMed.
- Q. Wei, J. Duan, G. Ma, W. Zhang, Q. Wang and Z. Hu, J. Mater. Chem. B, 2019, 7, 2220–2225 RSC.
- S. Y. Lee, J. Ma, T. S. Khoo, N. Abdullah, N. N. F. Nik Md Noordin Kahar, Z. A. Abdul Hamid and M. Mustapha, Front. Bioeng. Biotechnol., 2021, 9, 735090 CrossRef PubMed.
- O. Wichterle and D. Lím, Nature, 1960, 185, 117–118 CrossRef.
- S. Alven and B. A. Aderibigbe, Int. J. Mol. Sci., 2020, 21, 9656 CrossRef CAS PubMed.
- L. E. Kass and J. Nguyen, Wiley Interdiscip. Rev.: Nanomed. Nanobiotechnol., 2022, 14, e1756 CAS.
- M. F. P. Graça, S. P. Miguel, C. S. D. Cabral and I. J. Correia, Carbohydr. Polym., 2020, 241, 116364 CrossRef PubMed.
- W. Liu, R. Gao, C. Yang, Z. Feng, W. Ou-Yang, X. Pan, P. Huang, C. Zhang, D. Kong and W. Wang, Sci. Adv., 2022, 8, eabn7006 CrossRef CAS PubMed.
- J. Ye, W. Pei, J. Zhu, P. Li, H. Liu, L. Gao, C. Ma, R. Gu, S. Ye and D. Zhao, Mater. Adv., 2023, 4, 5431–5452 RSC.
- J. Zhu, W. Chen, Y. Sun, X. Huang, R. Chu, R. Wang, D. Zhou and S. Ye, Mater. Adv., 2022, 3, 7687–7708 RSC.
- W. Chen, R. Chu, H. Li, T. Hua, H. Chen, R. Li, D. Zhou, S. Cao, S. Ye and H. Li, Mater. Adv., 2023, 4, 2918–2925 RSC.
- J. Qu, X. Zhao, Y. Liang, T. Zhang, P. X. Ma and B. Guo, Biomaterials, 2018, 183, 185–199 CrossRef CAS PubMed.
- Y. Li, W. Song, L. Kong, Y. He and H. Li, Small, 2023, e2309599, DOI:10.1002/smll.202309599.
- H. N. Wilkinson and M. J. Hardman, Open Biol., 2020, 10, 200223 CrossRef CAS PubMed.
- H. Sorg, D. J. Tilkorn, S. Hager, J. Hauser and U. Mirastschijski, Eur. Surg. Res., 2017, 58, 81–94 CrossRef PubMed.
- A. Opneja, S. Kapoor and E. X. Stavrou, Thromb. Res., 2019, 179, 56–63 CrossRef CAS PubMed.
- M. Zhao, J. Shi, W. Cai, K. Liu, K. Shen, Z. Li, Y. Wang and D. Hu, Int. J. Nanomed., 2021, 16, 2647–2665 CrossRef PubMed.
- S. Patel, S. Srivastava, M. R. Singh and D. Singh, Biomed. Pharmacother., 2019, 112, 108615 CrossRef CAS PubMed.
- P. H. Wang, B. S. Huang, H. C. Horng, C. C. Yeh and Y. J. Chen, J. Chin. Med. Assoc., 2018, 81, 94–101 CrossRef PubMed.
- A. E. Boniakowski, A. S. Kimball, B. N. Jacobs, S. L. Kunkel and K. A. Gallagher, J. Immunol., 2017, 199, 17–24 CrossRef CAS PubMed.
- A. E. Fetz and G. L. Bowlin, Tissue Eng., Part B, 2022, 28, 437–450 CrossRef CAS PubMed.
- A. Doni, C. Garlanda and A. Mantovani, Semin. Immunol., 2016, 28, 570–577 CrossRef CAS PubMed.
- J. C. Brazil, M. Quiros, A. Nusrat and C. A. Parkos, J. Clin. Invest., 2019, 129, 2983–2993 CrossRef PubMed.
- E. Kanno, K. Kawakami, M. Ritsu, K. Ishii, H. Tanno, S. Toriyabe, Y. Imai, R. Maruyama and M. Tachi, Wound Repair Regen., 2011, 19, 608–621 CrossRef PubMed.
- A. Das, M. Sinha, S. Datta, M. Abas, S. Chaffee, C. K. Sen and S. Roy, Am. J. Pathol., 2015, 185, 2596–2606 CrossRef CAS PubMed.
- N. X. Landén, D. Li and M. Ståhle, Cell. Mol. Life Sci., 2016, 73, 3861–3885 CrossRef PubMed.
- A. El Ayadi, J. W. Jay and A. Prasai, Int. J. Mol. Sci., 2020, 21, 1105 CrossRef CAS PubMed.
- D. Kempuraj and R. R. Mohan, Biomedicines, 2022, 10, 339 CrossRef CAS PubMed.
- L. E. Tracy, R. A. Minasian and E. J. Caterson, Adv. Wound Care, 2016, 5, 119–136 CrossRef PubMed.
- T. J. Shaw and P. Martin, Curr. Opin. Cell Biol., 2016, 42, 29–37 CrossRef CAS PubMed.
- J. Li, J. Chen and R. Kirsner, Clin. Dermatol., 2007, 25, 9–18 CrossRef PubMed.
- H. Bi, H. Li, C. Zhang, Y. Mao, F. Nie, Y. Xing, W. Sha, X. Wang, D. M. Irwin and H. Tan, Stem Cell Res. Ther., 2019, 10, 302 CrossRef PubMed.
- S. P. Huang, M. S. Wu, C. T. Shun, H. P. Wang, C. Y. Hsieh, M. L. Kuo and J. T. Lin, J. Biomed. Sci., 2005, 12, 229–241 CrossRef CAS PubMed.
- A. Rattner, J. Williams and J. Nathans, J. Clin. Invest., 2019, 129, 3807–3820 CrossRef PubMed.
- Y. Peng, Y. Wang, N. Tang, D. Sun, Y. Lan, Z. Yu, X. Zhao, L. Feng, B. Zhang, L. Jin, F. Yu, X. Ma and C. Lv, J. Exp. Clin. Cancer Res., 2018, 37, 248 CrossRef CAS PubMed.
- L. Zhu, J. Qian, Y. Jiang, T. Yang, Q. Duan and X. Xiao, Front. Immunol., 2021, 12, 736153 CrossRef CAS PubMed.
- T. Hongu, M. Pein, J. Insua-Rodríguez, E. Gutjahr, G. Mattavelli, J. Meier, K. Decker, A. Descot, M. Bozza, R. Harbottle, A. Trumpp, H. P. Sinn, A. Riedel and T. Oskarsson, Nat. Cancer, 2022, 3, 486–504 CrossRef CAS PubMed.
- T. J. Keane, C. M. Horejs and M. M. Stevens, Adv. Drug Delivery Rev., 2018, 129, 407–419 CrossRef CAS PubMed.
- L. Moretti, J. Stalfort, T. H. Barker and D. Abebayehu, J. Biol. Chem., 2022, 298, 101530 CrossRef CAS PubMed.
- S. S. Mathew-Steiner, S. Roy and C. K. Sen, Bioengineering, 2021, 8, 63 CrossRef CAS PubMed.
- M. V. Plikus, C. F. Guerrero-Juarez, M. Ito, Y. R. Li, P. H. Dedhia, Y. Zheng, M. Shao, D. L. Gay, R. Ramos, T. C. Hsi, J. W. Oh, X. Wang, A. Ramirez, S. E. Konopelski, A. Elzein, A. Wang, R. J. Supapannachart, H. L. Lee, C. H. Lim, A. Nace, A. Guo, E. Treffeisen, T. Andl, R. N. Ramirez, R. Murad, S. Offermanns, D. Metzger, P. Chambon, A. D. Widgerow, T. L. Tuan, A. Mortazavi, R. K. Gupta, B. A. Hamilton, S. E. Millar, P. Seale, W. S. Pear, M. A. Lazar and G. Cotsarelis, Science, 2017, 355, 748–752 CrossRef CAS PubMed.
- K. Walsh, R. C. Smith and H. S. Kim, Circ. Res., 2000, 87, 184–188 CrossRef CAS PubMed.
- M. Byrne and A. Aly, Aesthetic Surg. J., 2019, 39, S67–s72 CrossRef PubMed.
- Y. Zou, C. Zhou, Z. Li, X. Han, L. Tong, T. Liu, L. Xiong, L. Bai, J. Liang, Y. Fan, X. Zhang and Y. Sun, Small, 2023, 19, e2303414 CrossRef PubMed.
- Y. Yi, C. Xie, J. Liu, Y. Zheng, J. Wang and X. Lu, J. Mater. Chem. B, 2021, 9, 8739–8767 RSC.
- Y. Zhao, S. Song, X. Ren, J. Zhang, Q. Lin and Y. Zhao, Chem. Rev., 2022, 122, 5604–5640 CrossRef CAS PubMed.
- Z. Bao, C. Xian, Q. Yuan, G. Liu and J. Wu, Adv. Healthcare Mater., 2019, 8, e1900670 CrossRef PubMed.
- A. H. J. Gowda, Y. Bu, O. Kudina, K. V. Krishna, R. A. Bohara, D. Eglin and A. Pandit, Int. J. Biol. Macromol., 2020, 164, 1384–1391 CrossRef CAS PubMed.
- Q. Shao, W. Zhang, J. Qi, H. Liao, H. Guo, X. Tan and B. Chi, J. Mech. Behav. Biomed. Mater., 2023, 145, 106009 CrossRef CAS PubMed.
- Y. Jiang, X. Zhang, W. Zhang, M. Wang, L. Yan, K. Wang, L. Han and X. Lu, ACS Nano, 2022, 16, 8662–8676 CrossRef CAS PubMed.
- L. Han, X. Lu, K. Liu, K. Wang, L. Fang, L. T. Weng, H. Zhang, Y. Tang, F. Ren, C. Zhao, G. Sun, R. Liang and Z. Li, ACS Nano, 2017, 11, 2561–2574 CrossRef CAS PubMed.
- K. Liu, C. Zhang, R. Chang, Y. He, F. Guan and M. Yao, Acta Biomater., 2023, 166, 224–240 CrossRef CAS PubMed.
- V. Falanga, R. R. Isseroff, A. M. Soulika, M. Romanelli, D. Margolis, S. Kapp, M. Granick and K. Harding, Nat. Rev. Dis. Primers, 2022, 8, 50 CrossRef PubMed.
- P. P. Kalelkar, M. Riddick and A. J. García, Nat. Rev. Mater., 2022, 7, 39–54 CrossRef CAS PubMed.
- M. Stracy, O. Snitser, I. Yelin, Y. Amer, M. Parizade, R. Katz, G. Rimler, T. Wolf, E. Herzel, G. Koren, J. Kuint, B. Foxman, G. Chodick, V. Shalev and R. Kishony, Science, 2022, 375, 889–894 CrossRef CAS PubMed.
- B. Jia, G. Li, E. Cao, J. Luo, X. Zhao and H. Huang, Mater. Today Bio, 2023, 19, 100582 CrossRef CAS PubMed.
- Z. Cao, Y. Luo, Z. Li, L. Tan, X. Liu, C. Li, Y. Zheng, Z. Cui, K. W. K. Yeung, Y. Liang, S. Zhu and S. Wu, Macromol. Biosci., 2021, 21, e2000252 CrossRef PubMed.
- W. Han, C. Chen, K. Yang, H. Wang, H. Xia, Y. Zhao, Y. Teng, G. Feng and Y. M. Chen, Int. J. Biol. Macromol., 2023, 227, 373–383 CrossRef CAS PubMed.
- M. Tavakolian, J. G. Munguia-Lopez, A. Valiei, M. S. Islam, J. M. Kinsella, N. Tufenkji and T. G. M. van de Ven, ACS Appl. Mater. Interfaces, 2020, 12, 39991–40001 CrossRef CAS PubMed.
- S. Guo, Y. Ren, R. Chang, Y. He, D. Zhang, F. Guan and M. Yao, ACS Appl. Mater. Interfaces, 2022, 14, 34455–34469 CrossRef CAS PubMed.
- J. Wang, L. Wang, C. Wu, X. Pei, Y. Cong, R. Zhang and J. Fu, ACS Appl. Mater. Interfaces, 2020, 12, 46816–46826 CrossRef CAS PubMed.
- T. Hu, G.-P. Wu, H. Bu, H. Zhang, W.-X. Li, K. Song and G.-B. Jiang, Chem. Eng. J., 2022, 450, 138201 CrossRef CAS.
- L. Shang, J. Liu, Y. Wu, M. Wang, C. Fei, Y. Liu, F. Xue, L. Zhang and F. Gu, ACS Appl. Mater. Interfaces, 2023, 15, 26273–26284 CrossRef CAS PubMed.
- Y. Zhong, H. Xiao, F. Seidi and Y. Jin, Biomacromolecules, 2020, 21, 2983–3006 CrossRef CAS PubMed.
- L. Mei, D. Zhang, H. Shao, Y. Hao, T. Zhang, W. Zheng, Y. Ji, P. Ling, Y. Lu and Q. Zhou, ACS Appl. Mater. Interfaces, 2022, 14, 20538–20550 CrossRef CAS PubMed.
- A. Kapanya, R. Somsunan, R. Molloy, S. Jiranusornkul, W. Leewattanapasuk, L. Jongpaiboonkit and Y. Kong, J. Biomater. Sci., Polym. Ed., 2020, 31, 895–909 CrossRef CAS PubMed.
- J. Zhang, C. Hurren, Z. Lu and D. Wang, Int. J. Biol. Macromol., 2022, 222, 1723–1733 CrossRef CAS PubMed.
- S. Chattopadhyay and R. T. Raines, Biopolymers, 2014, 101, 821–833 CrossRef CAS PubMed.
- P. Espinal, E. Fusté, J. M. Sierra, G. Jiménez-Galisteo, T. Vinuesa and M. Viñas, Expert Opin. Biol. Ther., 2023, 23, 641–650 CrossRef CAS PubMed.
- S. Wu, Y. Yang, S. Wang, C. Dong, X. Zhang, R. Zhang and L. Yang, Carbohydr. Polym., 2022, 278, 118994 CrossRef CAS PubMed.
- C. Wang, T. Hong, P. Cui, J. Wang and J. Xia, Adv. Drug Delivery Rev., 2021, 175, 113818 CrossRef CAS PubMed.
- L. Hong, X. Liu, L. Tan, Z. Cui, X. Yang, Y. Liang, Z. Li, S. Zhu, Y. Zheng, K. W. K. Yeung, D. Jing, D. Zheng, X. Wang and S. Wu, Adv. Healthcare Mater., 2019, 8, e1900835 CrossRef PubMed.
- J. Xi, G. Wei, Q. Wu, Z. Xu, Y. Liu, J. Han, L. Fan and L. Gao, Biomater. Sci., 2019, 7, 4131–4141 RSC.
- F. Perreault, A. F. de Faria, S. Nejati and M. Elimelech, ACS Nano, 2015, 9, 7226–7236 CrossRef CAS PubMed.
- Q. Jia, Q. Song, P. Li and W. Huang, Adv. Healthcare Mater., 2019, 8, e1900608 CrossRef PubMed.
- U. Chilakamarthi and L. Giribabu, Chem. Rec., 2017, 17, 775–802 CrossRef CAS PubMed.
- B. Mai, M. Jia, S. Liu, Z. Sheng, M. Li, Y. Gao, X. Wang, Q. Liu and P. Wang, ACS Appl. Mater. Interfaces, 2020, 12, 10156–10169 CrossRef CAS PubMed.
- Y. Zheng, Y. Zhu, J. Dai, J. Lei, J. You, N. Chen, L. Wang, M. Luo and J. Wu, Int. J. Biol. Macromol., 2023, 230, 123452 CrossRef CAS PubMed.
- M. Lafond, S. Yoshizawa and S. I. Umemura, J. Ultrasound Med., 2019, 38, 567–580 CrossRef PubMed.
- T. L. Szabo and P. A. Lewin, J. Ultrasound Med., 2013, 32, 573–582 CrossRef PubMed.
- Q. He, D. Liu, M. Ashokkumar, X. Ye, T. Z. Jin and M. Guo, Ultrason. Sonochem., 2021, 73, 105509 CrossRef CAS PubMed.
- D. Kessel, R. Jeffers, J. B. Fowlkes and C. Cain, Int. J. Radiat. Biol., 1994, 66, 221–228 CrossRef CAS PubMed.
- J. Roy, V. Pandey, I. Gupta and H. Shekhar, ACS Biomater. Sci. Eng., 2021, 7, 5326–5338 CrossRef CAS PubMed.
- F. Chen, H. Huang, L. Guo, Y. Zhang and T. Ma, Angew. Chem., Int. Ed., 2019, 58, 10061–10073 CrossRef CAS PubMed.
- C. Pan, J. Zhai and Z. L. Wang, Chem. Rev., 2019, 119, 9303–9359 CrossRef CAS PubMed.
- D. Liu, L. Li, B. L. Shi, B. Shi, M. D. Li, Y. Qiu, D. Zhao, Q. D. Shen and Z. Z. Zhu, Bioact. Mater., 2023, 24, 96–111 CAS.
- Y. Liang, M. Li, Y. Yang, L. Qiao, H. Xu and B. Guo, ACS Nano, 2022, 16, 3194–3207 CrossRef CAS PubMed.
- L. Pham, L. H. Dang, M. D. Truong, T. H. Nguyen, L. Le, V. T. Le, N. D. Nam, L. G. Bach, V. T. Nguyen and N. Q. Tran, Biomed. Pharmacother., 2019, 117, 109183 CrossRef CAS PubMed.
- J. Yuan, D. Zhang, X. He, Y. Ni, L. Che, J. Wu, B. Wu, Y. Wang, S. Wang, D. Sha, S. Y. Zheng and J. Yang, Int. J. Biol. Macromol., 2021, 190, 754–762 CrossRef CAS PubMed.
- Z. Tan, X. Li, C. Yu, M. Yao, Z. Zhao, B. Guo, L. Liang, Y. Wei, F. Yao, H. Zhang and J. Li, Int. J. Biol. Macromol., 2023, 232, 123449 CrossRef CAS PubMed.
- Z. Qiao, X. Lv, S. He, S. Bai, X. Liu, L. Hou, J. He, D. Tong, R. Ruan, J. Zhang, J. Ding and H. Yang, Bioact. Mater., 2021, 6, 2829–2840 CAS.
- J. Du, J. Wang, T. Xu, H. Yao, L. Yu and D. Huang, Molecules, 2023, 28, 5264 CrossRef CAS PubMed.
- Y. Guo, M. Wang, Q. Liu, G. Liu, S. Wang and J. Li, Theranostics, 2023, 13, 161–196 CrossRef CAS PubMed.
- Y. Chen, L. Wu, P. Li, X. Hao, X. Yang, G. Xi, W. Liu, Y. Feng, H. He and C. Shi, Macromol. Biosci., 2020, 20, e1900370 CrossRef PubMed.
- W. Peng, D. Li, K. Dai, Y. Wang, P. Song, H. Li, P. Tang, Z. Zhang, Z. Li, Y. Zhou and C. Zhou, Int. J. Biol. Macromol., 2022, 208, 400–408 CrossRef CAS PubMed.
- T. Carter, G. Qi, W. Wang, A. Nguyen, N. Cheng, Y. M. Ju, S. J. Lee, J. J. Yoo, A. Atala and X. S. Sun, Adv. Wound Care, 2021, 10, 191–203 CrossRef PubMed.
- E. Lih, J. S. Lee, K. M. Park and K. D. Park, Acta Biomater., 2012, 8, 3261–3269 CrossRef CAS PubMed.
- W. Cheng, Z. Zhang, R. Xu, P. Cai, P. Kristensen, M. Chen and Y. Huang, J. Biomed. Mater. Res., Part B, 2018, 106, 2588–2595 CrossRef CAS PubMed.
- P. Fathi, M. Sikorski, K. Christodoulides, K. Langan, Y. S. Choi, M. Titcomb, A. Ghodasara, O. Wonodi, H. Thaker, M. Vural, A. Behrens and P. Kofinas, J. Biomed. Mater. Res., Part B, 2018, 106, 1662–1671 CrossRef CAS PubMed.
- C. Wang, H. Zhou, H. Niu, X. Ma, Y. Yuan, H. Hong and C. Liu, Biomater. Sci., 2018, 6, 3318–3331 RSC.
- G. Li, K. Quan, Y. Liang, T. Li, Q. Yuan, L. Tao, Q. Xie and X. Wang, ACS Appl. Mater. Interfaces, 2016, 8, 35071–35080 CrossRef CAS PubMed.
- C. Liu, C. Liu, Z. Liu, Z. Shi, S. Liu, X. Wang, X. Wang and F. Huang, Int. J. Biol. Macromol., 2023, 224, 1091–1100 CrossRef CAS PubMed.
- G. Muñoz Taboada, P. Dosta, E. R. Edelman and N. Artzi, Adv. Mater., 2022, 34, e2203087 CrossRef PubMed.
- J. Cheng, H. Wang, J. Gao, X. Liu, M. Li, D. Wu, J. Liu, X. Wang, Z. Wang and P. Tang, Adv. Healthcare Mater., 2023, 12, e2300312 CrossRef PubMed.
- G. C. Gurtner, S. Werner, Y. Barrandon and M. T. Longaker, Nature, 2008, 453, 314–321 CrossRef CAS PubMed.
- H. Zhao, Z. Li, Y. Wang, K. Zhou, H. Li, S. Bi, Y. Wang, W. Wu, Y. Huang, B. Peng, J. Tang, B. Pan, B. Wang, Z. Chen and Z. Zhang, Front. Cell Dev. Biol., 2023, 11, 1029671 CrossRef PubMed.
- B. D. Lyttle, A. E. Vaughn, J. R. Bardill, A. Apte, L. T. Gallagher, C. Zgheib and K. W. Liechty, Front. Med., 2023, 10, 1140979 CrossRef PubMed.
- M. V. Giraudo, D. Di Francesco, M. C. Catoira, D. Cotella, L. Fusaro and F. Boccafoschi, Biomedicines, 2020, 8, 436 CrossRef CAS PubMed.
- Z. Wei, E. Volkova, M. R. Blatchley and S. Gerecht, Adv. Drug Delivery Rev., 2019, 149–150, 95–106 CrossRef CAS PubMed.
- J. Xiao, S. Chen, J. Yi, H. Zhang and G. A. Ameer, Adv. Funct. Mater., 2017, 27, 1604872 CrossRef PubMed.
- M. J. Jang, S. K. Bae, Y. S. Jung, J. C. Kim, J. S. Kim, S. K. Park, J. S. Suh, S. J. Yi, S. H. Ahn and J. O. Lim, Biomed. Mater., 2021, 16, 045013 CrossRef CAS PubMed.
- Y. Li, T. Xu, Z. Tu, W. Dai, Y. Xue, C. Tang, W. Gao, C. Mao, B. Lei and C. Lin, Theranostics, 2020, 10, 4929–4943 CrossRef CAS PubMed.
- Y. Zhang, M. Fang, W. Xie, Y. A. Zhang, C. Jiang, N. Li, L. Li, J. Tian and C. Zhou, Int. J. Biol. Macromol., 2023, 242, 125081 CrossRef CAS PubMed.
- B. M. Bakadia, A. A. Qaed Ahmed, L. Lamboni, Z. Shi, B. Mutu Mukole, R. Zheng, M. Pierre Mbang, B. Zhang, M. Gauthier and G. Yang, Bioact. Mater., 2023, 28, 74–94 CAS.
- J. Zeng, Z. Sun, F. Zeng, C. Gu and X. Chen, Mater. Today Bio, 2023, 20, 100649 CrossRef CAS PubMed.
- Y. Chen, M. R. Younis, G. He, Z. Zheng, Y. Wang, K. Xue, J. Sun, K. Liu, P. Huang and X. Wang, Adv. Healthcare Mater., 2023, 12, e2300456 CrossRef PubMed.
- J. Yang, Z. Chen, D. Pan, H. Li and J. Shen, Int. J. Nanomed., 2020, 15, 5911–5926 CrossRef CAS PubMed.
- Y. Chu, S. Chai, F. Li, C. Han, X. Sui and T. Liu, Polymers, 2022, 14, 2454 CrossRef CAS PubMed.
- L. Sheng, Z. Zhang, Y. Zhang, E. Wang, B. Ma, Q. Xu, L. Ma, M. Zhang, G. Pei and J. Chang, Biomaterials, 2021, 264, 120414 CrossRef CAS PubMed.
- X. Shi, T. Zhou, S. Huang, Y. Yao, P. Xu, S. Hu, C. Tu, W. Yin, C. Gao and J. Ye, Acta Biomater., 2023, 158, 266–280 CrossRef CAS PubMed.
- Z. Xu, S. Han, Z. Gu and J. Wu, Adv. Healthcare Mater., 2020, 9, e1901502 CrossRef PubMed.
- K. Khorsandi, R. Hosseinzadeh, H. Esfahani, K. Zandsalimi, F. K. Shahidi and H. Abrahamse, Inflammation Regener., 2022, 42, 40 CrossRef CAS PubMed.
- S. Polaka, P. Katare, B. Pawar, N. Vasdev, T. Gupta, K. Rajpoot, P. Sengupta and R. K. Tekade, ACS Omega, 2022, 7, 30657–30672 CrossRef CAS PubMed.
- N. Asadi, H. Pazoki-Toroudi, A. R. Del Bakhshayesh, A. Akbarzadeh, S. Davaran and N. Annabi, Int. J. Biol. Macromol., 2021, 170, 728–750 CrossRef CAS PubMed.
- J. Li, Q. Chen, J. Wang, X. Pan and J. Zhang, Curr. Med. Chem., 2021, 28, 8692–8710 CrossRef CAS PubMed.
- S. Prasad, S. C. Gupta and A. K. Tyagi, Cancer Lett., 2017, 387, 95–105 CrossRef CAS PubMed.
- N. Singh, M. A. Savanur, S. Srivastava, P. D'Silva and G. Mugesh, Nanoscale, 2019, 11, 3855–3863 RSC.
- J. Zeng, G. Du, X. Shao, K. N. Feng and Y. Zeng, Int. J. Biol. Macromol., 2019, 134, 139–145 CrossRef CAS PubMed.
- S. Xiang, P. Yang, H. Guo, S. Zhang, X. Zhang, F. Zhu and Y. Li, Macromol. Rapid Commun., 2017, 38, 1700446 CrossRef PubMed.
- Y. Li, S. Wang, Y. Li, G. Zhang, T. Wu, Y. Wei, X. Cao, H. Yan, P. Liang, Z. Yan, Y. Guo and Y. Zhang, Biomed. Mater., 2023, 18, 045007 CrossRef PubMed.
- N. Yu, Y. Li, Y. Wang, H. Xu, F. Ye and Q. Fu, Burns, 2022, 48, 902–914 CrossRef PubMed.
- J. Jing, S. Liang, Y. Yan, X. Tian and X. Li, ACS Biomater. Sci. Eng., 2019, 5, 4601–4611 CrossRef CAS PubMed.
- M. Wang, Z. Deng, Y. Guo and P. Xu, Nanoscale Adv., 2022, 5, 27–45 RSC.
- L. Ma, Y. Tan, X. Chen, Y. Ran, Q. Tong, L. Tang, W. Su, X. Wang and X. Li, Carbohydr. Polym., 2022, 293, 119733 CrossRef CAS PubMed.
- J. Xu, M. R. Younis, Z. Zhang, Y. Feng, L. Su, Y. Que, Y. Jiao, C. Fan, J. Chang, S. Ni and C. Yang, ACS Appl. Mater. Interfaces, 2023, 15, 7841–7854 CrossRef CAS PubMed.
- L. Shang, Y. Yu, Y. Jiang, X. Liu, N. Sui, D. Yang and Z. Zhu, ACS Nano, 2023, 17, 15962–15977 CrossRef CAS PubMed.
- J. H. Ryu, Y. Lee, W. H. Kong, T. G. Kim, T. G. Park and H. Lee, Biomacromolecules, 2011, 12, 2653–2659 CrossRef CAS PubMed.
- Y. Liang, X. Zhao, P. X. Ma, B. Guo, Y. Du and X. Han, J. Colloid Interface Sci., 2019, 536, 224–234 CrossRef CAS PubMed.
- Y. Zhu, Z. Cankova, M. Iwanaszko, S. Lichtor, M. Mrksich and G. A. Ameer, Proc. Natl. Acad. Sci. U. S. A., 2018, 115, 6816–6821 CrossRef CAS PubMed.
- A. Naskar and K. S. Kim, Pharmaceutics, 2020, 12, 499 CrossRef CAS PubMed.
- Z. Zhou, T. Deng, M. Tao, L. Lin, L. Sun, X. Song, D. Gao, J. Li, Z. Wang, X. Wang, J. Li, Z. Jiang, L. Luo, L. Yang and M. Wu, Biomaterials, 2023, 299, 122141 CrossRef CAS PubMed.
- L. Dong, C. Huang, B. Zhao, G. Hu, Y. Huang, X. Zhang, X. Hu, Y. Wang, X. Sun, W. Qian and G. Luo, J. Nanobiotechnol., 2023, 21, 213 CrossRef CAS PubMed.
- C. Huang, L. Dong, B. Zhao, Y. Lu, S. Huang, Z. Yuan, G. Luo, Y. Xu and W. Qian, Clin. Transl. Med., 2022, 12, e1094 CrossRef CAS PubMed.
- L. Deng, C. Du, P. Song, T. Chen, S. Rui, D. G. Armstrong and W. Deng, Oxid. Med. Cell. Longevity, 2021, 2021, 8852759 Search PubMed.
- M. Chang and T. T. Nguyen, Acc. Chem. Res., 2021, 54, 1080–1093 CrossRef CAS PubMed.
- L. Xiong, J. Huang, S. Wang, Q. Yuan, D. Yang, Z. Zheng, Y. Wu, C. Wu, Y. Gao, L. Zou and G. Hu, Arch. Toxicol., 2022, 96, 767–781 CrossRef CAS PubMed.
- Q. Xu, M. Venet, W. Wang, J. Creagh-Flynn, X. Wang, X. Li, Y. Gao, D. Zhou, M. Zeng, I. Lara-Sáez, S. A, H. Tai and W. Wang, ACS Appl. Mater. Interfaces, 2018, 10, 39494–39504 CrossRef CAS PubMed.
- K. Raziyeva, Y. Kim, Z. Zharkinbekov, K. Kassymbek, S. Jimi and A. Saparov, Biomolecules, 2021, 11, 700 CrossRef CAS PubMed.
- L. Schirmer, P. Atallah, U. Freudenberg and C. Werner, Adv. Sci., 2021, 8, e2100293 CrossRef PubMed.
- A. Ridiandries, J. T. M. Tan and C. A. Bursill, Int. J. Mol. Sci., 2018, 19, 3217 CrossRef PubMed.
- P. A. Rees, N. S. Greaves, M. Baguneid and A. Bayat, Adv. Wound Care, 2015, 4, 687–703 CrossRef PubMed.
- N. Lohmann, L. Schirmer, P. Atallah, E. Wandel, R. A. Ferrer, C. Werner, J. C. Simon, S. Franz and U. Freudenberg, Sci. Transl. Med., 2017, 9, eaai9044 CrossRef PubMed.
- J. M. Lord, M. J. Midwinter, Y. F. Chen, A. Belli, K. Brohi, E. J. Kovacs, L. Koenderman, P. Kubes and R. J. Lilford, Lancet, 2014, 384, 1455–1465 CrossRef CAS PubMed.
- H. C. Bygd, K. D. Forsmark and K. M. Bratlie, Biomaterials, 2015, 56, 187–197 CrossRef CAS PubMed.
- M. Kharaziha, A. Baidya and N. Annabi, Adv. Mater., 2021, 33, e2100176 CrossRef PubMed.
- D. M. Mosser and J. P. Edwards, Nat. Rev. Immunol., 2008, 8, 958–969 CrossRef CAS PubMed.
- J. Xue, S. V. Schmidt, J. Sander, A. Draffehn, W. Krebs, I. Quester, D. De Nardo, T. D. Gohel, M. Emde, L. Schmidleithner, H. Ganesan, A. Nino-Castro, M. R. Mallmann, L. Labzin, H. Theis, M. Kraut, M. Beyer, E. Latz, T. C. Freeman, T. Ulas and J. L. Schultze, Immunity, 2014, 40, 274–288 CrossRef CAS PubMed.
- C. S. Verbeke, S. Gordo, D. A. Schubert, S. A. Lewin, R. M. Desai, J. Dobbins, K. W. Wucherpfennig and D. J. Mooney, Adv. Healthcare Mater., 2017, 6, 1600773 CrossRef PubMed.
- S. M. Aitcheson, F. D. Frentiu, S. E. Hurn, K. Edwards and R. Z. Murray, Molecules, 2021, 26, 4917 CrossRef CAS PubMed.
- A. E. Louiselle, S. M. Niemiec, C. Zgheib and K. W. Liechty, Transl. Res., 2021, 236, 109–116 CrossRef CAS PubMed.
- A. Hassanshahi, M. Moradzad, S. Ghalamkari, M. Fadaei, A. J. Cowin and M. Hassanshahi, Cells, 2022, 11, 2953 CrossRef CAS PubMed.
- W. Sheng, H. Qin, T. Wang, J. Zhao, C. Fang, P. Zhang, P. Liu, A. Udduttula, H. Zeng and Y. Chen, Front. Bioeng. Biotechnol., 2023, 11, 1199939 CrossRef PubMed.
- Q. Wei, Y. Zhao, Y. Wei, Y. Wang, Z. Jin, G. Ma, Y. Jiang, W. Zhang and Z. Hu, Int. J. Biol. Macromol., 2023, 228, 99–110 CrossRef CAS PubMed.
- X. Dong, J. Chang and H. Li, J. Mater. Chem. B, 2017, 5, 5240–5250 RSC.
- W. Xie, X. Fu, F. Tang, Y. Mo, J. Cheng, H. Wang and X. Chen, J. Mater. Chem. B, 2019, 7, 940–952 RSC.
- Y. Zhu, Z. Ma, L. Kong, Y. He, H. F. Chan and H. Li, Biomaterials, 2020, 256, 120216 CrossRef CAS PubMed.
- H. Kim, S. Y. Wang, G. Kwak, Y. Yang, I. C. Kwon and S. H. Kim, Adv. Sci., 2019, 6, 1900513 CrossRef CAS PubMed.
- G. Kwak, J. Cheng, H. Kim, S. Song, S. J. Lee, Y. Yang, J. H. Jeong, J. E. Lee, P. B. Messersmith and S. H. Kim, Small, 2022, 18, e2200060 CrossRef PubMed.
- D. P. Vasconcelos, M. Costa, I. F. Amaral, M. A. Barbosa, A. P. Águas and J. N. Barbosa, Biomaterials, 2015, 37, 116–123 CrossRef CAS PubMed.
- B. Lu, X. Han, D. Zou, X. Luo, L. Liu, J. Wang, M. F. Maitz, P. Yang, N. Huang and A. Zhao, Mater. Today Bio, 2022, 16, 100392 CrossRef CAS PubMed.
- M. B. Reis, P. A. T. Pereira, G. F. Caetano, M. N. Leite, A. F. Galvão, F. W. G. Paula-Silva, M. A. C. Frade and L. H. Faccioli, PLoS One, 2017, 12, e0182381 CrossRef PubMed.
- M. C. P. Sok, N. Baker, C. McClain, H. S. Lim, T. Turner, L. Hymel, M. Ogle, C. Olingy, J. I. Palacios, J. R. Garcia, K. Srithar, A. J. García, P. Qiu and E. A. Botchwey, Biomaterials, 2021, 268, 120475 CrossRef CAS PubMed.
- Y. Tu, N. Chen, C. Li, H. Liu, R. Zhu, S. Chen, Q. Xiao, J. Liu, S. Ramakrishna and L. He, Acta Biomater., 2019, 90, 1–20 CrossRef CAS PubMed.
- Y. Bo, L. Zhang, Z. Wang, J. Shen, Z. Zhou, Y. Yang, Y. Wang, J. Qin and Y. He, ACS Biomater. Sci. Eng., 2021, 7, 5135–5143 CrossRef CAS PubMed.
- B. D. Zheng, J. Ye, Y. C. Yang, Y. Y. Huang and M. T. Xiao, Carbohydr. Polym., 2022, 275, 118770 CrossRef CAS PubMed.
- A. Zhang, Y. Liu, D. Qin, M. Sun, T. Wang and X. Chen, Int. J. Biol. Macromol., 2020, 164, 2108–2123 CrossRef CAS PubMed.
- Y. Liu and S. H. Hsu, Front. Chem., 2018, 6, 449 CrossRef CAS PubMed.
- X. Ding, L. Fan, L. Wang, M. Zhou, Y. Wang and Y. Zhao, Mater. Horiz., 2023, 10, 3929–3947 RSC.
- R. Li, C. Zhou, J. Chen, H. Luo, R. Li, D. Chen, X. Zou and W. Wang, Bioact. Mater., 2022, 18, 267–283 CAS.
- C. Wang, N. Zhao and W. Yuan, ACS Appl. Mater. Interfaces, 2020, 12, 9118–9131 CrossRef CAS PubMed.
- C. D. Meyer, C. S. Joiner and J. F. Stoddart, Chem. Soc. Rev., 2007, 36, 1705–1723 RSC.
- F. Ding, X. Shi, S. Wu, X. Liu, H. Deng, Y. Du and H. Li, Macromol. Mater. Eng., 2017, 302, 1700221 CrossRef.
- H. Cui, B. Cui, H. Chen, X. Geng, X. Geng, Z. Li, S. Cao, J. Shen and J. Li, Biomater. Sci., 2023, 11, 4226–4237 RSC.
- D. Chen, X. Liu, Y. Qi, X. Ma, Y. Wang, H. Song, Y. Zhao, W. Li and J. Qin, Colloids Surf., B, 2022, 214, 112430 CrossRef CAS PubMed.
- C. Shao, M. Wang, H. Chang, F. Xu and J. Yang, ACS Sustainable Chem. Eng., 2017, 5, 6167–6174 CrossRef CAS.
- A. Phadke, C. Zhang, B. Arman, C. C. Hsu, R. A. Mashelkar, A. K. Lele, M. J. Tauber, G. Arya and S. Varghese, Proc. Natl. Acad. Sci. U. S. A., 2012, 109, 4383–4388 CrossRef CAS PubMed.
- J. Li, Z. Wang, L. Wen, J. Nie, S. Yang, J. Xu and S. Z. D. Cheng, ACS Macro Lett., 2016, 5, 814–818 CrossRef CAS PubMed.
- H. Yu, Q. Xiao, G. Qi, F. Chen, B. Tu, S. Zhang, Y. Li, Y. Chen, H. Yu and P. Duan, Front. Bioeng. Biotechnol., 2022, 10, 855013 CrossRef PubMed.
- S. E. Wheeler, Acc. Chem. Res., 2013, 46, 1029–1038 CrossRef CAS PubMed.
- H. Huang, W. Gong, X. Wang, W. He, Y. Hou and J. Hu, Adv. Healthcare Mater., 2022, 11, e2102476 CrossRef PubMed.
- L. Han, M. Liu, B. Yan, Y. Li, J. Lan, L. Shi and R. Ran, Mater. Sci. Eng., C, 2020, 109, 110567 CrossRef CAS PubMed.
- C. Korupalli, H. Li, N. Nguyen, F. L. Mi, Y. Chang, Y. J. Lin and H. W. Sung, Adv. Healthcare Mater., 2021, 10, e2001384 CrossRef PubMed.
- S. Zhu, C. Yu, M. Zhao, N. Liu, Z. Chen, J. Liu, G. Li, Y. Deng, X. Sai, H. Huang, H. Guo, C. Chen, X. Wang, Y. Zheng, T. Sun, J. Chen, J. Zhuang and P. Zhu, Int. J. Biol. Macromol., 2022, 209, 1020–1031 CrossRef CAS PubMed.
- J. Xu, Y. L. Tsai and S. H. Hsu, Molecules, 2020, 25, 5296 CrossRef CAS PubMed.
- Y. Liang, X. Zhao, T. Hu, Y. Han and B. Guo, J. Colloid Interface Sci., 2019, 556, 514–528 CrossRef CAS PubMed.
- H. Yuk, B. Lu and X. Zhao, Chem. Soc. Rev., 2019, 48, 1642–1667 RSC.
- B. W. Walker, R. P. Lara, E. Mogadam, C. H. Yu, W. Kimball and N. Annabi, Prog. Polym. Sci., 2019, 92, 135–157 CrossRef CAS PubMed.
- C. Gao, S. Song, Y. Lv, J. Huang and Z. Zhang, Macromol. Biosci., 2022, 22, e2200051 CrossRef PubMed.
- Y. Liang, L. Qiao, B. Qiao and B. Guo, Chem. Sci., 2023, 14, 3091–3116 RSC.
- N. Saifuddin, A. Z. Raziah and A. R. Junizah, J. Chem., 2013, 2013, 676815 Search PubMed.
- H. Zhang, S. Zheng, C. Chen and D. Zhang, RSC Adv., 2021, 11, 6367–6373 RSC.
- C. Wu, L. Long, Y. Zhang, Y. Xu, Y. Lu, Z. Yang, Y. Guo, J. Zhang, X. Hu and Y. Wang, J. Controlled Release, 2022, 344, 249–260 CrossRef CAS PubMed.
- C. Wang, X. Jiang, H. J. Kim, S. Zhang, X. Zhou, Y. Chen, H. Ling, Y. Xue, Z. Chen, M. Qu, L. Ren, J. Zhu, A. Libanori, Y. Zhu, H. Kang, S. Ahadian, M. R. Dokmeci, P. Servati, X. He, Z. Gu, W. Sun and A. Khademhosseini, Biomaterials, 2022, 285, 121479 CrossRef CAS PubMed.
- R. Yu, H. Zhang and B. Guo, Nano-Micro Lett., 2021, 14, 1 Search PubMed.
- B. A. Mast and G. S. Schultz, Wound Repair Regen., 1996, 4, 411–420 CrossRef CAS PubMed.
- J. M. Shah, E. Omar, D. R. Pai and S. Sood, Indian J. Plast. Surg., 2012, 45, 220–228 CrossRef PubMed.
- M. H. Katz, A. F. Alvarez, R. S. Kirsner, W. H. Eaglstein and V. Falanga, J. Am. Acad. Dermatol., 1991, 25, 1054–1058 CrossRef CAS PubMed.
- M. Yang, X. Fei, J. Tian, L. Xu, Y. Wang and Y. Li, J. Mater. Chem. B, 2022, 10, 6026–6037 RSC.
- X. Zhao, Y. Liang, Y. Huang, J. He, Y. Han and B. Guo, Adv. Funct. Mater., 2020, 30, 1910748 CrossRef CAS.
- Y. Liang, Z. Li, Y. Huang, R. Yu and B. Guo, ACS Nano, 2021, 15, 7078–7093 CrossRef CAS PubMed.
- A. E. Rivera and J. M. Spencer, Clin. Dermatol., 2007, 25, 39–48 CrossRef PubMed.
- Y. Liang, X. Zhao, T. Hu, B. Chen, Z. Yin, P. X. Ma and B. Guo, Small, 2019, 15, e1900046 CrossRef PubMed.
- X. Yi, J. He, X. Wei, H. Li, X. Liu and F. Cheng, Int. J. Biol. Macromol., 2023, 247, 125663 CrossRef CAS PubMed.
- V. Panwar, A. Sharma, P. Murugesan, N. Salaria and D. Ghosh, Int. J. Biol. Macromol., 2023, 246, 125735 CrossRef CAS PubMed.
- H. Li, M. Li, P. Liu, K. Wang, H. Fang, J. Yin, D. Zhu, Q. Yang, J. Gao, Q. Ke, H. Yu, Y. Guo, Y. Gao and C. Zhang, Biomater. Sci., 2021, 9, 4199–4210 RSC.
- M. Horue, J. M. Silva, I. R. Berti, L. R. Brandão, H. D. S. Barud and G. R. Castro, Pharmaceutics, 2023, 15, 424 CrossRef CAS PubMed.
- S. Hema, V. V. Unni, Gayathri, B. Niranjan, S. Chandran and S. Sambhudevan, Biotechnol. J., 2023, 18, e2300006 CrossRef PubMed.
- C. N. Elangwe, S. N. Morozkina, R. O. Olekhnovich, A. Krasichkov, V. O. Polyakova and M. V. Uspenskaya, Polymers, 2022, 14, 5163 CrossRef CAS PubMed.
- B. Guo, R. Dong, Y. Liang and M. Li, Nat. Rev. Chem., 2021, 5, 773–791 CrossRef CAS PubMed.
- R. Zhao, H. Liang, E. Clarke, C. Jackson and M. Xue, Int. J. Mol. Sci., 2016, 17, 2085 CrossRef PubMed.
- S. Matoori, A. Veves and D. J. Mooney, Sci. Transl. Med., 2021, 13, eabe4839 CrossRef CAS PubMed.
- L. N. Kasiewicz and K. A. Whitehead, Bioeng. Transl. Med., 2019, 4, 75–82 CrossRef CAS PubMed.
- R. S. B. Lee, S. M. Hamlet, H. J. Moon and S. Ivanovski, Biomaterials, 2021, 267, 120464 CrossRef CAS PubMed.
- H. Zhao, J. Huang, Y. Li, X. Lv, H. Zhou, H. Wang, Y. Xu, C. Wang, J. Wang and Z. Liu, Biomaterials, 2020, 258, 120286 CrossRef CAS PubMed.
- L. Zhou, T. Min, X. Bian, Y. Dong, P. Zhang and Y. Wen, ACS Appl. Bio Mater., 2022, 5, 4055–4085 CrossRef CAS PubMed.
- N. J. Trengove, H. Bielefeldt-Ohmann and M. C. Stacey, Wound Repair Regen., 2000, 8, 13–25 CrossRef CAS PubMed.
- M. V. Mendez, J. D. Raffetto, T. Phillips, J. O. Menzoian and H. Y. Park, J. Vasc. Surg., 1999, 30, 734–743 CrossRef CAS PubMed.
- B. Bucalo, W. H. Eaglstein and V. Falanga, Wound Repair Regen., 1993, 1, 181–186 CrossRef CAS PubMed.
- W. C. Lin, C. C. Huang, S. J. Lin, M. J. Li, Y. Chang, Y. J. Lin, W. L. Wan, P. C. Shih and H. W. Sung, Biomaterials, 2017, 145, 1–8 CrossRef CAS PubMed.
- M. Walicka, M. Raczyńska, K. Marcinkowska, I. Lisicka, A. Czaicki, W. Wierzba and E. Franek, J. Diabetes Res., 2021, 2021, 8866126 Search PubMed.
- T. N. Golden and R. A. Simmons, Nat. Rev. Endocrinol., 2021, 17, 235–245 CrossRef CAS PubMed.
- A. M. Misic, S. E. Gardner and E. A. Grice, Adv. Wound Care, 2014, 3, 502–510 CrossRef PubMed.
- V. Falanga, Lancet, 2005, 366, 1736–1743 CrossRef PubMed.
- J. Z. Lim, N. S. Ng and C. Thomas, J. R. Soc. Med., 2017, 110, 104–109 CrossRef PubMed.
- H. Liu, R. Chen, P. Wang, J. Fu, Z. Tang, J. Xie, Y. Ning, J. Gao, Q. Zhong, X. Pan, D. Wang, M. Lei, X. Li, Y. Zhang, J. Wang and H. Cheng, Carbohydr. Polym., 2023, 316, 121050 CrossRef CAS PubMed.
- H. Zhang, H. Hu, Y. Dai, L. Xin, Q. Pang, S. Zhang and L. Ma, Acta Biomater., 2023, 167, 348–360 CrossRef CAS PubMed.
- S. A. Shah, M. Sohail, M. U. Minhas, S. Khan, Z. Hussain, A. Mahmood, M. Kousar, H. E. Thu, M. Abbasi and M. U. R. Kashif, Int. J. Biol. Macromol., 2021, 185, 350–368 CrossRef CAS PubMed.
- P. A. Shiekh, A. Singh and A. Kumar, Biomaterials, 2020, 249, 120020 CrossRef CAS PubMed.
- W. Zhu, Y. Dong, P. Xu, Q. Pan, K. Jia, P. Jin, M. Zhou, Y. Xu, R. Guo and B. Cheng, Acta Biomater., 2022, 154, 212–230 CrossRef CAS PubMed.
- T. Shen, K. Dai, Y. Yu, J. Wang and C. Liu, Acta Biomater., 2020, 117, 192–203 CrossRef CAS PubMed.
- L. Wang, Y. Yu, X. Zhao, Z. Zhang, X. Yuan, J. Cao, W. Meng, L. Ye, W. Lin and G. Wang, ACS Appl. Mater. Interfaces, 2022, 14, 46273–46289 CrossRef CAS PubMed.
- J. Mei, J. Zhou, L. Kong, Y. Dai, X. Zhang, W. Song and C. Zhu, J. Nanobiotechnol., 2022, 20, 232 CrossRef CAS PubMed.
- Y. Jiao, X. Chen, Y. Niu, S. Huang, J. Wang, M. Luo, G. Shi and J. Huang, Stem Cell Res. Ther., 2021, 12, 559 CrossRef CAS PubMed.
- Y. Zhao, X. Du, L. Jiang, H. Luo, F. Wang, J. Wang, L. Qiu, L. Liu, X. Liu, X. Wang, P. Jiang and J. Wang, J. Nanosci. Nanotechnol., 2020, 20, 2087–2094 CrossRef CAS PubMed.
- W. Zhou, Z. Duan, J. Zhao, R. Fu, C. Zhu and D. Fan, Bioact. Mater., 2022, 17, 1–17 CAS.
- Z. Ni, H. Yu, L. Wang, Y. Huang, H. Lu, H. Zhou and Q. Liu, ACS Appl. Mater. Interfaces, 2022, 14, 52643–52658 CrossRef CAS PubMed.
- Z. Li, Y. Zhao, H. Huang, C. Zhang, H. Liu, Z. Wang, M. Yi, N. Xie, Y. Shen, X. Ren, J. Wang and J. Wang, Adv. Healthcare Mater., 2022, 11, e2201524 CrossRef PubMed.
- J. Yang, W. Zeng, P. Xu, X. Fu, X. Yu, L. Chen, F. Leng, C. Yu and Z. Yang, Acta Biomater., 2022, 140, 206–218 CrossRef CAS PubMed.
- Z. Xu, G. Liu, P. Liu, Y. Hu, Y. Chen, Y. Fang, G. Sun, H. Huang and J. Wu, Acta Biomater., 2022, 147, 147–157 CrossRef CAS PubMed.
- P. S. Patil, S. Fathollahipour, A. Inmann, A. Pant, R. Amini, L. P. Shriver and N. D. Leipzig, Adv. Wound Care, 2019, 8, 374–385 CrossRef PubMed.
- Y. Pu, P. Wang, R. Yang, X. Tan, T. Shi, J. Ma, W. Xue and B. Chi, J. Mater. Chem. B, 2022, 10, 4083–4095 RSC.
- K. Xu, S. Deng, Y. Zhu, W. Yang, W. Chen, L. Huang, C. Zhang, M. Li, L. Ao, Y. Jiang, X. Wang and Q. Zhang, Adv. Healthcare Mater., 2023, 12, e2301370 CrossRef PubMed.
- Z. Lin, D. Fan, G. Li, L. He, X. Qin, B. Zhao, Q. Wang and W. Liang, Macromol. Biosci., 2023, 23, e2200349 CrossRef PubMed.
- J. Chen, J. He, Y. Yang, L. Qiao, J. Hu, J. Zhang and B. Guo, Acta Biomater., 2022, 146, 119–130 CrossRef CAS PubMed.
- Q. Ding, T. Sun, W. Su, X. Jing, B. Ye, Y. Su, L. Zeng, Y. Qu, X. Yang, Y. Wu, Z. Luo and X. Guo, Adv. Healthcare Mater., 2022, 11, e2102791 CrossRef PubMed.
- X. Liu, S. Zhou, B. Cai, Y. Wang, D. Deng and X. Wang, Biomater. Sci., 2022, 10, 3480–3492 RSC.
- B. Qin, S. Wu, H. Dong, S. Deng, Y. Liu, W. Zhang, G. Feng, L. Lei and H. Xie, ACS Appl. Mater. Interfaces, 2023, 15, 33207–33222 CrossRef CAS PubMed.
- S. Wang, H. Zheng, L. Zhou, F. Cheng, Z. Liu, H. Zhang, L. Wang and Q. Zhang, Nano Lett., 2020, 20, 5149–5158 CrossRef CAS PubMed.
- M. A. M. Fathil and H. Katas, Pharmaceutics, 2023, 15, 991 CrossRef CAS PubMed.
- S. Barman, S. Mukherjee, B. Bhattacharjee, K. De, R. Mukherjee and J. Haldar, Biomater. Sci., 2023, 11, 998–1012 RSC.
- J. Hu, S. Chen, Y. Yang, L. Li, X. Cheng, Y. Cheng and Q. Huang, Adv. Healthcare Mater., 2022, 11, e2200299 CrossRef PubMed.
- K. Cai, Y. Liu, Y. Yue, Y. Liu and F. Guo, Polymers, 2023, 15, 1376 CrossRef CAS PubMed.
- H. Willer, G. Spohn, K. Morgenroth, C. Thielemann, S. Elvers-Hornung, P. Bugert, B. Delorme, M. Giesen, T. Schmitz-Rixen, E. Seifried, C. Pfarrer, R. Schäfer and K. Bieback, Front. Immunol., 2022, 13, 976511 CrossRef CAS PubMed.
- K. Liu, M. Zhao, Y. Li, L. Luo and D. Hu, J. Biomater. Sci., Polym. Ed., 2022, 33, 569–589 CrossRef CAS PubMed.
- J. N. Huang, H. Cao, K. Y. Liang, L. P. Cui and Y. Li, World J. Diabetes, 2022, 13, 949–961 CrossRef PubMed.
- H. Lei, J. Zhao, H. Li and D. Fan, Carbohydr. Polym., 2022, 289, 119467 CrossRef CAS PubMed.
- L. C. Huang, H. C. Wang, L. H. Chen, C. Y. Ho, P. H. Hsieh, M. Y. Huang, H. C. Wu and T. W. Wang, Theranostics, 2019, 9, 7072–7087 CrossRef CAS PubMed.
- H. Zhu, J. Xu, M. Zhao, H. Luo, M. Lin, Y. Luo, Y. Li, H. He and J. Wu, Front. Bioeng. Biotechnol., 2022, 10, 968078 CrossRef PubMed.
- Y. Hao, W. Zhao, H. Zhang, W. Zheng and Q. Zhou, Carbohydr. Polym., 2022, 287, 119336 CrossRef CAS PubMed.
- N. Li, A. Zhan, Y. Jiang and H. Liu, Int. J. Biol. Macromol., 2022, 222, 1551–1559 CrossRef CAS PubMed.
- Z. Shao, T. Yin, J. Jiang, Y. He, T. Xiang and S. Zhou, Bioact. Mater., 2023, 20, 561–573 CAS.
- Y. Dong, M. Rodrigues, S. H. Kwon, X. Li, A. Sigen, E. A. Brett, N. Elvassore, W. Wang and G. C. Gurtner, Adv. Healthcare Mater., 2018, 7, e1800432 CrossRef PubMed.
- D. Gao, Y. Zhang, D. T. Bowers, W. Liu and M. Ma, APL Bioeng., 2021, 5, 031503 CrossRef CAS PubMed.
- S. Chen, J. Shi, M. Zhang, Y. Chen, X. Wang, L. Zhang, Z. Tian, Y. Yan, Q. Li, W. Zhong, M. Xing, L. Zhang and L. Zhang, Sci. Rep., 2015, 5, 18104 CrossRef CAS PubMed.
- Y. Ren, A. Aierken, L. Zhao, Z. Lin, J. Jiang, B. Li, J. Wang, J. Hua and Q. Tu, Carbohydr. Polym., 2022, 288, 119404 CrossRef CAS PubMed.
- M. Yang, S. He, Z. Su, Z. Yang, X. Liang and Y. Wu, ACS Omega, 2020, 5, 21015–21023 CrossRef CAS PubMed.
- K. C. Moon, H. S. Suh, K. B. Kim, S. K. Han, K. W. Young, J. W. Lee and M. H. Kim, Diabetes, 2019, 68, 837–846 CrossRef CAS PubMed.
- W. Sheng, Q. Song, X. Su, Y. Lu, Y. Bai, F. Ji, L. Zhang, R. Yang and X. Fu, J. Cosmet. Dermatol., 2023, 22, 1670–1679 CrossRef PubMed.
- Q. Shi, Z. Qian, D. Liu, J. Sun, X. Wang, H. Liu, J. Xu and X. Guo, Front. Physiol., 2017, 8, 904 CrossRef PubMed.
- A. J. M. Bailey, H. Li, A. M. Kirkham, A. Tieu, H. B. Maganti, R. Shorr, D. A. Fergusson, M. M. Lalu, H. Elomazzen and D. S. Allan, Stem Cell Rev. Rep., 2022, 18, 968–979 CrossRef PubMed.
- X. Liu, S. Tian, S. Xu, W. Lu, C. Zhong, Y. Long, Y. Ma, K. Yang, L. Zhang and J. Yang, Biosens. Bioelectron., 2022, 214, 114528 CrossRef CAS PubMed.
- Y. Yang, C. Zhang, M. Gong, Y. Zhan, Z. Yu, C. Shen, Y. Zhang, L. Yu and Z. Chen, Int. J. Biol. Macromol., 2023, 238, 124123 CrossRef CAS PubMed.
- Y. Chen, X. Hao, Z. Lu and D. Wang, ACS Appl. Mater. Interfaces, 2023, 15, 40255–40266 CrossRef CAS PubMed.
- Q. Pang, D. Lou, S. Li, G. Wang, B. Qiao, S. Dong, L. Ma, C. Gao and Z. Wu, Adv. Sci., 2020, 7, 1902673 CrossRef CAS PubMed.
- D. A. Jankowska, M. B. Bannwarth, C. Schulenburg, G. Faccio, K. Maniura-Weber, R. M. Rossi, L. Scherer, M. Richter and L. F. Boesel, Biosens. Bioelectron., 2017, 87, 312–319 CrossRef CAS PubMed.
- A. Wang, G. Fan, H. Qi, H. Li, C. Pang, Z. Zhu, S. Ji, H. Liang, B. P. Jiang and X. C. Shen, Biomaterials, 2022, 289, 121798 CrossRef CAS PubMed.
- J. Jiang, J. Ding, X. Wu, M. Zeng, Y. Tian, K. Wu, D. Wei, J. Sun, Z. Guo and H. Fan, J. Mater. Chem. B, 2023, 11, 4934–4945 RSC.
- Y. Zong, B. Zong, R. Zha, Y. Zhang, X. Li, Y. Wang, H. Fang, W. L. Wong and C. Li, Adv. Healthcare Mater., 2023, 12, e2300431 CrossRef PubMed.
- H. Liu, Z. Li, S. Che, Y. Feng, L. Guan, X. Yang, Y. Zhao, J. Wang, A. V. Zvyagin, B. Yang and Q. Lin, J. Mater. Chem. B, 2022, 10, 5804–5817 RSC.
- D. Li, X. Fei, L. Xu, Y. Wang, J. Tian and Y. Li, J. Colloid Interface Sci., 2022, 627, 942–955 CrossRef CAS PubMed.
- Q. Bai, K. Han, K. Dong, C. Zheng, Y. Zhang, Q. Long and T. Lu, Int. J. Nanomed., 2020, 15, 9717–9743 CrossRef CAS PubMed.
- T. Jiang, S. Liu, Z. Wu, Q. Li, S. Ren, J. Chen, X. Xu, C. Wang, C. Lu, X. Yang and Z. Chen, Mater. Today Bio, 2022, 16, 100365 CrossRef CAS PubMed.
- Y. Zhou, X. L. Zhang, S. T. Lu, N. Y. Zhang, H. J. Zhang, J. Zhang and J. Zhang, Stem Cell Res. Ther., 2022, 13, 407 CrossRef CAS PubMed.
- F. Tsegay, M. Elsherif and H. Butt, Polymers, 2022, 14, 1012 CrossRef CAS PubMed.
- N. Bolouki, Y. N. Hsu, Y. C. Hsiao, P. R. Jheng, J. H. Hsieh, H. L. Chen, B. W. Mansel, Y. Y. Yeh, Y. H. Chen, C. X. Lu, J. W. Lee and E. Y. Chuang, Int. J. Biol. Macromol., 2021, 192, 506–515 CrossRef CAS PubMed.
Footnote |
† These authors contributed equally to this work. |
|
This journal is © The Royal Society of Chemistry 2024 |
Click here to see how this site uses Cookies. View our privacy policy here.